- 1Vascular Biology Section, Boston University School of Medicine, Boston, MA, United States
- 2Department of Physiology, University of Murcia and Biomedical Research Institute in Murcia (IMIB), Murcia, Spain
Sirtuin-1 (SirT1) is a nicotinamide adenine dinucleotide-dependent deacetylase and the best characterized member of the sirtuins family in mammalians. Sirtuin-1 shuttles between the cytoplasm and the nucleus, where it deacetylates histones and non-histone proteins involved in a plethora of cellular processes, including survival, growth, metabolism, senescence, and stress resistance. In this brief review, we summarize the current knowledge on the anti-oxidant, anti-inflammatory, anti-apoptotic, and anti-senescence effects of SirT1 with an emphasis on vascular diseases. Specifically, we describe recent research advances on SirT1-mediated molecular mechanisms in aortic aneurysm (AA), and how these processes relate to oxidant stress and the heme-oxygenase (HO) system. HO-1 and HO-2 catalyze the rate-limiting step of cellular heme degradation and, similar to SirT1, HO-1 exerts beneficial effects in the vasculature through the activation of anti-oxidant, anti-inflammatory, anti-apoptotic, and anti-proliferative signaling pathways. SirT1 and HO-1 are part of an integrated system for cellular stress tolerance, and may positively interact to regulate vascular function. We further discuss sex differences in HO-1 and SirT1 activity or expression, and the potential interactions between the two proteins, in relation to the progression and severity of AA, as well as the ongoing efforts for translational applications of SirT1 activation and HO-1 induction in the treatment of cardiovascular diseases including AA.
Role of SirT1 in the Vasculature
Sirtuin-1 (SirT1, mammalian homolog of silent information regulator (Sir2) in yeast) is a nicotinamide adenine dinucleotide (NAD+)-dependent class III histone deacetylase (Frye, 2000) and the best characterized member of the sirtuins family in mammalians (Haigis and Sinclair, 2010). Compelling evidence have demonstrated beneficial effects of SirT1 in the cardiovascular system, generally attributed to anti-oxidant (Kawai et al., 2011; Zhou et al., 2011), anti-senescence (Ota et al., 2010a; Thompson et al., 2014; Chen et al., 2016), anti-apoptotic (Hibender et al., 2016; Hou et al., 2019), and anti-inflammatory effects (Sosnowska et al., 2017; D’Onofrio et al., 2018). In the vasculature, SirT1 is expressed in the endothelium, vascular smooth muscle (VSM) and adventitia (Potente et al., 2007; Miyazaki et al., 2008; Li et al., 2011; Fry et al., 2015; Chen et al., 2016; Ling et al., 2017). SirT1 is localized mostly in the nucleus where it regulates gene transcription, in a cell-specific manner, by deacetylating histone 3 (H3) and transcription factors, such as forkhead box O (FOXOs), nuclear factor kB (NF-kB), tumor protein 53 (p53), peroxisome proliferator-activated receptor-γ co-activator-1α (PPARγ), and the DNA repair protein Ku70 (Brunet et al., 2004; Michishita et al., 2005; Jeong et al., 2007; Chung et al., 2010; Zeng et al., 2013; Sosnowska et al., 2017). Specifically, activation of NF-kB and p53 promotes inflammation, apoptosis, senescence, and oxidant stress in animal models of aortic aneurysm (AA; Gomez et al., 2013; Leeper et al., 2013; Chen et al., 2016; Moran et al., 2017) while activation of FOXOs and PPARγ reverses these processes by opposing pro-inflammatory and apoptotic factors in animal and human studies (Jones et al., 2009; Oellerich and Potente, 2012; Radak et al., 2013; Motoki et al., 2015; Tai et al., 2016; Lu et al., 2020). In addition to gene transcription, SirT1 regulates the activity of several proteins, such as endothelial nitric oxide synthase (eNOS), which is activated to produce the vasoprotective mediator nitric oxide (NO), upon SirT1-mediated deacetylation of lysines 496 and 506 in the eNOS calmodulin-binding domain (Mattagajasingh et al., 2007). Conversely, NO is able to stimulate SirT1 expression and activity via a positive feedback mechanism, at least in the settings of statin- and cilostazol-induced SirT1 expression, which is prevented by the eNOS inhibitor L-NAME (Ota et al., 2008, 2010a,b; Man et al., 2019).
SirT1 is essential for VSM structural and functional homeostasis (Li et al., 2011; Gorenne et al., 2013; Fry et al., 2015; Chen et al., 2016). Physiological cyclic stretch promotes VSM contractile properties via SirT1/FOXO3a, thus maintaining vasoconstriction (Huang et al., 2015). Interestingly, lack of SirT1 in VSM-specific SirT1-ablated mice does not induce any vascular functional impairment per se (Fry et al., 2015). However, in response to pro-inflammatory and pro-oxidant stressors, such as angiotensin II (angII) infusion, arterial ligation, hyperlipidemic apoE–/– genetic background, aging and a diet rich in fat and sucrose, VSM SirT1 is absolutely essential to prevent maladaptive arterial remodeling that leads to vascular disease, such as atherosclerosis (Gorenne et al., 2013), aortic dissection (Fry et al., 2015), and arterial stiffness (Fry et al., 2016). Differential effects of SirT1 expression or activity levels in the vasculature are uniquely dependent on disease type, stage and interacting factors and thoroughly reviewed elsewhere (D’Onofrio et al., 2018; Man et al., 2019). In this review, we will focus on yet another role of SirT1 in vascular disease, namely aortic aneurysm, which only recently started to be appreciated.
SirT1 in Aortic Aneurysm
Aortic aneurysms are abnormal aortic enlargements which can develop in the thoracic (TAA) or abdominal (AAA) regions. Although the pathogenesis behind different forms of TAA and AAA may differ greatly, a combination of genetic predisposition and hypertension, particularly in Marfan’s and related syndromes, generally contribute to the development of TAA prone to dissections, while smoking, male gender and diabetes are the major risk factors for AAA prone to ruptures. Overall, the most troublesome aspect of AA (TAA and AAA), particularly in non-syndromic and idiopathic forms, is that they often remain clinically undiagnosed until the aortic wall dissects or ruptures, potentially causing sudden death (Davies, 1998; Mody et al., 2014). Currently, treatment options against these potentially lethal vascular conditions are limited to blood pressure control and surgical repair, for which the risk of mortality remains high at 30–50%, depending on the repair method employed (Virani et al., 2020); therefore, novel therapeutic targets are urgently needed. We have recently shown that mice with VSM-specific deletion of SirT1 have a drastically increased mortality (70%) in response to angII infusion due to aortic wall dissection, particularly in the thoracic region, which resulted from excess oxidant production and oxidant-stimulated matrix metalloproteinases (MMPs) activation (Fry et al., 2015). Consistent with these findings, VSM-specific overexpression of SirT1 protects aged mice on apoE-deficient genetic background, against angII-induced AAA and rupture by opposing vascular senescence and inflammation (Chen et al., 2016). Moreover, calorie restriction, which is known to activate SirT1 (Guarente, 2013), prevents angII-induced AAA through SirT1-dependent deacetylation of H3 at lysine 9 on the MMP2 gene promoter, which downregulates MMP2 and subsequent elastin fragmentation in the aortic wall (Liu et al., 2016).
Furthermore, endothelial SirT1 has been shown to counteract the deleterious effects of angII on AA formation, believed to be driven mainly by endothelial, but not VSM, angII type 1α receptors (ATR1α) (Rateri et al., 2011). In a model of Marfan’s syndrome (fibrillin-1 mutant mice, Fbn1mgR/mgR), deletion of endothelial ATR1α decreased the incidence of TAA (Galatioto et al., 2018). Since angII administration is known to downregulate SirT1 expression and activity in aortic endothelial cells (Marampon et al., 2013), these findings suggest that angII could accelerate the development of TAA in renin-dependent hypertensive Marfan individuals by suppressing SirT1/eNOS and impairing endothelial function.
It is worth mentioning that SirT1 in hematopoietic cells has recently emerged as an important mediator of AA formation. Macrophage-specific deficiency of SirT1 increased the incidence and exacerbated disease severity in a mouse model of angII-induced AAA, by increasing the pro-inflammatory inducible nitric oxide synthase, a marker of M1 macrophages, while decreasing arginase and mannose receptor, two markers of M2 macrophages. On the contrary, SirT1 overexpression in macrophages, achieved by adenoviral transfection, had an opposite effect (Zhang et al., 2018). Overall, multiple studies indicate that SirT1 in variety of vascular cells is essential for the maintenance of vascular wall integrity and to prevent AA.
SirT1 and Oxidative Stress in Aortic Aneurysm
An imbalance between the production of reactive oxygen species (ROS) and the cellular anti-oxidant systems, defined as oxidant stress, is a hallmark of vascular diseases, including atherosclerosis and diabetic endothelial dysfunction (Brown and Griendling, 2015; Quintana and Taylor, 2019). A role of oxidant stress in the development and progression of AA has been described recently (Quintana and Taylor, 2019). In human and animal studies, NADPH oxidase (Nox) has been identified as a major source of ROS contributing to the development of AA (Miller et al., 2002; Yang et al., 2010; Jimenez-Altayo et al., 2018; van der Pluijm et al., 2018). Ablation of Nox1, Nox2, Nox4 isoforms or of the non-catalytic Nox subunit p47 in mice prevented the development of AAA (Lu et al., 2016; Siu et al., 2017). This is explained by the fact that Nox-derived ROS are important second messengers in the vasculature, however, excessive ROS lead to increase MMPs activation as well as inflammatory and apoptotic factors, which contribute to the pathogenesis of AAA (Finkel, 2011; Miki and Funato, 2012; Holmstrom and Finkel, 2014; Brown and Griendling, 2015; Forrester et al., 2018; Quintana and Taylor, 2019). Similarly, the expression of anti-oxidant enzymes such as superoxide dismutase, glutathione peroxidase, glutathione reductase, and glutathione S-transferase decreases in aortae of AAA and TAA patients (Dubick et al., 1999; Liao et al., 2008; Zuniga-Munoz et al., 2017).
Endothelial and VSM SirT1 are known to regulate the cellular redox state in the vascular wall by multiple mechanisms, including direct deacetylation of FOXOs, NF-kB, Nrf2, mitochondrial superoxide dismutase and Nox, which overall decrease ROS production (Fry et al., 2015; Huang et al., 2015; Zhang et al., 2017). Moreover, SirT1 inhibits the mitochondrial adaptor protein p66Shc, a critical modulator of intracellular redox state and a major contributor of oxidative damage-induced endothelial dysfunction in the settings of diabetes (Zhou et al., 2011; Paneni et al., 2013), by directly deacetylating its lysine 81 (Kumar et al., 2017). Taken together, these well-established anti-oxidant effects of SirT1 are consistent with the findings that VSM-specific SirT1 deletions in mice, and decreased expression of SirT1 in human aorta, are associated with aortic dissection or aneurysms (Fry et al., 2015; Chen et al., 2016).
Interestingly, SirT1 itself is a redox-sensitive enzyme. We have shown that SirT1 oxidative post-translational modifications, namely S-glutathionylation, at cysteine residues of its catalytic domain, profoundly affects its enzymatic activity (Zee et al., 2010; Shao et al., 2014). Reversible oxidative post-translational modifications, such as S-glutathionylation, result in activation or inactivation of proteins, thereby regulating signaling cascades and preventing irreversible oxidation of protein thiols in the settings of oxidative stress (Cohen et al., 2016; Byrne et al., 2020). Consistent with our findings, redox factor-1 and apurinic/apyrimidinic endonuclease-1, two cellular reducing agents, are able to restore NO bioavailability and endothelium-dependent vasorelaxation, through the reduction of conserved cysteine sulfhydryls in the SirT1 deacetylase domain (Jung et al., 2013). The loss of this fine-tuned mechanism in human endothelial cells exposed to oxidant stress, such as after exposure to cigarette smoke or hydrogen peroxide, have been associated with endothelial dysfunction (Chung et al., 2010). More recently, we found that H2O2, as well as TGF-β1, a pro-fibrotic cytokine known to be activated in Marfan’s syndrome and to upregulate Nox4 in the vasculature (Lu et al., 2016; Siu et al., 2017; Zuniga-Munoz et al., 2017; Jimenez-Altayo et al., 2018), increases the reversible oxidation of SirT1 in aortic VSM cells (E. Budbazar and F. Seta, unpublished results). Therefore, an impairment of SirT1 activity by oxidative post-translational modifications may be causally linked to the development of AA, at least in individuals with Marfan’s syndrome, possibly by exacerbating the deleterious effects of oxidant stress, whereas preventing or boosting SirT1 activity in the aortic wall may prevent AA.
SirT1 and HO-1 in Aortic Aneurysm
Heme oxygenase-1 (HO-1) and 2 (HO-2) catalyze the rate-limiting step of the cellular degradation of heme. In the presence of oxygen and cofactors, HO-1 and HO-2 metabolize heme into carbon monoxide (CO), free iron and biliverdin, subsequently converted to bilirubin (BR; Abraham and Kappas, 2005). Both HO-1 and HO-2 isoforms are catalytically active in the vasculature (Thorup et al., 1999; Zhang et al., 2001), as demonstrated by HO-dependent release of CO in rat aorta, renal and cerebral arteries, and gracilis muscle arterioles (Kaide et al., 2001; Zhang et al., 2001).
HO-1 and HO-2 regulate vascular tone and hemodynamic function, mainly through CO production (Kaide et al., 2001, 2004; Zhang et al., 2001; Rodriguez et al., 2003; Arregui et al., 2004). We and others have shown that HO inhibition causes vasoconstriction (Kaide et al., 2001, 2004; Zhang et al., 2001; Rodriguez et al., 2004) while CO generally promotes vasodilation (Kaide et al., 2001, 2004; Arregui et al., 2004; Rodriguez et al., 2011). The vasoconstrictor effects associated with HO inhibitors are greater after NO synthesis inhibition (Zhang et al., 2001; Rodriguez et al., 2003), linked in part, to amplification of prevailing neurohormonal constrictor mechanisms (Rodriguez et al., 2003). Importantly, NO synthesis inhibition elevates CO formation both in vivo and in vitro (Rodriguez et al., 2004). Therefore, the significance of the HO–CO system might be particularly relevant in the settings of reduced NO bioavailability, as it is the case for conditions associated with increased oxidant stress and reduced NO levels, including numerous vascular and renal diseases (Salom et al., 2007; Rodriguez et al., 2011; Bonacasa et al., 2013).
HO-1 expression, but not HO-2, is increased in cultured VSM and endothelial cells in response to various stress stimuli (Christodoulides et al., 1995). Similar to SirT1, HO-1 overexpression serves a protective role by virtue of anti-oxidant (Ferrandiz and Devesa, 2008; Bonacasa et al., 2013), anti-inflammatory (Lee et al., 2004), anti-apoptotic (Ferrandiz and Devesa, 2008), and anti-proliferative (Deng et al., 2004; Lee et al., 2004) effects in endothelial, smooth muscle cells and macrophages in the vascular wall (Kim et al., 2011), by increasing CO and/or biliverdin production or by reducing the pro-oxidant heme levels (Abraham and Kappas, 2005; Duvigneau et al., 2019). Like SirT1, HO-1 confers protection in several vascular injury models, such us ischemic heart disease, atherosclerosis, hypertension, diabetes, or vascular proliferative diseases (Abraham and Kappas, 2005; Loboda et al., 2008; Kim et al., 2011). Interestingly, single nucleotide polymorphisms in the HO-1 promoter region, which results in a decreased ability to upregulate HO-1, has been linked to AA disease risk in humans (Schillinger et al., 2002). HO-1 deficiency in mice exacerbates the development of AA (Azuma et al., 2016; Ho et al., 2016), whereas HO-1 overexpression, induced by heme treatment (Azuma et al., 2016) or shear stress (Nakahashi et al., 2002), attenuated AA progression by opposing inflammation (Azuma et al., 2016; Ho et al., 2016) and oxidative stress (Nakahashi et al., 2002; Ho et al., 2016).
Overall, HO-1 and SirT1 are part of the integrated system that modulates the cellular response to stress and might positively interact to regulate cardiovascular function. Treatment with cobalt protoporphyrin, a HO-1 chemical inducer (Sodhi et al., 2015), or HO-1 overexpression in macrophages (Nakamura et al., 2018), consistently enhanced SirT1 expression. Conversely, SirT1 activity can modulate HO-1 biological effects in hepatic cells (Sodhi et al., 2015; Nakamura et al., 2018), adypocites (Lakhani et al., 2019), or myocardial tissue (Yu et al., 2019). Moreover, in a human model of endogenous AT1R antagonism, SirT1 directly associates with improved NO-dependent vasodilation via HO-1 (Davis et al., 2013). These interactions suggest that positive feedback mechanisms between SirT1 and HO-1 might be at play in the vasculature, and they may affect HO-1-mediated cytoprotection in cardiovascular diseases. To this end, pharmacological activation of miR-181b/SirT1/HO-1 signaling axis reduced the development of ang II-induced AAA in apoE-/- mice (Hou et al., 2019). Although the exact molecular mechanisms accounting for the interaction between SirT-1 and HO-1, and its functional significance in vascular pathology, remain to be fully elucidated, the development of specific targeted therapies that modulate the SirT1/HO-1 axis could represent a new therapeutic strategy for the management of AA disease, as well as other vascular diseases.
Sex Differences, Oxidative Stress, and Susceptibility to AA
Aortic aneurysm is more likely to occur in men than in women (Singh et al., 2001) but women have a greater risk of AA rupture and poorer outcome than men. Several factors may contribute to sex-specific susceptibility of male to AA namely differences in mechanical properties of the aorta, levels of aortic wall MMPs, renin angiotensin system activity, or inflammatory and immune responses, which might be influenced by sex hormones, as thoroughly reviewed elsewhere (Boese et al., 2018; Robinet et al., 2018). Interestingly, to dissociate the effects of sex hormones from sex chromosome, Alsiraj et al. (2017) used the four core genotype mouse model to create XX and XY female offspring, showing that XY phenotypic females had increased AA incidence and severity compared with XX females, through increased inflammation and oxidative stress. Moreover, castration prevented the progression of disease in a model of angII-induced AA (Zhang et al., 2015). Since estrogen and testosterone also differently influence redox balance (Boese et al., 2018), the higher susceptibility of male to oxidative stress and AA in animal studies, might be related to either the effects of sex hormones or sex chromosomes genes on oxidant pathways.
Increased activation of Nox and ROS production are known to be higher (Dantas et al., 2004), while the antioxidant capacity is decreased (Rodriguez et al., 2010; Bonacasa et al., 2013), in males compared to females in preclinical and clinical studies (Sartori-Valinotti et al., 2007). Estrogen increases HO-1 expression in cultured endothelial cells (Baruscotti et al., 2010; Marcantoni et al., 2012) and the uterus of ovariectomized animals (Cella et al., 2006). In contrast, HO-1 expression is higher in females compared to males in several experimental models of cardiovascular disease (Zampino et al., 2006; Bonacasa et al., 2013). Noteworthy, our own work and others’, showed that increased HO-1 expression and/or activity in female rats was associated with decreased nitrosative stress and glomerular damage in the diabetic kidney (Bonacasa et al., 2013) and a lower susceptibility to cardiac ischemia compared to males (Posa et al., 2013). In line with this notion, increased HO-1 expression and activity in the female offspring of diabetic mothers, was associated with lower nitrotyrosine levels, and improved cardiovascular function in females compared to males (F. Rodriguez and J. M. Sanchez, unpublished results). Therefore, antioxidant HO-1 activity could improve vascular function partly by compensating for the loss of NO bioavailability, in vascular diseases, particularly in females.
Similarly to HO-1, SirT1 activity may be influenced by sex or sex hormones. Estrogen induces SirT1 and SirT1/AMPK/histone H3 pathway, which relates to the cardiovascular protective effects of estrogen therapy (Bendale et al., 2013). Moreover, SirT-1 downregulation in the female, but not male, hearts directly correlated with a decline in mitochondrial anti-oxidative defense and a pro-inflammatory shift (Barcena de Arellano et al., 2019). Consistently, sex-specific downregulation of the SirT1/AMPK/FOXO3a/PGC-1α regulatory network was observed in male, but not female offspring, in response to maternal high-fat feeding (Nguyen et al., 2017). Overall, the possibility of a sex-specific regulation of SirT1 and HO-1 in the context of oxidative stress, such as in AA, is intriguing. Further studies addressing the functional significance of sex specific changes in SirT1 and HO-1 in AA, and the correlation with clinical features are warranted.
Translational Therapeutic Applications of SirT1 or HO Activation for AA
Substantial R&D has been invested in the quest of compounds that could boost SirT1 activity for the treatment of cardiovascular diseases. Thus far, numerous plant-derived molecules have shown promising results, although proving their pharmacological specificity remains a challenge. Liquorice-derived licochalcone attenuates angII-induced AAA by modulating the miR-181b/SirT1/HO-1 signaling axis (Hou et al., 2019). Resveratrol, a grape-derived non-toxic polyphenol known to activate SirT1, decreases the incidence of AAA in angII-infused apoE–/– mice fed a high fat diet by increasing ACE2 and downregulating NF-kB, Akt, ERK1/2 and ATR1 in human aortic VSM (Moran et al., 2017). Similarly, resveratrol, but not another putative SirT1 agonist SRT1720, prevented the development of TAA in Fbn1C1041G/+ mice, a model of Marfan’s syndrome, by stimulating an endothelial cell-derived factor, which downregulated miR-29b and upregulated Bcl-2 in VSM cells, inhibiting their apoptosis (Hibender et al., 2016). However, the beneficial effects of resveratrol and other putative SirT1-specific agonists, such as SRT1720 and SRT2104, are attributed to both SirT1-dependent and -independent mechanisms (Park et al., 2012; Zordoky et al., 2015; Bonnefont-Rousselot, 2016; Hibender et al., 2016; Moran et al., 2017; van Andel et al., 2019). It is well established that resveratrol can activate AMPK, inhibit cyclooxygenases and affect a variety of other enzymes (Bonnefont-Rousselot, 2016; Wicinski et al., 2018). Likewise, novel SirT1 activators, which were effective in decreasing arterial stiffness and improving the lipid profile of healthy cigarette smokers and elderly volunteers in clinical trials (Libri et al., 2012; Venkatasubramanian et al., 2013, 2016), function as partial agonists of SirT1 or exhibit off-target effects (Pacholec et al., 2010). Therefore, attempts to develop alternative approaches to activate SirT1, rather than direct agonists, are an active field of research. Manipulating the metabolism of NAD+, the essential co-factor for SirT1 deacetylase activity, has proven very promising, as in some cases, it recapitulates the effects of resveratrol (Baur, 2010). Both niacin and nicotinamide supplementation increases NAD+ levels and NAD+-dependent SirT1 activity in aortas and prevents the development of AAA in mice (Horimatsu et al., 2019). Similarly, nicotinamide phosphoribosyltransferase (NAMPT), also known as visfatin, a methyltransferase crucial for the synthesis of the NAD+ precursor nicotinamide mononucleotide (Galli et al., 2013), has been shown to maintain aortic VSM integrity through NAD/SirT1 pathway (Watson et al., 2017). Additionally, we propose that understanding the role of oxidative post-translational modifications, such as S-glutathionylation of SirT1 cysteine residues, may lead to novel therapeutic strategies (Figure 1). We previously shown that a redox-resistant mutant SirT1 and overexpression of glutaredoxin-1, a thioltransferase that removes S-glutathionylation on SirT1, protects against liver metabolic disease (Shao et al., 2014, 2017). Whether a similar approach could be implemented in the clinic to treat AA and other cardiovascular diseases warrants further research.
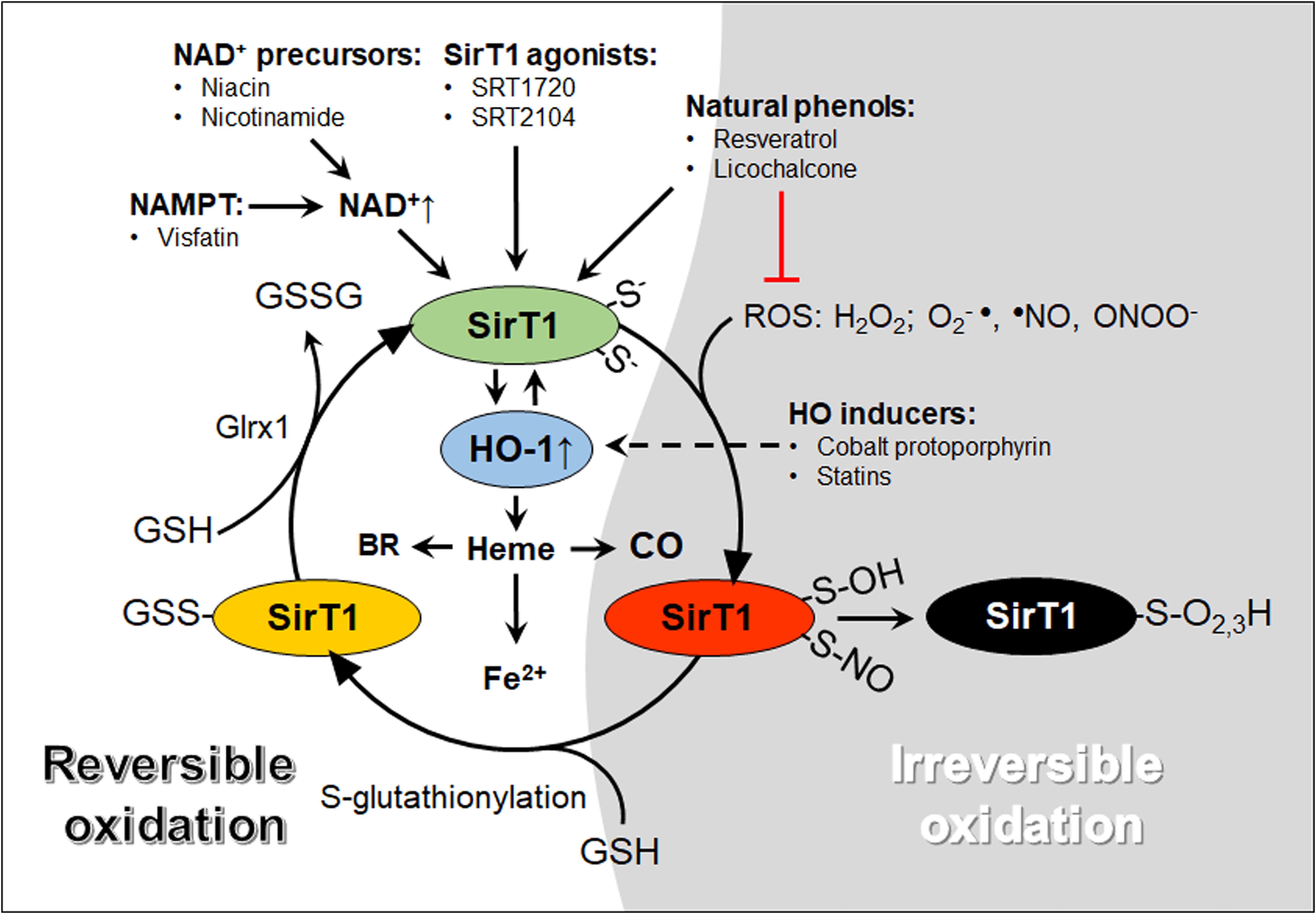
Figure 1. A graphical abstract of oxidative modifications of cysteine thiols and pharmacological agents that increase SirT1 and HO-1 activity. The reactive thiolate anions of SirT1 exposed to ROS can react to form reversible (red and yellow, temporary inactivation) or irreversible (black, permanent inactivation). Glutathione (GSH) adducts added by S-glutathionylation inactivate SirT1. SirT1-GSH adducts may be reversed by glutaredoxin-1 (Glrx1), forming oxidized GSH (GSSG) and active SirT1 (green). HO-1 may interact with SirT1 through positive feedback mechanisms and metabolize heme into carbon monoxide (CO), free iron (Fe2+) and biliverdin, subsequently converted to bilirubin (BR). NAD+, nicotinamide adenine dinucleotide; NAMPT, nicotinamide phosphoribosyltransferase; ROS, reactive oxygen species.
Lipid-lowering agents widely used in clinical settings, such as probucol or statins, showed anti-proliferative effects on VSM through HO-1 induction (Deng et al., 2004; Lee et al., 2004), and decreased the development of experimental AA by inducing HO-1 gene expression (Azuma et al., 2016; Piechota-Polanczyk et al., 2018; Chen et al., 2020). Likewise, simvastatin-treated patients with AA, showed higher levels of anti-oxidant HO-1 in vascular tissue (Piechota-Polanczyk et al., 2018). Taken together, these findings strongly support a key protective role of HO-1 in limiting AA progression, and that statins and probucol may limit AA progression through mechanisms involving HO-1, probably independently of their lipid-lowering effects.
In contrast, it is important to consider that deleterious effects of HO-1 (Jais et al., 2014) and SirT1 overexpression (Kawashima et al., 2011) have been reported. In the case of HO-1 induction, these deleterious effects relate to increased reactive free iron (Suttner and Dennery, 1999) or reduced heme availability (Duvigneau et al., 2019). Interestingly, it has been suggested that there is a beneficial threshold of HO-1 overexpression (Suttner and Dennery, 1999; Namba et al., 2014) operating in specific subcellular localizations (Namba et al., 2014). Therefore, attempts at targeting HO-1 enzymatic activity to treat AA, as well as other vascular diseases, should aim at generating sufficient amounts of HO-1 activity, in specific cell compartments, maintaining suitable levels of intracellular heme and heme derived products formed by HO, avoiding any undesirable effects. Similarly, SirT1 activation or overexpression should be titrated to avoid unwanted effects.
Overall, HO-1 and SirT1 are part of gene complexes termed vitagenes (Calabrese et al., 2014), which provide an integrated response to control oxidant stress-induced tissue injury. Vitagenes products (i.e., heat shock proteins, BR, CO, gluthathione) mediate anti-oxidant, anti-apoptotic, anti-proliferative and anti-inflammatory actions (Abraham and Kappas, 2005; Calabrese et al., 2014). Dietary anti-oxidants (Abraham and Kappas, 2005; Moran et al., 2017; Hou et al., 2019) and clinically widely used compounds, such us statins, have shown favorable pleiotropic effects partly mediated by HO-1 induction (Lee et al., 2004; Piechota-Polanczyk et al., 2018) or SirT1 expression (Strycharz et al., 2018), providing a strong rationale for their therapeutic benefits in cardiovascular diseases, including AA (Hou et al., 2019). Therefore, the importance of developing pharmacological agents that activate an integrated response against oxidant stress in AA seems evident. Still, there is no definitive evidence from large scale clinical studies with anti-oxidant supplementation (Egea et al., 2017), requiring a better understanding of how each compound would affect the sources of ROS in specific cell compartments, modulate NAD + levels (Horimatsu et al., 2019), or affect ROS-induced reversible modifications, such as protein S-glutathionylation (Shao et al., 2014, 2017), for the suitable translation into clinical settings.
Author Contributions
EB wrote and reviewed the manuscript, and prepared the figure. FR and FS wrote and reviewed the manuscript. JS reviewed the manuscript. All authors contributed to the article and approved the submitted version.
Funding
This work was supported by grants 12009/PI/09 (Fundación Séneca) and BFV 2006-06998 (Ministerio de Educación y Ciencia) to FR and NIH R01 HL136311 to FS.
Conflict of Interest
The authors declare that the research was conducted in the absence of any commercial or financial relationships that could be construed as a potential conflict of interest.
References
Abraham, N. G., and Kappas, A. (2005). Heme oxygenase and the cardiovascular-renal system. Free Radic. Biol. Med. 39, 1–25. doi: 10.1016/j.freeradbiomed.2005.03.010
Alsiraj, Y., Thatcher, S. E., Charnigo, R., Chen, K., Blalock, E., Daugherty, A., et al. (2017). Female mice with an xy sex chromosome complement develop severe angiotensin ii-induced abdominal aortic aneurysms. Circulation 135, 379–391. doi: 10.1161/circulationaha.116.023789
Arregui, B., Lopez, B., Garcia Salom, M., Valero, F., Navarro, C., and Fenoy, F. J. (2004). Acute renal hemodynamic effects of dimanganese decacarbonyl and cobalt protoporphyrin. Kidney Int. 65, 564–574. doi: 10.1111/j.1523-1755.2004.00409.x
Azuma, J., Wong, R. J., Morisawa, T., Hsu, M., Maegdefessel, L., Zhao, H., et al. (2016). Heme oxygenase-1 expression affects murine abdominal aortic aneurysm progression. PLoS One 11:e0149288. doi: 10.1371/journal.pone.0149288
Barcena de Arellano, M. L., Pozdniakova, S., Kuhl, A. A., Baczko, I., Ladilov, Y., and Regitz-Zagrosek, V. (2019). Sex differences in the aging human heart: decreased sirtuins, pro-inflammatory shift and reduced anti-oxidative defense. Aging 11, 1918–1933. doi: 10.18632/aging.101881
Baruscotti, I., Barchiesi, F., Jackson, E. K., Imthurn, B., Stiller, R., Kim, J. H., et al. (2010). Estradiol stimulates capillary formation by human endothelial progenitor cells: role of estrogen receptor-{alpha}/{beta}, heme oxygenase 1, and tyrosine kinase. Hypertension 56, 397–404. doi: 10.1161/hypertensionaha.110.153262
Baur, J. A. (2010). Biochemical effects of sirt1 activators. Biochim. Biophys. Acta 1804, 1626–1634. doi: 10.1016/j.bbapap.2009.10.025
Bendale, D. S., Karpe, P. A., Chhabra, R., Shete, S. P., Shah, H., and Tikoo, K. (2013). 17-beta oestradiol prevents cardiovascular dysfunction in post-menopausal metabolic syndrome by affecting sirt1/ampk/h3 acetylation. Br. J. Pharmacol. 170, 779–795. doi: 10.1111/bph.12290
Boese, A. C., Chang, L., Yin, K. J., Chen, Y. E., Lee, J. P., and Hamblin, M. H. (2018). Sex differences in abdominal aortic aneurysms. Am. J. Physiol. Heart Circ. Physiol. 314, H1137–H1152. doi: 10.1152/ajpheart.00519.2017
Bonacasa, B., Perez, C., Salom, M. G., Lopez, B., Saez-Belmonte, F., Martinez, P., et al. (2013). Sexual dimorphism in renal heme-heme oxygenase system in the streptozotocin diabetic rats. Curr. Pharm. Des. 19, 2678–2686. doi: 10.2174/1381612811319150002
Bonnefont-Rousselot, D. (2016). Resveratrol and cardiovascular diseases. Nutrients 8:250. doi: 10.3390/nu8050250
Brown, D. I., and Griendling, K. K. (2015). Regulation of signal transduction by reactive oxygen species in the cardiovascular system. Circ. Res. 116, 531–549. doi: 10.1161/circresaha.116.303584
Brunet, A., Sweeney, L. B., Sturgill, J. F., Chua, K. F., Greer, P. L., Lin, Y., et al. (2004). Stress-dependent regulation of foxo transcription factors by the sirt1 deacetylase. Science 303, 2011–2015. doi: 10.1126/science.1094637
Byrne, D. P., Shrestha, S., Galler, M., Cao, M., Daly, L. A., Campbell, A. E., et al. (2020). Aurora A regulation by reversible cysteine oxidation reveals evolutionarily conserved redox control of Ser/Thr protein kinase activity. Sci. Signal 13:eaax2713. doi: 10.1126/scisignal.aax2713
Calabrese, V., Scapagnini, G., Davinelli, S., Koverech, G., Koverech, A., De Pasquale, C., et al. (2014). Sex hormonal regulation and hormesis in aging and longevity: role of vitagenes. J. Cell Commun. Signal. 8, 369–384. doi: 10.1007/s12079-014-0253-7
Cella, M., Farina, M. G., Keller Sarmiento, M. I., Chianelli, M., Rosenstein, R. E., and Franchi, A. M. (2006). Heme oxygenase-carbon monoxide (ho-co) system in rat uterus: effect of sexual steroids and prostaglandins. J. Steroid Biochem. Mol. Biol. 99, 59–66. doi: 10.1016/j.jsbmb.2005.11.007
Chen, C., Wang, Y., Cao, Y., Wang, Q., Anwaier, G., Zhang, Q., et al. (2020). Mechanisms underlying the inhibitory effects of probucol on elastase-induced abdominal aortic aneurysm in mice. Br. J. Pharmacol. 177, 204–216. doi: 10.1111/bph.14857
Chen, H. Z., Wang, F., Gao, P., Pei, J. F., Liu, Y., Xu, T. T., et al. (2016). Age-associated sirtuin 1 reduction in vascular smooth muscle links vascular senescence and inflammation to abdominal aortic aneurysm. Circ. Res. 119, 1076–1088. doi: 10.1161/circresaha.116.308895
Christodoulides, N., Durante, W., Kroll, M. H., and Schafer, A. I. (1995). Vascular smooth muscle cell heme oxygenases generate guanylyl cyclase-stimulatory carbon monoxide. Circulation 91, 2306–2309. doi: 10.1161/01.cir.91.9.2306
Chung, S., Yao, H., Caito, S., Hwang, J. W., Arunachalam, G., and Rahman, I. (2010). Regulation of sirt1 in cellular functions: role of polyphenols. Arch. Biochem. Biophys. 501, 79–90. doi: 10.1016/j.abb.2010.05.003
Cohen, R. A., Murdoch, C. E., Watanabe, Y., Bolotina, V. M., Evangelista, A. M., Haeussler, D. J., et al. (2016). Endothelial cell redox regulation of ischemic angiogenesis. J. Cardiovasc. Pharmacol. 67, 458–464. doi: 10.1097/fjc.0000000000000381
Dantas, A. P., Franco Mdo, C., Silva-Antonialli, M. M., Tostes, R. C., Fortes, Z. B., Nigro, D., et al. (2004). Gender differences in superoxide generation in microvessels of hypertensive rats: role of nad(p)h-oxidase. Cardiovasc. Res. 61, 22–29. doi: 10.1016/j.cardiores.2003.10.010
Davies, M. J. (1998). Aortic aneurysm formation: lessons from human studies and experimental models. Circulation 98, 193–195. doi: 10.1161/01.cir.98.3.193
Davis, P. A., Pagnin, E., Dal Maso, L., Caielli, P., Maiolino, G., Fusaro, M., et al. (2013). Sirt1, heme oxygenase-1 and no-mediated vasodilation in a human model of endogenous angiotensin ii type 1 receptor antagonism: implications for hypertension. Hypertens. Res. 36, 873–878. doi: 10.1038/hr.2013.48
Deng, Y. M., Wu, B. J., Witting, P. K., and Stocker, R. (2004). Probucol protects against smooth muscle cell proliferation by upregulating heme oxygenase-1. Circulation 110, 1855–1860. doi: 10.1161/01.cir.0000142610.10530.25
D’Onofrio, N., Servillo, L., and Balestrieri, M. L. (2018). Sirt1 and sirt6 signaling pathways in cardiovascular disease protection. Antioxid. Redox Signal. 28, 711–732. doi: 10.1089/ars.2017.7178
Dubick, M. A., Keen, C. L., DiSilvestro, R. A., Eskelson, C. D., Ireton, J., and Hunter, G. C. (1999). Antioxidant enzyme activity in human abdominal aortic aneurysmal and occlusive disease. Proc. Soc. Exp. Biol. Med. Soc. Exp. Biol. Med. 220, 39–45. doi: 10.1046/j.1525-1373.1999.d01-6.x
Duvigneau, J. C., Esterbauer, H., and Kozlov, A. V. (2019). Role of heme oxygenase as a modulator of heme-mediated pathways. Antioxidants 8:475. doi: 10.3390/antiox8100475
Egea, J., Fabregat, I., Frapart, Y. M., Ghezzi, P., Gorlach, A., Kietzmann, T., et al. (2017). European contribution to the study of ros: a summary of the findings and prospects for the future from the cost action bm1203 (eu-ros). Redox Biol. 13, 94–162.
Ferrandiz, M. L., and Devesa, I. (2008). Inducers of heme oxygenase-1. Curr. Pharm. Des. 14, 473–486. doi: 10.2174/138161208783597399
Finkel, T. (2011). Signal transduction by reactive oxygen species. J. Cell Biol. 194, 7–15. doi: 10.1083/jcb.201102095
Forrester, S. J., Kikuchi, D. S., Hernandes, M. S., Xu, Q., and Griendling, K. K. (2018). Reactive oxygen species in metabolic and inflammatory signaling. Circ. Res. 122, 877–902. doi: 10.1161/circresaha.117.311401
Fry, J. L., Al Sayah, L., Weisbrod, R. M., Van Roy, I., Weng, X., Cohen, R. A., et al. (2016). Vascular smooth muscle sirtuin-1 protects against diet-induced aortic stiffness. Hypertension 68, 775–784. doi: 10.1161/hypertensionaha.116.07622
Fry, J. L., Shiraishi, Y., Turcotte, R., Yu, X., Gao, Y. Z., Akiki, R., et al. (2015). Vascular smooth muscle sirtuin-1 protects against aortic dissection during angiotensin ii-induced hypertension. J. Am. Heart Assoc. 4:e002384. doi: 10.1161/JAHA.115.002384
Frye, R. A. (2000). Phylogenetic classification of prokaryotic and eukaryotic sir2-like proteins. Biochem. Biophys. Res. Commun. 273, 793–798. doi: 10.1006/bbrc.2000.3000
Galatioto, J., Caescu, C. I., Hansen, J., Cook, J. R., Miramontes, I., Iyengar, R., et al. (2018). Cell type-specific contributions of the angiotensin ii type 1a receptor to aorta homeostasis and aneurysmal disease-brief report. Arterioscler. Thromb. Vasc. Biol. 38, 588–591. doi: 10.1161/atvbaha.117.310609
Galli, U., Travelli, C., Massarotti, A., Fakhfouri, G., Rahimian, R., Tron, G. C., et al. (2013). Medicinal chemistry of nicotinamide phosphoribosyltransferase (nampt) inhibitors. J. Med. Chem. 56, 6279–6296. doi: 10.1021/jm4001049
Gomez, D., Kessler, K., Michel, J. B., and Vranckx, R. (2013). Modifications of chromatin dynamics control smad2 pathway activation in aneurysmal smooth muscle cells. Circ. Res. 113, 881–890. doi: 10.1161/circresaha.113.301989
Gorenne, I., Kumar, S., Gray, K., Figg, N., Yu, H., Mercer, J., et al. (2013). Vascular smooth muscle cell sirtuin 1 protects against DNA damage and inhibits atherosclerosis. Circulation 127, 386–396. doi: 10.1161/circulationaha.112.124404
Guarente, L. (2013). Calorie restriction and sirtuins revisited. Genes Dev. 27, 2072–2085. doi: 10.1101/gad.227439.113
Haigis, M. C., and Sinclair, D. A. (2010). Mammalian sirtuins: biological insights and disease relevance. Ann. Rev. Pathol. 5, 253–295. doi: 10.1146/annurev.pathol.4.110807.092250
Hibender, S., Franken, R., van Roomen, C., Ter Braake, A., van der Made, I., Schermer, E. E., et al. (2016). Resveratrol inhibits aortic root dilatation in the fbn1c1039g/+ marfan mouse model. Arterioscler. Thromb. Vasc. Biol. 36, 1618–1626. doi: 10.1161/atvbaha.116.307841
Ho, Y. C., Wu, M. L., Gung, P. Y., Chen, C. H., Kuo, C. C., and Yet, S. F. (2016). Heme oxygenase-1 deficiency exacerbates angiotensin ii-induced aortic aneurysm in mice. Oncotarget 7, 67760–67776. doi: 10.18632/oncotarget.11917
Holmstrom, K. M., and Finkel, T. (2014). Cellular mechanisms and physiological consequences of redox-dependent signalling. Nat. Rev. Mol. Cell Biol. 15, 411–421. doi: 10.1038/nrm3801
Horimatsu, T., Blomkalns, A. L., Ogbi, M., Moses, M., Kim, D., Patel, S., et al. (2019). Niacin protects against abdominal aortic aneurysm formation via gpr109a independent mechanisms: role of nad+/nicotinamide. Cardiovasc. Res. doi: 10.1093/cvr/cvz303 [Online ahead of print]
Hou, X., Yang, S., and Zheng, Y. (2019). Licochalcone a attenuates abdominal aortic aneurysm induced by angiotensin ii via regulating the mir-181b/sirt1/ho-1 signaling. J. Cell. Physiol. 234, 7560–7568. doi: 10.1002/jcp.27517
Huang, K., Yan, Z. Q., Zhao, D., Chen, S. G., Gao, L. Z., Zhang, P., et al. (2015). Sirt1 and foxo mediate contractile differentiation of vascular smooth muscle cells under cyclic stretch. Cell. Physiol. Biochem. 37, 1817–1829. doi: 10.1159/000438544
Jais, A., Einwallner, E., Sharif, O., Gossens, K., Lu, T. T., Soyal, S. M., et al. (2014). Heme oxygenase-1 drives metaflammation and insulin resistance in mouse and man. Cell 158, 25–40. doi: 10.1016/j.cell.2014.04.043
Jeong, J., Juhn, K., Lee, H., Kim, S. H., Min, B. H., Lee, K. M., et al. (2007). Sirt1 promotes DNA repair activity and deacetylation of ku70. Exp. Mol. Med. 39, 8–13. doi: 10.1038/emm.2007.2
Jimenez-Altayo, F., Meirelles, T., Crosas-Molist, E., Sorolla, M. A., Del Blanco, D. G., Lopez-Luque, J., et al. (2018). Redox stress in marfan syndrome: dissecting the role of the nadph oxidase nox4 in aortic aneurysm. Free Radic. Biol. Med. 118, 44–58.
Jones, A., Deb, R., Torsney, E., Howe, F., Dunkley, M., Gnaneswaran, Y., et al. (2009). Rosiglitazone reduces the development and rupture of experimental aortic aneurysms. Circulation 119, 3125–3132. doi: 10.1161/circulationaha.109.852467
Jung, S. B., Kim, C. S., Kim, Y. R., Naqvi, A., Yamamori, T., Kumar, S., et al. (2013). Redox factor-1 activates endothelial sirtuin1 through reduction of conserved cysteine sulfhydryls in its deacetylase domain. PLoS One 8:e65415. doi: 10.1371/journal.pone.0065415
Kaide, J., Zhang, F., Wei, Y., Wang, W., Gopal, V. R., Falck, J. R., et al. (2004). Vascular co counterbalances the sensitizing influence of 20-hete on agonist-induced vasoconstriction. Hypertension 44, 210–216. doi: 10.1161/01.hyp.0000135658.57547.bb
Kaide, J. I., Zhang, F., Wei, Y., Jiang, H., Yu, C., Wang, W. H., et al. (2001). Carbon monoxide of vascular origin attenuates the sensitivity of renal arterial vessels to vasoconstrictors. J. Clin. Invest. 107, 1163–1171. doi: 10.1172/jci11218
Kawai, Y., Garduno, L., Theodore, M., Yang, J., and Arinze, I. J. (2011). Acetylation-deacetylation of the transcription factor nrf2 (nuclear factor erythroid 2-related factor 2) regulates its transcriptional activity and nucleocytoplasmic localization. J. Biol. Chem. 286, 7629–7640. doi: 10.1074/jbc.m110.208173
Kawashima, T., Inuzuka, Y., Okuda, J., Kato, T., Niizuma, S., Tamaki, Y., et al. (2011). Constitutive sirt1 overexpression impairs mitochondria and reduces cardiac function in mice. J. Mol. Cell Cardiol. 51, 1026–1036. doi: 10.1016/j.yjmcc.2011.09.013
Kim, Y. M., Pae, H. O., Park, J. E., Lee, Y. C., Woo, J. M., Kim, N. H., et al. (2011). Heme oxygenase in the regulation of vascular biology: from molecular mechanisms to therapeutic opportunities. Antioxid. Redox Signal. 14, 137–167. doi: 10.1089/ars.2010.3153
Kumar, S., Kim, Y. R., Vikram, A., Naqvi, A., Li, Q., Kassan, M., et al. (2017). Sirtuin1-regulated lysine acetylation of p66shc governs diabetes-induced vascular oxidative stress and endothelial dysfunction. Proc. Natl. Acad. Sci. U.S.A. 114, 1714–1719. doi: 10.1073/pnas.1614112114
Lakhani, H. V., Zehra, M., Pillai, S. S., Puri, N., Shapiro, J. I., Abraham, N. G., et al. (2019). Beneficial role of ho-1-sirt1 axis in attenuating angiotensin ii-induced adipocyte dysfunction. Int. J. Mol. Sci. 20:3205. doi: 10.3390/ijms20133205
Lee, T. S., Chang, C. C., Zhu, Y., and Shyy, J. Y. (2004). Simvastatin induces heme oxygenase-1: a novel mechanism of vessel protection. Circulation 110, 1296–1302. doi: 10.1161/01.cir.0000140694.67251.9c
Leeper, N. J., Raiesdana, A., Kojima, Y., Kundu, R. K., Cheng, H., Maegdefessel, L., et al. (2013). Loss of cdkn2b promotes p53-dependent smooth muscle cell apoptosis and aneurysm formation. Arterioscler. Thromb. Vasc. Biol. 33, e1–e10.
Li, L., Zhang, H. N., Chen, H. Z., Gao, P., Zhu, L. H., Li, H. L., et al. (2011). Sirt1 acts as a modulator of neointima formation following vascular injury in mice. Circ. Res. 108, 1180–1189. doi: 10.1161/circresaha.110.237875
Liao, M., Liu, Z., Bao, J., Zhao, Z., Hu, J., Feng, X., et al. (2008). A proteomic study of the aortic media in human thoracic aortic dissection: implication for oxidative stress. J. Thoracic Cardiovasc. Surg. 136, 65–72.
Libri, V., Brown, A. P., Gambarota, G., Haddad, J., Shields, G. S., Dawes, H., et al. (2012). A pilot randomized, placebo controlled, double blind phase i trial of the novel sirt1 activator srt2104 in elderly volunteers. PLoS One 7:e51395. doi: 10.1371/journal.pone.0051395
Ling, L., Gu, S., and Cheng, Y. (2017). Resveratrol inhibits adventitial fibroblast proliferation and induces cell apoptosis through the sirt1 pathway. Mol. Med. Rep. 15, 567–572. doi: 10.3892/mmr.2016.6098
Liu, Y., Wang, T. T., Zhang, R., Fu, W. Y., Wang, X., Wang, F., et al. (2016). Calorie restriction protects against experimental abdominal aortic aneurysms in mice. J. Exp. Med. 213, 2473–2488. doi: 10.1084/jem.20151794
Loboda, A., Jazwa, A., Grochot-Przeczek, A., Rutkowski, A. J., Cisowski, J., Agarwal, A., et al. (2008). Heme oxygenase-1 and the vascular bed: from molecular mechanisms to therapeutic opportunities. Antioxid. Redox Signal. 10, 1767–1812. doi: 10.1089/ars.2008.2043
Lu, C. L., Liao, M. T., Hou, Y. C., Fang, Y. W., Zheng, C. M., Liu, W. C., et al. (2020). Sirtuin-1 and its relevance in vascular calcification. Int. J. Mol. Sci. 21:1593. doi: 10.3390/ijms21051593
Lu, W. W., Jia, L. X., Ni, X. Q., Zhao, L., Chang, J. R., Zhang, J. S., et al. (2016). Intermedin1-53 attenuates abdominal aortic aneurysm by inhibiting oxidative stress. Arterioscler. Thromb. Vasc. Biol. 36, 2176–2190. doi: 10.1161/atvbaha.116.307825
Man, A. W. C., Li, H., and Xia, N. (2019). The role of sirtuin1 in regulating endothelial function, arterial remodeling and vascular aging. Front. Physiol. 10:1173. doi: 10.3389/fphys.2019.01173
Marampon, F., Gravina, G. L., Scarsella, L., Festuccia, C., Lovat, F., Ciccarelli, C., et al. (2013). Angiotensin-converting-enzyme inhibition counteracts angiotensin ii-mediated endothelial cell dysfunction by modulating the p38/sirt1 axis. J. Hypertens. 31, 1972–1983. doi: 10.1097/hjh.0b013e3283638b32
Marcantoni, E., Di Francesco, L., Totani, L., Piccoli, A., Evangelista, V., Tacconelli, S., et al. (2012). Effects of estrogen on endothelial prostanoid production and cyclooxygenase-2 and heme oxygenase-1 expression. Prostaglandins Other Lipid Mediat. 98, 122–128. doi: 10.1016/j.prostaglandins.2012.01.006
Mattagajasingh, I., Kim, C. S., Naqvi, A., Yamamori, T., Hoffman, T. A., Jung, S. B., et al. (2007). Sirt1 promotes endothelium-dependent vascular relaxation by activating endothelial nitric oxide synthase. Proc. Natl. Acad. Sci. U.S.A. 104, 14855–14860. doi: 10.1073/pnas.0704329104
Michishita, E., Park, J. Y., Burneskis, J. M., Barrett, J. C., and Horikawa, I. (2005). Evolutionarily conserved and nonconserved cellular localizations and functions of human sirt proteins. Mol. Biol. Cell 16, 4623–4635. doi: 10.1091/mbc.e05-01-0033
Miki, H., and Funato, Y. (2012). Regulation of intracellular signalling through cysteine oxidation by reactive oxygen species. J. Biochem. 151, 255–261. doi: 10.1093/jb/mvs006
Miller, F. J. Jr., Sharp, W. J., Fang, X., Oberley, L. W., Oberley, T. D., and Weintraub, N. L. (2002). Oxidative stress in human abdominal aortic aneurysms: a potential mediator of aneurysmal remodeling. Arterioscler. Thromb. Vasc. Biol. 22, 560–565. doi: 10.1161/01.atv.0000013778.72404.30
Miyazaki, R., Ichiki, T., Hashimoto, T., Inanaga, K., Imayama, I., Sadoshima, J., et al. (2008). Sirt1, a longevity gene, downregulates angiotensin ii type 1 receptor expression in vascular smooth muscle cells. Arterioscler. Thromb. Vasc. Biol. 28, 1263–1269. doi: 10.1161/atvbaha.108.166991
Mody, P. S., Wang, Y., Geirsson, A., Kim, N., Desai, M. M., Gupta, A., et al. (2014). Trends in aortic dissection hospitalizations, interventions, and outcomes among medicare beneficiaries in the united states, 2000-2011. Circulation 7, 920–928. doi: 10.1161/circoutcomes.114.001140
Moran, C. S., Biros, E., Krishna, S. M., Wang, Y., Tikellis, C., Morton, S. K., et al. (2017). Resveratrol inhibits growth of experimental abdominal aortic aneurysm associated with upregulation of angiotensin-converting enzyme 2. Arterioscler. Thromb. Vasc. Biol. 37, 2195–2203. doi: 10.1161/atvbaha.117.310129
Motoki, T., Kurobe, H., Hirata, Y., Nakayama, T., Kinoshita, H., Rocco, K. A., et al. (2015). Ppar-gamma agonist attenuates inflammation in aortic aneurysm patients. Gen. Thoracic Cardiovasc. Surg. 63, 565–571. doi: 10.1007/s11748-015-0576-1
Nakahashi, T. K., Hoshina, K., Tsao, P. S., Sho, E., Sho, M., Karwowski, J. K., et al. (2002). Flow loading induces macrophage antioxidative gene expression in experimental aneurysms. Arterioscler. Thromb. Vasc. Biol. 22, 2017–2022. doi: 10.1161/01.atv.0000042082.38014.ea
Nakamura, K., Kageyama, S., Yue, S., Huang, J., Fujii, T., Ke, B., et al. (2018). Heme oxygenase-1 regulates sirtuin-1-autophagy pathway in liver transplantation: from mouse to human. Am. J. Transplant. 18, 1110–1121. doi: 10.1111/ajt.14586
Namba, F., Go, H., Murphy, J. A., La, P., Yang, G., Sengupta, S., et al. (2014). Expression level and subcellular localization of heme oxygenase-1 modulates its cytoprotective properties in response to lung injury: a mouse model. PLoS One 9:e90936. doi: 10.1371/journal.pone.0090936
Nguyen, L. T., Chen, H., Pollock, C., and Saad, S. (2017). SIRT1 reduction is associated with sex-specific dysregulation of renal lipid metabolism and stress responses in offspring by maternal high-fat diet. Sci. Rep. 7:8982. doi: 10.1038/s41598-017-08694-4
Oellerich, M. F., and Potente, M. (2012). Foxos and sirtuins in vascular growth, maintenance, and aging. Circ. Res. 110, 1238–1251. doi: 10.1161/circresaha.111.246488
Ota, H., Eto, M., Kano, M. R., Kahyo, T., Setou, M., Ogawa, S., et al. (2010a). Induction of endothelial nitric oxide synthase, sirt1, and catalase by statins inhibits endothelial senescence through the akt pathway. Arterioscler. Thromb. Vasc. Biol. 30, 2205–2211. doi: 10.1161/atvbaha.110.210500
Ota, H., Eto, M., Kano, M. R., Ogawa, S., Iijima, K., Akishita, M., et al. (2008). Cilostazol inhibits oxidative stress-induced premature senescence via upregulation of sirt1 in human endothelial cells. Arterioscler. Thromb. Vasc. Biol. 28, 1634–1639. doi: 10.1161/atvbaha.108.164368
Ota, H., Eto, M., Ogawa, S., Iijima, K., Akishita, M., and Ouchi, Y. (2010b). Sirt1/enos axis as a potential target against vascular senescence, dysfunction and atherosclerosis. J. Atheroscler. Thromb. 17, 431–435. doi: 10.5551/jat.3525
Pacholec, M., Bleasdale, J. E., Chrunyk, B., Cunningham, D., Flynn, D., Garofalo, R. S., et al. (2010). Srt1720, srt2183, srt1460, and resveratrol are not direct activators of sirt1. J. Biol. Chem. 285, 8340–8351. doi: 10.1074/jbc.m109.088682
Paneni, F., Volpe, M., Luscher, T. F., and Cosentino, F. (2013). Sirt1, p66(shc), and set7/9 in vascular hyperglycemic memory: bringing all the strands together. Diabetes 62, 1800–1807. doi: 10.2337/db12-1648
Park, S. J., Ahmad, F., Philp, A., Baar, K., Williams, T., Luo, H., et al. (2012). Resveratrol ameliorates aging-related metabolic phenotypes by inhibiting camp phosphodiesterases. Cell 148, 421–433. doi: 10.1016/j.cell.2012.01.017
Piechota-Polanczyk, A., Kopacz, A., Kloska, D., Zagrapan, B., Neumayer, C., Grochot-Przeczek, A., et al. (2018). Simvastatin treatment upregulates ho-1 in patients with abdominal aortic aneurysm but independently of nrf2. Oxidat. Med. Cell. Longevity 2018:2028936.
Posa, A., Kupai, K., Menesi, R., Szalai, Z., Szabo, R., Pinter, Z., et al. (2013). Sexual dimorphism of cardiovascular ischemia susceptibility is mediated by heme oxygenase. Oxidat. Med. Cell. Longevity 2013:521563.
Potente, M., Ghaeni, L., Baldessari, D., Mostoslavsky, R., Rossig, L., Dequiedt, F., et al. (2007). Sirt1 controls endothelial angiogenic functions during vascular growth. Genes Dev. 21, 2644–2658. doi: 10.1101/gad.435107
Quintana, R. A., and Taylor, W. R. (2019). Cellular mechanisms of aortic aneurysm formation. Circ. Res. 124, 607–618. doi: 10.1161/circresaha.118.313187
Radak, Z., Koltai, E., Taylor, A. W., Higuchi, M., Kumagai, S., Ohno, H., et al. (2013). Redox-regulating sirtuins in aging, caloric restriction, and exercise. Free Radic. Biol. Med. 58, 87–97. doi: 10.1016/j.freeradbiomed.2013.01.004
Rateri, D. L., Moorleghen, J. J., Balakrishnan, A., Owens, A. P. III, Howatt, D. A., Subramanian, V., et al. (2011). Endothelial cell-specific deficiency of ang ii type 1a receptors attenuates ang ii-induced ascending aortic aneurysms in ldl receptor-/- mice. Circ. Res. 108, 574–581. doi: 10.1161/circresaha.110.222844
Robinet, P., Milewicz, D. M., Cassis, L. A., Leeper, N. J., Lu, H. S., and Smith, J. D. (2018). Consideration of sex differences in design and reporting of experimental arterial pathology studies-statement from atvb council. Arterioscler. Thromb. Vasc. Biol. 38, 292–303. doi: 10.1161/atvbaha.117.309524
Rodriguez, F., Lamon, B. D., Gong, W., Kemp, R., and Nasjletti, A. (2004). Nitric oxide synthesis inhibition promotes renal production of carbon monoxide. Hypertension 43, 347–351. doi: 10.1161/01.hyp.0000111721.97169.97
Rodriguez, F., Lopez, B., Perez, C., Fenoy, F. J., Hernandez, I., Stec, D. E., et al. (2011). Chronic tempol treatment attenuates the renal hemodynamic effects induced by a heme oxygenase inhibitor in streptozotocin diabetic rats. Am. J. Physiol. Regul. Integr. Comp. Physiol. 301, R1540–R1548.
Rodriguez, F., Nieto-Ceron, S., Fenoy, F. J., Lopez, B., Hernandez, I., Martinez, R. R., et al. (2010). Sex differences in nitrosative stress during renal ischemia. Am. J. Physiol. Regul. Integr. Comp Physiol. 299, R1387–R1395.
Rodriguez, F., Zhang, F., Dinocca, S., and Nasjletti, A. (2003). Nitric oxide synthesis influences the renal vascular response to heme oxygenase inhibition. Am. J. Physiol. Renal. Physiol. 284, F1255–F1262.
Salom, M. G., Ceron, S. N., Rodriguez, F., Lopez, B., Hernandez, I., Martinez, J. G., et al. (2007). Heme oxygenase-1 induction improves ischemic renal failure: role of nitric oxide and peroxynitrite. Am. J. Physiol. Heart Circ. Physiol. 293, H3542–H3549.
Sartori-Valinotti, J. C., Iliescu, R., Fortepiani, L. A., Yanes, L. L., and Reckelhoff, J. F. (2007). Sex differences in oxidative stress and the impact on blood pressure control and cardiovascular disease. Clin. Exp. Pharmacol. Physiol. 34, 938–945. doi: 10.1111/j.1440-1681.2007.04643.x
Schillinger, M., Exner, M., Mlekusch, W., Domanovits, H., Huber, K., Mannhalter, C., et al. (2002). Heme oxygenase-1 gene promoter polymorphism is associated with abdominal aortic aneurysm. Thromb. Res. 106, 131–136. doi: 10.1016/s0049-3848(02)00100-7
Shao, D., Fry, J. L., Han, J., Hou, X., Pimentel, D. R., Matsui, R., et al. (2014). A redox-resistant sirtuin-1 mutant protects against hepatic metabolic and oxidant stress. J. Biol. Chem. 289, 7293–7306. doi: 10.1074/jbc.m113.520403
Shao, D., Han, J., Hou, X., Fry, J., Behring, J. B., Seta, F., et al. (2017). Glutaredoxin-1 deficiency causes fatty liver and dyslipidemia by inhibiting sirtuin-1. Antioxid. Redox Signal. 27, 313–327. doi: 10.1089/ars.2016.6716
Singh, K., Bonaa, K. H., Jacobsen, B. K., Bjork, L., and Solberg, S. (2001). Prevalence of and risk factors for abdominal aortic aneurysms in a population-based study: the tromso study. Am. J. Epidemiol. 154, 236–244. doi: 10.1093/aje/154.3.236
Siu, K. L., Li, Q., Zhang, Y., Guo, J., Youn, J. Y., Du, J., et al. (2017). Nox isoforms in the development of abdominal aortic aneurysm. Redox Biol. 11, 118–125. doi: 10.1016/j.redox.2016.11.002
Sodhi, K., Puri, N., Favero, G., Stevens, S., Meadows, C., Abraham, N. G., et al. (2015). Fructose mediated non-alcoholic fatty liver is attenuated by ho-1-sirt1 module in murine hepatocytes and mice fed a high fructose diet. PLoS One 10:e0128648. doi: 10.1371/journal.pone.0128648
Sosnowska, B., Mazidi, M., Penson, P., Gluba-Brzozka, A., Rysz, J., and Banach, M. (2017). The sirtuin family members sirt1, sirt3 and sirt6: their role in vascular biology and atherogenesis. Atherosclerosis 265, 275–282. doi: 10.1016/j.atherosclerosis.2017.08.027
Strycharz, J., Rygielska, Z., Swiderska, E., Drzewoski, J., Szemraj, J., Szmigiero, L., et al. (2018). Sirt1 as a therapeutic target in diabetic complications. Curr. Med. Chem. 25, 1002–1035. doi: 10.2174/0929867324666171107103114
Suttner, D. M., and Dennery, P. A. (1999). Reversal of ho-1 related cytoprotection with increased expression is due to reactive iron. FASEB J. 13, 1800–1809. doi: 10.1096/fasebj.13.13.1800
Tai, H. C., Tsai, P. J., Chen, J. Y., Lai, C. H., Wang, K. C., Teng, S. H., et al. (2016). Peroxisome proliferator-activated receptor gamma level contributes to structural integrity and component production of elastic fibers in the aorta. Hypertension 67, 1298–1308. doi: 10.1161/hypertensionaha.116.07367
Thompson, A. M., Wagner, R., and Rzucidlo, E. M. (2014). Age-related loss of sirt1 expression results in dysregulated human vascular smooth muscle cell function. Am. J. Physiol. Heart Circ. Physiol. 307, H533–H541.
Thorup, C., Jones, C. L., Gross, S. S., Moore, L. C., and Goligorsky, M. S. (1999). Carbon monoxide induces vasodilation and nitric oxide release but suppresses endothelial nos. Am. J. Physiol. 277, F882–F889.
van Andel, M. M., Groenink, M., Zwinderman, A. H., Mulder, B. J. M., and de Waard, V. (2019). The potential beneficial effects of resveratrol on cardiovascular complications in marfan syndrome patients(-)insights from rodent-based animal studies. Int. J. Mol. Sci. 20:1122. doi: 10.3390/ijms20051122
van der Pluijm, I., Burger, J., van Heijningen, P. M., Aaa, I. J., van Vliet, N., Milanese, C., et al. (2018). Decreased mitochondrial respiration in aneurysmal aortas of fibulin-4 mutant mice is linked to pgc1a regulation. Cardiovasc. Res. 114, 1776–1793. doi: 10.1093/cvr/cvy150
Venkatasubramanian, S., Noh, R. M., Daga, S., Langrish, J. P., Joshi, N. V., Mills, N. L., et al. (2013). Cardiovascular effects of a novel sirt1 activator, srt2104, in otherwise healthy cigarette smokers. J. Am. Heart Assoc. 2:e000042.
Venkatasubramanian, S., Noh, R. M., Daga, S., Langrish, J. P., Mills, N. L., Waterhouse, B. R., et al. (2016). Effects of the small molecule sirt1 activator, srt2104 on arterial stiffness in otherwise healthy cigarette smokers and subjects with type 2 diabetes mellitus. Open Heart 3:e000402. doi: 10.1136/openhrt-2016-000402
Virani, S. S., Alonso, A., Benjamin, E. J., Bittencourt, M. S., Callaway, C. W., Carson, A. P., et al. (2020). American Heart Association Council on E. Prevention Statistics C, Stroke Statistics S. Heart disease and stroke statistics-2020 update: a report from the american heart association. Circulation 141, e139–e596.
Watson, A., Nong, Z., Yin, H., O’Neil, C., Fox, S., Balint, B., et al. (2017). Nicotinamide phosphoribosyltransferase in smooth muscle cells maintains genome integrity, resists aortic medial degeneration, and is suppressed in human thoracic aortic aneurysm disease. Circ. Res. 120, 1889–1902. doi: 10.1161/circresaha.116.310022
Wicinski, M., Socha, M., Walczak, M., Wodkiewicz, E., Malinowski, B., Rewerski, S., et al. (2018). Beneficial effects of resveratrol administration-focus on potential biochemical in cardiovascular conditions. Nutrients 10:1813. doi: 10.3390/nu10111813
Yang, H. H., van Breemen, C., and Chung, A. W. (2010). Vasomotor dysfunction in the thoracic aorta of marfan syndrome is associated with accumulation of oxidative stress. Vasc. Pharmacol. 52, 37–45. doi: 10.1016/j.vph.2009.10.005
Yu, L. M., Dong, X., Xue, X. D., Zhang, J., Li, Z., Wu, H. J., et al. (2019). Protection of the myocardium against ischemia/reperfusion injury by punicalagin through an sirt1-nrf-2-ho-1-dependent mechanism. Chem. Biol. Interact. 306, 152–162. doi: 10.1016/j.cbi.2019.05.003
Zampino, M., Yuzhakova, M., Hansen, J., McKinney, R. D., Goldspink, P. H., Geenen, D. L., et al. (2006). Sex-related dimorphic response of hif-1 alpha expression in myocardial ischemia. Am. J. Physiol. Heart Circ. Physiol. 291, H957–H964.
Zee, R. S., Yoo, C. B., Pimentel, D. R., Perlman, D. H., Burgoyne, J. R., Hou, X., et al. (2010). Redox regulation of sirtuin-1 by s-glutathiolation. Antioxid. Redox Signal. 13, 1023–1032. doi: 10.1089/ars.2010.3251
Zeng, H. T., Fu, Y. C., Yu, W., Lin, J. M., Zhou, L., Liu, L., et al. (2013). Sirt1 prevents atherosclerosis via liverxreceptor and nfkappab signaling in a u937 cell model. Mol. Med. Rep. 8, 23–28. doi: 10.3892/mmr.2013.1460
Zhang, F., Kaide, J. I., Rodriguez-Mulero, F., Abraham, N. G., and Nasjletti, A. (2001). Vasoregulatory function of the heme-heme oxygenase-carbon monoxide system. Am. J. Hypertens. 14, 62S–67S.
Zhang, W., Huang, Q., Zeng, Z., Wu, J., Zhang, Y., and Chen, Z. (2017). Sirt1 inhibits oxidative stress in vascular endothelial cells. Oxidative Med. Cell. Longevity 2017:7543973.
Zhang, X., Thatcher, S., Wu, C., Daugherty, A., and Cassis, L. A. (2015). Castration of male mice prevents the progression of established angiotensin ii-induced abdominal aortic aneurysms. J. Vasc. Surg. 61, 767–776. doi: 10.1016/j.jvs.2013.11.004
Zhang, Z., Xu, J., Liu, Y., Wang, T., Pei, J., Cheng, L., et al. (2018). Mouse macrophage specific knockout of sirt1 influences macrophage polarization and promotes angiotensin ii-induced abdominal aortic aneurysm formation. J. Genet. Genom. 45, 25–32. doi: 10.1016/j.jgg.2018.01.002
Zhou, S., Chen, H. Z., Wan, Y. Z., Zhang, Q. J., Wei, Y. S., Huang, S., et al. (2011). Repression of p66shc expression by sirt1 contributes to the prevention of hyperglycemia-induced endothelial dysfunction. Circ. Res. 109, 639–648. doi: 10.1161/circresaha.111.243592
Zordoky, B. N., Robertson, I. M., and Dyck, J. R. (2015). Preclinical and clinical evidence for the role of resveratrol in the treatment of cardiovascular diseases. Biochim. Biophys. Acta 1852, 1155–1177. doi: 10.1016/j.bbadis.2014.10.016
Zuniga-Munoz, A. M., Perez-Torres, I., Guarner-Lans, V., Nunez-Garrido, E., Velazquez Espejel, R., Huesca-Gomez, C., et al. (2017). Glutathione system participation in thoracic aneurysms from patients with marfan syndrome. VASA Zeitschrift Gefasskrankheiten 46, 177–186. doi: 10.1024/0301-1526/a000609
Keywords: sirtuin-1, aortic aneurysm, oxidative stress, heme oxgenase, vascular disease
Citation: Budbazar E, Rodriguez F, Sanchez JM and Seta F (2020) The Role of Sirtuin-1 in the Vasculature: Focus on Aortic Aneurysm. Front. Physiol. 11:1047. doi: 10.3389/fphys.2020.01047
Received: 25 June 2020; Accepted: 30 July 2020;
Published: 25 August 2020.
Edited by:
John D. Imig, Medical College of Wisconsin, United StatesReviewed by:
Hong S. Lu, University of Kentucky, United StatesModar Kassan, The University of Iowa, United States
Copyright © 2020 Budbazar, Rodriguez, Sanchez and Seta. This is an open-access article distributed under the terms of the Creative Commons Attribution License (CC BY). The use, distribution or reproduction in other forums is permitted, provided the original author(s) and the copyright owner(s) are credited and that the original publication in this journal is cited, in accordance with accepted academic practice. No use, distribution or reproduction is permitted which does not comply with these terms.
*Correspondence: Francisca Rodriguez, ZnJvZHJpQHVtLmVz; Francesca Seta, c2V0YWZAYnUuZWR1
†These authors have contributed equally to this work