- 1Graduate School of Sport and Health Science, Ritsumeikan University, Kusatsu, Japan
- 2School of Human Science and Environment, University of Hyogo, Kobe, Japan
- 3School of Human Sciences (Exercise and Sport Science), The University of Western Australia, Perth, WA, Australia
Purpose: We explored the effect of heat stress during an acute endurance exercise session in hypoxia on endocrine and metabolic responses.
Methods: A total of 12 healthy males cycled at a constant workload (60% of the power output associated with their maximal oxygen uptake under each respective condition) for 60 min in three different environments: exercise under hot and hypoxia (H+H; fraction of inspiratory oxygen or FiO2: 14.5%, 32°C), exercise under hypoxia (HYP; FiO2: 14.5%, 23°C), and exercise under normoxia (NOR; FiO2: 20.9%, 23°C). After completing the exercise, participants remained in the chamber for 3 h to evaluate metabolic and endocrine responses under each environment. Changes in muscle oxygenation (only during exercise), blood variables, arterial oxygen saturation, and muscle temperature were determined up to 3 h after exercise.
Results: Serum erythropoietin (EPO) level was increased to similar levels in both H+H and HYP at 3 h after exercise compared with before exercise (P < 0.05), whereas no significant increase was found under NOR. No significant difference between H+H and HYP was observed in the serum EPO level, blood lactate level, or muscle oxygenation at any time (P > 0.05). Exercise-induced serum growth hormone (GH) elevation was significantly greater in H+H compared with HYP (P < 0.05) and HYP showed significantly lower value than NOR (P < 0.05). Arterial oxygen saturation during exercise was significantly lower in H+H and HYP compared with NOR (P < 0.05). Furthermore, H+H showed higher value compared with HYP (P < 0.05).
Conclusion: The serum EPO level increased significantly with endurance exercise in hypoxia. However, the addition of heat stress during endurance exercise in hypoxia did not augment the EPO response up to 3 h after completion of exercise. Exercise-induced GH elevation was significantly augmented when the hot exposure was combined during endurance exercise in hypoxia. Muscle oxygenation levels during endurance exercise did not differ significantly among the conditions. These findings suggest that combined hot and hypoxic stresses during endurance exercise caused some modifications of metabolic and endocrine regulations compared with the same exercise in hypoxia.
Introduction
Hypoxic training (i.e., endurance training under normobaric hypoxia) is commonly used by endurance athletes to improve their endurance capacity (Vogt et al., 2001; Dufour et al., 2006). One of the key physiological mechanisms for improved endurance capacity is thought to be hypoxia-induced erythropoiesis, which may lead to improved maximal oxygen uptake (V̇O2max) (Levin, 2002; Millet et al., 2010). Erythropoietin (EPO) is an erythropoietic hormone derived from kidney, and endurance exercise in hypoxia increases EPO production. The increased EPO contributes to augmented red blood cells (Haase, 2013; Turner et al., 2017). The hypoxia-induced increase in hemoglobin (Hb) mass increases the oxygen supply capacity for working muscle, thereby improving endurance capacity (Mairbäurl, 2013).
Many reports have shown a significant EPO response to acute endurance exercise in hypoxia (Schmidt et al., 1991; Mackenzie et al., 2008; Wahl et al., 2013). For example, Wahl et al. (2013) found that endurance exercise (cycling) for 90 min under both moderate [fraction of inspiratory oxygen (FiO2): 15.9%] and severe (FiO2: 13.2%) hypoxia significantly increased the serum EPO level 3 h after completion of exercise. Similarly, Mackenzie et al. (2008) showed that 90 min of rest under hypoxia (FiO2: 14.8%) followed by running for 30 min at 50% of V̇O2max significantly increased the serum EPO level.
Aside from hypoxia, heat stress during endurance exercise augments hematological variables (Lee et al., 2016). Hot exposure during an acute endurance exercise session decreased endurance performance due to disrupted homeostasis (e.g., a greater increase in core temperature and augmented dehydration) (Cheuvront et al., 2003; Périard et al., 2011). However, it may also increase plasma volume (PV) on the following day after completing the endurance exercise session under hot conditions (Nielsen et al., 1993; Lorenzo et al., 2010). Additionally, endurance exercise under hot condition may upregulate EPO production. Although the effect of acute endurance exercise under hot condition on EPO production remains unclear, 10 consecutive days of endurance training at 40°C (90 min of cycling/session) significantly increased the serum EPO level (compared with the baseline level before commencing intervention), and additional hypoxic exposure at night (8 h/day) did not cause any further increase (Rendell et al., 2017). Currently, exercise-induced EPO responses to a combination of hypoxic and heat stressors during acute endurance exercise have not been explored.
Therefore, the purpose of the present study was to compare endocrine (in particular the EPO) and metabolic responses to submaximal constant-intensity cycling exercise under (1) hot and hypoxia, (2) hypoxia, and (3) normoxia. We hypothesized that exercise-induced EPO production would be augmented when hot and hypoxia were combined.
Materials and Methods
Participants
A total of 12 healthy physically active men [mean ± standard error (SE) age, height, and body weight were 21.5 ± 0.3 years, 168.1 ± 1.2 cm, and 63.6 ± 2.0 kg, respectively] volunteered for this study. All participants were born and living near sea level. They were informed of the experimental procedures and possible risks associated with this study before they provided written consent. The present study was approved by the Ethics Committee for Human Experiments at Ritsumeikan University, Japan.
Experimental Overview
All participants visited the laboratory on six occasions. During the initial three visits, they completed an incremental pedaling test on a cycle ergometer (Aerobike 75XLIII; Konami Corporation, Tokyo, Japan) under either hot and hypoxia (H+H; FiO2: 14.5%, 32°C), normobaric hypoxia (HYP; FiO2: 14.5%, 23°C), or normoxia (NOR; FiO2: 20.9%, 23°C) to evaluate V̇O2max. The order of each condition was randomized, and each test was performed at least 2 days apart. The test began at 50 W, and the load was progressively increased by 30 W every 2 min. Exercise was terminated when the participant remained below 70 rpm for >5 s (exhaustion). Respiratory gases were collected and analyzed using an automatic gas analyzer (AE300S; Minato Medical Science Co., Ltd., Tokyo, Japan). The data collected were averaged every 30 s. The highest V̇O2 during exercise was defined as the V̇O2max.
The three main experimental conditions (visits 4–6) involved cycling for 60 min at 60% of V̇O2max under each condition (H+H, HYP, and NOR). The order of each condition was randomized, and each condition was conducted at least 5 days apart. All conditions were completed in an environmental chamber (14.8 m2), and hypoxic exposure was simulated by increasing the nitrogen level in the room (Morishima et al., 2014; Kasai et al., 2017). Venous blood samples were collected before exercise, 20, 40, and 60 min after commencing exercise, and 3 h after completing exercise to evaluate the serum EPO and growth hormone (GH) levels, blood lactate, glucose, Hb levels, hematocrit (Hct) value, partial pressure of oxygen (pO2), partial pressure of carbon dioxide (pCO2), pH, and bicarbonate iron (HCO3–) levels.
Experimental Conditions
Exercise (Aerobike 75XLIII; Konami Corporation, Tokyo, Japan) was commenced following 30 min of rest after entering the chamber and obtaining baseline measurements. After completing the exercise, participants remained in the chamber for 3 h to evaluate metabolic and endocrine responses during the post-exercise period. The total duration of each session was approximately 4.5 h (including rest for 30 min, exercise for 60 min, and rest for 3 h post-exercise).
During all conditions, the FiO2 level was not displayed to the participant. Water intake (2 ml/body weight) was allowed before exercise and at 20 and 40 min during exercise. During the 3 h post-exercise period, participants consumed at least 600 ml water, and this volume was matched among the three conditions.
Measurements
SpO2 and Heart Rate (HR)
Arterial oxygen saturation (SpO2) was recorded continuously (every 1 s) using a finger pulse oximeter (PULSOX-Me300; Teijin Pharma Ltd., Tokyo, Japan). HR was recorded continuously during exercise (every 5 s) and at 3 h post-exercise using a wireless HR monitor (RCX5; Polar Electro, Tokyo, Japan). SpO2 and HR values were averaged every 10 min during exercise and every 30 min during the post-exercise period.
Muscle and Skin Temperatures
Vastus lateralis muscle and skin temperatures were measured continuously (at a distance of 50% between the greater trochanter and lateral condyle of the femur) before (after entering the chamber) and during exercise and at 3 h post-exercise. Muscle temperature was evaluated non-invasively (every 2 s) using a probe-type thermometer (Core temp CM-210; TERMO Co., Ltd., Tokyo, Japan) (Wakabayashi et al., 2018). Skin temperature was monitored (every 1 s) using a probe-type thermometer and logger (NT Logger N543; Nikkiso-Therm Co., Ltd., Tokyo, Japan) (Maruyama et al., 2019). Muscle and skin temperatures were averaged every 10 min during exercise and every 30 min during the post-exercise period.
Blood Variables
Following an overnight fast, the participants arrived at the laboratory in the morning (8:00 am). After 30 min of rest, baseline blood samples were collected from an inserted cannula in the antecubital vein. Afterward, blood samples were further collected at 20, 40, and 60 min after commencing exercise and at 3 h after completing the exercise. To obtain serum, the blood samples were centrifuged for 10 min at 4°C (3000 rpm) and stored at −80°C prior to analysis. Serum EPO and GH levels were measured in a clinical laboratory (SRL Inc., Tokyo, Japan) using an atomic absorption method (for EPO) and radioimmunoassay (for GH). The intra-assay coefficients of variation were 7.2% for EPO and 3.5% for GH, respectively.
Whole blood samples were also used to determine the blood Hb level, Hct value, pO2, pCO2, pH, and HCO3– levels using a blood gas analyzer (OPTI CCA-TS2; Sysmex Corporation, Hyogo, Japan). Exercise-induced changes in PV were calculated using the Hb level and Hct value, as described previously (Dill and Costill, 1974).
Blood glucose and lactate levels were measured every 20 min during exercise and at 3 h after completing the exercise using a glucose analyzer (Freestyle; Nipro Co., Osaka, Japan) and lactate analyzer (Lactate Pro; Arkray Inc., Kyoto, Japan), respectively.
Muscle Oxygenation Evaluated Using Near-Infrared Spectroscopy (NIRS)
Before exercise (before and after entering the chamber) and 20, 40, and 60 min after commencing exercise, the oxygenated Hb (oxy-Hb), deoxygenated Hb (deoxy-Hb), total Hb (total-Hb), and tissue oxygen saturation (StO2) were recorded from the vastus lateralis muscle (at a distance of 50% from the greater trochanter and lateral condyle of the femur) using NIRS (Hb14; ASTEM Co., Ltd., Kanagawa, Japan) (Yamaguchi et al., 2019). To determine muscle oxygenation variables, the NIRS probe was placed on the muscle at an inter-optode distance of 30 mm. All signals were recorded at a sampling frequency of 10 Hz. NIRS data during exercise were expressed relative to the baseline value (data collected before entering the chamber), which was determined under normoxic (FiO2: 20.9%) and normal (23°C) conditions.
Respiratory Gas Parameters
Respiratory gas parameters were collected every 20 min during exercise (15–20, 35–40, and 55–60 min during the 60-min exercise period). Oxygen uptake (V̇O2), carbon dioxide output (V̇CO2), and minute ventilation (V̇E) were evaluated using an automatic gas analyzer (AE300S; Minato Medical Science Co., Ltd., Tokyo, Japan). The respiratory exchange ratio (RER) was calculated using the V̇O2 and V̇CO2 values. All respiratory values were averaged every 30 s.
Statistical Analysis
All data are presented as means ± SE. Two-way repeated-measures analysis of variance (ANOVA) was used to investigate the main effects of condition, time, and the interaction between condition and time. When a significant effect was found, the Tukey–Kramer post-hoc test was performed. Absolute workload, average SpO2, HR, muscle temperature, and skin temperature were compared using one-way ANOVA followed by a post-hoc test. For all tests, a P-value < 0.05 was considered significant. The values of partial eta squared (partial η2) for one-way and two-way repeated-measures ANOVA were calculated to present effect size. The partial η2 around of around 0.02, 0.13, and 0.26 were considered as “small,” “medium,” and “large” (Dalton et al., 2017).
Results
Absolute Workload During 60 min of Endurance Exercise
Absolute workload during exercise under each condition was significantly lower in H+H (110 ± 4 W) and HYP (107 ± 5 W) than in NOR (136 ± 4 W).
Serum EPO Level
Figure 1 presents the absolute (A) and relative (B) changes in the serum EPO level before and after exercise. In both H+H and HYP, the serum EPO level was significantly increased at 3 h after exercise and was significantly higher than that in NOR. However, no significant difference in the serum EPO level was observed between the H+H and HYP. The relative change in the EPO level at 3 h after exercise was significantly higher in both H+H (141.4 ± 9.9%) and HYP (136.7 ± 9.1%) than in NOR (101.9 ± 7.1%), but no significant difference was observed between H+H and HYP.
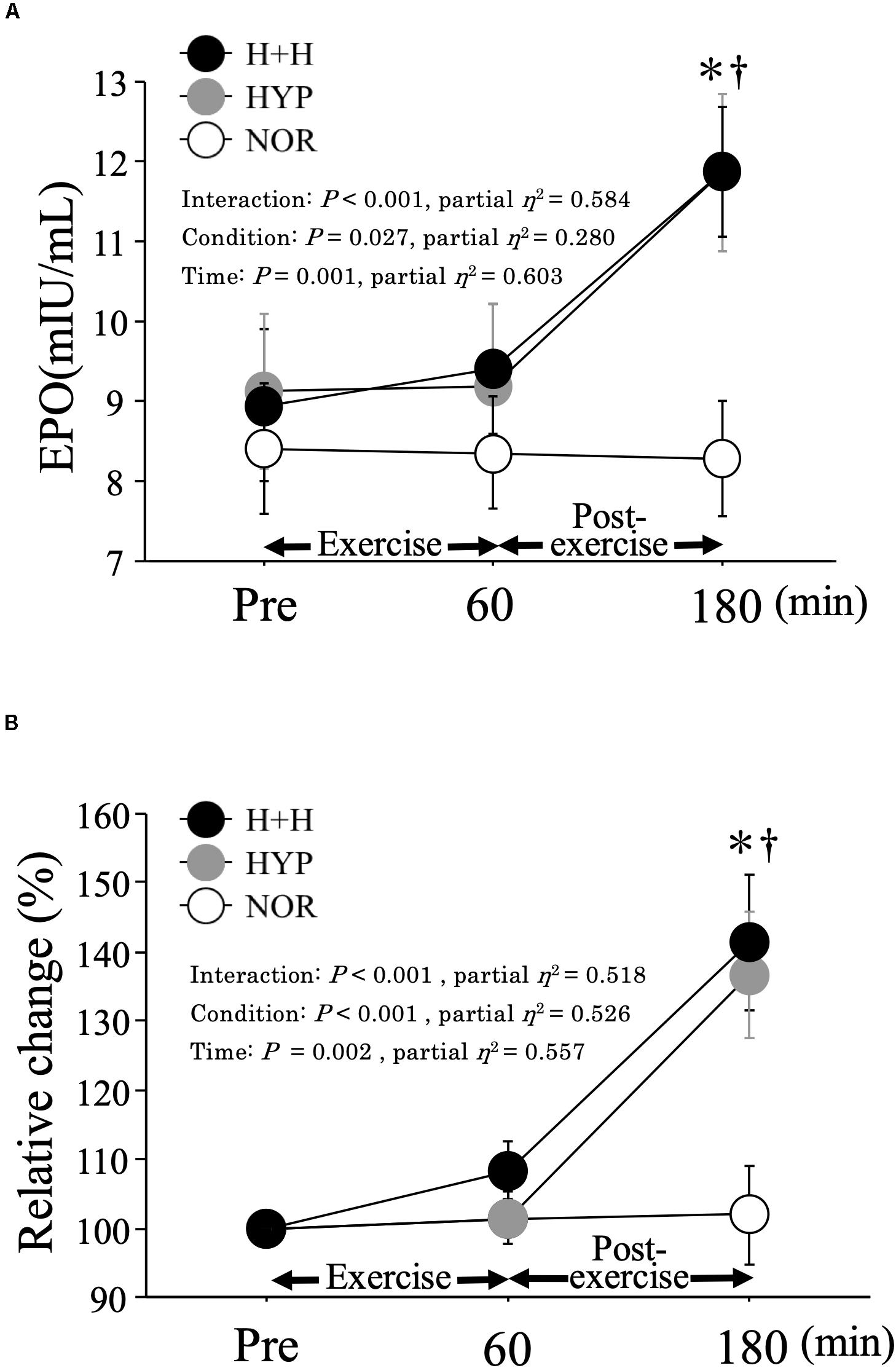
Figure 1. Absolute (A) and relative (B) changes in serum EPO level during exercise and post-exercise. Values are means ± SE. ∗P < 0.05 vs. Pre. † P < 0.05 vs. NOR.
SpO2 and HR
Figure 2 presents the changes in the SpO2 level from before to after exercise. The average SpO2 during exercise was significantly lower in H+H (86.9 ± 0.6%) and HYP (85.5 ± 0.6%) than in NOR (96.1 ± 0.3%, P < 0.01, partial η2 = 0.95). The SpO2 level during exercise was significantly higher in H+H than in HYP at the 50-min time point. During the 3 h post-exercise period, the average SpO2 remained significantly lower in both H+H (90.4 ± 0.4%) and HYP (91.0 ± 0.5%) than in NOR (96.8 ± 0.2%, P < 0.01, partial η2 = 0.97). The average HR during exercise was significantly higher in H+H (152 ± 3 bpm) and NOR (149 ± 3 bpm) than in HYP (142 ± 4 bpm, P = 0.01, partial η2 = 0.37). During the post-exercise period, the average HR was significantly higher in H+H (92 ± 3 bpm) compared with HYP (78 ± 3 bpm) and NOR (78 ± 3 bpm, P < 0.01, partial η2 = 0.74).
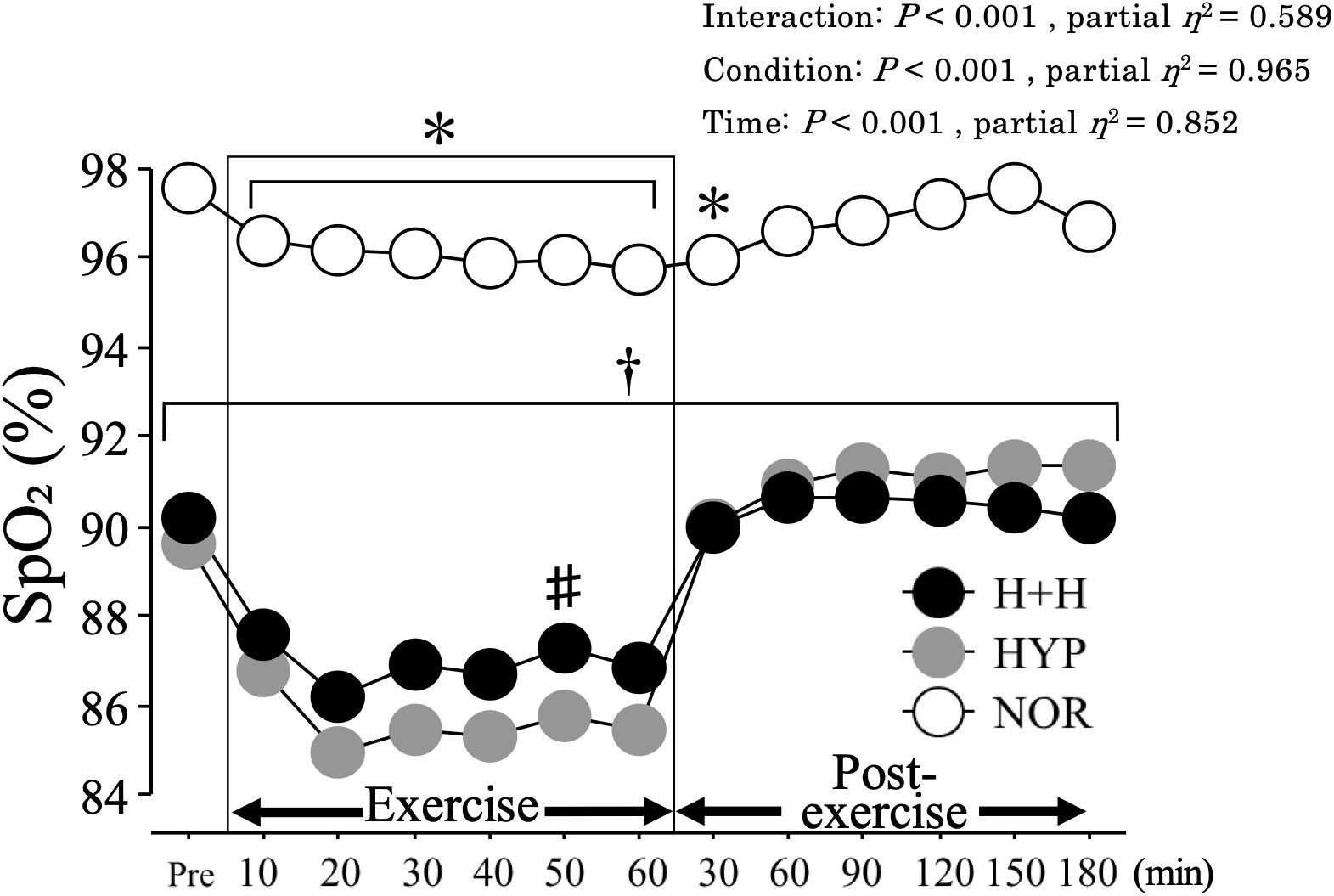
Figure 2. SpO2 during exercise and post-exercise. Values are means ± SE. ∗P < 0.05 vs. Pre. †P < 0.05 vs. NOR. #P < 0.05 vs. HYP. Shadow box indicates exercise duration.
Muscle and Skin Temperatures
Figure 3 presents the skin (A) and muscle (B) temperatures before and after exercise. Muscle temperature increased significantly during exercise under all conditions, with a higher average value in H+H (37.6 ± 0.1°C) than in HYP (37.1 ± 0.1°C) and NOR (37.3 ± 0.1°C, P < 0.01, partial η2 = 0.94). During the post-exercise period, the average muscle temperature was significantly higher in H+H (36.8 ± 0.1°C) than in HYP (35.9 ± 0.1°C) and NOR (35.8 ± 0.1°C, P < 0.01, partial η2 = 0.85).
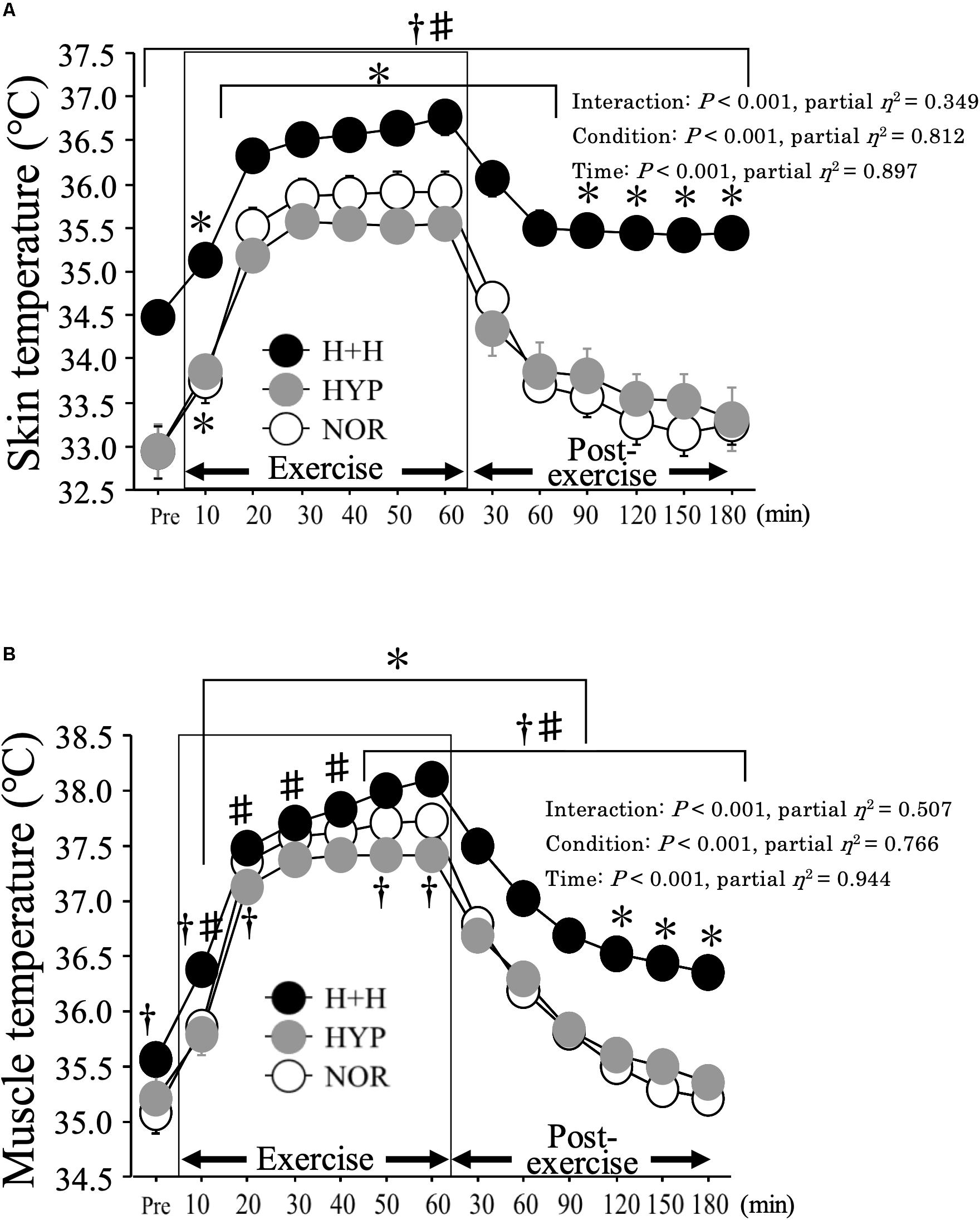
Figure 3. Skin (A) and muscle (B) temperatures during exercise and post-exercise. Values are means ± SE. ∗P < 0.05 vs. Pre. †P < 0.05 vs. NOR. #P < 0.05 vs. HYP. Shadow box indicates exercise duration.
Skin temperature increased during exercise, with a significantly higher average value in H+H (36.3 ± 0.2°C) than in HYP (35.2 ± 0.1°C) and NOR (35.5 ± 0.2°C, P < 0.01, partial η2 = 0.61). During the post-exercise period, the average skin temperature remained significantly elevated in H+H (35.6 ± 0.2°C) compared with HYP (33.7 ± 0.3°C) and NOR (33.6 ± 0.2°C, P < 0.01, partial η2 = 0.82).
Blood Variables
Table 1 presents the blood pO2, pCO2, pH, and HCO3– levels. The blood pO2 level during exercise was significantly higher in NOR than in HYP and H+H. The blood pCO2 level during exercise and post-exercise was significantly lower in H+H than in HYP and NOR. In contrast, the blood pH levels were significantly higher in H+H than in NOR and HYP. The HCO3– level decreased significantly during exercise, and it was significantly lower in H+H than in HYP and NOR.
Table 2 presents the blood glucose, lactate and Hb levels, and Hct value and PV. The blood glucose level decreased significantly during exercise, but there was no significant difference among the three condition. The blood lactate level significantly increased during exercise. However, these values did not significantly differ among the three condition.
The blood Hb level significantly increased during exercise and was significantly lower in H+H than in HYP and NOR at 3 h after completing exercise. The blood Hct value significantly increased under all conditions, and it was significantly lower in H+H than in HYP and NOR at 3 h after completing exercise. PV significantly decreased during exercise, with no significant difference among the three conditions.
Serum GH
Figure 4 shows the changes in serum GH level. During 60 min of exercise, the serum GH level increased significantly during exercise, with higher values in H+H and NOR than in HYP.
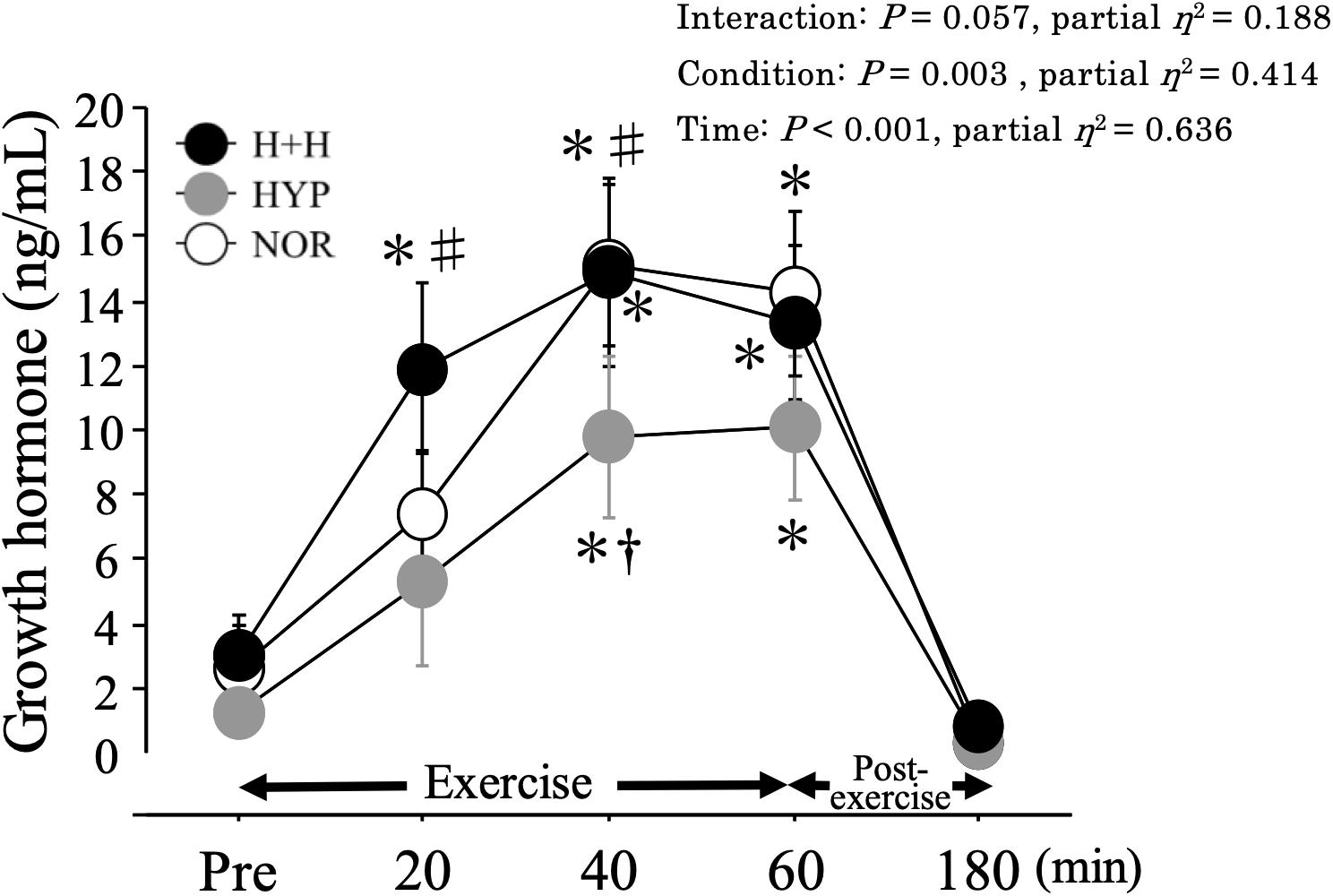
Figure 4. Serum growth hormone level during exercise and post-exercise. Values are means ± SE. ∗P < 0.05 vs. Pre. †P < 0.05 vs. NOR. #P < 0.05 vs. HYP.
Muscle Oxygenation
Figure 5 presents the changes in muscle oxygenation during exercise. The oxy-Hb level during exercise did not differ among the three condition (NOR; 110.3 ± 6.3%, HYP; 113.5 ± 10.0%, H+H; 110.8 ± 7.2% 60 min during exercise). Moreover, the deoxy-Hb level increased during exercise under all conditions, with no significant difference among three conditions (NOR; 182.0 ± 10.8%, HYP; 190.2 ± 10.2%, H+H; 196.7 ± 8.6% 60 min during exercise). The total-Hb level significantly increased during exercise in all conditions, but no significant difference was observed among the three conditions (NOR; 132.3 ± 6.4%, HYP; 136.8 ± 8.5%, H+H; 138.5 ± 6.5% 60 min during exercise). The StO2 decreased significantly during exercise, whereas no significant difference was observed among the three conditions (NOR; 83.2 ± 2.5%, HYP; 81.2 ± 3.6%, H+H; 78.0 ± 2.7% 60 min during exercise).
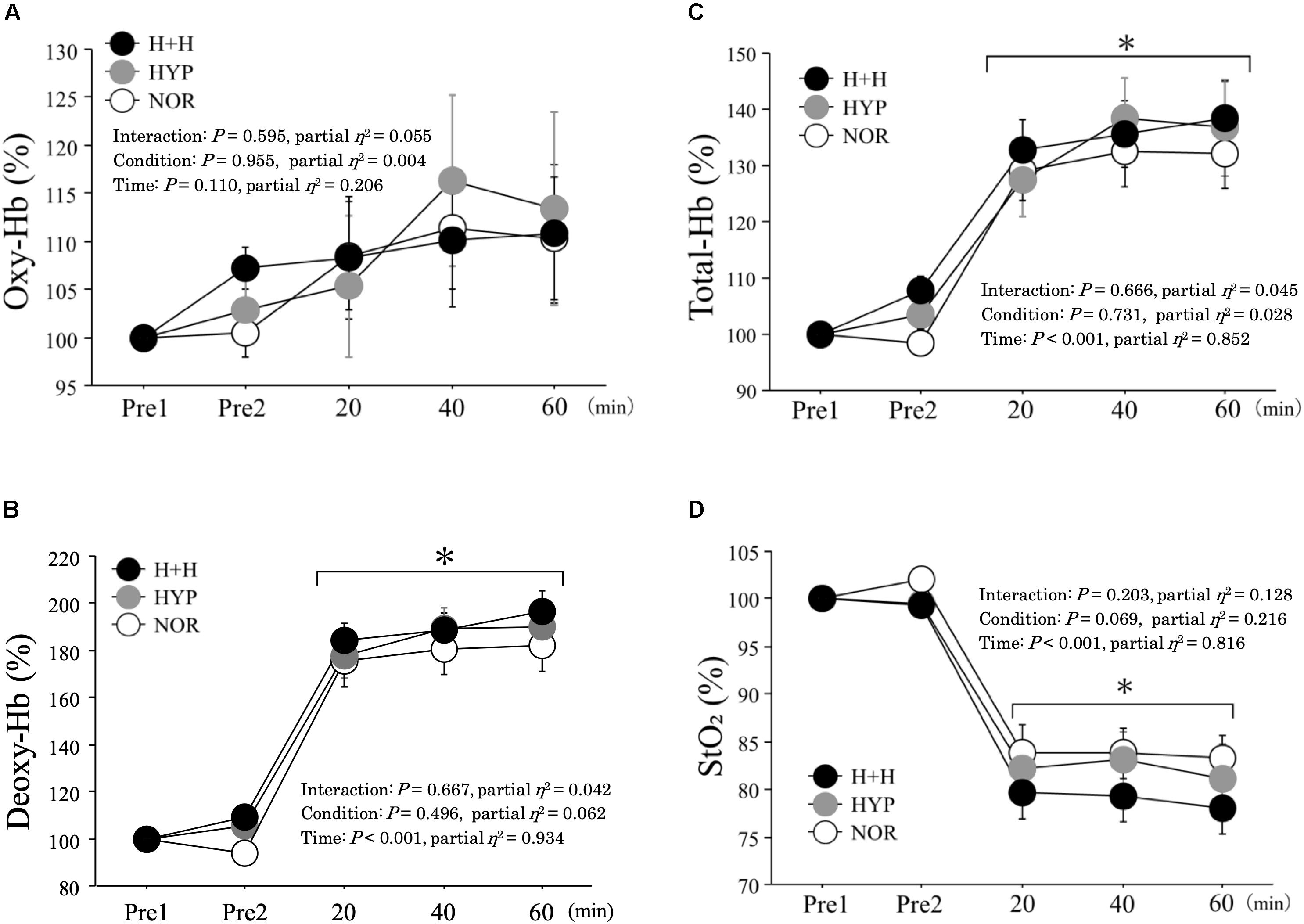
Figure 5. Oxy-hemoglobin (A), deoxy-hemoglobin (B), total-hemoglobin (C), tissue oxygen saturation (D) during exercise. All values were expressed as relative value (values were expressed in refer to pre-exercise value). Pre1 indicates rest under normoxic condition (normal temperature). Pre2 indicates rest under each condition. Values are means ± SE. ∗P < 0.05 vs. Pre1.
Respiratory Gas Parameters During Exercise
Table 3 presents the changes in respiratory gas parameters during exercise. V̇O2 and V̇CO2 values were significantly lower in H+H and HYP than in NOR at all time points. RER was significantly higher in H+H and HYP than in NOR at all time points. In terms of V̇E, it was significantly lower in HYP compared with NOR.
Discussion
The main purpose of the present study was to evaluate the endocrine (in particular EPO) responses to 60 min of endurance exercise under combined hot and hypoxic condition. We found that both the H+H and HYP caused significant increases in the serum EPO level 3 h after completion of exercise, but no difference was observed between H+H and HYP. Moreover, exercise-induced GH elevation was significantly augmented when the hot exposure was combined during exercise in hypoxia. Therefore, additional heat stress during endurance exercise in hypoxia did not affect the erythropoietic response compared with the same exercise under hypoxia alone, but it may be beneficial for increasing GH response.
Endurance exercise in HYP resulted in a significantly higher serum EPO level compared with NOR at 3 h after completion of exercise. This observation is consistent with previous studies reporting a 58% increase after endurance exercise under severe hypoxia (FiO2: 13.2%), as well as 61% (FiO2: 15.9%) and 25% (FiO2: 14.8%) increases after endurance exercise under moderate hypoxia (Mackenzie et al., 2008; Wahl et al., 2013). However, we observed that heat stress during endurance exercise under hypoxia did not further increase the EPO response. Another unique observation in our study was that the SpO2 level during endurance exercise was significantly higher in H+H than in HYP, despite similar FiO2 (14.5%). Body temperature and V̇E were further elevated in H+H than in HYP, leading to an increased blood pO2 level. Abbiss et al. (2007) compared blood pH, partial pressure of arterial oxygen, and partial pressure of arterial carbon dioxide levels during prolonged self-paced cycling under different environmental temperatures (10, 22, and 34°C). They observed that hot trial (34°C) resulted in higher blood pH and lower partial pressure of arterial carbon dioxide levels compared with cooler trials (10 and 22°C). Elevated blood pH during endurance exercise in hot would reflect augmented exercise-induced respiratory alkalosis. Similarly, our H+H condition induced significantly higher blood pH with a lower pCO2 level compared with HYP and NOR. Overall, additional hot exposure during endurance exercise in hypoxia would be expected to attenuate the reduction in SpO2. Therefore, combining hot and hypoxia during endurance exercise may not augment EPO production compared with the same endurance exercise under hypoxia alone. However, in the present study, we did not prepare hot alone condition. Therefore, it remains unclear how the heat stress itself affects EPO response.
Both muscle and skin temperatures increased significantly as exercise progressed under all conditions, but these values were significantly higher in H+H than in both HYP and NOR. Heat stress was rather modest in the present study (32°C) compared with previous studies (>35°C; Hunter et al., 2002; Lorenzo et al., 2010; Guy et al., 2016). However, in H+H, muscle and skin temperatures reached 38.1 ± 0.1°C and 36.2 ± 0.2°C, respectively, by the end of exercise. These levels are comparable with those observed in previous studies, reporting skin temperatures of approximately 35°C during exercise under 35°C (Bradbury et al., 2019). We also evaluated muscle oxygenation because previous studies reported that lower muscle oxygenation (increased muscle deoxygenation) may aggravate the accumulation of exercise-induced metabolites (Grassi et al., 1999). Moreover, the total-Hb level evaluated by NIRS reflects blood volume in muscles (van Beekvelt et al., 2001; De smet et al., 2017). Considering that heat stress increases blood flow due to vasodilatation (Périard et al., 2015) we hypothesized that exercise-induced increases in total-Hb would be augmented in H+H. In contrast to our hypothesis, there was no significant difference among the three conditions in either the muscle oxygenation level or total-Hb level. As potential reasons, the absolute workload was significantly lower in both H+H (19%) and HYP (21%) compared with NOR due to a significantly lower V̇O2max. Moreover, augmented skin blood flow during endurance exercise in hot condition may attenuate muscle blood flow (Périard et al., 2013) leading to the lack of increase in total-Hb in H+H.
We expected the exercise-induced GH elevation would be increased in both H+H and HYP compared with NOR because hypoxia has previously been shown to augment the exercise-induced GH response (Kon et al., 2010, 2015; Kurobe et al., 2015; Filopoulos et al., 2017). Unexpectedly, exercise-induced GH elevation was significantly lower in HYP compared with NOR due to a lower absolute workload (Felsing et al., 1992). However, H+H presented significantly greater GH elevation than HYP, suggesting the benefit of additional hot stress during endurance exercise in hypoxia.
Several limitations should be considered when interpreting our results. First, we did not implement a hot-alone condition. Therefore, it remains unclear whether heat stress alone affects the EPO response. Second, we did not measure core temperature because we used moderate heat stress (32°C). Finally, we evaluated the EPO response to endurance exercise until 3 h after exercise. However, previous studies have shown large interindividual differences in the exercise-induced EPO response (Sinex and Chapman, 2015). Thus, extending measurements during the post-exercise period to beyond 3 h may be required.
Conclusion
The serum EPO level significantly increased with endurance exercise under hypoxic conditions. However, the addition of heat stress during endurance exercise in hypoxia did not augment the EPO response at 3 h after completion of the exercise. Exercise-induced GH elevation was significantly augmented when the hot exposure was combined during endurance exercise in hypoxia. Muscle oxygenation levels during endurance exercise did not differ significantly among the conditions. These findings suggest that combined hot and hypoxic stresses during endurance exercise caused some modifications of metabolic and endocrine regulations compared with the same exercise in hypoxia.
Data Availability Statement
All datasets generated for this study are included in the article/supplementary material.
Ethics Statement
The present study was approved by the Ethics Committee for Human Experiments at Ritsumeikan University, Japan. The patients/participants provided their written informed consent to participate in this study.
Author Contributions
HY and KG designed the study. HY, HM, HI, and NH performed the material preparation, data collection, and analysis. HY, OG, and KG wrote the first draft of the manuscript. All authors read and approved the final manuscript.
Funding
The present study was funded by the Grant-in-Aid for Scientific Research from the Japan Society for the Promotion of Science.
Conflict of Interest
The authors declare that the research was conducted in the absence of any commercial or financial relationships that could be construed as a potential conflict of interest.
Acknowledgments
We thank all participants who participated in the present study. We would like to acknowledge Kazunobu Okazaki, Ph.D. for the excellent support to the present study.
References
Abbiss, C. R., Nosaka, K., and Laursen, P. B. (2007). Hyperthermic-induced hyperventilation and associated respiratory alkalosis in humans. Eur. J. Appl. Physiol. 100, 63–69. doi: 10.1007/s00421-007-0405-z
Bradbury, K. E., Coffman, K. E., Mitchell, K. M., Luippold, A. J., Fulco, C. S., and Kenefick, R. W. (2019). Separate and combined influences of heat and hypobaric hypoxia on self-paced aerobic exercise performance. J. Appl. Physiol. 127, 513–519. doi: 10.1152/japplphysiol.00023.2019
Cheuvront, S. N., Carter, R., and Sawka, M. N. (2003). Fluid balance and endurance exercise performance. Curr. Sports. Med. Rep. 2, 202–208. doi: 10.1249/00149619-200308000-00006
Dalton, R. L., Sowinski, R. J., Grubic, T. J., Collins, P. B., Coletta, A. M., Reyes, A. G., et al. (2017). Hematological and hemodynamic responses to acute and short-term creatine nitrate supplementation. Nutrients 9:1359. doi: 10.3390/nu9121359
De smet, S., Van herpt, P., D’hulst, G., Van thienen, R., Van leemputte, M., and Hespel, P. (2017). Physiological adaptations to hypoxic vs. normoxic training during intermittent living high. Front. Physiol. 8:347. doi: 10.3389/fphys.2017.00347
Dill, D. B., and Costill, D. L. (1974). Calculation of percentage changes in volumes of blood, plasma, and red cells in dehydration. J. Appl. Physiol. 37, 247–248. doi: 10.1152/jappl.1974.37.2.247
Dufour, S. P., Ponsot, E., Zoll, J., Doutreleau, S., Lonsdorfer-Wolf, E., Geny, B., et al. (2006). Exercise training in normobaric hypoxia in endurance runners. I. Improvement in aerobic performance capacity. J. Appl. Physiol. 100, 1238–1248. doi: 10.1152/japplphysiol.00742.2005
Felsing, N. E., Brasel, J. A., and Cooper, D. M. (1992). Effect of low and high intensity exercise on circulating growth hormone in men. J. Clin. Endocrinol. Metab. 75, 157–162. doi: 10.1210/jcem.75.1.1619005
Filopoulos, D., Cormack, S. J., and Whyte, D. G. (2017). Normobaric hypoxia increases the growth hormone response to maximal resistance exercise in trained men. Eur. J. Sport Sci. 17, 821–829. doi: 10.1080/17461391.2017.1317834
Grassi, B., Quaresima, V., Marconi, C., Ferrari, M., and Cerretelli, P. (1999). Blood lactate accumulation and muscle deoxygenation during incremental exercise. J. Appl. Physiol. 87, 348–355. doi: 10.1152/jappl.1999.87.1.348
Guy, J. H., Pyne, D. B., Deakin, G. B., Miller, C. M., and Edwards, A. M. (2016). Acclimation training improves endurance cycling performance in the heat without inducing endotoxemia. Front. Physiol. 7:318. doi: 10.3389/fphys.2016.00318
Haase, V. H. (2013). Regulation of erythropoiesis by hypoxia-inducible factors. Blood Rev. 27, 41–53. doi: 10.1016/j.blre.2012.12.003
Hunter, A. M., St clair Gibson, A., Mbambo, Z., Lambert, M. I., and Noakes, T. D. (2002). The effects of heat stress on neuromuscular activity during endurance exercise. Pflugers. Arch. 444, 738–743. doi: 10.1007/s00424-002-0841-x
Kasai, N., Kojima, C., Sumi, D., Takahashi, H., Goto, K., and Suzuki, Y. (2017). Impact of 5 days of sprint training in hypoxia on performance and muscle energy substances. Int J Sports Med. 38, 983–991. doi: 10.1055/s-0043-117413
Kon, M., Ikeda, T., Homma, T., Akimoto, T., Suzuki, Y., and Kawahara, T. (2010). Effects of acute hypoxia on metabolic and hormonal responses to resistance exercise. Med. Sci. Sports. Exerc. 42, 1279–1285. doi: 10.1249/MSS.0b013e3181ce61a5
Kon, M., Nakagaki, K., Ebi, Y., Nishiyama, T., and Russell, A. P. (2015). Hormonal and metabolic responses to repeated cycling sprints under different hypoxic conditions. Growth Horm. IGF Res. 25, 121–126. doi: 10.1016/j.ghir.2015.03.002
Kurobe, K., Huang, Z., Nishiwaki, M., Yamamoto, M., Kanehisa, H., and Ogita, F. (2015). Effects of resistance training under hypoxic conditions on muscle hypertrophy and strength. Clin. Physiol. Funct. Imaging. 35, 197–202. doi: 10.1111/cpf.12147
Lee, B. J., Miller, A., James, R. S., and Thake, C. D. (2016). Cross acclimation between heat and hypoxia: heat acclimation improves cellular tolerance and exercise performance in acute normobaric hypoxia. Front. Physiol. 7:78. doi: 10.3389/fphys.2016.00078
Levin, B. D. (2002). Intermittent hypoxic training: fact and fancy. High Alt. Med. Biol. 3, 177–193. doi: 10.1089/15270290260131911
Lorenzo, S., Halliwill, J. R., Sawka, M. N., and Minson, C. T. (2010). Heat acclimation improves exercise performance. J. Appl. Physiol. 109, 1140–1147. doi: 10.1152/japplphysiol.00495.2010
Mackenzie, R., Watt, P. W., and Maxwell, N. (2008). Acute normobaric hypoxia stimulates erythropoietin release. High Alt. Med. Biol. 9, 28–37. doi: 10.1089/ham.2007.1043
Mairbäurl, H. (2013). Red blood cells in sports: effects of exercise and training on oxygen supply by red blood cells. Front. Physiol. 4:332. doi: 10.3389/fphys.2013.00332
Maruyama, T., Miuzno, S., and Goto, K. (2019). Effects of cold water immersion and wearing compression garment after eccentric exercise on recovery. J. Exerc. Nutrition Biochem. 23, 48–54. doi: 10.20463/jenb.2019.0007
Millet, G. P., Roels, B., Schmitt, L., Woorons, X., and Richalet, J. P. (2010). Combining hypoxic methods for peak performance. Sports Med. 40, 1–25. doi: 10.2165/11317920-000000000-00000
Morishima, T., Mori, A., Sasaki, H., and Goto, K. (2014). Impact of exercise and moderate hypoxia on glycemic regulation and substrate oxidation pattern. PLoS One 9:e108629. doi: 10.1371/journal.pone.0108629
Nielsen, B., Hales, J. R., Strange, S., Christensen, N. J., Warberg, J., and Saltin, B. (1993). Human circulatory and thermoregulatory adaptations with heat acclimation and exercise in a hot, dry environment. J. Physiol. 460, 467–485. doi: 10.1113/jphysiol.1993.sp019482
Périard, J. D., Cramer, M. N., Chapman, P. G., Caillaud, C., and Thompson, M. W. (2011). Cardiovascular strain impairs prolonged self-paced exercise in the heat. Exp. Physiol. 96, 134–144. doi: 10.1113/expphysiol.2010.054213
Périard, J. D., Racinais, S., and Sawka, M. N. (2015). Adaptations and mechanisms of human heat acclimation : applications for competitive athletes and sports. Scand. J. Med. Sci. Sports. 25, 20–38. doi: 10.1111/sms.12408
Périard, J. D., Thompson, M. W., Caillaud, C., and Quaresima, V. (2013). Influence of heat stress and exercise intensity on vastus lateralis muscle and prefrontal cortex do oxygenation. Eur. J. Appl. Physiol. 113, 211–222. doi: 10.1007/s00421-012-2427-4
Rendell, R. A., Prout, J., Costello, J. T., Massey, H. C., Tipton, M. J., Young, J. S., et al. (2017). Effects of 10 days of separate heat and hypoxic exposure on heat acclimation and temperate exercise performance. Am. J. Physiol. Regul. Integr. Comp. Phys. 313, R191–R201. doi: 10.1152/ajpregu.00103.2017
Schmidt, W., Eckardt, K. U., Hilgendorf, A., Strauch, S., and Bauer, C. (1991). Effects of maximal and submaximal exercise under normoxic and hypoxic conditions on serum erythropoietin level. Int. J. Sports Med. 12, 457–461. doi: 10.1055/s-2007-1024713
Sinex, J. A., and Chapman, R. F. (2015). Hypoxic training methods for improving endurance exercise performance. J. Sport Health Sci. 4, 325–332. doi: 10.1016/j.jshs.2015.07.005
Turner, G., Gibson, O. R., Watt, P. W., Pringle, J. S. M., Richardson, A. J., and Maxwell, N. S. (2017). The time course of endogenous erythropoietin, IL-6, and TNFα in response to acute hypoxic exposures. Scand. J. Med. Sci. Sports 27, 714–723. doi: 10.1111/sms.12700
van Beekvelt, M. C., Colier, W. N., Wevers, R. A., and Engelen, B. G. (2001). Performance of near-infrared spectroscopy in measuring local O2 consumption and blood flow in skeletal muscle. J. Appl. Physiol. 90, 511–519. doi: 10.1152/jappl.2001.90.2.511
Vogt, M., Puntschart, A., Geiser, J., Billeter, R., and Hoppeler, H. (2001). Molecular adaptations in human skeletal muscle to endurance training under simulated hypoxic conditions. J. Appl. Physiol. 91, 173–182. doi: 10.1152/jappl.2001.91.1.173
Wahl, P., Schmidt, A., Demarees, M., Achtzehn, S., Bloch, W., and Mester, J. (2013). Responses of angiogenic growth factors to exercise, to hypoxia and to exercise under hypoxic conditions. Int. J. Sports Med. 34, 95–100. doi: 10.1055/s-0032-1314815
Wakabayashi, H., Osawa, M., Koga, S., Li, K., Sakaue, H., Sengoku, Y., et al. (2018). Effects of muscle cooling on kinetics of pulmonary oxygen uptake and muscle deoxygenation at the onset of exercise. Physiol. Rep. 6:e13910. doi: 10.14814/phy2.13910
Keywords: hypoxia, hot, erythropoietin, endurance exercise, muscle oxygenation
Citation: Yatsutani H, Mori H, Ito H, Hayashi N, Girard O and Goto K (2020) Endocrine and Metabolic Responses to Endurance Exercise Under Hot and Hypoxic Conditions. Front. Physiol. 11:932. doi: 10.3389/fphys.2020.00932
Received: 07 February 2020; Accepted: 10 July 2020;
Published: 19 August 2020.
Edited by:
François Billaut, Laval University, CanadaReviewed by:
Martin Burtscher, University of Innsbruck, AustriaLouise Deldicque, Catholic University of Louvain, Belgium
Copyright © 2020 Yatsutani, Mori, Ito, Hayashi, Girard and Goto. This is an open-access article distributed under the terms of the Creative Commons Attribution License (CC BY). The use, distribution or reproduction in other forums is permitted, provided the original author(s) and the copyright owner(s) are credited and that the original publication in this journal is cited, in accordance with accepted academic practice. No use, distribution or reproduction is permitted which does not comply with these terms.
*Correspondence: Kazushige Goto, kagoto@fc.ritsumei.ac.jp