- Department of Biochemistry, Universityof Nebraska, Lincoln, NE, United States
Liver sinusoidal endothelial cells (LSECs) are the most abundant non-parenchymal cells lining the sinusoidal capillaries of the hepatic system. LSECs are characterized with numerous fenestrae and lack basement membrane as well as a diaphragm. These unique morphological characteristics of LSECs makes them the most permeable endothelial cells of the mammalian vasculature and aid in regulating flow of macromolecules and small lipid-based structures between sinusoidal blood and parenchymal cells. LSECs have a very high endocytic capacity aided by scavenger receptors (SR), such as SR-A, SR-B (SR-B1 and CD-36), SR-E (Lox-1 and mannose receptors), and SR-H (Stabilins). Other high-affinity receptors for mediating endocytosis include the FcγRIIb, which assist in the antibody-mediated removal of immune complexes. Complemented with intense lysosomal activity, LSECs play a vital role in the uptake and degradation of many blood borne waste macromolecules and small (<280 nm) colloids. Currently, seven Toll-like receptors have been investigated in LSECs, which are involved in the recognition and clearance of pathogen-associated molecular pattern (PAMPs) as well as damage associated molecular pattern (DAMP). Along with other SRs, LSECs play an essential role in maintaining lipid homeostasis with the low-density lipoprotein receptor-related protein-1 (LRP-1), in juxtaposition with hepatocytes. LSECs co-express two surface lectins called L-Specific Intercellular adhesion molecule-3 Grabbing Non-integrin Receptor (L-SIGN) and liver sinusoidal endothelial cell lectin (LSECtin). LSECs also express several adhesion molecules which are involved in the recruitment of leukocytes at the site of inflammation. Here, we review these cell surface receptors as well as other components expressed by LSECs and their functions in the maintenance of liver homeostasis. We further discuss receptor expression and activity and dysregulation associated with the initiation and progression of many liver diseases, such as hepatocellular carcinoma, liver fibrosis, and cirrhosis, alcoholic and non-alcoholic fatty liver diseases and pseudocapillarization with aging.
Introduction
The liver is considered a crucial organ of the body due to its involvement in numerous processes, such as metabolism, immunity, detoxification, nutrient storage, among others. The liver is composed primarily of four distinct cell types, differentiated into two categories as parenchymal cells (PC, 60–80%) and non-parenchymal cells (NPC 20–40%). The NPC population is composed of liver sinusoidal endothelial cells/LSECs (50%), Kupffer cells/KCs (20%) and stellate cells (<1%). The remaining NPCs are composed of lymphocytes (25%) and biliary cells (5%) (Racanelli and Rehermann, 2006). The role of hepatocytes, KCs and stellate cells in maintaining liver homeostasis is well documented. However, the LSEC, is the most understudied due to technical challenges in purification and culturing ex vivo.
Prof. Eddie Wisse first proposed the ultrastructure of liver sinusoidal endothelial cells (LSEC) in 1970, which differentiated LSECs from KCs and paved the way for future study on LSECs and elucidation of their function (Wisse, 1970, 1972). A few years later, several research groups developed LSEC isolation techniques and identified their role in the uptake of various substances in vitro (Seglen, 1976; Smedsrod et al., 1984). In the early 1980s, LSECs were identified as a significant clearance site for blood-borne hyaluronan, which established their role as scavenger cells (Fraser et al., 1981). This led to an increase in interest among scientists from other discipline, such as immunology, virology, cancer, and more to further comprehend the various roles performed by LSECs. This review focuses on the detailed description of LSEC morphology and their scavenger, adhesion and other prominent receptors that define their functional roles in the hematological and hepatic systems during health and disease.
LSEC Morphology
Liver sinusoidal endothelial cells (LSECs) form the inner lining of liver sinusoidal blood vessels or capillary bed which serves as the site for mixing nutrient-rich blood from the hepatic portal vein and oxygen-rich blood from the hepatic artery (Sorensen et al., 2015). Here, the LSECs assist in clearing macromolecular waste (extracellular matrix material and foreign molecules) from the blood and regulate hepatic vascularity. Individual LSEC’s are flat and very small in size, no thicker than 5 μm at the center and 0.3 μm at the periphery (Wisse, 1970, 1972; Smedsrod et al., 1988b; Falkowska-Hansen et al., 2007). Cytoplasmic projections, such as filopodia, lamellipodia, and microvilli are absent in LSECs, giving them a smooth appearance. LSECs contain numerous fenestrae (small open pores) that facilitate the selective exchange of molecules between the blood and underlying stellate and hepatocytes (Wisse et al., 1985; Braet and Wisse, 2002). Fenestrae are located in the cytoplasm of LSECs and usually are 50–200 nm in diameter and organized in groups known as sieve plates (Wisse et al., 1985). They are also distributed individually on the surface of endothelium or organized in a labyrinth or mesh-like structure (Taira, 1994; Braet et al., 2007, 2009; Figure 1). LSECs do not have a basement membrane or basal lamina and diaphragm, permitting direct access of solutes to the perisinusoidal space or the Space of Disse. The lack of a basal lamina has also been identified in several animals, such as chickens and bony fish (Fraser et al., 1986; Eng and Youson, 1992). The Space of Disse hosts the stellate cells as well as hepatocyte microvilli. During fibrosis or chronic inflammation, activated stellate cells contribute to the deposition of extracellular matrix in the Space of Disse, forming a continuous basal membrane. This new basal lamina is mostly composed of collagen that inhibits the permeability of the Space of Disse and reduces the solute exchange between the parenchyma and blood (Han et al., 2001; Mak et al., 2012).
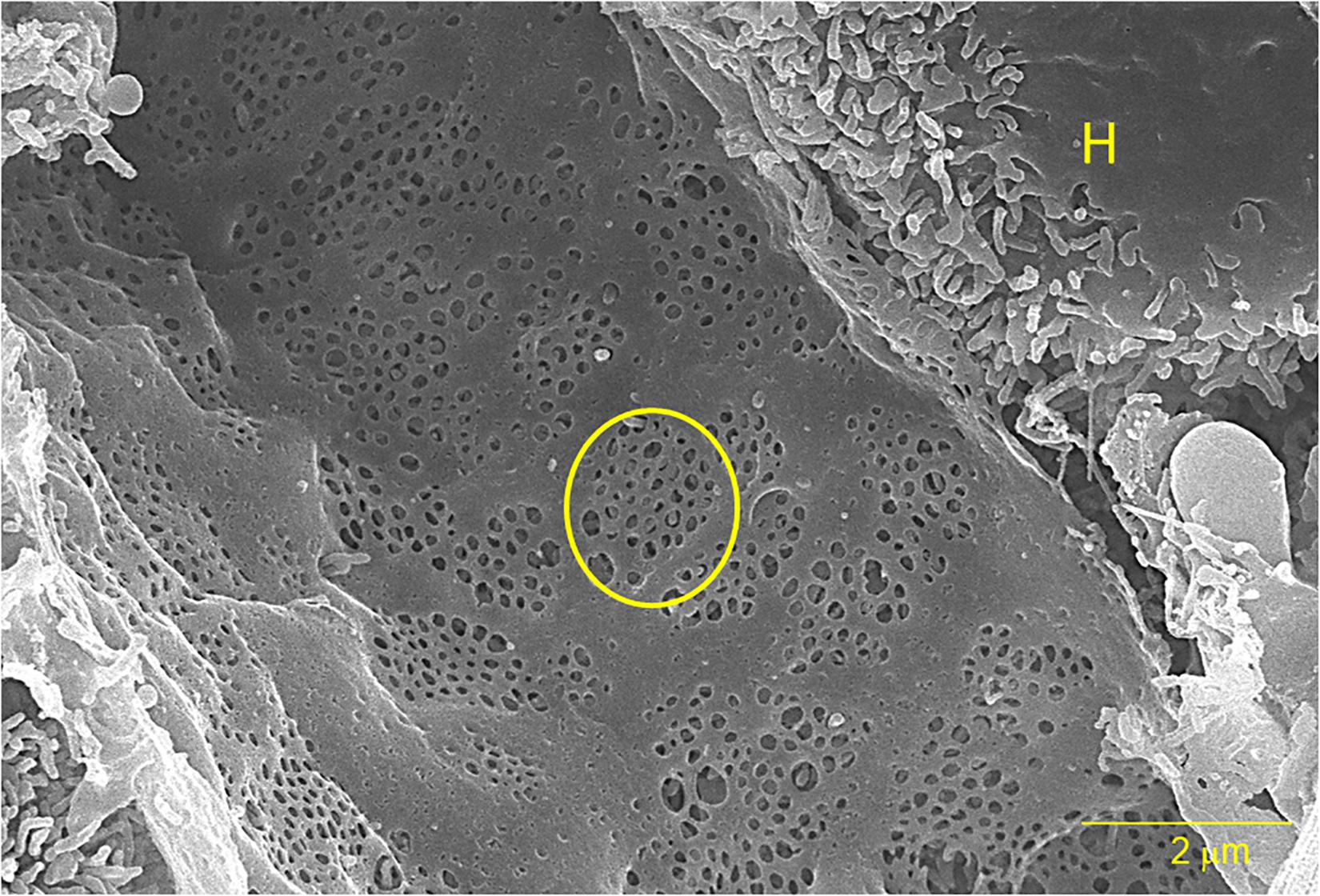
Figure 1. A scanning electron micrograph of a sliced rat liver at 10,000× magnification. The yellow circle outlines one of several sieve plates and the “H” indicates an adjacent hepatocyte. The bar represents 2 μm.
LSEC’s are unique because of their plentiful fenestrae. The LSEC fenestrae can change their diameter in response to the cellular environment (Wisse et al., 1996; Braet and Wisse, 2002). The mechanism for fenestral contraction and dilation was first discovered by researchers in the 1980s and is now known as a dynamic process (Monkemoller et al., 2015; Zapotoczny et al., 2019). Fenestrae is surrounded by actin filaments, suggesting the role of cytoskeleton is central in the formation and maintenance of fenestrae. This idea was further supported by several researchers in the successive years as immunofluorescence microscopic study substantiated the presence of actin, myosin, microtubule and calmodulin as necessary in forming these structures (Wisse, 1970; Braet and Wisse, 2002). Actin, myosin, and calmodulin influence the diameter of each pore and determine how long it persists. Serotonin has also been found to regulate contraction of fenestrae by increasing the intracellular calcium concentration (Gatmaitan et al., 1996; Yokomori, 2008; Furrer et al., 2011). Increase in the thickness of LSECs, unexpected formation of basal lamina and reduction in the number of pores is known as defenestration. Defenestration of LSECs gives rise to pseudocapillarization, a disorder evident in liver fibrosis, atherosclerosis and other aging associated diseases (Le Couteur et al., 2001, 2007). Fenestrae is maintained cooperatively by hepatocytes and stellate cells mediated paracrine and autocrine signaling (DeLeve et al., 2004). Vascular endothelial growth factor (VGEF), a hormone that functions in blood vessel growth, promotes paracrine signaling from hepatocytes and stellate cells stimulates autocrine signaling of nitric oxide (NO) from LSECs. Animals lacking VEGF tend to exhibit increased defenestration in their LSECs, suggesting that this molecule plays a mechanistic role in either their formation or maintenance (Kus et al., 2019).
Defenestrated LSECs results in the reduction of hepatic uptake of lipoproteins and is one of the causes of hyperlipoproteinemia (Fraser et al., 2012). Similarly, defenestration has also been observed to play an important role in the progression of non-alcoholic fatty liver disease (NAFLD). Miyao et al. demonstrated that since LSECs are a “gatekeeper” for the liver parenchyma, their injury during early stages of NAFLD determines the severity of subsequent injury and progression of NAFLD (Miyao et al., 2015; Shetty et al., 2018). In the past few years, some of this data has been called into question in that some have observed no difference in NAFLD and fenestration levels of LSECs and that LSECs have a more calming effect on injured hepatocytes and activated stellate cells (Kus et al., 2019). This idea is reinforced in a study of autoimmune hepatitis in which human LSECs in biopsies from young people less than 20 years old were assessed for damage and the formation of a basement membrane. Defenestration did occur in about half of the subjects, but little to no basement membrane formed in the Space of Disse suggesting that LSECs have regenerative properties and may soften the assault on the liver (Lotowska et al., 2018). While there was no causative relationship proven, the jury is still out on whether progressive NAFLD is associated with defenestration.
Another salient characteristic of LSECs that differentiate them from other endothelial cells is their higher endocytic ability. LSECs only make up about 3% of total liver volume, however, they contribute to about 45% of pinocytic vesicles in the liver (Blouin et al., 1977). A study was conducted to elucidate the mechanism of cross presentation by LSECs as a link was found between endocytosis and antigen presentation (Burgdorf et al., 2008). This study reported that LSECs were most efficient at internalizing circulating antigen in the blood as compared to dendritic cells (DC) or macrophages of the spleen as well as by KC and DC of the liver (Schurich et al., 2009). LSECs are well equipped with high affinity endocytic scavenger receptors and lysosomal activity that helps in the internalization and catabolization of a large number of waste substances (Table 1) as well as small colloidal particles (Knook and Sleyster, 1980; Juvet et al., 1997; Kawai et al., 1998; Elvevold et al., 2008a).
Kjeken et al. (2001) showed that a clathrin-dependent mechanism is used by LSECs for fluid phase endocytosis. Furthermore, they found that LSECs have higher expression of clathrin protein and twice the number of clathrin-coated pits as compared to KC or hepatocytes. Several years later, Falkowska-Hansen et al. (2007) reported an interesting finding about clathrin-coated vesicles in primary rat LSECs. They found that clathrin heavy chain (CHC) is distributed as net-like structure which was unique to primary rat LSECs. They also showed the co-localization of CHC with microtubules. Furthermore, they found that clathrin-coated vesicle (CCV) function is dependent on microtubules as disruption of microtubules resulted in dysregulation of intracellular transport as well as aberrant signaling of organelles involved in clathrin-mediated endocytosis in LSECs (Falkowska-Hansen et al., 2007). Experiments evaluating the endocytosis capacity of primary LSECs in vitro must be performed the same day or within 24 h of purification of the LSECs. After this time period, endocytosis sharply decreases and ceases altogether around day 4 (Braet et al., 1994). Interestingly, the disappearance of fenestrae takes place over the same time course (Braet et al., 2005). To date, the record holder for maintaining LSECs in culture with their native endocytic capacity is the Smedsrod group using an animal-free medium which allowed endocytosis to occur out to 30 days using a pig model (Elvevold et al., 2005). It should be kept in mind that the study of endocytosis in LSECs must be performed with the freshly purified primary cells as no cell line to date expresses all of the specialized receptors nor morphological features that make these cells so dynamic.
Recently Established Nomenclature for Scavenger Receptors
Scavenger receptors are defined as protein receptors that bind to a broad range of ligands from exogenous sources (bacteria, yeast, viruses) and modified endogenous sources [oxidized lipoprotein, advanced glycation end (AGE) products, etc.]. In 2017, a workshop was organized by the National Institute of Allergy and Infectious Diseases at the National Institutes of Health in the United States to develop a clear definition of the various groups of scavenger receptors known to date. This group categorized the scavenger receptors in 11 different classes (A through L) depending on their structure and function and clarified the nomenclature of the various receptors (PrabhuDas et al., 2017). This review will only discuss the role and function of scavenger receptors in the liver sinusoids. LSECs are known to express SR-A, two SR-B variants (SR-B1 and SR-B2/CD36), mannose receptor/CD206/SR-E3, SR-H1 and SR-H2, FcγRIIb and others under normal conditions (Table 1 and Figure 2). LSECs are also known to express SR-E1 under various pathological conditions. Here, an overview of LSEC scavenger receptors are presented.
Scavenger Receptor A (SR-A)
The class A scavenger receptors (also known as MSR1, SR-AI, SCARA1) were first cloned by the Kreiger group (Kodama et al., 1990; Rohrer et al., 1990) and may have been involved with binding to modified low-density lipoproteins. Scavenger receptor-A (SR-A) is a type II trimeric integral plasma membrane receptor that is encoded on chromosome 8 in both humans and mice. It is characterized by the following six domains: a transmembrane region, a spacer region, a coiled-coil region, a collagenous stretch of repeated Gly-X-P/K, an N-terminal cytoplasmic tail, and a carboxyl terminal-type specific-domain (Figure 2). Alternative splicing results in three variants of SR-A, namely SR-A1.1 and SR-A1.2 (Emi et al., 1993). The structure of the three isoforms are very similar and the differences lie in their cysteine-rich carboxy terminal domain which is involved in cell adhesion and, possibly, bacterial binding (Bowdish and Gordon, 2009; Yap et al., 2015). SR-A type-I is characterized with a cysteine rich carboxy terminal domain whereas SR-A type-II has short C-terminal domain lacking cysteine-rich site at the C-terminus (Rohrer et al., 1990). SR-A type-III has a truncated cysteine-rich domain (Gough et al., 1999). SR-A type1/1.1 are involved in the binding of diverse macromolecules, such as acetylated and oxidized low density lipoprotein (ac/oxLDL) (Suzuki et al., 1997; Kunjathoor et al., 2002), β-amyloid fibrils (El Khoury et al., 1996), AGE products (Araki et al., 1995), molecules present on the surface of gram negative and positive bacteria, such as lipopolysaccharide (LPS) and lipoteichoic acid (LTA) (Dunne et al., 1994). Rohrer et al. (1990) demonstrated that the fibrous coiled-coil and collagen-like domains are responsible for binding different ligands in SR-A type-1/1.1 as removal of cysteine-rich C-terminal domain in SR-A type-II did not affect its binding capacity. SR-A type-1.2 does not bind to SR-A type-1/1.1 ligands but it has been shown to negatively regulate the functions of SR-A type-1/1.1 in vitro (Gough et al., 1999).
SR-A expression was once thought to be only expressed in macrophages/KCs, but recent work have shown their expression in brain microglia and astrocytes, LSECs and vascular smooth muscle cells (Nagelkerke et al., 1983; Hughes et al., 1995; Christie et al., 1996; Gough et al., 1999; Godoy et al., 2012). Work conducted in rats have shown that SR-A expressed on LSECs are primarily responsible for carrying out the uptake of the artificial ligand, acLDL, in liver (Nagelkerke et al., 1983). They also theorized the role of an acLDL receptor present on LSECs in the prevention of accumulation of cholesterol under normal condition, though the pathways involved remain elusive. Similarly, another report has demonstrated the uptake of ac/oxLDL by two scavenger receptors; an unidentified 95 kDa protein and the likely candidates, SR-A1/1.1 present on rat LSECs and KCs (De Rijke et al., 1992, 1994; De Rijke and Van Berkel, 1994). In 1990, a commentary in Nature written by Brown and Goldstein had attempted to bring about a universal idea or theory involving the numerous ligands including LDLs that bind to a few scavenger receptors, of which SR-A was a very likely candidate (Brown and Goldstein, 1990). Furthermore, several studies have shown the elevated expression of SR-A in atherosclerotic lesions (Matsumoto et al., 1990) and accumulation of oxLDL, a SR-A ligand in plaques, suggesting it’s putative role in atherogenesis (Hiltunen et al., 2001). SR-AI in LSEC is also involved in uptake and degradation of malondialdehydes-acetaldehyde-serum albumin (MAA), which is considered immunogenic and capable of producing inflammatory responses in the liver (Duryee et al., 2005). It has also been observed that chronic ethanol administration diminishes the uptake of MAA by SR-A in rats. This leads to the accumulation of acetaldehyde and aldehyde modified protein adducts in the circulation. These adducts cause secretion of monocyte chemoattractant protein (MCP)-1 and macrophage inflammatory protein (MIP)-2 via hepatic stellate cells, contributing to alcoholic liver disease (Kharbanda et al., 2001).
Despite all of the evidence that SR-A receptors were involved with the uptake and catabolism of LDLs, the use of knock-out mice in the late 1990’s demonstrated that the SR-A receptors had a negligible effect on liver uptake and decay of serum acLDL (Ling et al., 1997; Van Berkel et al., 1998). Furthermore, SR-A1/1.1-deficient and wild type (WT) mice were compared with the liver sequestration of other non-parenchymal ligands, namely AGE, N-terminal propeptide of type III procollagen (PIIINP) and formaldehyde-treated serum albumin. The results indicated that the SR-A receptors were of minor importance for plasma clearance of these ligands and the distribution in other tissues and organs was not altered (Hansen et al., 2002).
Scavenger Receptor B (SR-B)
Scavenger receptor class B type 1 (SR-B1/SCARB1) is a member of scavenger receptor B, which is located on chromosome 12 in humans and chromosome 5 in mice (Acton et al., 1994). SR-B1 is a membrane glycoprotein consisting of two short cytoplasmic N- and C-terminal domains, two short hydrophobic membrane regions, and a highly N-glycosylated large looped extracellular domain (Krieger, 1999). They exist as two isoforms, SR-B1 and-B1.1, resulting from alternative splicing (Webb et al., 1997). CD36 and LIMPII Analogous (CLA-1) is the human homolog of SR-B1 (Calvo and Vega, 1993). Liver and steroidogenic tissues (adrenal glands, ovaries, and testis) have been shown to highly express SR-B1 (Acton et al., 1996). Malerod et al. (2002) were the first group to demonstrate the expression of SR-B1 in LSECs from isolated rat liver. Several year later, Ganesan et al. (2016) showed the abundant expression of SR-B1 in mouse LSECs by confocal microscopy. Mutational and knock-out studies have suggested an important role of the SR-B1 receptor in facilitating high density lipoprotein (HDL) uptake in liver and steroidogenic tissues, thereby, its role in atherosclerosis regulation (Acton et al., 1996; Kozarsky et al., 1997; Varban et al., 1998). Other than HDL, SR-B1 also binds to oxLDL, apoptotic cells, unmodified LDL, VLDL (Kozarsky et al., 1997) as well as vitamin E (Reboul et al., 2006), carotenoids (During et al., 2005), and silica (Tsugita et al., 2017). The liver is the primary organ involved in LPS clearance and LSECs are a major contributor for this activity. SR-B1 may be involved with some of the LPS clearance, but as of this date, that is not very clear. There may be a multitude of receptors or “built-in redundancy” in this LPS clearance system so that LPS levels from the gut never get high enough to cause acute inflammation (Ganesan et al., 2016).
CD36 is another member of the Scavenger Receptor B family, which is located on chromosome 7 and chromosome 5 in humans and mice, respectively (Cao et al., 1997). The entire extracellular domain of CD36 and SR-BI share high sequence homology, though the difference lies in their transmembrane and cytoplasmic domain sequences (Acton et al., 1996). It is expressed on adipocytes, capillary endothelial cells, heart and skeletal muscles, and platelets (Talle et al., 1983; Tandon et al., 1989b; Abumrad et al., 1993; Greenwalt et al., 1995; Febbraio et al., 2001) and abundantly expressed on LSECs in the liver (Strauss et al., 2017). The role of CD36 in the metabolism of lipoprotein is well-documented (Febbraio and Silverstein, 2007; Jay and Hamilton, 2018). One study demonstrated the role of CD36 in the uptake of HDL by hepatic NPCs, and their CD36 knock-out mouse model resulted in a modest but significant decrease in the HDL uptake in both hepatocytes and NPCs (Brundert et al., 2011). Similar to SR-B1, CD36 also binds to LDL, VLDL (Calvo et al., 1998), apoptotic cells (Savill et al., 1991) as well as anionic phospholipids (Rigotti et al., 1995), and collagen (Tandon et al., 1989a). Aldehyde modified proteins are likely taken up by CD36 as the SR-A KO mice had significant, but decreased uptake of these proteins (Duryee et al., 2005). An earlier study showed the involvement of CD36 in the endocytosis and degradation of AGE products, implicating its role in diabetes (Ohgami et al., 2001). However, Nakajou et al. (2005) demonstrated that CD36 is not involved in the endocytosis of AGE proteins in LSECs, suggesting the involvement of other scavenger receptors. This is quite plausible since “AGE” is a catch-all for all glycation modifications that occur involving reducing sugars and independent laboratories likely make and use their own “AGE modified protein” preparations.
Upregulation of SR-B1 expression has been documented in the livers of mice fed with a high-fat diet as compared to control mice, suggesting crucial involvement of SR-B1 in NAFLD pathogenesis (Qiu et al., 2013). Translating that to humans, a similar higher SR-B1 expression was observed in type 2 diabetic patients, but no change in hepatic SR-B1 was observed in NASH and hypercholesterolemia patients, predicting only a minimal role of SR-B1 in NAFLD (Rein-Fischboeck et al., 2015). Patients manifesting severe alcoholic hepatitis, inflammation and oxidative stress symptoms had a correlation with a decrease of circulating paroxanase/arylesterase 1 (PON1) in the blood and stimulated alternatively activated (M2) macrophages through activation of CD36. The idea here is that activated macrophages internalize increased amounts of oxLDLs via CD36 and SRA1 and that contributes to the pathophysiology of severe alcoholic hepatitis (Maras et al., 2019). However, none of the studies have documented the contribution of SR-B1 or CD36 expressed on LSECs in maintaining a homeostatic environment in the liver or a modulation in their expression in various diseases.
Scavenger Receptor E (SR-E)
SR-E1/LOX-1, also known as lectin-type oxidized LDL receptor or oxidized LDL receptor 1 (OLR1) is a type II transmembrane protein belonging to scavenger receptor E family. It is located on chromosome 12 in humans and chromosome 6 in mice. It consists of a transmembrane domain, a short cytoplasmic domain at the N-terminus, a connecting neck domain and a C-type lectin-like extracellular domain at the C-terminus (Sawamura et al., 1997; Park et al., 2005). Maturation of Lox-1 requires post-translational N-linked glycosylation of the extracellular domain at the C-terminus and this modification was shown to be important for binding of various ligands, intracellular transport, signaling processes, and several other biological functions (Kataoka et al., 2000). Mutational studies have shown the importance of conserved C-terminal residues in the lectin like domain in LOX-1 for ligand binding (Chen et al., 2001b). LOX-1 is expressed by endothelial cells, macrophages, vascular smooth muscle cells, adipocytes and chondrocytes in low levels (Sawamura et al., 1997; Yoshida et al., 1998; Chui et al., 2005; Akagi et al., 2006). However, an increase in expression is seen by various pro-inflammatory markers, shear stress and mechanical stimuli, such as tumor necrosis factor-alpha (TNF-α), and phorbol 12-myristate 13-acetate (PMA) in vitro (Kume et al., 1998; Murase et al., 1998). Despite having no homology with other oxLDL binding SRs, such as SR-A and B, it is considered as the major endothelial SR for binding, uptake, and degradation of oxLDL (Sawamura et al., 1997). Other than oxLDL, LOX-1 is also involved in the binding with apoptotic and aged cells, C-reactive protein (CRP), bacteria, platelets and anionic phospholipids, such as phosphatidylserine and phosphatidylinositol, suggesting their role in various physiological conditions (Oka et al., 1998; Li and Mehta, 2000; Chen et al., 2001a; Shih et al., 2009).
Under normal conditions, LOX-1 is expressed in low amounts, in contrast to its high expression during various pathophysiological events (Ogura et al., 2009; Balzan and Lubrano, 2018). A study has documented an increased expression of LOX-1 in the aorta of hypercholesterolemic, hyperlipidemic rabbits suggesting that a Western diet may induce this receptor for the overall metabolism of lipids (Chen H. et al., 2000; Chen M. et al., 2000). Another study was conducted on a hypertensive rat model using Dahl salt-sensitive and Dahl salt-resistant rats. Dahl salt sensitive rats develop hypertension when fed with a high salt diet and Dahl salt resistant rats show minor blood pressure changes on the same diet. They reported up-regulation of LOX-1 expression in hypertensive rats, thereby, suggesting its role in hypertension and a putative target for treating atherosclerosis (Nagase et al., 1997, 1998). The role of LOX-1 contributes to the pathology NAFLD resulting, in part, from LSEC dysfunction. Zhang et al. (2014) studied the effect of human LSEC LOX-1 gene knockdown in oxLDL-induced hepatic injury. They found an increase in ROS production and enhanced p65 expression in oxLDL treated human LSECs. This effect was significantly reduced after LOX-1 siRNA treatment, suggesting the role of LOX-1 in activation of NF-kB and the ROS pathway. A reduction in the expression of eNOS was found in human LSEC culture treated with oxLDL, whereas, LOX-1 siRNA treatment resulted in an enhanced expression of eNOS in this culture system. They also reported a reduction in the number of fenestrae, diameter and porosity in ox-LDL-treated human LSEC culture and LOX-1 siRNA resulted in reversal of these effects, suggesting its role in stress related atherogenesis (Fraser et al., 1995). LSEC defenestration was found to be mediated by LOX-1 by upregulating ET-1 and caveolin-1. Although, the association between LOX-1, ET-1, and caveolin-1 remains unclear, oxidative stress generated due to ROS production suggests the role of LOX-1 in NASH and NAFLD pathological manifestations (Pasarin et al., 2012). Along with SR-A, LOX-1 was also shown to be responsible for degradation of MAA-Alb (Duryee et al., 2005). Furthermore, a study conducted on aortic endothelial cells reported a reduction in LOX-1 expression with aging and associated it with the progression of aging related diseases (Khaidakov et al., 2011).
Mannose receptor (MR)/CD206/SR-E3 is another member of the scavenger receptor E belonging to the C-type lectin family. It is a type I integral membrane protein which is present on chromosome 10 in humans and chromosome 2 in mice. The mannose receptor is composed of an N-terminal extracellular region and a C-terminal intracellular region. The N-terminus is composed of three distinct domains and each domain binds to its specific ligands. First, eight consecutive C-type carbohydrate recognition domains (CRDs) that bind to terminal mannose residues, N-acetylglucosamine and L-fucose (Ezekowitz et al., 1990; Taylor et al., 1992). Second, a fibronectin type-II repeat domain that binds to collagen I-IV (Martinez-Pomares et al., 2006; Napper et al., 2006). Third, a cysteine-rich domain at the N-terminus binds to sulfated sugars, such as GalNAc-4-sulfate and chondroitin sulfates A and B (Fiete et al., 1998). Besides binding of specific ligands by their respective domains, MR is involved in the binding of numerous endogenous ligands, such as lysosomal hydrolases (Stahl et al., 1976; Elvevold et al., 2008a), tissue plasminogen activator (tPA) (Smedsrod et al., 1988a), and Procollagen type I carboxy-terminal propeptide (PICP) (Smedsrod et al., 1990). MR has been implicated in the binding of several pathogens including Candida albicans, Pneumocystis carinii, and Leishmania donovani by its cysteine-rich domain (Stahl and Ezekowitz, 1998; Gordon, 2002; Allavena et al., 2004). More recent studies have reported MRs binding with influenza, herpes simplex virus as well as HIV (Milone and Fitzgerald-Bocarsly, 1998; Reading et al., 2000; Turville et al., 2002) with varying affinities. The MR is expressed in most tissue macrophages, LSECs, lymph node and spleen; kidney mesangial cells, and dendritic cells subsets (Linehan et al., 1999; McGreal et al., 2004; Linehan et al., 2005).
Due to their ability to recognize and bind with carbohydrate moieties present on the surface of pathogens, MR expressed on LSECs is involved in the clearance of denatured collagen (Malovic et al., 2007). This study showed a reduction in plasma clearance of radiolabeled DebColl, a heat-denatured type-1 collagen, in a MR KO mouse model in vivo. LSECs isolated from MR KO mice were not able to internalize radiolabeled DebColl as efficiently as WT LSECs in vitro. Reduction in the endocytic capacity of LSECs for removing denatured collagen might contribute to the onset of pseudo-capillarization or fibrosis (Ala-Kokko et al., 1987). Subsequently, Elvevold et al. (2008a) reported the importance of MR in maintaining the high lysosomal degradation capacity using the MR knock-out model. They found a reduction in lysosomal enzymes in freshly isolated LSECs from MR KO mice as compared to WT. They further examined the endocytic and intracellular degradative capacity of LSECs and reported that MR KO mice took twice as long to degrade injected radiolabeled formaldehyde-treated serum albumin (FSA) as compared to WT, though endocytic capacity remained the same for both cell types. In accordance with the former, the MR KO mice contained nearly twice as much radioactivity than the WT mice 2 h-post-injection. It was concluded that the cellular uptake of SR ligand is not hampered in MR KO mice and there is only a deficiency in the amount of lysosomal enzyme content, hence, reduced degradative capacity in MR KO mice. This demonstrates that the MR is involved with the turnover and homeostasis of many intrinsic molecules within the mammalian organism.
Several studies have shown the expression of MR on LSEC is impacted by cytokines and inflammatory stimuli. Asumendi et al. (1996) demonstrated the upregulation of the LSEC MR after exogenous administration of human recombinant IL-1b in the rat in vivo. IL-1b is a pro-inflammatory cytokine present in acute infections. A similar upregulation was observed in the LSEC MR when IL-1b was induced with LPS in the rat endogenously (Asumendi et al., 1996). Similarly, enhanced MR expression by LSECs in culture when incubated with IL-10 or IL-4 with IL-13 (Liu Y. et al., 2013) acts contrary to IL-1b and is involved with the “type-II” activation of the immune system. Similarly, a study on mouse model of C26 colon carcinoma hepatic metastasis has shown that MR expression level and endocytic capacity increases with an increase in the production of IL-1b from LSECs. The increased production of IL-1b resulted from the binding of ICAM-1 expressed on LSECs with LFA-1 on C26 colon carcinoma cells (Arteta et al., 2010). This activity decreased secretion of IFN-γ as well as anti-tumor cytotoxicity. This study discovered the role of MR in promoting IL-1b and ICAM-1 mediated pro-metastatic effects in the liver.
Scavenger Receptor H (SR-H)
Stabilin (or FEEL/CLEVER/HARE) receptors are class H scavenger receptors. They are type I transmembrane proteins consisting of 20–21 EGF/EGF-like domains, seven fasciclin-1 domains, an X-linked domain, a transmembrane region, and a short cytoplasmic domain (Politz et al., 2002). This family consists of two members; Stabilin-1 and Stabilin-2. Stabilin-1 is also known as SR-H1/MS-1/FEEL-1/CLEVER-1. Stabilin-2 is also known as SR-H2/FEEL-2 and HARE, which is a shorter isoform of Stabilin-2 generated by proteolytic cleavage (Goerdt et al., 1991; Weigel, 2019). The main structural difference between Stabilin-1 and Stabilin-2 is the presence of 20 EGF-like domains in stabilin-2 as compared to 21 domains in stabilin-1. Stabilin-1 is located on chromosome 3 and chromosome 14 in humans and mice, respectively. Stabilin-2 is located on chromosome 12 in humans and chromosome 10 in mice, respectively. Their extracellular domains share 55% similar homology, but their short intracellular domains are highly diverse, which results in differential abundance in different tissues and cells (Harris and Cabral, 2019). Sinusoidal endothelial cells in the liver, spleen, adrenal cortex and tissue macrophages express Stabilin-1 (Goerdt et al., 1991; Kzhyshkowska et al., 2006a), whereas, the expression of stabilin-2 is abundant in the sinusoids of liver, spleen, lymph node and bone marrow (Yannariello-Brown et al., 1997; Weigel and Weigel, 2003; Qian et al., 2009). Both receptors are also expressed in several other tissues throughout the body at lower levels (Falkowski et al., 2003). Stabilin-1/2 are considered the primary scavenger receptors of LSECs and are responsible for the binding, uptake, and degradation of multifarious ligands, such as hyaluronan, N-terminal pro-peptide of type I procollagen (PINP) (Yannariello-Brown et al., 1997; McCourt et al., 1999), chondroitin sulfates (Harris et al., 2004), AGE (Li R. et al., 2009), oxLDLs (Li et al., 2011), SPARC (Kzhyshkowska et al., 2006b), heparin (Harris et al., 2008), von Willebrand factor-factor VIII (Swystun et al., 2018) and synthetic phosphorothioate antisense oligonucleotides (Miller et al., 2016).
Stabilin-1, and to some extent, Stabilin-2, in LSECs, are specifically involved in the uptake of oxLDL, thus playing a role in the prevention of atherogenesis (Li et al., 2011). The Stabilin receptors are also involved in the internalization and clearance of various macromolecules that cannot be cleared by the kidney due to size limitation for some of these macromolecules. In 2011, it was reported that mice lacking both Stabilin-1 and Stabilin-2 did not live as long as their WT littermates due to mild perisinusoidal liver fibrosis and severe glomerular fibrosis. This study showed that both Stabilin-1 and -2 are essential for the normal clearance of extracellular matrix material, thus preserving the homeostasis of the liver as well as other distantly located organs (Schledzewski et al., 2011). In humans, it is not known what occurs in Stabilin allelic insufficiency or loss of function. However, in de-differentiated tissues in cases of liver cancer, loss in the expression of Stabilin-1 and -2 was seen in hepatocellular carcinoma (HCC) patients, and this loss was inversely related to patient survival. Additionally, loss of Stabilin-1 and -2 as well as CD32b was also observed around the tissues surrounding the tumor in HCC patients (Geraud et al., 2013). Conversely, another study has shown that Stabilin-1 retains its expression in the LSECs of the diseased liver and mediates transmigration of T cells, especially T regulatory cells across the endothelium in the inflamed liver. They also found increased expression of Stabilin-1 in the vessels and sinusoids lining the tumor in HCC (Shetty et al., 2011). A survey was conducted on young and old rats to delineate Stabilin expression and endocytic capacity in LSECs in response to aging. Their results found that there was an attenuation in the endocytic capacity of old rats, although the degradation capacity for both ages were similar. An increase in LSEC thickness was also observed in old age rats, which might be responsible for lower endocytic capacity (Simon-Santamaria et al., 2010). Similarly, another study had documented a decrease in Stabilin-2 receptors expression on primary LSECs in an aged rat model (Maeso-Diaz et al., 2018). From these studies, we may generally conclude that aging results in reduced expression of the Stabilin receptors and disease may alter expression and function of these receptors.
LSECs are involved in the synthesis of Factor VIII, a blood coagulation factor. This pro-coagulant is either defective or missing in Hemophilia A patients (Powell, 2009). Do et al. (1999) first identified FVIII mRNA in purified LSECs and hepatocytes from mice and in cultured LSECs by RT-PCR. They also found higher FVIII mRNA expression in LSECs quantitatively. In agreement with this finding, the transplantation of LSECs isolated from FVB/N-Tie2–GFP mice in a hemophilia A mouse model restored plasma level of FVIII (Follenzi et al., 2008). An increase in plasma FVIII correlated with the proliferation of transplanted LSEC in a Hemophilia A mice model. Two months after the LSEC transplantation, a bleeding experiment was performed to evaluate coagulation. The results showed that bleeding stopped in 15–20 min after a tail-cut which corrected this disorder. To take this one step further, an interesting attempt was made to transplant human fetal LSECs in uPA-NOG (or immunodeficient) mice and evaluate human FVIII plasma levels in mice (Fomin et al., 2013). Factor VIII levels were about half as high as a normal human plasma sample, but the amount far surpassed the non-transplanted controls indicating that LSECs produce FVIII in appreciable quantities. These findings suggest a novel role of LSECs in the treatment of hemophilia A patients suffering from FVIII deficiency. Interestingly, Stabilin-2 which is highly expressed in LSECs and in the sinusoids of spleen, also regulates the clearance rates of FVIII. Based on the data derived from genome wide association studies (GWAS), both Stabilin-2 and CLEC4M (see below) have been identified to affect vWF-FVIII levels in plasma. vWF or von Willebrand factor is normally found in tight physical association with FVIII in plasma (Lollar, 1991). Using a combination of KO mice and immunofluorescence techniques, Swystun and co-workers firmly established that Stabilin-2 and CLEC4M expressed in LSECs of mice, regulates turnover of FVIII (Swystun et al., 2018, 2019).
Scavenger Receptor L (SR-L)
LRP-1 (Low-density lipoprotein receptor-related protein or CD91 or α2macroglobulin receptor) is a scavenger and endocytic receptor present on the cell surface belonging to the family of low-density lipoprotein receptor (Herz et al., 1988). The structure of LRP-1 is composed of five domains (i) the ligand-binding domain (ii) the O-linked sugar domain (iii) the EGF-precursor homology domain (iv) the transmembrane domain and the intracellular domain. Proteolytic cleavage of LRP-1 results in alpha subunit ligand-binding domain at the N-terminus and ß subunit consisting of other remaining domain at the C-terminus (Lillis et al., 2008). LRP-1 binds to more than 30 ligands, such as ApoE (Hussain et al., 1999), tPA (Salama et al., 2019), a receptor-associated protein (RAP) (Prasad et al., 2016), in addition to trypsin-activated α2-macroglobulin (α2M∗), lactoferrin, Factor VIII and others (for a complete list of ligands, see Herz and Strickland, 2001). The presence of different motifs at the cytoplasmic tail of LRP-1, such as two dileucine motifs, NPXY motifs, one YXXL motif has been suggested to be responsible for its high endocytic uptake (Li et al., 2000; Deane et al., 2008). LRP-1 is expressed in the liver, lung, brain, intestine, and muscles (Herz et al., 1988).
Oie et al. (2011) reported the expression of LRP-1 on LSECs with immunofluorescence. They showed that LRP-1 expressed in LSECs is responsible for partial hepatic clearance of RAP, an inhibitor of all known ligand interactions with LRP-1 and α2M∗, along with other hepatic cells, suggesting the role of LSECs in lipid homeostasis. A hepatocyte-specific LRP-1 KO study showed that NAFLD disease progression is accelerated by the deficiency of LRP-1 in mice fed with a high-fat high cholesterol diet and that LSEC and KC LRP-1 were not sufficient to rescue the phenotype (Hamlin et al., 2018). Similarly, another hepatocyte-specific LRP-1 KO study has demonstrated an increase in the accumulation of lipid in primary culture of LRP-1 KO hepatocytes due to an impairment in the lysosomal degradation capacity of auto-phagolysosomes leading to cell death (Hamlin et al., 2016). These studies suggests severe impact of LRP-1 dysregulation on NAFLD progression. However, not much is known about the LSEC specific function of LRP-1 in various diseases and this needs to be elucidated.
Other Cell Surface Receptors
FcγRIIb/CD32b
Fc gamma receptors have four major classes in mice (FcγRI-IV) and six in humans (Daeron, 1997). Fc gamma receptors IIb (FcγRIIb) binds to the monomeric immunoglobulin G (IgG) Fc domain with low affinity and inhibit the response produced by activating FcγRs. It is located on chromosome 1 in both humans and mice (Fagerberg et al., 2014; Yue et al., 2014). FcγRIIb consists of a cytoplasmic region characterized by a 13 amino acid long YSLL sequence, also known as Immunoreceptor Tyrosine based Inhibitory motif (ITIM) and an extracellular domain (Van den Herik-Oudijk et al., 1995). Alternative splicing of mRNA sequence results in two isoform namely FcγRIIb1 and FcγRIIb2. ITIM is essential for its inhibitory function (Van den Herik-Oudijk et al., 1995). When immunoglobulins on the cell surface bind and crosslink these receptors, tyrosine 309 is phosphorylated. Tatsushi et al. demonstrated that mutation of Tyr 309 to phenylalanine in the ITIM motif abolished its inhibitory effect of B cell activation (Amigorena et al., 1992; Muta et al., 1994). Daeron et al. (1993) demonstrated that a single mutation of Tyr26 to glycine at the intra-cytoplasmic domain and YSLL motif resulted in impairment of endocytosis and phagocytosis processes, suggesting that phosphorylation or dephosphorylation of tyrosine residues is important for normal function of the receptor. Similarly, another study reported that single amino acid substitution of tyrosine to alanine using alanine scanning mutagenesis methods resulted in the abolition of endocytosis (Hunziker and Fumey, 1994). This investigation discovered that the endocytosis signaling and di-leucine-based signaling overlap so that you could not have one without the other in terms of function. The expression of FcγRIIb is found on the sinusoids of spleen and liver as well as in immune cells, such as B cells, dendritic cells, myeloid cells as well as leukemia and lymphoma cells (Lim et al., 2011; Tutt et al., 2015).
FcγRIIb has been implicated in the regulation of immunoreactivity. One study has reported a decrease in expression of FcγRIIb on B cells in active systemic lupus erythematosus (SLE) patients as compared to healthy control, suggesting its protective role in SLE (Ochi and Kawabi, 1992). Similarly, an increase in FcγRIIb expression on B cells upon delivery of retroviral transduced FcγRIIb bone in spontaneous lupus-prone mice compared to the mice that received parent retrovirus transduced bone marrow (McGaha et al., 2005). They also found a decrease in the immune complex accumulation in the kidney as compared to control. Besides its expression on hematopoietic cells, FcγRIIb expression is also found exclusively on LSECs, and it is used as a marker to distinguish LSECs from other liver cell types (Mousavi et al., 2007). In hepatic sinusoids, FcγRIIb is responsible for removing small immune complexes (SIC). Ganesan et al. (2012) studied the blood clearance rate of SIC using radio iodinated SIC in WT and FcγRIIb KO mice model. They found an inhibition in the clearance rate of SIC in FcγRIIb KO mice as compared to control, suggesting the involvement of LSECs FcγRIIb in immune complex mediated diseases (Ganesan et al., 2012).
A study conducted on NAFL and NASH biopsy specimens to assess the expression of FcγRIIb on LSECs reported a medium negative correlation between serum collagen type IV and hyaluronan with FcγRIIb expression (Ishikawa et al., 2019). An increase in type IV collagen and hyaluronan contents have already been shown with NASH progression, suggesting a reduction in scavenger function of FcγRIIb. They also witnessed an inversely proportional relation between FcγRIIb expression and fibrosis stages, reporting the highest expression at the initial stage of fibrosis and lowest at fibrosis stage 3. However, taking all of the data together across the various grades of fibrosis and NAFLD activity scores, Ishikawa and coworkers did not find a significant difference in expression level of FcγRIIb. A new insight in the hepatic fibrosis study was provided by Vilar-Gomez et al. (2017) who reported platelet counts as a novel indirect biomarker for portal hypertension and advanced hepatic liver disease assessment. They reported the inhibitory role of platelets in fibrosis. In agreement with this study, Ishikawa et al. (2019) have shown a positive relationship between platelet count and FcγRIIb expression on LSECs through regression analysis, suggesting a low platelet count in high fibrosis stage, thereby, decreased FcγRIIb expression. For HCC, a decrease in FcγRIIb expression is co-commitment with an increase in cancer grade similar to Stabilin-2 (Geraud et al., 2013). A study conducted on peritumoral tissue samples of HCC patients showed that the expression of FcγRIIb was decreased in 63% of samples taken into consideration for the study. A microarray analysis of these tissues found that loss of FcγRIIb is related to significantly longer tumor-specific survival. However, in terms of the 5-yr survival rate, there was more of an impact or survival rate for Stabilin-2 (42%) than FcγRIIb (16%).
Another ligand of FcγRIIb, fibrinogen-like protein 2 (FGL2), was found to be increased in NAFLD patients demonstrating severe forms of NAFLD, suggesting a decrease in FcγRIIb expression (Colak et al., 2011). This correlation did not hold across the fibrosis stages or grades of steatosis. Furthermore, Maeso-Diaz et al. (2018) have shown an even or slight increase in FcγRIIb expression in the comparison of young and old LSECs, however, there is a very stark reduction in Stabilin-2, eNOS, BMP-2, Lamb1, and HGF in aged LSECs suggesting that the LSECs become vulnerable to acute or chronic injury in old age.
Toll-Like Receptors
Besides scavenger receptors, LSECs express multifarious pattern recognition receptors mostly consisting of toll-like receptors (TLRs). TLRs are capable of recognizing pathogen-associated molecular patterns (PAMP) present on invading microbes or damage-associated molecular pattern (DAMP) originating from endogenous damaged or apoptotic cells (Kawasaki and Kawai, 2014). They are type I transmembrane glycoprotein consisting of an extracellular N-terminal ligand-binding domain, single transmembrane domain, and a C-terminal cytoplasmic domain. Ligand binding is mediated through an ectodomain characterized by leucine-rich repeats (Bell et al., 2003). Downstream signaling is mediated by adaptor proteins associated with the Toll/IL-1 receptor (TIR) present at the cytoplasmic C-terminal domain (O’Neill and Bowie, 2007). TLRs form an important bridge between innate and adaptive immune response system (Werling and Jungi, 2003; Pasare and Medzhitov, 2004). TLRs also induce the production of pro-inflammatory and effector cytokines and aid in the activation of T-cells by upregulating co-stimulatory molecules present on antigen-presenting cells (Vasselon and Detmers, 2002). Humans express 10 TLRs (TLR 1–10), whereas, mice express 12 TLRs (Hopkins and Sriskandan, 2005). Of these, LSECs express seven Toll-like receptors; TLR1–4, 6, 8, 9.
Uhrig et al. (2005) demonstrated a constitutive expression of TLR4 on cultured LSECs isolated from mice. TLR4 binds to LPS present on gram-negative bacteria and initiates an immune response for its clearance (Poltorak et al., 1998). In this study, cultured LSECs developed tolerance upon repetitive exposure with LPS, though this effect was not mediated by downregulation in the expression of TLR4. Reduction in the activation of NF-kB was responsible for developing tolerance against LPS stimulation in LSECs. Not too long thereafter, Martin-Armas et al. (2006) showed that CpGs are taken up by TLR9 present in murine LSECs. The bacterial DNA is characterized by unmethylated CpG motifs that act as a potent immune stimulator by inducing the production of cytokines from various immune cells, such as dendritic cells, macrophages, B cells and NK cells (Ashkar and Rosenthal, 2002; Dalpke et al., 2006). These studies reported the presence of TLR9 in murine LSECs for the first-time using RT-PCR and immunolabeling. LSECs accumulate the most FITC-labeled CpG than other liver cell types shown by both in vitro and in vivo experiments. They also reported the binding of CpG to TLR9 in the endo-lysosomal compartment which activated NF-κB signaling for the IL-1β and IL-6 production. This study suggests the role of LSECs in mediating innate immune response in the liver. A TLR4 KO mouse study demonstrated the role of TLR4 in NAFLD by enhancing the secretion of hepatic TGF-ß and collagen associated with fibrosis (Sutter et al., 2016). These results strongly suggest that the chronic inflammation associated with fatty liver disease is regulated, in part, by TLR4.
What is the functional role of TLRs in response to ligands in LSECs? To answer this question, responses to many TLR specific agonists were evaluated in murine LSECs (Wu et al., 2010). Upon treatment with TLR specific agonists, the high mRNA expression level of TLR1,4,6,8 and moderate mRNA expression level of TLR9 were observed in murine LSEC, however, TLR5, 6, and TLR9 showed very low mRNA expression. Contrary to mRNA expression, TLR5 and TLR6 did not show any expression at the protein level in flow cytometry. They also showed that the antiviral response is produced by TLR3 in LSECs by the secretion of IFN-β. Furthermore, TNF-α production was reported in LSECs treated with TLR4 agonists in high amounts and by TLR2,3 and TLR8 in a moderate amount. Similarly, a 4-fold and 16-fold upregulation was observed in LSECs treated with TLR4 and TLR3 agonists, respectively. LSECs were also able to upregulate MHC class II expression with TLR8 agonists as well as the proliferation of T-cells with TLR1,2 and TLR6 agonists. Additionally, LSECs were involved with the activation of CD4 and CD8 T-cells when treated with TLR1,2 and TLR6 agonists. These results suggest that LSECs are capable of initiating antiviral and pro-inflammatory responses by TLR3 as well as adaptive responses through TLR1,2,6 and TLR8, thereby maintaining hepatic immune response. Similarly, the role of TLR3 stimulated the murine LSECs is reported in suppressing Hepatitis B viral replication mediated by IFN-ß production in vitro (Wu et al., 2007).
The portal circulation continuously exposes the liver to microbial antigens and food from the gut; therefore, it possesses a piece of special machinery to maintain immune tolerance. Liu J. et al. (2013) reported that incubation of LSECs with palmitoyl-3-cysteine-serine-lysine-4 (P3C), a TLR2 ligand, resulted in the reversal of immune tolerance. LSECs were co-cultured with stimulated T cells isolated from mice and treated with P3C. This resulted in an increase in proliferation of T cells as well as cytokine production in co-culture as compared to T cells cultured alone. An increase in CD8+ effector T cell population, IL-12 production, and decrease in PD-L1 expression on LSECs was also observed in these co-culture systems. This study outlined the role of TLRs in regulating the immunosuppressive property in LSECs. Similarly, one study has demonstrated the role of TLR2 expressed on LSECs in initiating an innate immune response toward adeno-associated viral vectors (rAAV) and efficiency of gene therapy mediated by rAAV which may be enhanced by understanding this mechanism (Hosel et al., 2012). As the anatomical sieve of the liver, the LSECs are continually monitoring antigens and confer tolerance to maintain proper homeostasis.
L-SIGN and LSECtin
Liver/lymph node-specific ICAM-3 grabbing non-integrin (L-SIGN)/CD299L/CLEC4M and Liver and lymph node sinusoidal endothelial cell C-type lectin (LSECtin)/CLEC4G are type II transmembrane proteins belonging to the C-type lectin family (Bashirova et al., 2001; Pohlmann et al., 2001; Gardner et al., 2003; Liu et al., 2004). These two receptors are encoded on chromosome 19 in humans (Grimwood et al., 2004). They are characterized by an intracellular domain, a transmembrane domain, and an extracellular domain composed of a neck domain and a C-type carbohydrate recognition domain (CRD). Ligand binding is mediated through C-type CRD. L-SIGN is expressed by liver and lymph node sinusoidal endothelial and placental capillary endothelial cells (Pohlmann et al., 2001). Likewise, liver and lymph node sinusoidal endothelial cells specifically co-express LSECtin (Liu et al., 2004). LSECtin expression is also seen on bone marrow sinusoidal endothelial cells as well as on KCs in the liver (Dominguez-Soto et al., 2009). L-SIGN binds to high mannose oligosaccharide (Feinberg et al., 2001), whereas, LSECtin can bind with N-acetylglucosamine, mannose, and fucose (Liu et al., 2004). Since L-SIGN is expressed on placental capillary endothelium, one study has shown their possible involvement in mother to child transmission of HIV-1 virus (Boily-Larouche et al., 2012), while several other studies relating L-SIGN with HIV-1 entry remain contradictory. L-SIGN expressed on pulmonary endothelial cells serve as the gateway for the entrance of SARS-CoV as it was able to bind HEK293T cells expressing purified soluble SARS-CoV glycoproteins (Jeffers et al., 2004). Similarly, LSECtin might also play a role in mediating SARS-CoV infection in hepatocytes (Gramberg et al., 2005).
L-SIGN has been reported to interact with glycoprotein E2 of the Hepatitis C viron (HCV) (Gardner et al., 2003). This study has shown that L-SIGN transfected Hela cells were able to bind purified HCV-E2 protein as compared to parental HeLa cells using FACS analysis. Since, mannan is a ligand for L-SIGN, incubating the recombinant L-SIGN expressing HeLa cells (HeLa-L-SIGN) with mannan inhibited binding between L-SIGN and purified HCV-E2 protein. They confirmed the finding by exposing the HeLa-L-SIGN cells to HCV-virion and detected the HCV genome in L-SIGN transfected Hela cells by RT-PCR and Southern blotting. Similarly, another group has shown the involvement of L-SIGN in facilitating the entry of HCV and passing it to nearby hepatocytes present in the liver using HCV pseudotype particles (Lozach et al., 2004). Since LSECtin and L-SIGN belongs to the same C-type lectin family and share a 32% sequence identity, Li Y. et al. (2009) demonstrated that the central domain of LSECtin binds with L-SIGN and along with the C terminal CRD domain bind with E2 glycoprotein present on HCV suggesting that LSECtin binding with L-SIGN might play a role in the HCV binding to LSECs. LSECtin is also involved in mediating T-cell immune response in the hepatic system (Tang et al., 2009). This study showed that LSECtin binds to CD44, a hyaluronan binding receptor, present on activated T cells, halting T-cell activation and proliferation, thereby preventing liver injury. Both of these receptors bind to mannose residues, which help them in clearing pathogens from circulation (Liu et al., 2004). These studies suggest that L-SIGN and LSECtin may be targeted for the treatment of HCV and inflammatory liver diseases.
LYVE-1
Lymphatic vessel endothelial hyaluronan receptor 1 (LYVE-1) is a type I integral membrane glycoprotein which was firstly identified exclusively on lymph vessels responsible for sequestering hyaluronic acid in the lymph vessel endothelium (Banerji et al., 1999). Similar to its homolog CD44, LYVE-1 contains a single Link module in the extracellular domain that is responsible for Hyaluronic acid (HA) binding (Day and Prestwich, 2002). It is present on chromosome 11 in humans and chromosome 7 in mice. With the use of better antibodies, LYVE-1 expression was also detected in the liver, spleen, and lymph node sinusoidal endothelial cells in humans (Mouta Carreira et al., 2001; Akishima et al., 2004). However, a recent study conducted in rodents has revealed their expression in the non-sinusoidal endothelium of many other organs, such as lungs, heart, and adrenal gland (Zheng et al., 2016). Once regarded as the main receptor responsible for the internalization and transport of HA in the lymph circulation, a study using LYVE-1 KO mice model revealed that it is not crucial for the metabolism of HA in the lymphatic endothelium (Gale et al., 2007). Since HA is the only known ligand for LYVE-1, to date, and LSECs play a very important role in the degradation of HA, Mouta Carreira et al. (2001) hypothesized that LYVE-1 expression might be present on LSECs. They performed immunohistochemistry analysis and confirmed the expression of LYVE-1 on human and murine LSECs (Mouta Carreira et al., 2001). Since LSECs are the only LYVE-1 expressing cells in the liver, it can be used to distinguish LSEC from other liver cell types. Earlier studies had suggested a link between increased HA level and cirrhosis (Ichida et al., 1996). This study showed that LSECs from the cirrhotic and HCC liver had a lower capacity to degrade HA and serum levels of HA were increased. We now know that Stabilin-2/SR-H2/HARE previously described in this article is the determining factor for HA degradation in liver (Eriksson et al., 1983; Harris et al., 2007).
Similarly, data from murine as well as a human models of HCC reported a reduction in the LYVE-1 expression in liver tumors with immunohistochemical (IHC) analysis (Geraud et al., 2013). Additionally, a tissue microarray analysis of 191 HCC samples found complete loss of LYVE-1 expression in 83% of the cases as compared to control. There was also a positive correlation between LYVE-1 expression and histological grade of the individual tumor with a 47% loss in grade G1 (least) and 89% in grade G3 (most). Furthermore, Arimoto et al. (2010) conducted an IHC experiment on frozen normal and diseased liver tissue and found decreased expression of LYVE-1 and increased vWF expression in inflamed or fibrotic liver. A weak negative correlation was also observed between LYVE-1 expression and fibrosis stage. An ultrastructural analysis revealed loss in LSEC fenestration and appearance of the basement membrane-like structure in the diseased liver, suggesting the onset of sinusoidal capillarization. They found a similar reduction in LYVE-1 expression in chronic viral hepatitis and virus-related cirrhosis liver tissues. This study suggests the possible role of LYVE-1 in the progression of liver fibrosis. An increase in vWF expression with a similar decrease in LYVE-1 expression poses disturbances in microcirculation. Sinusoidal capillarization results in circulatory problems and disturbs the transport of various macromolecules between blood and hepatocytes in the diseased liver state. Given their role in hepatic disease progression, LYVE-1 and vWF may be used as a potential marker for sinusoidal capillarization.
Adhesion Molecules Expressed by LSECs
Adhesion molecules play an important role in mobilizing leukocytes at the site of inflammation. This process involves several steps and carried out by a different set of adhesion molecules, such as integrins, the selectins, and Ig superfamily members (Shetty et al., 2018). Each of the adhesion molecules are tightly regulated to maintain a homeostatic environment and their expression is modulated under certain diseased condition. Unlike capillary and microvascular endothelial cells, LSECs express few integrins. Integrins are heterodimers consisting of alpha and beta subunits mediating cell-extracellular matrix adhesion (Bokel and Brown, 2002). Couvelard et al. (1993) evaluated the expression of different cell-matrix adhesion proteins in LSECs and found that LSECs express only α1ß1 and α5ß1 under normal condition whereas, αVß3 and αIIbß3 in low and variable levels. α5ß1 acts as fibronectin receptor (Schaffner et al., 2013), whereas, α1ß1 binds to collagen (Eble et al., 1993). αVβ3 binds to vitronectin (Horton, 1997) as well as fibronectin (Van Agthoven et al., 2014) and αIIbß3 binds to fibrinogen (Wippler et al., 1994). Furthermore, they found a strong enhanced expression of α1ß1 and α5ß1 as well as αVß3 and αIIbß3 in cirrhotic liver that were faintly expressed in normal liver. In addition, other integrins (α6ß1, α6ß4, α2ß1, and α3ß1) that did not show any expression in normal liver, and showed an increased expression in cirrhotic liver, suggesting their contribution to capillarization. These integrins bind to laminin, an important component of the basal lamina of the extracellular matrix (Languino et al., 1989; Felch et al., 1992; Lee et al., 1992; Chang et al., 1995). Several studies have documented an increased deposition of collagen IV, fibronectin and laminin in LSEC basement membranes during liver fibrosis and inflammation (Walsh et al., 2000; Xu et al., 2003; Mak and Mei, 2017), suggesting a possible role of these integrins in inflammation and fibrosis.
Similar to microvascular endothelial cells, the LSEC Ig-superfamily of adhesion molecules is composed of ICAM-1 (Intercellular adhesion molecule-1), ICAM-2 (Intercellular adhesion molecule-2), VCAM-1 (Vascular cell-adhesion molecule) and PECAM-1 (Platelet endothelial cell adhesion molecule/CD31). VCAM-1 is an adhesion molecule that helps in mediating leukocyte-trans-endothelial migration. VCAM-1 expression is absent in normal liver, but is strongly enhanced under inflammatory conditions (Volpes et al., 1992). LSECs constitutively express ICAM-1 along with hepatocytes, KCs and HSCs (Gulubova, 2005; Yin et al., 2007). The expression of ICAM-1 in LSECs is found to be upregulated by several inflammatory cytokines, such as TNF-α, IL-1β, or IFN-γ (Gangopadhyay et al., 1998; Oudar et al., 1998). A study was conducted on various endothelial cells isolated from the liver after transplantation rejection (Steinhoff et al., 1993). In LSECs, weak expression of ICAM-1 and ICAM-2 was observed under normal conditions. However, an elevated expression of ICAM-1 and ICAM-2 in LSECs were observed in chronic rejection as well as sepsis or viral infection cases. VCAM-1 was also shown to be partially upregulated in LSECs of irreversible and chronic rejection conditions. This study reflected the clinical relevance of sinusoidal endothelial adhesion molecules during liver transplantation.
LFA-1 (Lymphocyte Function Associated-1) belongs to the integrin family and is present on lymphocytes and leukocytes. It is an important component in the extravasation process mediating leukocyte and lymphocyte entry into the tissues from the bloodstream (Mitroulis et al., 2015; Walling and Kim, 2018). Binding of ICAM-1 expressed on LSECs to its ligand, LFA-1, expressed in pro-inflammatory cells mediates the migration of cells across the sinusoidal lining (Wong et al., 1997). This was one of the first studies to determine that Selectins, expressed on continuous vascular endothelium, are not an essential component for leukocyte transmigration into inflamed tissue of the liver. Similarly, a co-culture study conducted with LSECs and C26 tumor cells showed that LSECs expressing ICAM-1 mediate tumor migration (Benedicto et al., 2019). They also found an increase in the inflammatory IL-1β, IL-6, TNF-α, and PGE2 in the co-culture as compare to LSECs cultured alone. Interrupting the interaction between ICAM-1 and LFA-1 expressed on LSECs and C26, respectively, reduced the secretion of observed inflammatory molecules. This suggests the role of ICAM-1 and LFA-1 interaction in providing an inflammatory microenvironment suitable for colonization of tumor cells in the liver.
Another Ig superfamily adhesion molecule is PECAM-1 (CD31) (Newman et al., 1990). It is an adhesion molecule located at the cellular side and is involved in mediating endothelial cell-cell adhesion. CD31 is also responsible for carrying out leukocyte and monocyte trans-endothelial migration (Albelda et al., 1991). Whether LSECs express CD31 or not has long been debated and remains cryptic (Couvelard et al., 1993; DeLeve et al., 2004; Elvevold et al., 2008b). An upregulation in CD31 expression has been shown during the capillarization of LSECs in cirrhotic human liver (Couvelard et al., 1993). Also, its enhanced expression is detected in LSECs during focal nodular hyperplasia (Scoazec et al., 1995). Likewise, enhanced expression of CD31 has been related to the non-fenestrated and de-differentiated state of LSECs (DeLeve et al., 2006). Neubauer et al. (2000b) for the first time showed the expression of CD31 on LSECs and found no observable difference in CD31 expression under normal conditions and carbon tetrachloride-induced (CCl4) liver fibrosis. However, another study by the same authors demonstrated a constitutive expression of CD31 on LSECs, whereas, a decrease of CD31 was observed after CCl4 administration mediated by TNF-α in vitro. They hypothesized that reduced CD31 expression might aid in more desirable mononuclear cell transmigration (Neubauer et al., 2000a). Clearly, the on-going debate for the expression of CD31 on LSECs needs to be resolved and it should never be used as a marker for rodent LSEC purification.
Perspectives and Conclusion
The past 35 years have proven to be a treasure-trove of discovery of the unique sinusoidal endothelium in liver. Advancements in their purification from rats and mice have enabled researchers to assess their physiological and biological role in normal and diseased phenotypes. Starting with the efforts of Seglen (1976) in the early 1970s and culminating to the current date, the procedure of dissociating the liver with collagenase-based enzyme mixtures have been very similar. The final steps in purification have involved differential centrifugation, fluorescent-activated cell sorting, and magnetic-activated cell sorting or a combination of these methods. A recent review on these techniques may be found in Meyer et al. (2016).
The anatomical shape and position of LSECs make them uniquely optimal for sequestering macromolecular materials from the blood. In this position, they act as “guardians” of the liver expressing numerous scavenger receptors and constantly monitoring the antigenic profile of the blood. As the liver is bathed in portal vein blood which drains the GI tract, there are many food and bacterial antigens flowing through in which LSECs play a major role in cleaning up and tempering other immune cells within the liver. Table 1 of this manuscript outlined many of the exogenous and endogenous ligands for all of these receptors. Redundancy in binding of multiple ligands shared by several receptors suggest the importance of physiological homeostasis with regards to external material coming into contact with blood and internal tissues. What is not known so much is the biochemistry of how these receptors are taking up multiple ligands at the same time or which amino acids/domains are interacting with each ligand. We think that this is an important step forward with the advancements in crystallography and cryo-EM methodologies. With an understanding of receptor-ligand interactions, pharmacological agents may be made to enhance or block these interactions according to required circumstances in the patient.
Author Contributions
EP wrote the manuscript. AN wrote part of the manuscript and created the figures. EH edited and organized the manuscript with some brief writing in various sections. All authors contributed to the article and approved the submitted version.
Funding
This manuscript was funded in part by the National Institutes of Health grant R01 HL130864.
Conflict of Interest
The authors declare that the research was conducted in the absence of any commercial or financial relationships that could be construed as a potential conflict of interest.
References
Abumrad, N. A., El-Maghrabi, M. R., Amri, E. Z., Lopez, E., and Grimaldi, P. A. (1993). Cloning of a rat adipocyte membrane protein implicated in binding or transport of long-chain fatty acids that is induced during preadipocyte differentiation. Homology with human CD36. J. Biol. Chem. 268, 17665–17668.
Acton, S., Rigotti, A., Landschulz, K. T., Xu, S., Hobbs, H. H., and Krieger, M. (1996). Identification of scavenger receptor SR-BI as a high density lipoprotein receptor. Science 271, 518–520. doi: 10.1126/science.271.5248.518
Acton, S. L., Scherer, P. E., Lodish, H. F., and Krieger, M. (1994). Expression cloning of SR-BI, a CD36-related class B scavenger receptor. J. Biol. Chem. 269, 21003–21009.
Akagi, M., Nishimura, S., Yoshida, K., Kakinuma, T., Sawamura, T., Munakata, H., et al. (2006). Cyclic tensile stretch load and oxidized low density lipoprotein synergistically induce lectin-like oxidized ldl receptor-1 in cultured bovine chondrocytes, resulting in decreased cell viability and proteoglycan synthesis. J. Orthop. Res. 24, 1782–1790. doi: 10.1002/jor.20211
Akishima, Y., Ito, K., Zhang, L., Ishikawa, Y., Orikasa, H., Kiguchi, H., et al. (2004). Immunohistochemical detection of human small lymphatic vessels under normal and pathological conditions using the LYVE-1 antibody. Virchows. Arch. 444, 153–157. doi: 10.1007/s00428-003-0950-8
Ala-Kokko, L., Pihlajaniemi, T., Myers, J. C., Kivirikko, K. I., and Savolainen, E. R. (1987). Gene expression of type I, III and IV collagens in hepatic fibrosis induced by dimethylnitrosamine in the rat. Biochem. J. 244, 75–79. doi: 10.1042/bj2440075
Albelda, S. M., Muller, W. A., Buck, C. A., and Newman, P. J. (1991). Molecular and cellular properties of PECAM-1 (endoCAM/CD31): a novel vascular cell-cell adhesion molecule. J. Cell Biol. 114, 1059–1068. doi: 10.1083/jcb.114.5.1059
Allavena, P., Chieppa, M., Monti, P., and Piemonti, L. (2004). From pattern recognition receptor to regulator of homeostasis: the double-faced macrophage mannose receptor. Crit. Rev. Immunol. 24, 179–192. doi: 10.1615/critrevimmunol.v24.i3.20
Amigorena, S., Bonnerot, C., Drake, J. R., Choquet, D., Hunziker, W., Guillet, J. G., et al. (1992). Cytoplasmic domain heterogeneity and functions of IgG Fc receptors in B lymphocytes. Science 256, 1808–1812. doi: 10.1126/science.1535455
Araki, N., Higashi, T., Mori, T., Shibayama, R., Kawabe, Y., Kodama, T., et al. (1995). Macrophage scavenger receptor mediates the endocytic uptake and degradation of advanced glycation end products of the Maillard reaction. Eur. J. Biochem. 230, 408–415. doi: 10.1111/j.1432-1033.1995.0408h.x
Arimoto, J., Ikura, Y., Suekane, T., Nakagawa, M., Kitabayashi, C., Iwasa, Y., et al. (2010). Expression of LYVE-1 in sinusoidal endothelium is reduced in chronically inflamed human livers. J. Gastroenterol. 45, 317–325. doi: 10.1007/s00535-009-0152-5
Arteta, B., Lasuen, N., Lopategi, A., Sveinbjornsson, B., Smedsrod, B., and Vidal-Vanaclocha, F. (2010). Colon carcinoma cell interaction with liver sinusoidal endothelium inhibits organ-specific antitumor immunity through interleukin-1-induced mannose receptor in mice. Hepatology 51, 2172–2182. doi: 10.1002/hep.23590
Ashkar, A. A., and Rosenthal, K. L. (2002). Toll-like receptor 9. CpG DNA and innate immunity. Curr. Mol. Med. 2, 545–556. doi: 10.2174/1566524023362159
Asumendi, A., Alvarez, A., Martinez, I., Smedsrod, B., and Vidal-Vanaclocha, F. (1996). Hepatic sinusoidal endothelium heterogeneity with respect to mannose receptor activity is interleukin-1 dependent. Hepatology 23, 1521–1529. doi: 10.1053/jhep.1996.v23.pm0008675173
Balzan, S., and Lubrano, V. (2018). LOX-1 receptor: a potential link in atherosclerosis and cancer. Life Sci. 198, 79–86. doi: 10.1016/j.lfs.2018.02.024
Banerji, S., Ni, J., Wang, S. X., Clasper, S., Su, J., Tammi, R., et al. (1999). LYVE-1, a new homologue of the CD44 glycoprotein, is a lymph-specific receptor for hyaluronan. J. Cell Biol. 144, 789–801. doi: 10.1083/jcb.144.4.789
Bashirova, A. A., Geijtenbeek, T. B., van Duijnhoven, G. C., van Vliet, S. J., Eilering, J. B., Martin, M. P., et al. (2001). A dendritic cell-specific intercellular adhesion molecule 3-grabbing nonintegrin (DC-SIGN)-related protein is highly expressed on human liver sinusoidal endothelial cells and promotes HIV-1 infection. J. Exp. Med. 193, 671–678. doi: 10.1084/jem.193.6.671
Bell, J. K., Mullen, G. E., Leifer, C. A., Mazzoni, A., Davies, D. R., and Segal, D. M. (2003). Leucine-rich repeats and pathogen recognition in Toll-like receptors. Trends Immunol. 24, 528–533. doi: 10.1016/s1471-4906(03)00242-4
Benedicto, A., Herrero, A., Romayor, I., Marquez, J., Smedsrod, B., Olaso, E., et al. (2019). Liver sinusoidal endothelial cell ICAM-1 mediated tumor/endothelial crosstalk drives the development of liver metastasis by initiating inflammatory and angiogenic responses. Sci. Rep. 9:13111. doi: 10.1038/s41598-019-49473-7
Blouin, A., Bolender, R. P., and Weibel, E. R. (1977). Distribution of organelles and membranes between hepatocytes and nonhepatocytes in the rat liver parenchyma. A stereological study. J. Cell Biol. 72, 441–455. doi: 10.1083/jcb.72.2.441
Boily-Larouche, G., Milev, M. P., Zijenah, L. S., Labbe, A. C., Zannou, D. M., Humphrey, J. H., et al. (2012). Naturally-occurring genetic variants in human DC-SIGN increase HIV-1 capture, cell-transfer and risk of mother-to-child transmission. PLoS One 7:e40706. doi: 10.1371/journal.pone.0040706
Bokel, C., and Brown, N. H. (2002). Integrins in development: moving on, responding to, and sticking to the extracellular matrix. Dev. Cell 3, 311–321. doi: 10.1016/s1534-5807(02)00265-4
Bowdish, D. M., and Gordon, S. (2009). Conserved domains of the class A scavenger receptors: evolution and function. Immunol. Rev. 227, 19–31. doi: 10.1111/j.1600-065X.2008.00728.x
Braet, F., De Zanger, R., Sasaoki, T., Baekeland, M., Janssens, P., Smedsrod, B., et al. (1994). Assessment of a method of isolation, purification, and cultivation of rat liver sinusoidal endothelial cells. Lab. Invest. 70, 944–952.
Braet, F., Riches, J., Geerts, W., Jahn, K. A., Wisse, E., and Frederik, P. (2009). Three-dimensional organization of fenestrae labyrinths in liver sinusoidal endothelial cells. Liver. Int. 29, 603–613. doi: 10.1111/j.1478-3231.2008.01836.x
Braet, F., and Wisse, E. (2002). Structural and functional aspects of liver sinusoidal endothelial cell fenestrae: a review. Comp. Hepatol. 1:1.
Braet, F., Wisse, E., Bomans, P., Frederik, P., Geerts, W., Koster, A., et al. (2007). Contribution of high-resolution correlative imaging techniques in the study of the liver sieve in three-dimensions. Microsc. Res. Tech. 70, 230–242. doi: 10.1002/jemt.20408
Braet, F., Wisse, E., and Probst, I. (2005). The long-term culture of pig liver sinusoidal endothelial cells: the Holy Grail found. Eur. J. Cell Biol. 84, 745–748. doi: 10.1016/j.ejcb.2005.06.005
Brown, M. S., and Goldstein, J. L. (1990). Atherosclerosis. Scavenging for receptors. Nature 343, 508–509. doi: 10.1038/343508a0
Brundert, M., Heeren, J., Merkel, M., Carambia, A., Herkel, J., Groitl, P., et al. (2011). Scavenger receptor CD36 mediates uptake of high density lipoproteins in mice and by cultured cells. J. Lipid. Res. 52, 745–758. doi: 10.1194/jlr.M011981
Burgdorf, S., Scholz, C., Kautz, A., Tampe, R., and Kurts, C. (2008). Spatial and mechanistic separation of cross-presentation and endogenous antigen presentation. Nat. Immunol. 9, 558–566. doi: 10.1038/ni.1601
Calvo, D., Gomez-Coronado, D., Suarez, Y., Lasuncion, M. A., and Vega, M. A. (1998). Human CD36 is a high affinity receptor for the native lipoproteins HDL. LDL, and VLDL. J. Lipid Res. 39, 777–788.
Calvo, D., and Vega, M. A. (1993). Identification, primary structure, and distribution of CLA-1, a novel member of the CD36/LIMPII gene family. J. Biol. Chem. 268, 18929–18935.
Cao, G., Garcia, C. K., Wyne, K. L., Schultz, R. A., Parker, K. L., and Hobbs, H. H. (1997). Structure and localization of the human gene encoding SR-BI/CLA-1. Evidence for transcriptional control by steroidogenic factor 1. J. Biol. Chem. 272, 33068–33076. doi: 10.1074/jbc.272.52.33068
Chang, A. C., Salomon, D. R., Wadsworth, S., Hong, M. J., Mojcik, C. F., Otto, S., et al. (1995). Alpha 3 beta 1 and alpha 6 beta 1 integrins mediate laminin/merosin binding and function as costimulatory molecules for human thymocyte proliferation. J. Immunol. 154, 500–510.
Chen, H., Li, D., Sawamura, T., Inoue, K., and Mehta, J. L. (2000). Upregulation of LOX-1 expression in aorta of hypercholesterolemic rabbits: modulation by losartan. Biochem. Biophys. Res. Commun. 276, 1100–1104. doi: 10.1006/bbrc.2000.3532
Chen, M., Kakutani, M., Minami, M., Kataoka, H., Kume, N., Narumiya, S., et al. (2000). Increased expression of lectin-like oxidized low density lipoprotein receptor-1 in initial atherosclerotic lesions of Watanabe heritable hyperlipidemic rabbits. Arterioscler. Thromb. Vasc. Biol. 20, 1107–1115. doi: 10.1161/01.atv.20.4.1107
Chen, M., Kakutani, M., Naruko, T., Ueda, M., Narumiya, S., Masaki, T., et al. (2001a). Activation-dependent surface expression of LOX-1 in human platelets. Biochem. Biophys. Res. Commun. 282, 153–158. doi: 10.1006/bbrc.2001.4516
Chen, M., Narumiya, S., Masaki, T., and Sawamura, T. (2001b). Conserved C-terminal residues within the lectin-like domain of LOX-1 are essential for oxidized low-density-lipoprotein binding. Biochem. J. 355(Pt 2), 289–296. doi: 10.1042/0264-60213550289
Christie, R. H., Freeman, M., and Hyman, B. T. (1996). Expression of the macrophage scavenger receptor, a multifunctional lipoprotein receptor, in microglia associated with senile plaques in Alzheimer’s disease. Am. J. Pathol. 148, 399–403.
Chui, P. C., Guan, H. P., Lehrke, M., and Lazar, M. A. (2005). PPARgamma regulates adipocyte cholesterol metabolism via oxidized LDL receptor 1. J. Clin. Invest. 115, 2244–2256. doi: 10.1172/JCI24130
Colak, Y., Senates, E., Ozturk, O., Yilmaz, Y., Coskunpinar, E., Kahraman, O. T., et al. (2011). Plasma fibrinogen-like protein 2 levels in patients with non-alcoholic fatty liver disease. Hepatogastroenterology 58, 2087–2090. doi: 10.5754/hge11248
Couvelard, A., Scoazec, J. Y., and Feldmann, G. (1993). Expression of cell-cell and cell-matrix adhesion proteins by sinusoidal endothelial cells in the normal and cirrhotic human liver. Am. J. Pathol. 143, 738–752.
Daeron, M. (1997). Fc receptor biology. Annu. Rev. Immunol. 15, 203–234. doi: 10.1146/annurev.immunol.15.1.203
Daeron, M., Malbec, O., Latour, S., Bonnerot, C., Segal, D. M., and Fridman, W. H. (1993). Distinct intracytoplasmic sequences are required for endocytosis and phagocytosis via murine Fc gamma RII in mast cells. Int. Immunol. 5, 1393–1401. doi: 10.1093/intimm/5.11.1393
Dalpke, A., Frank, J., Peter, M., and Heeg, K. (2006). Activation of toll-like receptor 9 by DNA from different bacterial species. Infect. Immun. 74, 940–946. doi: 10.1128/IAI.74.2.940-946.2006
Day, A. J., and Prestwich, G. D. (2002). Hyaluronan-binding proteins: tying up the giant. J. Biol. Chem. 277, 4585–4588. doi: 10.1074/jbc.R100036200
De Rijke, Y. B., Biessen, E. A., Vogelezang, C. J., and van Berkel, T. J. (1994). Binding characteristics of scavenger receptors on liver endothelial and Kupffer cells for modified low-density lipoproteins. Biochem. J. 304(Pt 1), 69–73. doi: 10.1042/bj3040069
De Rijke, Y. B., Jürgens, G., Hessels, E. M., Hermann, A., and van Berkel, T. J. (1992). In vivo fate and scavenger receptor recognition of oxidized lipoprotein[a] isoforms in rats. J. Lipid Res. 33, 1315–1325.
De Rijke, Y. B., and Van Berkel, T. J. (1994). Rat liver Kupffer and endothelial cells express different binding proteins for modified low density lipoproteins. Kupffer cells express a 95-kDa membrane protein as a specific binding site for oxidized low density lipoproteins. J. Biol. Chem. 269, 824–827.
Deane, R., Sagare, A., Hamm, K., Parisi, M., Lane, S., Finn, M. B., et al. (2008). apoE isoform-specific disruption of amyloid beta peptide clearance from mouse brain. J. Clin. Invest. 118, 4002–4013. doi: 10.1172/JCI36663
DeLeve, L. D., Wang, X., Hu, L., McCuskey, M. K., and McCuskey, R. S. (2004). Rat liver sinusoidal endothelial cell phenotype is maintained by paracrine and autocrine regulation. Am. J. Physiol. Gastrointest. Liver. Physiol. 287, G757–G763. doi: 10.1152/ajpgi.00017.2004
DeLeve, L. D., Wang, X., McCuskey, M. K., and McCuskey, R. S. (2006). Rat liver endothelial cells isolated by anti-CD31 immunomagnetic separation lack fenestrae and sieve plates. Am. J. Physiol. Gastrointest. Liver Physiol. 291, G1187–G1189.
Do, H., Healey, J. F., Waller, E. K., and Lollar, P. (1999). Expression of factor VIII by murine liver sinusoidal endothelial cells. J. Biol. Chem. 274, 19587–19592. doi: 10.1074/jbc.274.28.19587
Dominguez-Soto, A., Aragoneses-Fenoll, L., Gomez-Aguado, F., Corcuera, M. T., Claria, J., Garcia-Monzon, C., et al. (2009). The pathogen receptor liver and lymph node sinusoidal endotelial cell C-type lectin is expressed in human Kupffer cells and regulated by PU.1. Hepatology 49, 287–296. doi: 10.1002/hep.22678
Dunne, D. W., Resnick, D., Greenberg, J., Krieger, M., and Joiner, K. A. (1994). The type I macrophage scavenger receptor binds to gram-positive bacteria and recognizes lipoteichoic acid. Proc. Natl. Acad. Sci. U.S.A. 91, 1863–1867. doi: 10.1073/pnas.91.5.1863
During, A., Dawson, H. D., and Harrison, E. H. (2005). Carotenoid transport is decreased and expression of the lipid transporters SR-BI, NPC1L1, and ABCA1 is downregulated in Caco-2 cells treated with ezetimibe. J. Nutr. 135, 2305–2312. doi: 10.1093/jn/135.10.2305
Duryee, M. J., Freeman, T. L., Willis, M. S., Hunter, C. D., Hamilton, B. C. III, Suzuki, H., et al. (2005). Scavenger receptors on sinusoidal liver endothelial cells are involved in the uptake of aldehyde-modified proteins. Mol. Pharmacol. 68, 1423–1430. doi: 10.1124/mol.105.016121
Duryee, M. J., Klassen, L. W., Freeman, T. L., Willis, M. S., Tuma, D. J., and Thiele, G. M. (2004). Lipopolysaccharide is a cofactor for malondialdehyde-acetaldehyde adduct-mediated cytokine/chemokine release by rat sinusoidal liver endothelial and Kupffer cells. Alcohol. Clin. Exp. Res. 28, 1931–1938. doi: 10.1097/01.alc.0000148115.90045.c5
Eble, J. A., Golbik, R., Mann, K., and Kuhn, K. (1993). The alpha 1 beta 1 integrin recognition site of the basement membrane collagen molecule [alpha 1(IV)]2 alpha 2(IV). EMBO J. 12, 4795–4802. doi: 10.1002/j.1460-2075.1993.tb06168.x
El Khoury, J., Hickman, S. E., Thomas, C. A., Cao, L., Silverstein, S. C., and Loike, J. D. (1996). Scavenger receptor-mediated adhesion of microglia to beta-amyloid fibrils. Nature 382, 716–719. doi: 10.1038/382716a0
Elvevold, K., Nedredal, G. I., Revhaug, A., Bertheussen, K., and Smedsrod, B. (2005). Long-term preservation of high endocytic activity in primary cultures of pig liver sinusoidal endothelial cells. Eur. J. Cell Biol. 84, 749–764. doi: 10.1016/j.ejcb.2005.05.003
Elvevold, K., Simon-Santamaria, J., Hasvold, H., McCourt, P., Smedsrod, B., and Sorensen, K. K. (2008a). Liver sinusoidal endothelial cells depend on mannose receptor-mediated recruitment of lysosomal enzymes for normal degradation capacity. Hepatology 48, 2007–2015. doi: 10.1002/hep.22527
Elvevold, K., Smedsrod, B., and Martinez, I. (2008b). The liver sinusoidal endothelial cell: a cell type of controversial and confusing identity. Am. J. Physiol. Gastrointest. Liver Physiol. 294, G391–G400. doi: 10.1152/ajpgi.00167.2007
Emi, M., Asaoka, H., Matsumoto, A., Itakura, H., Kurihara, Y., Wada, Y., et al. (1993). Structure, organization, and chromosomal mapping of the human macrophage scavenger receptor gene. J. Biol. Chem. 268, 2120–2125.
Eng, F., and Youson, J. H. (1992). Morphology of the liver of the brook lamprey, Lampetra lamottenii before and during infection with the nematode, Truttaedacnitis stelmioides, hepatocytes, sinusoids, and perisinusoidal cells. Tissue Cell 24, 575–592. doi: 10.1016/0040-8166(92)90073-g
Eriksson, S., Fraser, J. R., Laurent, T. C., Pertoft, H., and Smedsrod, B. (1983). Endothelial cells are a site of uptake and degradation of hyaluronic acid in the liver. Exp. Cell Res. 144, 223–228. doi: 10.1016/0014-4827(83)90458-5
Ezekowitz, R. A., Sastry, K., Bailly, P., and Warner, A. (1990). Molecular characterization of the human macrophage mannose receptor: demonstration of multiple carbohydrate recognition-like domains and phagocytosis of yeasts in Cos-1 cells. J. Exp. Med. 172, 1785–1794. doi: 10.1084/jem.172.6.1785
Fagerberg, L., Hallstrom, B. M., Oksvold, P., Kampf, C., Djureinovic, D., Odeberg, J., et al. (2014). Analysis of the human tissue-specific expression by genome-wide integration of transcriptomics and antibody-based proteomics. Mol. Cell Proteomics 13, 397–406. doi: 10.1074/mcp.M113.035600
Falkowska-Hansen, B., Falkowski, M., Metharom, P., Krunic, D., and Goerdt, S. (2007). Clathrin-coated vesicles form a unique net-like structure in liver sinusoidal endothelial cells by assembling along undisrupted microtubules. Exp. Cell Res. 313, 1745–1757. doi: 10.1016/j.yexcr.2007.02.026
Falkowski, M., Schledzewski, K., Hansen, B., and Goerdt, S. (2003). Expression of stabilin-2, a novel fasciclin-like hyaluronan receptor protein, in murine sinusoidal endothelia, avascular tissues, and at solid/liquid interfaces. Histochem. Cell Biol. 120, 361–369. doi: 10.1007/s00418-003-0585-5
Febbraio, M., Hajjar, D. P., and Silverstein, R. L. (2001). CD36: a class B scavenger receptor involved in angiogenesis, atherosclerosis, inflammation, and lipid metabolism. J. Clin. Invest. 108, 785–791. doi: 10.1172/JCI14006
Febbraio, M., and Silverstein, R. L. (2007). CD36: implications in cardiovascular disease. Int. J. Biochem. Cell Biol. 39, 2012–2030. doi: 10.1016/j.biocel.2007.03.012
Feinberg, H., Mitchell, D. A., Drickamer, K., and Weis, W. I. (2001). Structural basis for selective recognition of oligosaccharides by DC-SIGN and DC-SIGNR. Science 294, 2163–2166. doi: 10.1126/science.1066371
Felch, M. E., Willis, R. A., Penney, D. P., Keng, P. C., and Phipps, R. P. (1992). Expression of alpha 6 beta 1 integrin, the laminin receptor, on subsets of normal murine lung fibroblasts and its upregulation by the inflammatory cytokines IFN-gamma and TNF-alpha. Reg. Immunol. 4, 363–370.
Fiete, D. J., Beranek, M. C., and Baenziger, J. U. (1998). A cysteine-rich domain of the “mannose” receptor mediates GalNAc-4-SO4 binding. Proc. Natl. Acad. Sci. U.S.A. 95, 2089–2093. doi: 10.1073/pnas.95.5.2089
Follenzi, A., Benten, D., Novikoff, P., Faulkner, L., Raut, S., and Gupta, S. (2008). Transplanted endothelial cells repopulate the liver endothelium and correct the phenotype of hemophilia A mice. J. Clin. Invest. 118, 935–945. doi: 10.1172/JCI32748
Fomin, M. E., Zhou, Y., Beyer, A. I., Publicover, J., Baron, J. L., and Muench, M. O. (2013). Production of factor VIII by human liver sinusoidal endothelial cells transplanted in immunodeficient uPA mice. PLoS One 8:e77255. doi: 10.1371/journal.pone.0077255
Fraser, J. R., Laurent, T. C., Pertoft, H., and Baxter, E. (1981). Plasma clearance, tissue distribution and metabolism of hyaluronic acid injected intravenously in the rabbit. Biochem. J. 200, 415–424. doi: 10.1042/bj2000415
Fraser, R., Cogger, V. C., Dobbs, B., Jamieson, H., Warren, A., Hilmer, S. N., et al. (2012). The liver sieve and atherosclerosis. Pathology 44, 181–186. doi: 10.1097/PAT.0b013e328351bcc8
Fraser, R., Dobbs, B. R., and Rogers, G. W. (1995). Lipoproteins and the liver sieve: the role of the fenestrated sinusoidal endothelium in lipoprotein metabolism, atherosclerosis, and cirrhosis. Hepatology 21, 863–874. doi: 10.1002/hep.1840210337
Fraser, R., Heslop, V. R., Murray, F. E., and Day, W. A. (1986). Ultrastructural studies of the portal transport of fat in chickens. Br. J. Exp. Pathol. 67, 783–791.
Furrer, K., Rickenbacher, A., Tian, Y., Jochum, W., Bittermann, A. G., Kach, A., et al. (2011). Serotonin reverts age-related capillarization and failure of regeneration in the liver through a VEGF-dependent pathway. Proc. Natl. Acad. Sci. U.S.A. 108, 2945–2950. doi: 10.1073/pnas.1012531108
Gale, N. W., Prevo, R., Espinosa, J., Ferguson, D. J., Dominguez, M. G., Yancopoulos, G. D., et al. (2007). Normal lymphatic development and function in mice deficient for the lymphatic hyaluronan receptor LYVE-1. Mol. Cell Biol. 27, 595–604. doi: 10.1128/MCB.01503-06
Ganesan, L. P., Kim, J., Wu, Y., Mohanty, S., Phillips, G. S., Birmingham, D. J., et al. (2012). FcgammaRIIb on liver sinusoidal endothelium clears small immune complexes. J. Immunol. 189, 4981–4988. doi: 10.4049/jimmunol.1202017
Ganesan, L. P., Mates, J. M., Cheplowitz, A. M., Avila, C. L., Zimmerer, J. M., Yao, Z., et al. (2016). Scavenger receptor B1, the HDL receptor, is expressed abundantly in liver sinusoidal endothelial cells. Sci. Rep. 6:20646. doi: 10.1038/srep20646
Gangopadhyay, A., Lazure, D. A., and Thomas, P. (1998). Adhesion of colorectal carcinoma cells to the endothelium is mediated by cytokines from CEA stimulated Kupffer cells. Clin. Exp. Metastasis 16, 703–712. doi: 10.1023/a:1006576627429
Gardner, J. P., Durso, R. J., Arrigale, R. R., Donovan, G. P., Maddon, P. J., Dragic, T., et al. (2003). L-SIGN (CD 209L) is a liver-specific capture receptor for hepatitis C virus. Proc. Natl. Acad. Sci. U.S.A. 100, 4498–4503. doi: 10.1073/pnas.0831128100
Gatmaitan, Z., Varticovski, L., Ling, L., Mikkelsen, R., Steffan, A. M., and Arias, I. M. (1996). Studies on fenestral contraction in rat liver endothelial cells in culture. Am. J. Pathol. 148, 2027–2041.
Geraud, C., Mogler, C., Runge, A., Evdokimov, K., Lu, S., Schledzewski, K., et al. (2013). Endothelial transdifferentiation in hepatocellular carcinoma: loss of Stabilin-2 expression in peri-tumourous liver correlates with increased survival. Liver Int. 33, 1428–1440. doi: 10.1111/liv.12262
Godoy, B., Murgas, P., Tichauer, J., and Von Bernhardi, R. (2012). Scavenger receptor class A ligands induce secretion of IL1beta and exert a modulatory effect on the inflammatory activation of astrocytes in culture. J. Neuroimmunol. 251, 6–13. doi: 10.1016/j.jneuroim.2012.06.004
Goerdt, S., Walsh, L. J., Murphy, G. F., and Pober, J. S. (1991). Identification of a novel high molecular weight protein preferentially expressed by sinusoidal endothelial cells in normal human tissues. J. Cell Biol. 113, 1425–1437. doi: 10.1083/jcb.113.6.1425
Gordon, S. (2002). Pattern recognition receptors: doubling up for the innate immune response. Cell 111, 927–930. doi: 10.1016/s0092-8674(02)01201-1
Gough, P. J., Greaves, D. R., Suzuki, H., Hakkinen, T., Hiltunen, M. O., Turunen, M., et al. (1999). Analysis of macrophage scavenger receptor (SR-A) expression in human aortic atherosclerotic lesions. Arterioscler. Thromb. Vasc. Biol. 19, 461–471. doi: 10.1161/01.atv.19.3.461
Gramberg, T., Hofmann, H., Moller, P., Lalor, P. F., Marzi, A., Geier, M., et al. (2005). LSECtin interacts with filovirus glycoproteins and the spike protein of SARS coronavirus. Virology 340, 224–236. doi: 10.1016/j.virol.2005.06.026
Greenwalt, D. E., Scheck, S. H., and Rhinehart-Jones, T. (1995). Heart CD36 expression is increased in murine models of diabetes and in mice fed a high fat diet. J. Clin. Invest. 96, 1382–1388. doi: 10.1172/JCI118173
Grimwood, J., Gordon, L. A., Olsen, A., Terry, A., Schmutz, J., Lamerdin, J., et al. (2004). The DNA sequence and biology of human chromosome 19. Nature 428, 529–535. doi: 10.1038/nature02399
Gulubova, M. V. (2005). Expression of cell adhesion molecules and their beta1 and beta2 integrin ligands in human liver peliosis. Pathol. Res. Pract. 201, 503–511. doi: 10.1016/j.prp.2005.05.006
Hamlin, A. N., Basford, J. E., Jaeschke, A., and Hui, D. Y. (2016). LRP1 Protein deficiency exacerbates palmitate-induced steatosis and toxicity in hepatocytes. J. Biol. Chem. 291, 16610–16619. doi: 10.1074/jbc.M116.717744
Hamlin, A. N., Chinnarasu, S., Ding, Y., Xian, X., Herz, J., Jaeschke, A., et al. (2018). Low-density lipoprotein receptor-related protein-1 dysfunction synergizes with dietary cholesterol to accelerate steatohepatitis progression. J. Biol. Chem. 293, 9674–9684. doi: 10.1074/jbc.RA118.001952
Hampton, R. Y., Golenbock, D. T., Penman, M., Krieger, M., and Raetz, C. R. (1991). Recognition and plasma clearance of endotoxin by scavenger receptors. Nature 352, 342–344. doi: 10.1038/352342a0
Han, N. I., Chung, K. W., Ahn, B. M., Choi, S. W., Lee, Y. S., Lee, C. D., et al. (2001). Ultrastructural changes of hepatic stellate cells in the space of Disse in alcoholic fatty liver. Korean J. Intern. Med. 16, 160–166. doi: 10.3904/kjim.2001.16.3.160
Hansen, B., Arteta, B., and Smedsrod, B. (2002). The physiological scavenger receptor function of hepatic sinusoidal endothelial and Kupffer cells is independent of scavenger receptor class A type I and II. Mol. Cell Biochem. 240, 1–8. doi: 10.1023/a:1020660303855
Harris, E. N., and Cabral, F. (2019). Ligand Binding and Signaling of HARE/Stabilin-2. Biomolecules 9:273. doi: 10.3390/biom9070273
Harris, E. N., Kyosseva, S. V., Weigel, J. A., and Weigel, P. H. (2007). Expression, processing, and glycosaminoglycan binding activity of the recombinant human 315-kDa hyaluronic acid receptor for endocytosis (HARE). J. Biol. Chem. 282, 2785–2797. doi: 10.1074/jbc.M607787200
Harris, E. N., Weigel, J. A., and Weigel, P. H. (2004). Endocytic function, glycosaminoglycan specificity, and antibody sensitivity of the recombinant human 190-kDa hyaluronan receptor for endocytosis (HARE). J. Biol. Chem. 279, 36201–36209. doi: 10.1074/jbc.m405322200
Harris, E. N., Weigel, J. A., and Weigel, P. H. (2008). The human hyaluronan receptor for endocytosis (HARE/Stabilin-2) is a systemic clearance receptor for heparin. J. Biol. Chem. 283, 17341–17350. doi: 10.1074/jbc.m710360200
Harris, E. N., and Weigel, P. H. (2008). The ligand-binding profile of HARE: hyaluronan and chondroitin sulfates A, C, and D bind to overlapping sites distinct from the sites for heparin, acetylated low-density lipoprotein, dermatan sulfate, and CS-E. Glycobiology 18, 638–648. doi: 10.1093/glycob/cwn045
Herz, J., Hamann, U., Rogne, S., Myklebost, O., Gausepohl, H., and Stanley, K. K. (1988). Surface location and high affinity for calcium of a 500-kd liver membrane protein closely related to the LDL-receptor suggest a physiological role as lipoprotein receptor. EMBO J. 7, 4119–4127. doi: 10.1002/j.1460-2075.1988.tb03306.x
Herz, J., and Strickland, D. K. (2001). LRP: a multifunctional scavenger and signaling receptor. J. Clin. Invest. 108, 779–784. doi: 10.1172/JCI13992
Hiltunen, T. P., Gough, P. J., Greaves, D. R., Gordon, S., and Yla-Herttuala, S. (2001). Rabbit atherosclerotic lesions express scavenger receptor AIII mRNA, a naturally occurring splice variant that encodes a non-functional, dominant negative form of the macrophage scavenger receptor. Atherosclerosis 154, 415–419. doi: 10.1016/s0021-9150(00)00519-0
Hopkins, P. A., and Sriskandan, S. (2005). Mammalian Toll-like receptors: to immunity and beyond. Clin. Exp. Immunol. 140, 395–407. doi: 10.1111/j.1365-2249.2005.02801.x
Horton, M. A. (1997). The alpha v beta 3 integrin “vitronectin receptor”. Int. J. Biochem. Cell Biol. 29, 721–725. doi: 10.1016/s1357-2725(96)00155-0
Hosel, M., Broxtermann, M., Janicki, H., Esser, K., Arzberger, S., Hartmann, P., et al. (2012). Toll-like receptor 2-mediated innate immune response in human nonparenchymal liver cells toward adeno-associated viral vectors. Hepatology 55, 287–297. doi: 10.1002/hep.24625
Hughes, D. A., Fraser, I. P., and Gordon, S. (1995). Murine macrophage scavenger receptor: in vivo expression and function as receptor for macrophage adhesion in lymphoid and non-lymphoid organs. Eur. J. Immunol. 25, 466–473. doi: 10.1002/eji.1830250224
Hunziker, W., and Fumey, C. (1994). A di-leucine motif mediates endocytosis and basolateral sorting of macrophage IgG Fc receptors in MDCK cells. EMBO J. 13, 2963–2969. doi: 10.1002/j.1460-2075.1994.tb06594.x
Hussain, M. M., Strickland, D. K., and Bakillah, A. (1999). The mammalian low-density lipoprotein receptor family. Annu. Rev. Nutr. 19, 141–172. doi: 10.1146/annurev.nutr.19.1.141
Ichida, T., Sugitani, S., Satoh, T., Matsuda, Y., Sugiyama, M., Yonekura, K., et al. (1996). Localization of hyaluronan in human liver sinusoids: a histochemical study using hyaluronan-binding protein. Liver 16, 365–371. doi: 10.1111/j.1600-0676.1996.tb00763.x
Ishikawa, T., Yokoyama, H., Matsuura, T., and Fujiwara, Y. (2019). Fc gamma RIIb expression levels in human liver sinusoidal endothelial cells during progression of non-alcoholic fatty liver disease. PLoS One 14:e0211543. doi: 10.1371/journal.pone.0211543
Jay, A. G., and Hamilton, J. A. (2018). The enigmatic membrane fatty acid transporter CD36: New insights into fatty acid binding and their effects on uptake of oxidized LDL. Prostagl. Leukot Essent Fatty Acids 138, 64–70. doi: 10.1016/j.plefa.2016.05.005
Jeffers, S. A., Tusell, S. M., Gillim-Ross, L., Hemmila, E. M., Achenbach, J. E., Babcock, G. J., et al. (2004). CD209L (L-SIGN) is a receptor for severe acute respiratory syndrome coronavirus. Proc. Natl. Acad. Sci. U.S.A. 101, 15748–15753. doi: 10.1073/pnas.0403812101
Juvet, L. K., Berg, T., and Gjoen, T. (1997). The expression of endosomal rab proteins correlates with endocytic rate in rat liver cells. Hepatology 25, 1204–1212. doi: 10.1002/hep.510250524
Kataoka, H., Kume, N., Miyamoto, S., Minami, M., Murase, T., Sawamura, T., et al. (2000). Biosynthesis and post-translational processing of lectin-like oxidized low density lipoprotein receptor-1 (LOX-1). N-linked glycosylation affects cell-surface expression and ligand binding. J. Biol. Chem. 275, 6573–6579. doi: 10.1074/jbc.275.9.6573
Kawai, Y., Smedsrod, B., Elvevold, K., and Wake, K. (1998). Uptake of lithium carmine by sinusoidal endothelial and Kupffer cells of the rat liver: new insights into the classical vital staining and the reticulo-endothelial system. Cell Tissue Res. 292, 395–410. doi: 10.1007/s004410051069
Kawasaki, T., and Kawai, T. (2014). Toll-like receptor signaling pathways. Front. Immunol. 5:461. doi: 10.3389/fimmu.2014.00461
Khaidakov, M., Wang, X., and Mehta, J. L. (2011). Potential involvement of LOX-1 in functional consequences of endothelial senescence. PLoS One 6:e20964. doi: 10.1371/journal.pone.0020964
Kharbanda, K. K., Todero, S. L., Shubert, K. A., Sorrell, M. F., and Tuma, D. J. (2001). Malondialdehyde-acetaldehyde-protein adducts increase secretion of chemokines by rat hepatic stellate cells. Alcohol 25, 123–128. doi: 10.1016/s0741-8329(01)00174-4
Kjeken, R., Mousavi, S. A., Brech, A., Gjoen, T., and Berg, T. (2001). Fluid phase endocytosis of [125I]iodixanol in rat liver parenchymal, endothelial and Kupffer cells. Cell Tissue Res. 304, 221–230. doi: 10.1007/s004410100348
Knook, D. L., and Sleyster, E. C. (1980). Isolated parenchymal, Kupffer and endothelial rat liver cells characterized by their lysosomal enzyme content. Biochem. Biophys. Res. Commun. 96, 250–257. doi: 10.1016/0006-291x(80)91207-3
Kodama, T., Freeman, M., Rohrer, L., Zabrecky, J., Matsudaira, P., and Krieger, M. (1990). Type I macrophage scavenger receptor contains alpha-helical and collagen-like coiled coils. Nature 343, 531–535. doi: 10.1038/343531a0
Kozarsky, K. F., Donahee, M. H., Rigotti, A., Iqbal, S. N., Edelman, E. R., and Krieger, M. (1997). Overexpression of the HDL receptor SR-BI alters plasma HDL and bile cholesterol levels. Nature 387, 414–417. doi: 10.1038/387414a0
Krieger, M. (1999). Charting the fate of the “good cholesterol”: identification and characterization of the high-density lipoprotein receptor SR-BI. Annu. Rev. Biochem. 68, 523–558. doi: 10.1146/annurev.biochem.68.1.523
Kume, N., Murase, T., Moriwaki, H., Aoyama, T., Sawamura, T., Masaki, T., et al. (1998). Inducible expression of lectin-like oxidized LDL receptor-1 in vascular endothelial cells. Circ. Res. 83, 322–327. doi: 10.1161/01.res.83.3.322
Kunjathoor, V. V., Febbraio, M., Podrez, E. A., Moore, K. J., Andersson, L., Koehn, S., et al. (2002). Scavenger receptors class A-I/II and CD36 are the principal receptors responsible for the uptake of modified low density lipoprotein leading to lipid loading in macrophages. J. Biol. Chem. 277, 49982–49988. doi: 10.1074/jbc.M209649200
Kus, E., Kaczara, P., Czyzynska-Cichon, I., Szafranska, K., Zapotoczny, B., Kij, A., et al. (2019). LSEC fenestrae are preserved despite pro-inflammatory phenotype of liver sinusoidal endothelial cells in mice on high fat diet. Front. Physiol. 10:6. doi: 10.3389/fphys.2019.00006
Kzhyshkowska, J., Gratchev, A., and Goerdt, S. (2006a). Stabilin-1, a homeostatic scavenger receptor with multiple functions. J. Cell Mol. Med. 10, 635–649. doi: 10.1111/j.1582-4934.2006.tb00425.x
Kzhyshkowska, J., Gratchev, A., Schmuttermaier, C., Brundiers, H., Krusell, L., Mamidi, S., et al. (2008). Alternatively activated macrophages regulate extracellular levels of the hormone placental lactogen via receptor-mediated uptake and transcytosis. J. Immunol. 180, 3028–3037. doi: 10.4049/jimmunol.180.5.3028
Kzhyshkowska, J., Workman, G., Cardo-Vila, M., Arap, W., Pasqualini, R., Gratchev, A., et al. (2006b). Novel function of alternatively activated macrophages: stabilin-1-mediated clearance of SPARC. J. Immunol. 176, 5825–5832. doi: 10.4049/jimmunol.176.10.5825
Languino, L. R., Gehlsen, K. R., Wayner, E., Carter, W. G., Engvall, E., and Ruoslahti, E. (1989). Endothelial cells use alpha 2 beta 1 integrin as a laminin receptor. J. Cell Biol. 109, 2455–2462. doi: 10.1083/jcb.109.5.2455
Le Couteur, D. G., Cogger, V. C., Markus, A. M., Harvey, P. J., Yin, Z. L., Ansselin, A. D., et al. (2001). Pseudocapillarization and associated energy limitation in the aged rat liver. Hepatology 33, 537–543. doi: 10.1053/jhep.2001.22754
Le Couteur, D. G., Cogger, V. C., McCuskey, R. S., Cabo, R. D. E., Smedsrod, B., Sorensen, K. K., et al. (2007). Age-related changes in the liver sinusoidal endothelium: a mechanism for dyslipidemia. Ann. N. Y. Acad. Sci. 1114, 79–87. doi: 10.1196/annals.1396.003
Lee, E. C., Lotz, M. M., Steele, G. D. Jr., and Mercurio, A. M. (1992). The integrin alpha 6 beta 4 is a laminin receptor. J. Cell Biol. 117, 671–678. doi: 10.1083/jcb.117.3.671
Li, D., and Mehta, J. L. (2000). Upregulation of endothelial receptor for oxidized LDL (LOX-1) by oxidized LDL and implications in apoptosis of human coronary artery endothelial cells: evidence from use of antisense LOX-1 mRNA and chemical inhibitors. Arterioscler. Thromb. Vasc. Biol. 20, 1116–1122. doi: 10.1161/01.atv.20.4.1116
Li, R., McCourt, P., Schledzewski, K., Goerdt, S., Moldenhauer, G., Liu, X., et al. (2009). Endocytosis of advanced glycation end-products in bovine choriocapillaris endothelial cells. Microcirculation 16, 640–655. doi: 10.1080/10739680903133185
Li, R., Oteiza, A., Sorensen, K. K., McCourt, P., Olsen, R., Smedsrod, B., et al. (2011). Role of liver sinusoidal endothelial cells and stabilins in elimination of oxidized low-density lipoproteins. Am. J. Physiol. Gastrointest. Liver Physiol. 300, G71–G81. doi: 10.1152/ajpgi.00215.2010
Li, Y., Hao, B., Kuai, X., Xing, G., Yang, J., Chen, J., et al. (2009). C-type lectin LSECtin interacts with DC-SIGNR and is involved in hepatitis C virus binding. Mol. Cell Biochem. 327, 183–190. doi: 10.1007/s11010-009-0056-y
Li, Y., Marzolo, M. P., van Kerkhof, P., Strous, G. J., and Bu, G. (2000). The YXXL motif, but not the two NPXY motifs, serves as the dominant endocytosis signal for low density lipoprotein receptor-related protein. J. Biol. Chem. 275, 17187–17194. doi: 10.1074/jbc.M000490200
Lillis, A. P., Van Duyn, L. B., Murphy-Ullrich, J. E., and Strickland, D. K. (2008). LDL receptor-related protein 1: unique tissue-specific functions revealed by selective gene knockout studies. Physiol. Rev. 88, 887–918. doi: 10.1152/physrev.00033.2007
Lim, S. H., Vaughan, A. T., Ashton-Key, M., Williams, E. L., Dixon, S. V., Chan, H. T., et al. (2011). Fc gamma receptor IIb on target B cells promotes rituximab internalization and reduces clinical efficacy. Blood 118, 2530–2540. doi: 10.1182/blood-2011-01-330357
Linehan, S. A., Martinez-Pomares, L., Stahl, P. D., and Gordon, S. (1999). Mannose receptor and its putative ligands in normal murine lymphoid and nonlymphoid organs: In situ expression of mannose receptor by selected macrophages, endothelial cells, perivascular microglia, and mesangial cells, but not dendritic cells. J. Exp. Med. 189, 1961–1972. doi: 10.1084/jem.189.12.1961
Linehan, S. A., Weber, R., McKercher, S., Ripley, R. M., Gordon, S., and Martin, P. (2005). Enhanced expression of the mannose receptor by endothelial cells of the liver and spleen microvascular beds in the macrophage-deficient PU.1 null mouse. Histochem. Cell Biol. 123, 365–376. doi: 10.1007/s00418-005-0767-4
Ling, W., Lougheed, M., Suzuki, H., Buchan, A., Kodama, T., and Steinbrecher, U. P. (1997). Oxidized or acetylated low density lipoproteins are rapidly cleared by the liver in mice with disruption of the scavenger receptor class A type I/II gene. J. Clin. Invest. 100, 244–252. doi: 10.1172/JCI119528
Liu, J., Jiang, M., Ma, Z., Dietze, K. K., Zelinskyy, G., Yang, D., et al. (2013). TLR1/2 ligand-stimulated mouse liver endothelial cells secrete IL-12 and trigger CD8+ T cell immunity in vitro. J. Immunol. 191, 6178–6190. doi: 10.4049/jimmunol.1301262
Liu, W., Tang, L., Zhang, G., Wei, H., Cui, Y., Guo, L., et al. (2004). Characterization of a novel C-type lectin-like gene, LSECtin: demonstration of carbohydrate binding and expression in sinusoidal endothelial cells of liver and lymph node. J. Biol. Chem. 279, 18748–18758. doi: 10.1074/jbc.M311227200
Liu, Y., Gardner, C. R., Laskin, J. D., and Laskin, D. L. (2013). Classical and alternative activation of rat hepatic sinusoidal endothelial cells by inflammatory stimuli. Exp. Mol. Pathol. 94, 160–167. doi: 10.1016/j.yexmp.2012.10.015
Lollar, P. (1991). The association of factor VIII with von Willebrand factor. Mayo. Clin. Proc. 66, 524–534. doi: 10.1016/s0025-6196(12)62395-7
Lotowska, J. M., Sobaniec-Lotowska, M. E., Sobaniec, P., and Lebensztejn, D. M. (2018). Liver sinusoidal endothelial cells in morphogenesis of pediatric autoimmune hepatitis. Ultrastructural characteristics - a novel report. Pol. J. Pathol. 69, 327–334. doi: 10.5114/pjp.2018.81691
Lozach, P. Y., Amara, A., Bartosch, B., Virelizier, J. L., Arenzana-Seisdedos, F., Cosset, F. L., et al. (2004). C-type lectins L-SIGN and DC-SIGN capture and transmit infectious hepatitis C virus pseudotype particles. J. Biol. Chem. 279, 32035–32045. doi: 10.1074/jbc.M402296200
Maeso-Diaz, R., Ortega-Ribera, M., Fernandez-Iglesias, A., Hide, D., Munoz, L., Hessheimer, A. J., et al. (2018). Effects of aging on liver microcirculatory function and sinusoidal phenotype. Aging Cell 17:e12829. doi: 10.1111/acel.12829
Mak, K. M., Chu, E., Lau, K. H., and Kwong, A. J. (2012). Liver fibrosis in elderly cadavers: localization of collagen types I, III, and IV, alpha-smooth muscle actin, and elastic fibers. Anat. Rec. (Hoboken) 295, 1159–1167. doi: 10.1002/ar.22504
Mak, K. M., and Mei, R. (2017). Basement membrane type iv collagen and laminin: an overview of their biology and value as fibrosis biomarkers of liver disease. Anat. Rec. (Hoboken) 300, 1371–1390. doi: 10.1002/ar.23567
Malerod, L., Juvet, K., Gjoen, T., and Berg, T. (2002). The expression of scavenger receptor class B, type I (SR-BI) and caveolin-1 in parenchymal and nonparenchymal liver cells. Cell Tissue Res. 307, 173–180. doi: 10.1007/s00441-001-0476-9
Malovic, I., Sorensen, K. K., Elvevold, K. H., Nedredal, G. I., Paulsen, S., Erofeev, A. V., et al. (2007). The mannose receptor on murine liver sinusoidal endothelial cells is the main denatured collagen clearance receptor. Hepatology 45, 1454–1461. doi: 10.1002/hep.21639
Maras, J. S., Das, S., Bhat, A., Kumar Vyas, A., Yadav, G., Chaudhary, S., et al. (2019). Dysregulated lipid transport proteins correlate with pathogenesis and outcome in severe alcoholic hepatitis. Hepatol. Commun. 3, 1598–1625. doi: 10.1002/hep4.1438
Martin-Armas, M., Simon-Santamaria, J., Pettersen, I., Moens, U., Smedsrod, B., and Sveinbjornsson, B. (2006). Toll-like receptor 9 (TLR9) is present in murine liver sinusoidal endothelial cells (LSECs) and mediates the effect of CpG-oligonucleotides. J. Hepatol. 44, 939–946. doi: 10.1016/j.jhep.2005.09.020
Martinez-Pomares, L., Wienke, D., Stillion, R., McKenzie, E. J., Arnold, J. N., Harris, J., et al. (2006). Carbohydrate-independent recognition of collagens by the macrophage mannose receptor. Eur. J. Immunol. 36, 1074–1082. doi: 10.1002/eji.200535685
Matsumoto, A., Naito, M., Itakura, H., Ikemoto, S., Asaoka, H., Hayakawa, I., et al. (1990). Human macrophage scavenger receptors: primary structure, expression, and localization in atherosclerotic lesions. Proc. Natl. Acad. Sci. U.S.A. 87, 9133–9137. doi: 10.1073/pnas.87.23.9133
McCourt, P. A., Smedsrod, B. H., Melkko, J., and Johansson, S. (1999). Characterization of a hyaluronan receptor on rat sinusoidal liver endothelial cells and its functional relationship to scavenger receptors. Hepatology 30, 1276–1286. doi: 10.1002/hep.510300521
McGaha, T. L., Sorrentino, B., and Ravetch, J. V. (2005). Restoration of tolerance in lupus by targeted inhibitory receptor expression. Science 307, 590–593. doi: 10.1126/science.1105160
McGreal, E. P., Martinez-Pomares, L., and Gordon, S. (2004). Divergent roles for C-type lectins expressed by cells of the innate immune system. Mol. Immunol. 41, 1109–1121. doi: 10.1016/j.molimm.2004.06.013
Meyer, J., Gonelle-Gispert, C., Morel, P., and Buhler, L. (2016). Methods for isolation and purification of murine liver sinusoidal endothelial cells: a systematic review. PLoS One 11:e0151945. doi: 10.1371/journal.pone.0151945
Miller, C. M., Donner, A. J., Blank, E. E., Egger, A. W., Kellar, B. M., Ostergaard, M. E., et al. (2016). Stabilin-1 and Stabilin-2 are specific receptors for the cellular internalization of phosphorothioate-modified antisense oligonucleotides (ASOs) in the liver. Nucleic Acids Res. 44, 2782–2794. doi: 10.1093/nar/gkw112
Milone, M. C., and Fitzgerald-Bocarsly, P. (1998). The mannose receptor mediates induction of IFN-alpha in peripheral blood dendritic cells by enveloped RNA and DNA viruses. J. Immunol. 161, 2391–2399.
Mitroulis, I., Alexaki, V. I., Kourtzelis, I., Ziogas, A., Hajishengallis, G., and Chavakis, T. (2015). Leukocyte integrins: role in leukocyte recruitment and as therapeutic targets in inflammatory disease. Pharmacol. Ther. 147, 123–135. doi: 10.1016/j.pharmthera.2014.11.008
Miyao, M., Kotani, H., Ishida, T., Kawai, C., Manabe, S., Abiru, H., et al. (2015). Pivotal role of liver sinusoidal endothelial cells in NAFLD/NASH progression. Lab. Invest. 95, 1130–1144. doi: 10.1038/labinvest.2015.95
Monkemoller, V., Oie, C., Hubner, W., Huser, T., and McCourt, P. (2015). Multimodal super-resolution optical microscopy visualizes the close connection between membrane and the cytoskeleton in liver sinusoidal endothelial cell fenestrations. Sci. Rep. 5:16279. doi: 10.1038/srep16279
Mousavi, S. A., Sporstol, M., Fladeby, C., Kjeken, R., Barois, N., and Berg, T. (2007). Receptor-mediated endocytosis of immune complexes in rat liver sinusoidal endothelial cells is mediated by FcgammaRIIb2. Hepatology 46, 871–884. doi: 10.1002/hep.21748
Mouta Carreira, C., Nasser, S. M., di Tomaso, E., Padera, T. P., Boucher, Y., Tomarev, S. I., et al. (2001). LYVE-1 is not restricted to the lymph vessels: expression in normal liver blood sinusoids and down-regulation in human liver cancer and cirrhosis. Cancer Res. 61, 8079–8084.
Murase, T., Kume, N., Korenaga, R., Ando, J., Sawamura, T., Masaki, T., et al. (1998). Fluid shear stress transcriptionally induces lectin-like oxidized LDL receptor-1 in vascular endothelial cells. Circ. Res. 83, 328–333. doi: 10.1161/01.res.83.3.328
Muta, T., Kurosaki, T., Misulovin, Z., Sanchez, M., Nussenzweig, M. C., and Ravetch, J. V. (1994). A 13-amino-acid motif in the cytoplasmic domain of Fc gamma RIIB modulates B-cell receptor signalling. Nature 368, 70–73. doi: 10.1038/368070a0
Nagase, M., Hirose, S., and Fujita, T. (1998). Unique repetitive sequence and unexpected regulation of expression of rat endothelial receptor for oxidized low-density lipoprotein (LOX-1). Biochem. J. 330(Pt 3), 1417–1422. doi: 10.1042/bj3301417
Nagase, M., Hirose, S., Sawamura, T., Masaki, T., and Fujita, T. (1997). Enhanced expression of endothelial oxidized low-density lipoprotein receptor (LOX-1) in hypertensive rats. Biochem. Biophys. Res. Commun. 237, 496–498. doi: 10.1006/bbrc.1997.7176
Nagelkerke, J. F., Barto, K. P., and van Berkel, T. J. (1983). In vivo and in vitro uptake and degradation of acetylated low density lipoprotein by rat liver endothelial, Kupffer, and parenchymal cells. J. Biol. Chem. 258, 12221–12227.
Nakajou, K., Horiuchi, S., Sakai, M., Hirata, K., Tanaka, M., Takeya, M., et al. (2005). CD36 is not involved in scavenger receptor-mediated endocytic uptake of glycolaldehyde- and methylglyoxal-modified proteins by liver endothelial cells. J. Biochem. 137, 607–616. doi: 10.1093/jb/mvi071
Napper, C. E., Drickamer, K., and Taylor, M. E. (2006). Collagen binding by the mannose receptor mediated through the fibronectin type II domain. Biochem. J. 395, 579–586. doi: 10.1042/BJ20052027
Neubauer, K., Ritzel, A., Saile, B., and Ramadori, G. (2000a). Decrease of platelet-endothelial cell adhesion molecule 1-gene-expression in inflammatory cells and in endothelial cells in the rat liver following CCl(4)-administration and in vitro after treatment with TNFalpha. Immunol. Lett. 74, 153–164. doi: 10.1016/s0165-2478(00)00203-0
Neubauer, K., Wilfling, T., Ritzel, A., and Ramadori, G. (2000b). Platelet-endothelial cell adhesion molecule-1 gene expression in liver sinusoidal endothelial cells during liver injury and repair. J. Hepatol. 32, 921–932. doi: 10.1016/s0168-8278(00)80096-3
Newman, P. J., Berndt, M. C., Gorski, J., White, G. C. II, Lyman, S., Paddock, C., et al. (1990). PECAM-1 (CD31) cloning and relation to adhesion molecules of the immunoglobulin gene superfamily. Science 247, 1219–1222. doi: 10.1126/science.1690453
Ogura, S., Kakino, A., Sato, Y., Fujita, Y., Iwamoto, S., Otsui, K., et al. (2009). Lox-1: the multifunctional receptor underlying cardiovascular dysfunction. Circ. J. 73, 1993–1999. doi: 10.1253/circj.cj-09-0587
Ohgami, N., Nagai, R., Ikemoto, M., Arai, H., Kuniyasu, A., Horiuchi, S., et al. (2001). CD36, a member of class B scavenger receptor family, is a receptor for advanced glycation end products. Ann. N. Y. Acad. Sci. 947, 350–355. doi: 10.1111/j.1749-6632.2001.tb03961.x
Oie, C. I., Appa, R. S., Hilden, I., Petersen, H. H., Gruhler, A., Smedsrod, B., et al. (2011). Rat liver sinusoidal endothelial cells (LSECs) express functional low density lipoprotein receptor-related protein-1 (LRP-1). J. Hepatol. 55, 1346–1352. doi: 10.1016/j.jhep.2011.03.013
Oka, K., Sawamura, T., Kikuta, K., Itokawa, S., Kume, N., Kita, T., et al. (1998). Lectin-like oxidized low-density lipoprotein receptor 1 mediates phagocytosis of aged/apoptotic cells in endothelial cells. Proc. Natl. Acad. Sci. U.S.A. 95, 9535–9540. doi: 10.1073/pnas.95.16.9535
O’Neill, L. A., and Bowie, A. G. (2007). The family of five: TIR-domain-containing adaptors in Toll-like receptor signalling. Nat. Rev. Immunol. 7, 353–364. doi: 10.1038/nri2079
Oudar, O., Moreau, A., Feldmann, G., and Scoazec, J. Y. (1998). Expression and regulation of intercellular adhesion molecule-1 (ICAM-1) in organotypic cultures of rat liver tissue. J. Hepatol. 29, 901–909. doi: 10.1016/s0168-8278(98)80117-7
Park, H., Adsit, F. G., and Boyington, J. C. (2005). The 1.4 angstrom crystal structure of the human oxidized low density lipoprotein receptor lox-1. J. Biol. Chem. 280, 13593–13599. doi: 10.1074/jbc.M500768200
Park, S. Y., Jung, M. Y., Kim, H. J., Lee, S. J., Kim, S. Y., Lee, B. H., et al. (2008). Rapid cell corpse clearance by stabilin-2, a membrane phosphatidylserine receptor. Cell Death Differ 15, 192–201. doi: 10.1038/sj.cdd.4402242
Park, S. Y., Jung, M. Y., Lee, S. J., Kang, K. B., Gratchev, A., Riabov, V., et al. (2009). Stabilin-1 mediates phosphatidylserine-dependent clearance of cell corpses in alternatively activated macrophages. J. Cell Sci. 122(Pt 18), 3365–3373. doi: 10.1242/jcs.049569
Pasare, C., and Medzhitov, R. (2004). Toll-like receptors: linking innate and adaptive immunity. Microbes Infect. 6, 1382–1387. doi: 10.1016/j.micinf.2004.08.018
Pasarin, M., La Mura, V., Gracia-Sancho, J., Garcia-Caldero, H., Rodriguez-Vilarrupla, A., Garcia-Pagan, J. C., et al. (2012). Sinusoidal endothelial dysfunction precedes inflammation and fibrosis in a model of NAFLD. PLoS One 7:e32785. doi: 10.1371/journal.pone.0032785
Pempe, E. H., Xu, Y., Gopalakrishnan, S., Liu, J., and Harris, E. N. (2012). Probing structural selectivity of synthetic heparin binding to stabilin protein receptors. J. Biol. Chem. 287, 20774–20783. doi: 10.1074/jbc.M111.320069
Pohlmann, S., Soilleux, E. J., Baribaud, F., Leslie, G. J., Morris, L. S., Trowsdale, J., et al. (2001). DC-SIGNR, a DC-SIGN homologue expressed in endothelial cells, binds to human and simian immunodeficiency viruses and activates infection in trans. Proc. Natl. Acad. Sci. U.S.A. 98, 2670–2675. doi: 10.1073/pnas.051631398
Politz, O., Gratchev, A., McCourt, P. A., Schledzewski, K., Guillot, P., Johansson, S., et al. (2002). Stabilin-1 and -2 constitute a novel family of fasciclin-like hyaluronan receptor homologues. Biochem. J. 362(Pt 1), 155–164. doi: 10.1042/bj3620155
Poltorak, A., He, X., Smirnova, I., Liu, M. Y., Van Huffel, C., Du, X., et al. (1998). Defective LPS signaling in C3H/HeJ and C57BL/10ScCr mice: mutations in Tlr4 gene. Science 282, 2085–2088. doi: 10.1126/science.282.5396.2085
Powell, J. S. (2009). Recombinant factor VIII in the management of hemophilia A: current use and future promise. Ther. Clin. Risk Manag. 5, 391–402. doi: 10.2147/tcrm.s4412
PrabhuDas, M. R., Baldwin, C. L., Bollyky, P. L., Bowdish, D. M. E., Drickamer, K., Febbraio, M., et al. (2017). A consensus definitive classification of scavenger receptors and their roles in health and disease. J. Immunol. 198, 3775–3789. doi: 10.4049/jimmunol.1700373
Prasad, J. M., Young, P. A., and Strickland, D. K. (2016). High affinity binding of the receptor-associated protein D1D2 domains with the low density lipoprotein receptor-related protein (LRP1) involves bivalent complex formation: critical roles of lysineS 60 and 191. J. Biol. Chem. 291, 18430–18439. doi: 10.1074/jbc.M116.744904
Qian, H., Johansson, S., McCourt, P., Smedsrod, B., and Ekblom, M. (2009). Stabilins are expressed in bone marrow sinusoidal endothelial cells and mediate scavenging and cell adhesive functions. Biochem. Biophys. Res. Commun. 390, 883–886. doi: 10.1016/j.bbrc.2009.10.068
Qiu, Y., Liu, S., Chen, H. T., Yu, C. H., Teng, X. D., Yao, H. T., et al. (2013). Upregulation of caveolin-1 and SR-B1 in mice with non-alcoholic fatty liver disease. Hepatobiliary Pancreat. Dis. Int. 12, 630–636. doi: 10.1016/s1499-3872(13)60099-5
Racanelli, V., and Rehermann, B. (2006). The liver as an immunological organ. Hepatology 43(2 Suppl. 1), S54–S62. doi: 10.1002/hep.21060
Reading, P. C., Miller, J. L., and Anders, E. M. (2000). Involvement of the mannose receptor in infection of macrophages by influenza virus. J. Virol. 74, 5190–5197. doi: 10.1128/jvi.74.11.5190-5197.2000
Reboul, E., Klein, A., Bietrix, F., Gleize, B., Malezet-Desmoulins, C., Schneider, M., et al. (2006). Scavenger receptor class B type I (SR-BI) is involved in vitamin E transport across the enterocyte. J. Biol. Chem. 281, 4739–4745. doi: 10.1074/jbc.M509042200
Rein-Fischboeck, L., Krautbauer, S., Eisinger, K., Pohl, R., Meier, E. M., Weiss, T. S., et al. (2015). Hepatic scavenger receptor BI is associated with type 2 diabetes but unrelated to human and murine non-alcoholic fatty liver disease. Biochem. Biophys. Res. Commun. 467, 377–382. doi: 10.1016/j.bbrc.2015.09.149
Rigotti, A., Acton, S. L., and Krieger, M. (1995). The class B scavenger receptors SR-BI and CD36 are receptors for anionic phospholipids. J. Biol. Chem. 270, 16221–16224. doi: 10.1074/jbc.270.27.16221
Rohrer, L., Freeman, M., Kodama, T., Penman, M., and Krieger, M. (1990). Coiled-coil fibrous domains mediate ligand binding by macrophage scavenger receptor type II. Nature 343, 570–572. doi: 10.1038/343570a0
Salama, Y., Lin, S. Y., Dhahri, D., Hattori, K., and Heissig, B. (2019). The fibrinolytic factor tPA drives LRP1-mediated melanoma growth and metastasis. FASEB J. 33, 3465–3480. doi: 10.1096/fj.201801339RRR
Savill, J., Hogg, N., and Haslett, C. (1991). Macrophage vitronectin receptor, CD36, and thrombospondin cooperate in recognition of neutrophils undergoing programmed cell death. Chest 99(Suppl. 3), 6S–7S. doi: 10.1378/chest.99.3_supplement.6s-a
Sawamura, T., Kume, N., Aoyama, T., Moriwaki, H., Hoshikawa, H., Aiba, Y., et al. (1997). An endothelial receptor for oxidized low-density lipoprotein. Nature 386, 73–77. doi: 10.1038/386073a0
Schaffner, F., Ray, A. M., and Dontenwill, M. (2013). Integrin alpha5beta1, the fibronectin receptor, as a pertinent therapeutic target in solid tumors. Cancers (Basel) 5, 27–47. doi: 10.3390/cancers5010027
Schledzewski, K., Geraud, C., Arnold, B., Wang, S., Grone, H. J., Kempf, T., et al. (2011). Deficiency of liver sinusoidal scavenger receptors stabilin-1 and -2 in mice causes glomerulofibrotic nephropathy via impaired hepatic clearance of noxious blood factors. J. Clin. Invest. 121, 703–714. doi: 10.1172/JCI44740
Schurich, A., Bottcher, J. P., Burgdorf, S., Penzler, P., Hegenbarth, S., Kern, M., et al. (2009). Distinct kinetics and dynamics of cross-presentation in liver sinusoidal endothelial cells compared to dendritic cells. Hepatology 50, 909–919. doi: 10.1002/hep.23075
Scoazec, J. Y., Flejou, J. F., D’Errico, A., Couvelard, A., Kozyraki, R., Fiorentino, M., et al. (1995). Focal nodular hyperplasia of the liver: composition of the extracellular matrix and expression of cell-cell and cell-matrix adhesion molecules. Hum. Pathol. 26, 1114–1125. doi: 10.1016/0046-8177(95)90274-0
Seglen, P. O. (1976). Preparation of isolated rat liver cells. Methods Cell Biol. 13, 29–83. doi: 10.1016/s0091-679x(08)61797-5
Shetty, S., Lalor, P. F., and Adams, D. H. (2018). Liver sinusoidal endothelial cells - gatekeepers of hepatic immunity. Nat. Rev. Gastroenterol Hepatol. 15, 555–567. doi: 10.1038/s41575-018-0020-y
Shetty, S., Weston, C. J., Oo, Y. H., Westerlund, N., Stamataki, Z., Youster, J., et al. (2011). Common lymphatic endothelial and vascular endothelial receptor-1 mediates the transmigration of regulatory T cells across human hepatic sinusoidal endothelium. J. Immunol. 186, 4147–4155. doi: 10.4049/jimmunol.1002961
Shih, H. H., Zhang, S., Cao, W., Hahn, A., Wang, J., Paulsen, J. E., et al. (2009). CRP is a novel ligand for the oxidized LDL receptor LOX-1. Am. J. Physiol. Heart Circ. Physiol. 296, H1643–H1650. doi: 10.1152/ajpheart.00938.2008
Simon-Santamaria, J., Malovic, I., Warren, A., Oteiza, A., Le Couteur, D., Smedsrod, B., et al. (2010). Age-related changes in scavenger receptor-mediated endocytosis in rat liver sinusoidal endothelial cells. J. Gerontol. A Biol. Sci. Med. Sci. 65, 951–960. doi: 10.1093/gerona/glq108
Smedsrod, B., Einarsson, M., and Pertoft, H. (1988a). Tissue plasminogen activator is endocytosed by mannose and galactose receptors of rat liver cells. Thromb. Haemost. 59, 480–484. doi: 10.1055/s-0038-1647519
Smedsrod, B., Malmgren, M., Ericsson, J., and Laurent, T. C. (1988b). Morphological studies on endocytosis of chondroitin sulphate proteoglycan by rat liver endothelial cells. Cell Tissue Res. 253, 39–45.
Smedsrod, B., Melkko, J., Risteli, L., and Risteli, J. (1990). Circulating C-terminal propeptide of type I procollagen is cleared mainly via the mannose receptor in liver endothelial cells. Biochem. J. 271, 345–350. doi: 10.1042/bj2710345
Smedsrod, B., Pertoft, H., Eriksson, S., Fraser, J. R., and Laurent, T. C. (1984). Studies in vitro on the uptake and degradation of sodium hyaluronate in rat liver endothelial cells. Biochem. J. 223, 617–626. doi: 10.1042/bj2230617
Sorensen, K. K., Simon-Santamaria, J., McCuskey, R. S., and Smedsrod, B. (2015). Liver sinusoidal endothelial cells. Compr. Physiol. 5, 1751–1774. doi: 10.1002/cphy.c140078
Stahl, P., Schlesinger, P. H., Rodman, J. S., and Doebber, T. (1976). Recognition of lysosomal glycosidases in vivo inhibited by modified glycoproteins. Nature 264, 86–88. doi: 10.1038/264086a0
Stahl, P. D., and Ezekowitz, R. A. (1998). The mannose receptor is a pattern recognition receptor involved in host defense. Curr. Opin. Immunol. 10, 50–55. doi: 10.1016/s0952-7915(98)80031-9
Steinhoff, G., Behrend, M., Schrader, B., Duijvestijn, A. M., and Wonigeit, K. (1993). Expression patterns of leukocyte adhesion ligand molecules on human liver endothelia. Lack of ELAM-1 and CD62 inducibility on sinusoidal endothelia and distinct distribution of VCAM-1, ICAM-1, ICAM-2, and LFA-3. Am. J. Pathol. 142, 481–488.
Strauss, O., Phillips, A., Ruggiero, K., Bartlett, A., and Dunbar, P. R. (2017). Immunofluorescence identifies distinct subsets of endothelial cells in the human liver. Sci. Rep. 7:44356. doi: 10.1038/srep44356
Sutter, A. G., Palanisamy, A. P., Lench, J. H., Esckilsen, S., Geng, T., Lewin, D. N., et al. (2016). Dietary saturated fat promotes development of hepatic inflammation through toll-like receptor 4 in mice. J. Cell Biochem. 117, 1613–1621. doi: 10.1002/jcb.25453
Suzuki, H., Kurihara, Y., Takeya, M., Kamada, N., Kataoka, M., Jishage, K., et al. (1997). A role for macrophage scavenger receptors in atherosclerosis and susceptibility to infection. Nature 386, 292–296. doi: 10.1038/386292a0
Swystun, L. L., Lai, J. D., Notley, C., Georgescu, I., Paine, A. S., Mewburn, J., et al. (2018). The endothelial cell receptor stabilin-2 regulates VWF-FVIII complex half-life and immunogenicity. J. Clin. Invest. 128, 4057–4073. doi: 10.1172/JCI96400
Swystun, L. L., Notley, C., Georgescu, I., Lai, J. D., Nesbitt, K., James, P. D., et al. (2019). The endothelial lectin clearance receptor CLEC4M binds and internalizes factor VIII in a VWF-dependent and independent manner. J. Thromb. Haemost 17, 681–694. doi: 10.1111/jth.14404
Taira, K. (1994). Trabecular meshworks in the sinusoidal endothelial cells of the golden hamster liver: a freeze-fracture study. J. Submicrosc. Cytol. Pathol. 26, 271–277.
Talle, M. A., Rao, P. E., Westberg, E., Allegar, N., Makowski, M., Mittler, R. S., et al. (1983). Patterns of antigenic expression on human monocytes as defined by monoclonal antibodies. Cell Immunol. 78, 83–99. doi: 10.1016/0008-8749(83)90262-9
Tandon, N. N., Kralisz, U., and Jamieson, G. A. (1989a). Identification of glycoprotein IV (CD36) as a primary receptor for platelet-collagen adhesion. J. Biol. Chem. 264, 7576–7583.
Tandon, N. N., Lipsky, R. H., Burgess, W. H., and Jamieson, G. A. (1989b). Isolation and characterization of platelet glycoprotein IV (CD36). J. Biol. Chem. 264, 7570–7575.
Tang, L., Yang, J., Liu, W., Tang, X., Chen, J., Zhao, D., et al. (2009). Liver sinusoidal endothelial cell lectin. LSECtin, negatively regulates hepatic T-cell immune response. Gastroenterology 149, e1491–e1495. doi: 10.1053/j.gastro.2009.07.051
Taylor, M. E., Bezouska, K., and Drickamer, K. (1992). Contribution to ligand binding by multiple carbohydrate-recognition domains in the macrophage mannose receptor. J. Biol. Chem. 267, 1719–1726.
Tsugita, M., Morimoto, N., Tashiro, M., Kinoshita, K., and Nakayama, M. (2017). SR-B1 is a silica receptor that mediates canonical inflammasome activation. Cell Rep. 18, 1298–1311. doi: 10.1016/j.celrep.2017.01.004
Turville, S. G., Cameron, P. U., Handley, A., Lin, G., Pohlmann, S., Doms, R. W., et al. (2002). Diversity of receptors binding HIV on dendritic cell subsets. Nat. Immunol. 3, 975–983. doi: 10.1038/ni841
Tutt, A. L., James, S., Laversin, S. A., Tipton, T. R., Ashton-Key, M., French, R. R., et al. (2015). Development and characterization of monoclonal antibodies specific for mouse and human fcgamma receptors. J. Immunol. 195, 5503–5516. doi: 10.4049/jimmunol.1402988
Uhrig, A., Banafsche, R., Kremer, M., Hegenbarth, S., Hamann, A., Neurath, M., et al. (2005). Development and functional consequences of LPS tolerance in sinusoidal endothelial cells of the liver. J. Leukoc. Biol. 77, 626–633. doi: 10.1189/jlb.0604332
Van Agthoven, J. F., Xiong, J. P., Alonso, J. L., Rui, X., Adair, B. D., Goodman, S. L., et al. (2014). Structural basis for pure antagonism of integrin alphaVbeta3 by a high-affinity form of fibronectin. Nat. Struct. Mol. Biol. 21, 383–388. doi: 10.1038/nsmb.2797
Van Berkel, T. J., Van Velzen, A., Kruijt, J. K., Suzuki, H., and Kodama, T. (1998). Uptake and catabolism of modified LDL in scavenger-receptor class A type I/II knock-out mice. Biochem. J. 331(Pt 1), 29–35. doi: 10.1042/bj3310029
Van den Herik-Oudijk, I. E., Capel, P. J., van der Bruggen, T., and Van de Winkel, J. G. (1995). Identification of signaling motifs within human Fc gamma RIIa and Fc gamma RIIb isoforms. Blood 85, 2202–2211. doi: 10.1182/blood.v85.8.2202.bloodjournal8582202
Varban, M. L., Rinninger, F., Wang, N., Fairchild-Huntress, V., Dunmore, J. H., Fang, Q., et al. (1998). Targeted mutation reveals a central role for SR-BI in hepatic selective uptake of high density lipoprotein cholesterol. Proc. Natl. Acad. Sci. U.S.A. 95, 4619–4624. doi: 10.1073/pnas.95.8.4619
Vasselon, T., and Detmers, P. A. (2002). Toll receptors: a central element in innate immune responses. Infect. Immun. 70, 1033–1041. doi: 10.1128/iai.70.3.1033-1041.2002
Vilar-Gomez, E., Calzadilla-Bertot, L., Friedman, S. L., Gra-Oramas, B., Gonzalez-Fabian, L., Lazo-Del Vallin, S., et al. (2017). Serum biomarkers can predict a change in liver fibrosis 1 year after lifestyle intervention for biopsy-proven NASH. Liver Int. 37, 1887–1896. doi: 10.1111/liv.13480
Volpes, R., van den Oord, J. J., De Vos, R., and Desmet, V. J. (1992). Hepatic expression of type A and type B receptors for tumor necrosis factor. J. Hepatol. 14, 361–369. doi: 10.1016/0168-8278(92)90184-q
Walling, B. L., and Kim, M. (2018). LFA-1 in T cell migration and differentiation. Front. Immunol. 9:952. doi: 10.3389/fimmu.2018.00952
Walsh, K. M., Fletcher, A., MacSween, R. N., and Morris, A. J. (2000). Basement membrane peptides as markers of liver disease in chronic hepatitis C. J. Hepatol. 32, 325–330. doi: 10.1016/s0168-8278(00)80079-3
Webb, N. R., de Villiers, W. J., Connell, P. M., de Beer, F. C., and van der Westhuyzen, D. R. (1997). Alternative forms of the scavenger receptor BI (SR-BI). J. Lipid Res. 38, 1490–1495.
Weigel, J. A., and Weigel, P. H. (2003). Characterization of the recombinant rat 175-kDa hyaluronan receptor for endocytosis (HARE). J. Biol. Chem. 278, 42802–42811. doi: 10.1074/jbc.M307201200
Weigel, P. H. (2019). Discovery of the liver hyaluronan receptor for endocytosis (HARE) and its progressive emergence as the multi-ligand scavenger receptor stabilin-2. Biomolecules 9:454. doi: 10.3390/biom9090454
Werling, D., and Jungi, T. W. (2003). TOLL-like receptors linking innate and adaptive immune response. Vet. Immunol. Immunopathol. 91, 1–12. doi: 10.1016/s0165-2427(02)00228-3
Wippler, J., Kouns, W. C., Schlaeger, E. J., Kuhn, H., Hadvary, P., and Steiner, B. (1994). The integrin alpha IIb-beta 3, platelet glycoprotein IIb-IIIa, can form a functionally active heterodimer complex without the cysteine-rich repeats of the beta 3 subunit. J. Biol. Chem. 269, 8754–8761.
Wisse, E. (1970). An electron microscopic study of the fenestrated endothelial lining of rat liver sinusoids. J. Ultrastruct. Res. 31, 125–150. doi: 10.1016/s0022-5320(70)90150-4
Wisse, E. (1972). An ultrastructural characterization of the endothelial cell in the rat liver sinusoid under normal and various experimental conditions, as a contribution to the distinction between endothelial and Kupffer cells. J. Ultrastruct. Res. 38, 528–562. doi: 10.1016/0022-5320(72)90089-5
Wisse, E., Braet, F., Luo, D., De Zanger, R., Jans, D., Crabbe, E., et al. (1996). Structure and function of sinusoidal lining cells in the liver. Toxicol. Pathol. 24, 100–111. doi: 10.1177/019262339602400114
Wisse, E., De Zanger, R. B., Charels, K., Van Der Smissen, P., and McCuskey, R. S. (1985). The liver sieve: considerations concerning the structure and function of endothelial fenestrae, the sinusoidal wall and the space of Disse. Hepatology 5, 683–692. doi: 10.1002/hep.1840050427
Wong, J., Johnston, B., Lee, S. S., Bullard, D. C., Smith, C. W., Beaudet, A. L., et al. (1997). A minimal role for selectins in the recruitment of leukocytes into the inflamed liver microvasculature. J. Clin. Invest. 99, 2782–2790. doi: 10.1172/JCI119468
Wu, J., Lu, M., Meng, Z., Trippler, M., Broering, R., Szczeponek, A., et al. (2007). Toll-like receptor-mediated control of HBV replication by nonparenchymal liver cells in mice. Hepatology 46, 1769–1778. doi: 10.1002/hep.21897
Wu, J., Meng, Z., Jiang, M., Zhang, E., Trippler, M., Broering, R., et al. (2010). Toll-like receptor-induced innate immune responses in non-parenchymal liver cells are cell type-specific. Immunology 129, 363–374. doi: 10.1111/j.1365-2567.2009.03179.x
Xu, B., Broome, U., Uzunel, M., Nava, S., Ge, X., Kumagai-Braesch, M., et al. (2003). Capillarization of hepatic sinusoid by liver endothelial cell-reactive autoantibodies in patients with cirrhosis and chronic hepatitis. Am. J. Pathol. 163, 1275–1289. doi: 10.1016/S0002-9440(10)63487-6
Yannariello-Brown, J., Zhou, B., and Weigel, P. H. (1997). Identification of a 175 kDa protein as the ligand-binding subunit of the rat liver sinusoidal endothelial cell hyaluronan receptor. Glycobiology 7, 15–21. doi: 10.1093/glycob/7.1.15
Yap, N. V., Whelan, F. J., Bowdish, D. M., and Golding, G. B. (2015). The evolution of the scavenger receptor cysteine-rich domain of the class a scavenger receptors. Front. Immunol. 6:342. doi: 10.3389/fimmu.2015.00342
Yin, Z., Jiang, G., Fung, J. J., Lu, L., and Qian, S. (2007). ICAM-1 expressed on hepatic stellate cells plays an important role in immune regulation. Microsurgery 27, 328–332. doi: 10.1002/micr.20366
Yokomori, H. (2008). New insights into the dynamics of sinusoidal endothelial fenestrae in liver sinusoidal endothelial cells. Med. Mol. Morphol. 41, 1–4. doi: 10.1007/s00795-007-0390-7
Yoshida, H., Kondratenko, N., Green, S., Steinberg, D., and Quehenberger, O. (1998). Identification of the lectin-like receptor for oxidized low-density lipoprotein in human macrophages and its potential role as a scavenger receptor. Biochem. J. 334(Pt 1), 9–13. doi: 10.1042/bj3340009
Yue, F., Cheng, Y., Breschi, A., Vierstra, J., Wu, W., Ryba, T., et al. (2014). A comparative encyclopedia of DNA elements in the mouse genome. Nature 515, 355–364. doi: 10.1038/nature13992
Zapotoczny, B., Szafranska, K., Kus, E., Braet, F., Wisse, E., Chlopicki, S., et al. (2019). Tracking fenestrae dynamics in live murine liver sinusoidal endothelial cells. Hepatology 69, 876–888. doi: 10.1002/hep.30232
Zhang, Q., Liu, J., Liu, J., Huang, W., Tian, L., Quan, J., et al. (2014). oxLDL induces injury and defenestration of human liver sinusoidal endothelial cells via LOX1. J. Mol. Endocrinol. 53, 281–293. doi: 10.1530/JME-14-0049
Keywords: liver, sinusoidal endothelial cells, scavenger receptors, cell surface receptor, endocytosis, ligand binding
Citation: Pandey E, Nour AS and Harris EN (2020) Prominent Receptors of Liver Sinusoidal Endothelial Cells in Liver Homeostasis and Disease. Front. Physiol. 11:873. doi: 10.3389/fphys.2020.00873
Received: 31 March 2020; Accepted: 29 June 2020;
Published: 21 July 2020.
Edited by:
Leo A. van Grunsven, Vrije University Brussel, BelgiumReviewed by:
Karen Kristine Sørensen, UiT The Arctic University of Norway, NorwayMatthias J. Bahr, Sana Kliniken Lübeck GmbH, Germany
Copyright © 2020 Pandey, Nour and Harris. This is an open-access article distributed under the terms of the Creative Commons Attribution License (CC BY). The use, distribution or reproduction in other forums is permitted, provided the original author(s) and the copyright owner(s) are credited and that the original publication in this journal is cited, in accordance with accepted academic practice. No use, distribution or reproduction is permitted which does not comply with these terms.
*Correspondence: Edward N. Harris, ZWhhcnJpczVAdW5sLmVkdQ==