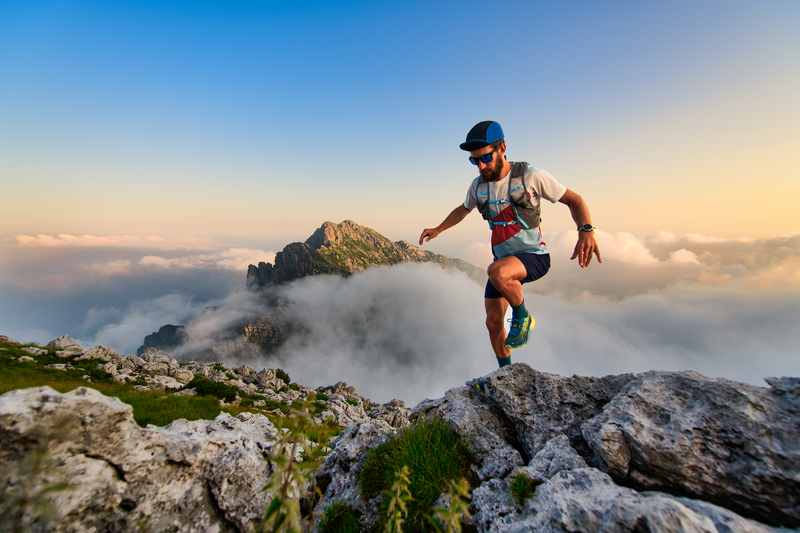
94% of researchers rate our articles as excellent or good
Learn more about the work of our research integrity team to safeguard the quality of each article we publish.
Find out more
REVIEW article
Front. Physiol. , 26 June 2020
Sec. Membrane Physiology and Membrane Biophysics
Volume 11 - 2020 | https://doi.org/10.3389/fphys.2020.00634
This article is part of the Research Topic Kv7 Channels: Structure, Physiology and Pharmacology View all 18 articles
Lung diseases constitute a global health concern causing disability. According to WHO in 2016, respiratory diseases accounted for 24% of world population mortality, the second cause of death after cardiovascular diseases. The Kv7 channels family is a group of voltage-dependent K+ channels (Kv) encoded by KCNQ genes that are involved in various physiological functions in numerous cell types, especially, cardiac myocytes, smooth muscle cells, neurons, and epithelial cells. Kv7 channel α-subunits are regulated by KCNE1–5 ancillary β-subunits, which modulate several characteristics of Kv7 channels such as biophysical properties, cell-location, channel trafficking, and pharmacological sensitivity. Kv7 channels are mainly expressed in two large groups of lung tissues: pulmonary arteries (PAs) and bronchial tubes. In PA, Kv7 channels are expressed in pulmonary artery smooth muscle cells (PASMCs); while in the airway (trachea, bronchus, and bronchioles), Kv7 channels are expressed in airway smooth muscle cells (ASMCs), airway epithelial cells (AEPs), and vagal airway C-fibers (VACFs). The functional role of Kv7 channels may vary depending on the cell type. Several studies have demonstrated that the impairment of Kv7 channel has a strong impact on pulmonary physiology contributing to the pathophysiology of different respiratory diseases such as cystic fibrosis, asthma, chronic obstructive pulmonary disease, chronic coughing, lung cancer, and pulmonary hypertension. Kv7 channels are now recognized as playing relevant physiological roles in many tissues, which have encouraged the search for Kv7 channel modulators with potential therapeutic use in many diseases including those affecting the lung. Modulation of Kv7 channels has been proposed to provide beneficial effects in a number of lung conditions. Therefore, Kv7 channel openers/enhancers or drugs acting partly through these channels have been proposed as bronchodilators, expectorants, antitussives, chemotherapeutics and pulmonary vasodilators.
Lung diseases constitute a global health concern causing severe physical limitations and premature death, and entails enormous costs associated with treatment, hospital-based and primary care (Tobin, 2005). Mortality from overall lung diseases is very striking according to data collected by the WHO in 2016, in which respiratory diseases account for 24% of world population mortality, the second cause of death after cardiovascular diseases (WHO Global Health Estimates, 2020). At the top of mortality rate within lung diseases, the 9% of the population dies of tuberculosis. Second and third positions include chronic obstructive pulmonary disease (COPD) and lower respiratory infections with 5.4 and 5.2%, respectively. These are followed by lung cancer (affecting trachea, bronchus, and lungs) with 3% and, lastly, asthma and other respiratory diseases with a 0.7 and 0.6%, respectively (Figure 1). Most major respiratory diseases are avoidable and can be mitigated by reducing exposure to air pollution, toxic smoke of biomass fuel, tobacco smoke, and different allergens (Laumbach and Kipen, 2012). Additional factors, such as aging, genetic predisposition, nutritional status, obesity, physical inactivity, raised blood pressure, human immunodeficiency virus 1 infection (HIV-1), or other infections may influence the risk of developing lung diseases. Lung diseases can be classified into three main groups depending on the lung tissue involved. First, airways diseases including asthma, COPD, emphysema, cystic fibrosis, or bronchitis in which the bronchial tubes are narrowed or blocked and the transport of oxygen and other gases are affected (Athanazio, 2012). Second, lung tissue diseases which affect the structure of alveoli or the interstitium (Corvol et al., 2009), such as pneumonia, tuberculosis, pulmonary edema, acute respiratory distress syndrome, sarcoidosis, and pulmonary fibrosis, where the lungs are unable to expand fully (Dellaripa, 2018). The third group includes diseases affecting the pulmonary vessels, such as pulmonary hypertension characterized by vasoconstriction, vascular remodeling, inflammation, and thrombosis (Cummings and Bhalla, 2015). This last group of diseases may end up affecting heart function.
Figure 1. Global lung diseases epidemiological data adapted from the World Health Organization (WHO) Website: Mortality rates in 2016 measured as % of total deaths.
Coronavirus disease 2019 (COVID-19), caused by severe acute respiratory syndrome coronavirus 2 (SARS-CoV-2), has recently rapidly evolved into a global pandemic. To date, risk factors associated with admission to ICU and mortality include cardiovascular comorbidities while respiratory diseases paradoxically do not appear to increase the prevalence or severity of the viral infection (Halpin et al., 2020; Li et al., 2020).
The Kv7 channel family, is a group of voltage-dependent K+ channels subfamily Q, which are involved in various physiological activities of numerous cell types, especially, cardiac myocytes, smooth muscle cells, neurons, and epithelial cells (Stott et al., 2014). The different Kv7 channels isoforms are encoded by KCNQ1–5 genes located at chromosomal loci 11p15, 20q13, 8q24, 1p34, and 6q13, respectively (Barrese et al., 2018). KCNQ genes encode for five Kv7 proteins, Kv7.1–Kv7.5 channels, comprising approximately 650–940 aminoacids. Each pore-forming α-subunit of Kv7 channels has six helices transmembrane domains (TM). The TM5 and TM6 are part of the pore while the TM4 constitutes the voltage-detection domain. Four α-subunits form the transmembrane pore assembling into a homo‐ or hetero-tetrameric channel (Taylor and Sanders, 2017). Kv7 channels can be regulated by potassium voltage-gated channel subfamily E regulatory subunits (KCNEs) ancillary subunits (KCNE1–5, also called minK-related peptides), which present a single transmembrane domain with 103–177 residues, encoded by the five KCNE1–5 genes located at chromosomal loci 21q11, 21q11, 11q13, 2q36, and Xq23, respectively (Lundquist et al., 2006). These KCNE subunits modulate several features of Kv7 channels such as biophysical properties, cell localization, channel trafficking, and sensitivity to different drugs (Barrese et al., 2018).
Mutations in all five KCNQ and KCNE genes have been shown to underlie excitability hereditary disorders (Jentsch, 2000; Maljevic et al., 2010; Lehman et al., 2017; Barrese et al., 2018). Particularly, mutations affecting KCNQ1 gene cause disorders related to cardiac electrical activity such as long-QT syndrome, short-QT syndrome, and atrial fibrillation leading to severe arrhythmias and sudden death (Li et al., 1998; Chen et al., 2003). KCNQ2 and KCNQ3 gene mutations have been identified in different epilepsy syndromes (Neubauer et al., 2008) and, moreover, KCNQ3 gain-of-function variant have been related to autism and developmental disability (Sands et al., 2019). Kv7.4 channels expressed in the cochlea are essential for normal hearing, and KCNQ4 mutations are linked to hearing loss (Kubisch et al., 1999; Jung et al., 2019). Lastly, mutations in the Kv7.5 channel encoded by KCNQ5 gene cause epilepsy and intellectual disability (Lehman et al., 2017). Different mutations have also been found in all five KCNE genes, mainly related to cardiac arrhythmias (Splawski et al., 2000; Lundby et al., 2008; Ohno et al., 2009; Abbott, 2016).
Different combinations of Kv7 channels and KCNE subunits can be found depending on the cell type. Thus, there is a Kv7 channel isoform-specific expression playing different roles depending on tissue types (see section Kv7 Channel Expression in the Lung). Many KCNE subunits have been shown to modulate Kv7 channels α-subunits expressed in heterologous systems, providing a valuable experimental platform for elucidating structure-function relationships and mechanistic differences. Thus, all five KCNE proteins have been demonstrated to modulate Kv7.1 channels expressed in heterologous systems with diverse effects (Van Horn et al., 2011; Sun and MacKinnon, 2020). For instance, KCNE1 causes the Kv7.1 channel to activate at more positive voltages, slows its activation and deactivation, increases its conductance, and suppresses its inactivation (Sun and MacKinnon, 2020). While, KCNE2 and KCNE4 have inhibitory effects on Kv7.1 activity (Grunnet et al., 2002; Vanoye et al., 2009; Hu et al., 2019), KCNE3 stabilizes Kv7.1 channels voltage sensors (S4 segment) in an activated state turning the channel voltage-independent (Barro-Soria et al., 2015). Such a mechanism seems to require the participation of the signaling lipid phosphatidylinositol 4,5-bisphosphate (PIP2) in non-excitable cells such as lung epithelial cells (Zhou et al., 2019; Sun and MacKinnon, 2020). Sun and MacKinnon have recently identified binding sites of PIP2 on Kv7.1 channels present in the S0, the S2-S3 loop, and the S4-S5 linker, and proposed a model in which the conformational change following PIP2 interaction would results in dilation of the pore’s gate (Sun and MacKinnon, 2020). In addition, KCNE subunits regulate traffic and cell surface expression of Kv7.1 channels (Roura-Ferrer et al., 2010; Hu et al., 2019). The information about the regulation of Kv7.2 and Kv7.3 channels by KCNE subunits is more limited. In Xenopus oocytes, KCNE1 was found to slow Kv7.2/7.3 heteromer currents and decreased their magnitude (Yang et al., 1998). Likewise, KCNE2 was shown to accelerate the deactivation kinetics of Kv7.2 and Kv7.2/7.3 channels transiently expressed in COS cells (Tinel et al., 2000). Whereas, KCNE1, KCNE2, and especially KCNE4 and KCNE5 increased Kv7.4 currents, co-expression with KCNE3 led to a strong attenuation (Strutz-Seebohm et al., 2006; de Jong and Jepps, 2018). Moreover, KCNE4 was shown to co-localize with Kv7.4 channels, regulating its activity and membrane abundance in mesenteric arterial myocytes (Jepps et al., 2015). Finally, Kv7.5 channels were shown to be affected by KCNE1 and KCNE3, but not by other KCNE subunits in Xenopus oocytes and HEK-293 cells (Roura-Ferrer et al., 2009, 2012). Thus, while KCNE1 was shown to slow activation and increase Kv7.5 currents, KCNE3 drastically inhibited these currents (Roura-Ferrer et al., 2009, 2012).
The activity of Kv7 channels is modulated by G protein-coupled membrane receptors (GPCRs). Thus, activation of different GPCR have been shown to inhibit M-type (Kv7) currents, with a dominant participation of the Gq and G11 α-subunits (Caulfield et al., 1994; Haley et al., 1998; Delmas and Brown, 2005). For instance, in neurons, Kv7 activity is decreased by M1 and M3 muscarinic and H1 histaminic receptors coupled to Gq/11 proteins through different signal transduction pathways, including depletion of PIP2, activation of protein kinase C (PKC), or increased intracellular calcium (Delmas and Brown, 2005; Higashida et al., 2005; Taylor and Sanders, 2017; Barrese et al., 2018). In human airway smooth muscle cells, histamine was found to inhibit Kv7.5 channels though a PKC-dependent phosphorylation of Ser441 (Haick et al., 2017). On the other hand, it is well recognized that stimulation of β-adrenergic receptor increases the activity of different Kv7 channels in different tissues such as cardiac (Kv7.1), neuronal (Kv7.2/Kv7.3), and vascular (Kv7.4/Kv7.5 and Kv7.5) channels (Jentsch, 2000; Marx et al., 2002; Chadha et al., 2014; Mani et al., 2016; Barrese et al., 2018). Likewise, in human airway smooth muscle cells, Kv7.5 channels are robustly enhanced by activation of β-adrenoceptors via a mechanism involving cyclic adenosine monophosphate/protein kinase A (cAMP/PKA)-dependent phosphorylation (Brueggemann et al., 2018). This effect might be shared with other GPCRs but does not seem to account for Kv7.4 activation. The mechanism may involve increased membrane expression of Kv7 channels mediated by colchicine-sensitive microtubule disruption (Lindman et al., 2018). Kv7 channels are also regulated by GPCR-independent mediators activating soluble guanylate cyclase (sGC) such as nitric oxide (NO) (Mondejar-Parreño et al., 2019) and natriuretic peptide (Stott et al., 2015). The implications in respiratory diseases and drug therapy are discussed below.
In addition to regulation by KCNE β-subunits and GPCRs, Kv7 channels can be regulated by scaffolding proteins, sodium-coupled myo-inositol transporter (SMIT), lipids, microRNAs, and/or further signaling mechanisms as extensively reviewed elsewhere (Delmas and Brown, 2005; Zaydman and Cui, 2014; Taylor and Sanders, 2017; Barrese et al., 2018; Byron and Brueggemann, 2018).
Kv7 channels are expressed mainly in pulmonary arteries (PAs) and in the bronchial tubes (Figure 2). In PA, Kv7 channels are expressed in pulmonary artery smooth muscle cells (PASMCs); while in the bronchial tubes (trachea, bronchus and bronchioles), Kv7 channels are expressed in airway smooth muscle cells (ASMCs), airway epithelial cells (AEPs) and vagal airway C-fibers (VACFs). The functional role of Kv7 channels may vary depending on the cell type. While most of the available studies have provided relevant information on the particular expression of α-subunits in these cell types, evidence for the expression of heteromeric Kv7 complexes or KCNE subunits is still very scarce.
Figure 2. Expression and functions of isoform-specific Kv7 channel and potassium voltage-gated channel subfamily E regulatory subunits (KCNE) ancillary subunit in lung cells.
The expression of Kv7 channels in mice and rat PA has been demonstrated in several studies. Specifically, their expression is located in PASMC. In these cells, the expression of Kv7.1, Kv7.4, and Kv7.5 channels predominates; but Kv7.4 channels expression appears to be the most relevant. The expression of Kv7.2 and Kv7.3 channels in PASMCs is still controversial (Joshi et al., 2009; Morecroft et al., 2009; Chadha et al., 2012; Li et al., 2014a; Sedivy et al., 2015; Eid and Gurney, 2018; Mondejar-Parreño et al., 2018). The existence of Kv7.1/7.5 or Kv7.4/7.5 heteromeric complexes in vascular smooth muscle has been postulated on several studies (Chadha et al., 2014; Oliveras et al., 2014; Barrese et al., 2018). In particular, Kv7.4/7.5 heteromers have been proposed as the dominant functional vascular Kv7 channels, supported by electrophysiological data showing that Kv7 currents in freshly isolated vascular smooth muscle cells have phenotypes intermediate to the respective homomeric Kv7.4 and Kv7.5 channels (Brueggemann et al., 2014a; Chadha et al., 2014; Fosmo and Skraastad, 2017; Barrese et al., 2018). We have recently confirmed that Kv7.4/7.5 heteromeric complexes are also present in PASMC (authors’ unpublished observations). Although, in other types of arteries, the expression of Kv7 channels has been located also in endothelial cells (Goodwill et al., 2016), whether this is also the case for pulmonary artery endothelial cells (PAECs) remains unknown. The main function of these channels is to regulate pulmonary vascular tone, due to their contribution to maintain the membrane potential (Em) of PASMC (Joshi et al., 2009; Sedivy et al., 2015; Mondejar-Parreño et al., 2018, 2019).
There is some interspecies variability in the expression of Kv7 channel isoforms in the airways. Thus, ASMCs from guinea pigs were shown to express Kv7 channels with a relative abundance of KCNQ2 > KCNQ5 > KCNQ4 > KCNQ3 and, to a lesser extent, KCNQ1 (Brueggemann et al., 2011). Comparatively, KCNQ mRNA expression in ASMC revealed a high expression of KCNQ4 followed by a fainter expression of KCNQ5 and KCNQ1 transcripts (Brueggemann et al., 2014b). Studies in human tracheal muscle revealed abundant expression of KCNQ1 relative to guinea pig, a modest expression of KCNQ4 and KCNQ5 and no detectable expression of KCNQ2 or KCNQ3 (Brueggemann et al., 2011). A further study showed that cultured human ASMCs express all KCNQ genes with a predominant relative abundance of KCNQ5 mRNA followed by KCNQ3 or KCNQ4, while KCNQ2 and KCNQ1 mRNA are expressed to a lesser extent (Brueggemann et al., 2018). These differences in the expression profile of Kv7 channels in human tissue could be due to phenotypic changes associated with cell culture. Measurements of Kv7 currents and functional studies assessing airway smooth muscle contractility in the bronchioles from different species have pointed to Kv7 channels as important players in maintaining, resting Em, and controlling ASMC excitability (Evseev et al., 2013; Brueggemann et al., 2014b, 2018).
Airway epithelial cells (AECs) predominates the expression of Kv7.1 channels, also named KvLQT1, along with the expression of KCNE1 and KCNE3 ancillary subunits (Mall et al., 2000; Boucherot et al., 2001; Trinh et al., 2007; Preston et al., 2010; Frizzell and Hanrahan, 2012; Kroncke et al., 2016; Hynes and Harvey, 2019). Nevertheless, KCNE2 has also been found to be expressed in Calu-3 cells, a model for human submucosal serous cells of the lung, where it has been suggested to regulate KCNQ1 (Cowley and Linsdell, 2002a). Chloride (Cl−) secretion in AEC is essential to mucociliary clearance and requires the co-activation of Cl− channels in luminal membrane and, in parallel, of K+ channels in the basolateral membrane which involves Kv7.1 channels (Frizzell and Hanrahan, 2012).
Using single-cell mRNA, Sun et al. have recently demonstrated that KCNQ2, KCNQ3, and KCNQ5 are consistently expressed in mouse airway sensory afferent nerves and remarkably, the expression of KCNQ3 mRNA was the most prevalent (Sun et al., 2019). Most vagal sensory nerves in the respiratory tract are nociceptive C-fibers. Increased VACF activity may contribute to the nonproductive coughing, dyspnea, parasympathetic reflex mucus secretion, and bronchoconstriction. Thus, it has been proposed that activation of Kv7 channels may reduce the vagal C-fibers excitability innervating the airways, and being useful to treat chronic cough.
This section covers the potential pathophysiological role of Kv7 channels in different respiratory diseases such as cystic fibrosis, asthma, COPD, chronic coughing, lung cancer, and pulmonary hypertension.
The airway surface liquid layer represents a key primary defense mechanism of mucociliary clearance, which crucially depends on the transport of chloride into the lumen of the airways, and the subsequent movement of water. Cl− ion transport across the apical membrane is mainly driven by a cAMP-regulated Cl− channel named cystic fibrosis transmembrane conductance regulator (CFTR) and by the calcium-activated Cl− channels (CaCC) (Frizzell and Hanrahan, 2012; Bartoszewski et al., 2017). However, a possible overlap between these two pathways has also been proposed (Billet and Hanrahan, 2013). Cystic fibrosis is an autosomal recessive disease due to mutations in the gene encoding CFTR and characterized by alterations in the composition and volume of secreted luminal fluids. The CFTR is a member of the ATP-binding cassette (ABC) transporter superfamily known to function as an ion cannel that conducts chloride ions across epithelial cell membranes and is responsible for salt secretion in response to cAMP/PKA stimulation. Thus, mutations of the CFTR gene lead to defective cAMP-dependent Cl− secretion and enhanced Na+ absorption throughout the apical membrane of airway epithelia.
CFTR has been shown to influence both function and properties of other membrane channels, such as epithelial Na+ channels, inward rectifying renal outer medullary K+ channel (ROMK), outwardly rectifying Cl− channels, and aquaporin 3 (Schwiebert et al., 1999). Cl− secretion in AEC requires the parallel co-activation of Cl− channels and K+ channels, in the luminal surface and the basolateral membrane, respectively. Whereas Cl− channels are required for Cl− efflux to the luminal side of the AEC, K+ channels are needed for K+ recycling via the basolateral membrane (Boucherot et al., 2001; Bartoszewski et al., 2017). Kv7.1 channels have been shown to contribute to the basolateral cAMP-activated K+ conductance in human airway epithelium (Mall et al., 1996, 2000; Bardou et al., 2009). This study also showed that the Kv7.1 channel inhibitor chromanol 293B inhibited Cl− secretion suggesting an important role of these K+ channels in maintaining cAMP-dependent Cl− secretion in human airways. Since CFTR is a modulator of other ion channels, several studies have analyzed whether it controls the activity of Kv7.1 channels. Studies analyzing this issue have led to contradictory results. Thus, while initial studies suggested that CFTR could directly modulate Kv7.1 conductance (Cunningham et al., 1992; Loussouarn et al., 1996; Mall et al., 2000), this was not the case in other studies (Boucherot et al., 2001). Interestingly, using CFTR KO mice, Belfodil et al. (2003) found that cAMP-sensitive Kv7.1 currents were regulated by CFTR in renal distal but not in proximal tubule cells, suggesting that the possible modulation of Kv7.1 is unlikely to involve a direct interaction.
As stated above, human airway epithelia also express CaCC that are activated by extracellular nucleotides [adenosine triphosphate (ATP) and/or uracil triphosphate (UTP)] and other Ca2+-increasing agonists. CaCC was shown to be preserved or enhanced in human and murine cystic fibrosis airways as a mechanism to compensate for the lack of CFTR (Clarke et al., 1994; Grubb et al., 1994). Since, CaCC-mediated Cl− secretion appears to be preserved in cystic fibrosis its pharmacological stimulation has been suggested as a therapeutic tool for bypassing dysfunctional CFTR. Mall et al. demonstrated that activation of the cAMP pathway increased CaCC-mediated secretion in cystic fibrosis and, conversely, chromanol 293B, an inhibitor of cAMP-activated Kv7.1 channels was able to fully abolish nucleotide-activated Cl− secretion (Mall et al., 2003). Hence, the authors proposed that activation of Kv7.1 channels, together with hSK4 K+ channels, may improve Cl− secretion via CaCC in human cystic fibrosis airway tissues (Mall et al., 2003). Altogether, these studies indicate the Kv7.1 channel activation may help to improve mucociliary clearance in cystic fibrosis airways.
Elevated levels of reactive oxygen species (ROS) lead to oxidative stress and DNA damage in several tissues providing a common pathological mechanism in all inflammatory lung diseases. Hydrogen peroxide (H2O2) has been proven to stimulate several components of the Cl− secretion in the airway epithelia, including the cAMP-dependent activation of CFTR and the basolateral K+ channels (Cowley and Linsdell, 2002b; Ivonnet et al., 2015). The basolateral K+ conductance was abrogated by clofilium, a Kv7.1 channel inhibitor, suggesting a role for these channels in the H2O2-stimulated response (Cowley and Linsdell, 2002b; Ivonnet et al., 2015). In line with this, H2O2 has been reported to potently enhance Kv7 currents (Gamper et al., 2006; Kim et al., 2016). Activation of transmembrane adenylyl cyclase by H2O2 has been proposed as a triggering mechanism for the cAMP-activated basolateral Kv7.1 current (Ivonnet et al., 2015). The stimulated anion secretion by ROS could act as a compensatory protective mechanism to keep the airways clear from damaging radicals and to hold acceptable airway surface liquid levels.
While the Kv7.1 channel is the member of the KCNQ family with the strongest evidence of its functional expression and physiological role in lung epithelial cells, others (KCNQ3 and KCNQ5) have been detected in the apical or lateral membranes (Moser et al., 2008). In addition, mRNA transcripts for several KCNE accessory subunits (KCNE2 and KCNE3) have been found in human airway Calu-3 cells (Cowley and Linsdell, 2002a). Kv7.1 channels expressed in Xenopus oocytes were shown to be activated by an increase in intracellular cAMP concentration, independently of co-expression with KCNE1 or KCNE3 ancillary subunits (Boucherot et al., 2001). On the other hand, Potet et al. demonstrated that recombinant Kv7.1/KCNE1 complexes expressed in Cos-7 cells were totally insensitive to cAMP (Potet et al., 2001). The co-assembly of Kv7.1 with KCNE3 subunits markedly affects Kv7.1 channel properties (abolishing its voltage dependence) and has been proposed to form the cAMP-activated basolateral K+ channels contributing to Cl− secretion in colonic epithelial cells (Schroeder et al., 2000). Interestingly, Preston et al. evidenced that cAMP-stimulated Cl− secretion across tracheal epithelia was severely reduced in KCNE3(−/−) mice (Preston et al., 2010). While the involvement of KCNE3 in airway secretion requires confirmation in future studies, the structural basis of the interaction of Kv7.1 with KCNE3 leading to a constitutive activation of Kv7.1 channel activity has been recently established (Kroncke et al., 2016; Sun and MacKinnon, 2020).
Asthma is characterized by hyper-contractility of bronchial smooth muscle attributed to an increased concentration of different bronchoconstrictor agonists, or enhanced sensitivity to them, leading to aberrant narrowing of the airways (Penn, 2008). Acetylcholine, leukotriene D4, endothelin, and histamine represent the most relevant locally released bronchoconstrictor agonists that activate GPCR on ASMC, leading to increased cytosolic Ca2+ concentration and contraction (Jude et al., 2008; Penn, 2008). This together with the strong inflammation leads to an acute hypersensitivity to different air stimulants that produce ASMC bronchospasm and that, in the long term, promote smooth muscle proliferation and hypertrophy (Stott et al., 2014). Kv7 channels are considered negative regulators of ASMC contraction due to their major contribution to maintain negative Em negative, preventing L-type-Ca2+ channel activation and the subsequent bronchoconstriction (Brueggemann et al., 2011). Several members of the Kv7 family are expressed in ASMCs (Brueggemann et al., 2011; Bartoszewski et al., 2017). The relative abundance expression of the Kv7 members appears to differ depending on the location (trachea vs. bronchioles) and depending on the animal species as mentioned above (Brueggemann et al., 2011, 2014b Evseev et al., 2013). Several studies have evidenced that bronchoconstrictor agonists negatively regulate the activity of Kv7 channels. Thus, methacholine, carbachol, or histamine reduce Kv7 currents in guinea pig, mouse, and rat ASMCs (Brueggemann et al., 2011, 2014; Haick et al., 2017). On the other hand, structurally unrelated Kv7 channel activators cause a concentration-dependent relaxation of small bronchioles (Brueggemann et al., 2014b) and tracheal rings (Evseev et al., 2013). Of note, in vivo administration of the Kv7 channel opener retigabine was proven to transiently reduce bronchoconstriction induced by methacholine in mice (Evseev et al., 2013).
Chronic administration of long-acting β2-adrenergic receptor agonist elicits a time‐ and concentration-dependent relaxation of rat airways, with remarkable desensitization to short-term or with repeated treatments. Co-administration of retigabine plus the long-acting β2-adrenergic receptor agonist formoterol was shown to cause a sustained reduction of methacholine-induced bronchoconstriction and reduced the desensitization observed with formoterol alone (Brueggemann et al., 2014b). These findings suggest that using a Kv7 channel enhancer together a β2-adrenergic receptor agonist may improve bronchodilator therapy.
In addition to exaggerated bronchoconstriction, asthma is characterized by a chronic inflammatory response in the airways that is targeted primarily with inhaled corticosteroids. As stated in the previous section basolateral membrane K+ channels play a key role in stimulating Cl− transport across AEPs (Frizzell and Hanrahan, 2012). Unlike in cystic fibrosis, a decrease in airway mucus secretion ameliorates the symptomatology of asthma. Very recently, Hynes and Harvey have demonstrated that dexamethasone produces an inhibition of transepithelial chloride ion secretion though a rapid non-genomic inhibition of Kv7.1 channels and KCNN4 (KCa3.1) (Hynes and Harvey, 2019). Thus, it is plausible that down-regulation of Kv7.1 channel activity might contribute to the potential benefit of corticosteroids to reverse airway hypersecretion.
COPD is a progressive life-threatening lung disease characterized by airway obstruction due to inflammation of the small airways. The disease evolves with irreversible limitation of airflow, loss of lung function and severe acute exacerbations which significantly deteriorate the patient’s quality of life (Barnes et al., 2003). β-adrenergic receptors (β-AR) agonists promote airway smooth muscle relaxation and are the drugs of choice for rescue from acute bronchoconstriction in asthma and COPD. A recent study has shown that the long-acting β-AR formoterol robustly enhanced Kv7 currents in human ASMCs (Brueggemann et al., 2018). Furthermore, these authors provided evidence that Kv7.5 is the predominant Kv7 α-subunit in human ASMCs and identified a PKA-dependent phosphorylation of S53 on the N-terminus of Kv7.5 channel subunits as a potential mechanism of activation following the stimulation of the βAR/cAMP pathway (Brueggemann et al., 2018).
The exposure to cigarette smoking is the principal cause of COPD (Salvi, 2014). Recently Sevilla-Montero et al. have analyzed the impact of cigarette smoke exposure on the pulmonary vasculature (Sevilla-Montero et al., 2019). Authors showed that cigarette smoke extract exposure had direct effects on human fibroblasts and PASMCs, promoting a senescent phenotype which contributed to the secretion of inflammatory molecules and increase the proliferation of non-exposed cells. Furthermore, cigarette smoke extract exposure affected cell contractility attenuated the pulmonary vascular responses to Kv7 channel modulators and dysregulated the expression and activity of Kv7.4 channels. The results suggest that cigarette smoke may impair pulmonary vascular tone atleast in part through Kv7.4 downregulation (Sevilla-Montero et al., 2019).
A sequence polymorphism of the human KCNE2 gene has been found to be associated with altered lung function (Soler-Artigas et al., 2011; Sabater-Lleal et al., 2014). The deletion of KCNE2 reduced pulmonary expression of the Kv7.1 α-subunit suggesting the formation of pulmonary KCNQ1-KCNE2 complexes (Zhou et al., 2019). KCNE2(-/-) mice had reduced blood O2, increased CO2, increased pulmonary apoptosis, and increased inflammatory mediators TNF-α, IL-6, and leukocytes in bronchoalveolar lavage fluids (Zhou et al., 2019). These data indicated KCNE2 regulates KCNQ1 in the lungs and is required for normal lung function.
Airway sensory afferent nerves are essential for triggering different reflex responses to potential injurious stimuli (Undem and Kollarik, 2005). Most vagal sensory afferent nerves which terminate in the respiratory tract are C-fibers (Undem and Kollarik, 2005). The excessive activity of VACF induces different symptoms associated with inflammatory lung diseases, such as nonproductive coughing, dyspnea, parasympathetic reflex mucus secretion and bronchoconstriction (Mazzone and Undem, 2016). Consequently, ion channels that regulate the excitability of C-fibers in the airways can be an attractive therapeutic target aimed at reducing the symptoms of various inflammatory diseases of the airways. Using single-cell mRNA analysis, Sun and collaborators have very recently shown that KCNQ2, KCNQ3, and KCNQ5 are consistently expressed in mouse lung specific nodose neurons with KCNQ3 being the most predominant (Sun et al., 2019). This study suggested that Kv7.3 channels are the major contributors to the Kv7-M current in the nodal neurons of the mouse lungs. The Kv7 channel activator retigabine increased the amplitude and accelerated the activation rate of the M-current, caused a prominent hyperpolarization, and suppressed the excitability of mouse nodose neurons. These effects were reversed or prevented in the presence of XE991, a Kv7 channel inhibitor. Remarkably, nebulized retigabine was found to attenuate cough evoked by inhalation of irritant gases (SO2 and NH3) in awake mice (Sun et al., 2019). Although future studies are needed to confirm their role in the regulation of C-fiber excitability of the airways in humans, these data indicate that Kv7.3 channels may provide a novel therapeutic target to reduce the conditions associated with pulmonary inflammatory diseases such as chronic coughing.
K+ channels regulate a number of processes of key relevance in cancer biology such as cell volume control, pH regulation, migration, proliferation, differentiation, apoptosis, and drug resistance (Rao et al., 2015; Prevarskaya et al., 2018; Haworth and Brackenbury, 2019; Serrano-Novillo et al., 2019). Thus, there is increasing evidence that K+ channels play an important role in cancer; but a clear scenario is still far due to the plethora of K+ channel isoforms and their diverse repertoire of effects. Notably, K+ channels regulate cell cycle, but according to the channel isoform, its level of expression, the cell type, and the stage of differentiation the effects are diverse. For instance, Kv channels may exhibit proliferative or anti-proliferative roles, depending on the channel subtype and the phase of the cell cycle (Rao et al., 2015). Moreover changes in the phenotypes, signaling pathways, and pattern of expression of K+ channels may be observed in cancer, which increases the complexity of K+ channels regulation on cell viability. In addition to the well-characterized involvement of several Kv channels (most notably Kv1.1, Kv1.3, Kv1.5, Kv10.1, Kv10.2, and Kv11.1) in cancer (Rao et al., 2015), some members of the Kv7 family have also been suggested to participate in cell proliferation and cancer (Serrano-Novillo et al., 2019).
K+ channels participate in tumor cell invasion and metastasis propagation via regulation of cell migration and growth (O’Grady and Lee, 2005; Schwab et al., 2008; Arcangeli et al., 2009; Prevarskaya et al., 2010). Indeed, inhibiting or silencing K+ channels reduces the proliferation of cancer cells (Pancrazio et al., 1993; Jang et al., 2011). Kv7.1 channels have been highlighted in cell migration, proliferation, and repair of AECs (Trinh et al., 2007). In 2014, Girault and colleagues showed an increased expression (by 1.5‐ to 7-fold) of Kv7.1 channels in tumor lung adenocarcinoma samples, compared to non-neoplastic tissues from 17 of 26 patients (Girault et al., 2014). Authors found that the proliferation rates of lung adenocarcinoma cell monolayers were reduced by the Kv7.1 channel blockers clofilium and chromanol, or after silencing Kv7.1 channels using a specific siRNA. These data suggest that Kv7.1 channels could be a potential therapeutic target in lung cancer (Girault et al., 2014).
On the other hand, K+ channels have also been implicated in both early and late stages of apoptosis. The decrease in cell volume is one of the earliest and necessary morphological changes observed in cells undergoing apoptosis. Active K+ channels in the plasma membrane leads to K+ and Cl− outflow through K+ and Cl− channels, this generates an osmotic gradient leading to an outward water transport through aquaporins which results in a decrease in cell volume (Maeno et al., 2000). In later stages of apoptosis, the augmented intracellular K+ concentration ([K+]int) suppresses caspase and nuclease activity and, conversely, the decreased [K+]int caused by K+ channel activation, promotes apoptosis (Burg et al., 2008). There is a wealth of literature supporting that the M‐ channel activators have a neuroprotective role via Kv7 activation and reduction of hyperactivity and, hence, suppression of glutamate excitotoxicity (Gamper et al., 2006; Bierbower et al., 2015; Vigil et al., 2020). It is likely that flupirtine would work the same way (Panchanathan et al., 2013; Li et al., 2014a). Nevertherless, its ability to inhibit cell viability has also been reported. Thus, Lee et al. showed that flupirtine exerted anti-proliferative effects in canine osteosarcoma cells by enhancing Kv7.5 function and arresting cells in the G0/G1 phases (Lee et al., 2014). Similarly, retigabine was found to exhibit anti-proliferative effects in C2C12 myoblasts (Iannotti et al., 2010).
Non-small-cell lung cancer (NSCLC) accounts for most cancer deaths. Aberrant activation of the epidermal growth factor receptor (EGFR) is involved in the pathogenesis and progression of several human cancers, including NSCLC (Melosky, 2014). Indeed, approximately 10–38% of NSCLC patients have EGFR gene mutations (Lin et al., 2014). EGFR tyrosine kinase inhibitors, such as Gefitinib have become standard first-line treatment for these patients (Cohen et al., 2003). However, acquired resistance represents a major limitation for drug efficacy. Cancer stem cells have self-renewal properties in various solid tumors, and play a key role in tumor growth and progression (Wang et al., 2013) and in therapy resistance (Steinbichler et al., 2018). A subset of cancer stem cells, termed the “side population” (SP), have elevated clonogenic potential and higher expression levels of ABC-transporters than main-population cells known as non-SP cells (Richard et al., 2013) and are considered to be responsible for anti-cancer drug resistance (Singh et al., 2010; Leon et al., 2016). Choi and colleagues have demonstrated a reduced mRNA expression of KCNQ3 and KCNQ5 in SP cells compared to non-SP cells (Choi et al., 2017). The treatment of flupirtine (a Kv7.2–7.5 channel enhancer) decreased the viability of SP and non-SP cells. In addition, the combination treatment of gefitinib plus flupirtine was more effective, compared to the individual treatment of both drugs, in decreasing the viability of both non-SP cells, and gefitinib-resistant SP cells. According to their results, the combination treatment of gefitinib plus flupirtine was proposed to reduce the viability of gefitinib-resistant cells through inhibition of the EGFR-Ras-Raf-ERK pathway via (Choi et al., 2017). These results suggest that the activation of Kv7.3 and Kv7.5 channels could play an important role in the induction of apoptosis in SP cells, either by participating in the decrease of the cellular volume necessary for apoptosis initiation; or by decreasing [K+]int, which results in the activation of caspases and nucleases.
Pulmonary hypertension is defined by an increase in mean pulmonary arterial pressure at rest, as assessed by right heart catheterization. Based on pathophysiological findings, clinical presentation, hemodynamic characteristics, and therapeutic considerations, pulmonary hypertension is categorized into five groups by WHO: pulmonary arterial hypertension (PAH), pulmonary hypertension due to left-sided heart disease, pulmonary hypertension due to lung disease or hypoxia, chronic thromboembolic pulmonary hypertension, and multifactorial pulmonary hypertension (Simonneau et al., 2019). PAH is characterized by excessive pulmonary vasoconstriction and progressive remodeling of the distal PAs, resulting in elevated pulmonary vascular resistance and, eventually, in right ventricular failure (McLaughlin et al., 2015). Reduced expression or function of K+ channels (notably Kv1.5 and TASK-1 channels) in PASMCs results in a more depolarized Em, and is considered to contribute to the enhanced vasoconstriction and proliferation (Moudgil et al., 2006; Boucherat et al., 2015; Hayabuchi, 2017). In 2009, Joshi et al. demonstrated that Kv7 channel blockers, linopirdine, and XE991, inhibited the IKN current in PASMCs and raised mean pulmonary arterial pressure (mPAP); while the Kv7 channel openers, retigabine and flupirtine, had the opposite effects (Joshi et al., 2009). Linopirdine can also increase mesenteric vascular resistance and systemic arterial pressure (Mackie et al., 2008). Functional expression of Kv7 channels in the pulmonary circulation was later confirmed by others (Morecroft et al., 2009; Eid and Gurney, 2018; Mondejar-Parreño et al., 2018). As in the systemic circulation, KCNQ1 and especially KCNQ4 and KCNQ5 appear the most predominant KCNQ channels in the pulmonary circulation (Joshi et al., 2009; Morales-Cano et al., 2014; Sedivy et al., 2015; Mondejar-Parreño et al., 2018). The lack of selective drugs for the different Kv7 subunits hinders identification of the functional impact of individual Kv7 members (Barrese et al., 2018). Structurally related Kv7 enhancers such as retigabine and flupirtine (which activate all Kv7 isoforms except Kv7.1 channels) (Barrese et al., 2018) and zinc pyrithione (which activate all Kv7 members but Kv7.3) have been shown to induce pulmonary vasodilation (Joshi et al., 2009; Morecroft et al., 2009; Sedivy et al., 2015; Eid and Gurney, 2018; Mondejar-Parreño et al., 2018). In addition, drugs that block all Kv7 channels (i.e., linopirdine and XE991) are able to contract PAs previously primed (Chadha et al., 2012; Sedivy et al., 2015) or not (Joshi et al., 2009), whereas selective Kv7.1 blockers HMR1556, L768, L673, and JNJ39490282 (Barrese et al., 2018) have no contractile effects in PAs (Chadha et al., 2012). On the other hand, the application of R-L3, a compound purported to activate Kv7.1 channels, relaxed efficiently precontracted intrapulmonary arteries (Chadha et al., 2012; Mondejar-Parreño et al., 2018). These data suggest that even when Kv7.4 and Kv7.5 appear to be the most relevant Kv7 channels in controlling resting Em in PASMC, Kv7.1 channels are also functionally expressed.
The NO/cGMP pathway represents a key physiological signaling controlling tone in PAs, and drugs stimulating this pathway are used to treat PAH (Barnes and Liu, 1995), and drugs activating this pathway are used to treat pulmonary arterial hypertension. Recently, we have found that NO donors and riociguat, a stimulator of sGC used for the treatment of pulmonary arterial hypertension, enhance Kv7 current and induce membrane hyperpolarization in fresh isolated PASMCs contributing to pulmonary vasodilation (Mondejar-Parreño et al., 2019). Further, we identified Kv7.5 channels as a potential member of the Kv7 family targeted by these drugs.
The expression of Kv7 channels has been explored in several experimental models of pulmonary hypertension. While the hypoxic pulmonary vasoconstriction (HPV) reflects a physiological intrinsic property of PASMCs, which allows shifting blood flow from hypoxic to normoxic lung areas, coupling ventilation and perfusion, the chronic exposure to hypoxic air causes the pulmonary circulation to become hypertensive (Sylvester et al., 2012). Sedivy et al. found a significant loss of KCNQ4 mRNA expression in PA from rats maintained for 3 days in a hypoxic environment, even when a significant reduction of the Kv7.4 protein expression could not be confirmed (Sedivy et al., 2015). On the other hand, the expression of KCNQ1 and KCNQ5 was unaffected. However, Li et al. reported that longer incubation on hypoxia (9 days) was associated with a downregulation of KCNQ5 in the PAs (Li et al., 2014b). These authors also demonstrated that microRNA-190 (miR-190), whose expression is increased following the hypoxic exposure, directly targeted KCNQ5. This led the authors to propose the increased in miR-190 as a mechanism involved in the KCNQ5 downregulation and enhanced vasoconstriction induced by hypoxia. In addition to the hypoxic pulmonary hypertension models, a slight reduction of KCNQ5 expression has also been observed in rat lungs from monocrotaline-induced pulmonary hypertension (Morales-Cano et al., 2014). On the other hand, we found the expression of KCNQ1, KCNQ4, and KCNQ5 mRNAs unaltered in lungs from Zucker rats, a model of type 2 diabetes, developing increased pulmonary arterial pressure, and right ventricular hypertrophy (Morales-Cano et al., 2019).
The potential impairment of KCNQ channels has been also explored in conditions associated with pulmonary hypertension. The HIV-1 infection is an established risk factor for PAH, although the pathological mechanisms underlying the development of HIV-related PAH remain unclear (Butrous, 2015). In a recent study using HIV-transgenic mice expressing seven of the nine HIV viral proteins and wild-type mice we observed that HIV mice had reduced lung expression of KCNQ1 and KCNQ5 channels (Mondejar-Parreño et al., 2018). By using vascular reactivity and patch-clamp experimental approaches we found decreased responses to the Kv7.1 channel activator L-364,373 but unaltered responses to retigabine (Mondejar-Parreño et al., 2018). These results suggest that Kv7 channels (especially Kv7.1) are impaired in mice expressing HIV-1 proteins.
Congenital diaphragmatic hernia is a rare congenital anomaly commonly associated with persistent pulmonary hypertension. A recent study showed that the expression of KCNQ5, but not that of KCNQ1 or KCNQ4, was significantly downregulated at the mRNA and protein levels in rat lungs from nitrofen-induced congenital diaphragmatic hernia (Zimmer et al., 2017). Likewise, a markedly diminished KCNQ5 expression was found in the pulmonary vasculature of congenital diaphragmatic hernia fetuses. Since Kv7.5 channels have shown to contribute to NO donors-induced pulmonary vasodilation (Mondejar-Parreño et al., 2019), its reduced availability in congenital diaphragmatic hernia could partly explain the poor therapeutic response to inhaled NO in these patients (Group (NINOS), 1997; Putnam et al., 2016).
Studies in experimental models have shown evidence that drugs activating Kv7 channels may be of benefit in the treatment of pulmonary hypertension with different etiologies (Morecroft et al., 2009; Sedivy et al., 2015). Morecroft et al. also demonstrated that flupirtine attenuated the development of chronic hypoxia-induced pulmonary hypertension in mice and reversed established pulmonary hypertension in mice that over-express the 5-HT transporter (SERT), apparently via Kv7 activation (Morecroft et al., 2009). In this study authors showed that the chronic treatment with flupirtine reduced mean right ventricle pressure (mRVP), pulmonary remodeling, and RV hypertrophy (RVH). More recently, Sedivy et al. confirmed the potential benefit of Kv7 channel openers showing the pulmonary antihypertensive effects of flupirtine in rats exposed to short-term (3–5 days) hypoxia (Sedivy et al., 2015). In line with this, we have found that retigabine-induced vasodilation is increased in PAs from pulmonary hypertensive animals and this is associated with an increased contribution of Kv7 channels to the net K+ current present in pulmonary arterial myocytes (unpublished data from the author’s laboratory).
Altogether, these findings suggest that Kv7 channels play a protective role in the pulmonary circulation limiting pulmonary vasoconstriction, and their activation may represent a promising therapeutic strategy in pulmonary hypertension.
In addition to their well-established role in neural and cardiac tissue, studies over the last 20 decades have evidenced the expression and the plethora of cellular functions of Kv7 channels in numerous cell types. Thus, Kv7 channels are now recognized as playing relevant physiological roles in many tissues, which has encouraged the search for the therapeutic potential of Kv7 channel modulators in many diseases including those affected the lung. As stated in the previous section activation of Kv7 channels has been proposed to provide beneficial effects in a number of lung conditions. Therefore, Kv7 channel openers/enhancers or drugs acting partly through these channels could be proposed as bronchodilators, expectorants, antitussives, chemotherapeutics, and pulmonary vasodilators, as summarized below.
Flupirtine and retigabine are structurally related Kv7.2–7.5 channel activators. The discovery of Kv7 channels came later than the extensive use of flupirtine and retigabine as an analgesic and anticonvulsant, respectively, and whose mechanism of action was unknown until then (Schenzer et al., 2005). Retigabine has been shown to increase the open probability and cause a hyperpolarizing shift in the voltage dependence of activation of KCNQ channels by binding at the intracellular side of the pore-forming S5 helix around a key tryptophan (Trp) Trp236 in Kv7.2 and analogous residues in Kv7.3, 4, and 5 which is absent in Kv7.1 channels (Schenzer et al., 2005; Lange et al., 2009; Kim et al., 2015). Kim et al. have suggested that the retigabine interaction with this Trp residue depends largely on the formation of a hydrogen bond (Kim et al., 2015).
As the relevant role of Kv7 channels was discovered, the idea that these channels could constitute a new therapeutic strategy became more attractive, leading to investment in the development of new activators. The key residues for retigabine-binding have also been recognized to be also essential for the binding of other compounds such as S1 and BMS-204352; though these last drugs have a greater efficacy enhancing Kv7.4 and Kv7.5 isoforms rather than Kv7.2 or/and Kv7.3 channels (Dupuis et al., 2002; Bentzen et al., 2006). Zinc pyrithione activates the Kv7 channel by interacting with a binding site different to that of retigabine (Xiong et al., 2008). Hence, zinc pyrithione activates all Kv7 channels except for Kv7.3 channels, which is the most retigabine-sensitive. It is worth noting that the active compound in the action of zinc pyrithione on Kv7 channels is zinc, as administration of free zinc also activates these channels by reducing their dependence on PIP2 (Gao et al., 2017). The activity of ICA-27243, a drug more potent at activating Kv7.2/7.3 heteromeric channels than Kv7.4 homomeric channels or Kv7.3/7.5 heteromeric channels, is determined by a novel site inside the Kv7 channel voltage-detection domain (Wickenden et al., 2008; Blom et al., 2010). R-L3 is a benzodiazepine that activates Kv7.1 homomeric channels, but is considerably weaker when Kv7.1 is coexpressed with KCNE1 subunit, suggesting that R-L3 and KCNE1 subunits compete for the same interaction site on KCNQ1 subunits (Salata et al., 1998).
Kv7 channels are currently new therapeutic targets in the field of lung diseases; consequently, there is still much to be learned about their physiological role, regulation, and possible impairment by pathological processes. The specific expression of certain Kv7 channel in different types of cells is of great pharmacological interest, since their selective modulation could provide beneficial therapeutic effects while avoiding the adverse effects associated with other isoforms (Stott et al., 2014). A clear example comes from the previous experience with retigabine and flupirtine, which have been used as analgesic and anticonvulsant, respectively, for many years but were withdrawn from the marked due to their adverse effects including the risk of serious liver injury, pigment changes in the retina and urinary retention (Herrmann et al., 1987; Brickel et al., 2012). While, as mentioned, both drugs activate Kv7.2–Kv7.5, they appear to display greater efficacy for Kv7.2 and Kv7.3 channels over Kv7.4 and Kv7.5 channels, which predominate in smooth muscle. Thus, it is expected that compounds selectively activate the Kv7.4 and/or the Kv7.5 subunit may be used in the treatment of vascular smooth muscle disorders with few neurological side effects. For instance, selective activation of Kv7.4 and Kv7.4/Kv7.5 channels (but not Kv7.1, Kv7.2, and Kv7.2/Kv7.3) has been implicated in the vasorelaxant effects of the Rho kinase inhibitor fasudil (Zhang et al., 2016). Thus, it is likely that, in addition to Rho kinase inhibition, activation of these channels may contribute to the beneficial effects of fasudil in cardiovascular conditions such as hypertension, vasospasm, stroke arteriosclerosis, pulmonary hypertension, and heart failure (Shi and Wei, 2013; Shimokawa and Satoh, 2015). Likewise, it is expected that selective Kv7.2 and Kv7.3 channel enhancers may be used to the treatment of neurological disorders with few adverse effects in smooth muscle cells (Stott et al., 2014; Barrese et al., 2018). Remarkably, Manville et al. have recently reported that the neurotransmitter γ-aminobutyric acid (GABA) and various metabolites (β-hydroxybutyric acid and γ-amino-β-hydroxybutyric acid) directly activate Kv7.3 and Kv7.5 but not Kv7.1, 2, and 4 (Manville et al., 2018). Manville and colleagues found that Trp residues in Kv7.3 (Trp265) and Kv7.5 (Trp270) were required for binding but not sufficient for GABA-induced activation. Thus, while Kv7.2 and Kv7.4 share analogous Trp residues and bind GABA, they are not activated by GABA (Manville and Abbott, 2018). Likewise, the synthetic GABA analog gabapentin, but not pregabalin, was also found to activate Kv7.3 and Kv7.5 (Manville and Abbott, 2018). Very recently, these authors have proposed a model in which the sodium-coupled myo-inositol transporter 1 (SMIT1) interacts with KCNQ2/3 and tunes the channel response to GABA and related metabolites (Manville and Abbott, 2020).
The search for Kv7 activators with a better safety profile than retigabine and flupirtine as well as the identification of novel potential therapeutic applications, has driven the development of a large number of compounds with Kv7 enhancing properties in the last decade (Jepps et al., 2013; Stott et al., 2014; Haick and Byron, 2016; Barrese et al., 2018).
Given the functional roles and ubiquitous expression of Kv7 channels in many tissues, drugs modulating Kv7 channels are presumed to cause off-target effects including alterations of the QT interval at the heart level, systemic hypotension, urinary retention or neuronal effects. This could be largely avoided by using localized drug delivery to the lungs by oral inhalation. Inhaled administration is the route of choice for many drugs (i.e., corticosteroids; anticholinegics, or β2-adrenergic receptor agonists) to treat respiratory diseases. Similarly, targeting lung Kv7 channels specifically using inhaled Kv7 activators may be an innovative approach for the treatment of COPD, cystic fibrosis, pulmonary hypertension, asthmatic events, lung cancer, bronchitis, and pulmonary edema.
Progress in the treatment of respiratory diseases is a global challenge because of its health and socio-economic implications. The optimal management and treatment of these diseases should be aimed at achieving adequate control of the disease, improving the quality of life of patients, and reducing hospitalizations and the enormous financial burden on healthcare systems. Targeting Kv7 channels in the treatment of lung disorders could be a promising therapeutic strategy. Here, we summarized the potential usefulness of Kv channel modulators in treating respiratory diseases.
Asthma and COPD are characterized by hyper-contraction of ASMC attributed to abnormally high concentrations of different bronchoconstrictor agonists and/or increased sensitivity to them, leading to pathological narrowing of the airways (Penn, 2008). K+ channels are key regulators of Em in ASMC and their impairment causes membrane depolarization and bronchoconstriction (Hall, 2000). Among them, Kv7 channels contribute significantly to the negative resting Em in ASMC (Brueggemann et al., 2011). By acting on a different target, Kv7 channel openers offer the potential of being combined with standard bronchodilators such as β2-adrenergic receptor agonists and antimuscarinics as an adjuvant therapy to increase the efficacy or overcome desensitization (Brueggemann et al., 2014b).
The function of Kv7.1 together with KCNE1/E2/E3 subunits seems to play a key role in secretions in airway epithelial cells. The co-activation of Cl− channels in the luminal surface and K+ channels in the basolateral membrane in AEC is an essential requirement for Cl− secretion (Frizzell and Hanrahan, 2012). The activation of Kv7.1 channels has an expectorant effect provoking or promoting the expulsion of accumulated bronchial secretions and the productive cough helping to airway clearance (Mall et al., 2000; Al-Hazza et al., 2016; Kroncke et al., 2016; Hynes and Harvey, 2019). Kv7.1 channel openers or drugs that indirectly activate Kv7.1 channels might be beneficial in the treatment of bronchitis, COPD, cystic fibrosis and asthma (Boucherot et al., 2001; Athanazio, 2012; Hynes and Harvey, 2019).
Stimulation of airway C-fibers can be activated by inflammatory factors leading to the impulse to unproductive cough, dyspnea, mucus secretion and bronchoconstriction (Mazzone and Undem, 2016). Activation of Kv7.3 channels in VACF by retigabine has been shown to increase the M-current amplitude and to inhibit unproductive coughing (Sun et al., 2019). Thus, Kv7 activators could be useful as chronic coughing relievers in pulmonary inflammatory diseases.
Although, the role of Kv7 channel modulators as chemotherapy agents has been only barely studied, flupirtine has been shown to attenuate the resistance to gefitinib in a human lung cancer cell line (Choi et al., 2017). The anti-proliferative effect of flupirtine has also been proposed as potentially beneficial in other types of cancer (Lee et al., 2014). Additional studies are required to evaluate the potential of Kv7 channel activators with selectivity for particular isoforms as chemotherapy agents.
K+ channels are also key regulators of cellular Em in PASMCs, controlling the activity of voltage-dependent Ca2+ channels and, subsequently, pulmonary artery smooth muscle contraction. Pulmonary vascular tone is controlled by the interaction of different circulating or locally released vasoactive factors with vasoconstrictor or vasodilating properties. Acute modulation of the activity of K+ channels has been extensively shown to contribute to the vascular effects of these vasoactive factors. Thus, different vasodilators such as nitric oxide, prostacyclin and calcitonin gene-related activate several K+ channels and, conversely, vasoconstrictors as serotonin, angiotensin II, thromboxane A2, noradrenaline and endothelin-1 have been shown to inhibit K+ channels (Cogolludo et al., 2007; Stott et al., 2014). With such a crucial role in modulating and maintaining pulmonary arterial tone, changes in function and expression of K+ channels during the development and pathogenesis of pulmonary hypertension results in aberrations of normal physiological functions. Consequently, K+ channels openers are presumed to have a beneficial therapeutic effect, especially if their action can be directed to the pulmonary circulation (Gurney et al., 2010). Up to the present time, the availability of drugs modulating Kv7 channels has been relatively scarce. Advances in the understanding of the role of Kv7 channels in the pulmonary vasculature and the development of new selective drugs for these channels indicate that they may represent a useful therapeutic target for the treatment of pulmonary arterial hypertension. As detailed above, different studies have provided evidence that treatment with Kv7 channel activators such as flupirtine, retigabine, or fasudil is beneficial in different pulmonary hypertension models (Morecroft et al., 2009; Duong-Quy et al., 2013; Sedivy et al., 2015; Zhang and Wu, 2017). In addition, Kv7 channel activation has been shown to contribute to the pulmonary vasodilating effect induced by drugs approved for the pulmonary hypertension treatment such as nitric oxide or riociguat (Mondejar-Parreño et al., 2019).
In the last decade, a significant progress in the understanding of the role of Kv7 channels in lung physiology has been made. The recognition of the involvement of these channels in cellular functions of crucial relevance in the normal physiology of the lung, such as the control of water and salt transport across the epithelial cell membrane, the excitability of airway afferent nerves, and the regulation of airway and lung vascular smooth muscle tone, makes them attractive therapeutic targets in lung diseases. In this regard, deciphering the involvement and importance of specific Kv7 subunits in these cellular functions will lead to a significant advance that may constitute the basis for the therapeutic potential of selective Kv7 channel modulators in lung diseases.
GM-P, FP-V, and AC wrote the manuscript. All authors contributed to the article and approved the submitted version.
This work was funded by Ministerio de Economía y Competitividad grants (SAF2016-77222R to FP-V and AC), the Cardiovascular Medical Research and Education Fund (AC), Comunidad de Madrid (B2017/BMD-3727 to AC) and Ciber Enfermedades Respiratorias (Ciberes). GM-P is supported by a predoctoral CIBERES/Fundación Contra la Hipertensión Pulmonar-EMPATHY grant.
The authors declare that the research was conducted in the absence of any commercial or financial relationships that could be construed as a potential conflict of interest.
Abbott, G. W. (2016). KCNE4 and KCNE5: K(+) channel regulation and cardiac arrhythmogenesis. Gene 593, 249–260. doi: 10.1016/j.gene.2016.07.069
Al-Hazza, A., Linley, J., Aziz, Q., Hunter, M., and Sandle, G. (2016). Upregulation of basolateral small conductance potassium channels (KCNQ1/KCNE3) in ulcerative colitis. Biochem. Biophys. Res. Commun. 470, 473–478. doi: 10.1016/j.bbrc.2015.12.086
Arcangeli, A., Crociani, O., Lastraioli, E., Masi, A., Pillozzi, S., and Becchetti, A. (2009). Targeting ion channels in cancer: a novel frontier in antineoplastic therapy. Curr. Med. Chem. 16, 66–93. doi: 10.2174/092986709787002835
Athanazio, R. (2012). Airway disease: similarities and differences between asthma, COPD and bronchiectasis. Clinics 67, 1335–1343. doi: 10.6061/clinics/2012(11)19
Bardou, O., Trinh, N. T. N., and Brochiero, E. (2009). Molecular diversity and function of K+ channels in airway and alveolar epithelial cells. Am. J. Phys. Lung Cell. Mol. Phys. 296, L145–L155. doi: 10.1152/ajplung.90525.2008
Barnes, P. J., and Liu, S. F. (1995). Regulation of pulmonary vascular tone. Pharmacol. Rev. 47, 87–131.
Barnes, P. J., Shapiro, S. D., and Pauwels, R. A. (2003). Chronic obstructive pulmonary disease: molecular and cellularmechanisms. Eur. Respir. J. 22, 672–688. doi: 10.1183/09031936.03.00040703
Barrese, V., Stott, J. B., and Greenwood, I. A. (2018). KCNQ-encoded potassium channels as therapeutic targets. Annu. Rev. Pharmacol. Toxicol. 58, 625–648. doi: 10.1146/annurev-pharmtox-010617-052912
Barro-Soria, R., Perez, M. E., and Larsson, H. P. (2015). KCNE3 acts by promoting voltage sensor activation in KCNQ1. Proc. Natl. Acad. Sci. 112, E7286–E7292. doi: 10.1073/pnas.1516238112
Bartoszewski, R., Matalon, S., and Collawn, J. F. (2017). Ion channels of the lung and their role in disease pathogenesis. Am. J. Phys. Lung Cell. Mol. Phys. 313, L859–L872. doi: 10.1152/ajplung.00285.2017
Belfodil, R., Barrière, H., Rubera, I., Tauc, M., Poujeol, C., Bidet, M., et al. (2003). CFTR-dependent and -independent swelling-activated K+ currents in primary cultures of mouse nephron. Am. J. Physiol. Ren. Physiol. 284, F812–F828. doi: 10.1152/ajprenal.00238.2002
Bentzen, B. H., Schmitt, N., Calloe, K., Dalby Brown, W., Grunnet, M., and Olesen, S.-P. (2006). The acrylamide (S)-1 differentially affects Kv7 (KCNQ) potassium channels. Neuropharmacology 51, 1068–1077. doi: 10.1016/j.neuropharm.2006.07.001
Bierbower, S. M., Choveau, F. S., Lechleiter, J. D., and Shapiro, M. S. (2015). Augmentation of M-type (KCNQ) potassium channels as a novel strategy to reduce stroke-induced brain injury. J. Neurosci. 35, 2101–2111. doi: 10.1523/JNEUROSCI.3805-14.2015
Billet, A., and Hanrahan, J. W. (2013). The secret life of CFTR as a calcium-activated chloride channel. J. Physiol. 591, 5273–5278. doi: 10.1113/jphysiol.2013.261909
Blom, S. M., Schmitt, N., and Jensen, H. S. (2010). Differential effects of ICA-27243 on cloned K(V)7 channels. Pharmacology 86, 174–181. doi: 10.1159/000317525
Boucherat, O., Chabot, S., Antigny, F., Perros, F., Provencher, S., and Bonnet, S. (2015). Potassium channels in pulmonary arterial hypertension. Eur. Respir. J. 46, 1167–1177. doi: 10.1183/13993003.00798-2015
Boucherot, A., Schreiber, R., and Kunzelmann, K. (2001). Regulation and properties of KCNQ1 (K(V)LQT1) and impact of the cystic fibrosis transmembrane conductance regulator. J. Membr. Biol. 182, 39–47. doi: 10.1007/s00232-001-0030-4
Brickel, N., Gandhi, P., VanLandingham, K., Hammond, J., and DeRossett, S. (2012). The urinary safety profile and secondary renal effects of retigabine (ezogabine): a first-in-class antiepileptic drug that targets KCNQ (K(v)7) potassium channels. Epilepsia 53, 606–612. doi: 10.1111/j.1528-1167.2012.03441.x
Brueggemann, L. I., Cribbs, L. L., Schwartz, J., Wang, M., Kouta, A., and Byron, K. L. (2018). Mechanisms of PKA-dependent potentiation of Kv7.5 channel activity in human airway smooth muscle cells. Int. J. Mol. Sci. 19:2223. doi: 10.3390/ijms19082223
Brueggemann, L. I., Haick, J. M., Cribbs, L. L., and Byron, K. L. (2014a). Differential activation of vascular smooth muscle Kv7.4, Kv7.5, and Kv7.4/7.5 channels by ML213 and ICA-069673. Mol. Pharmacol. 86, 330–341. doi: 10.1124/mol.114.093799
Brueggemann, L. I., Haick, J. M., Neuburg, S., Tate, S., Randhawa, D., Cribbs, L. L., et al. (2014b). KCNQ (Kv7) potassium channel activators as bronchodilators: combination with a β2-adrenergic agonist enhances relaxation of rat airways. Am. J. Phys. Lung Cell. Mol. Phys. 306, L476–L486. doi: 10.1152/ajplung.00253.2013
Brueggemann, L. I., Kakad, P. P., Love, R. B., Solway, J., Dowell, M. L., Cribbs, L. L., et al. (2011). Kv7 potassium channels in airway smooth muscle cells: signal transduction intermediates and pharmacological targets for bronchodilator therapy. Am. J. Phys. Lung Cell. Mol. Phys. 302, L120–L132. doi: 10.1152/ajplung.00194.2011
Burg, E. D., Remillard, C. V., and Yuan, J. X.-J. (2008). Potassium channels in the regulation of pulmonary artery smooth muscle cell proliferation and apoptosis: pharmacotherapeutic implications. Br. J. Pharmacol. 153, S99–S111. doi: 10.1038/sj.bjp.0707635
Butrous, G. (2015). Human immunodeficiency virus-associated pulmonary arterial hypertension: considerations for pulmonary vascular diseases in the developing world. Circulation 131, 1361–1370. doi: 10.1161/CIRCULATIONAHA.114.006978
Byron, K. L., and Brueggemann, L. I. (2018). Kv7 potassium channels as signal transduction intermediates in the control of microvascular tone. Microcirculation 25:e12419. doi: 10.1111/micc.12419
Caulfield, M. P., Jones, S., Vallis, Y., Buckley, N. J., Kim, G. D., Milligan, G., et al. (1994). Muscarinic M-current inhibition via G alpha q/11 and alpha-adrenoceptor inhibition of Ca2+ current via G alpha o in rat sympathetic neurones. J. Physiol. 477, 415–422. doi: 10.1113/jphysiol.1994.sp020203
Chadha, P. S., Jepps, T. A., Carr, G., Stott, J. B., Zhu, H.-L., Cole, W. C., et al. (2014). Contribution of kv7.4/kv7.5 heteromers to intrinsic and calcitonin gene-related peptide-induced cerebral reactivity. Arterioscler. Thromb. Vasc. Biol. 34, 887–893. doi: 10.1161/ATVBAHA.114.303405
Chadha, P. S., Zunke, F., Davis, A. J., Jepps, T. A., Linders, J. T. M., Schwake, M., et al. (2012). Pharmacological dissection of K(v)7.1 channels in systemic and pulmonary arteries. Br. J. Pharmacol. 166, 1377–1387. doi: 10.1111/j.1476-5381.2012.01863.x
Chen, Y.-H., Xu, S.-J., Bendahhou, S., Wang, X.-L., Wang, Y., Xu, W.-Y., et al. (2003). KCNQ1 gain-of-function mutation in familial atrial fibrillation. Science 299, 251–254. doi: 10.1126/science.1077771
Choi, S. Y., Kim, H.-R., Ryu, P. D., and Lee, S. Y. (2017). Regulation of voltage-gated potassium channels attenuates resistance of side-population cells to gefitinib in the human lung cancer cell line NCI-H460. BMC Pharmacol. Toxicol. 18:14. doi: 10.1186/s40360-017-0118-9
Clarke, L. L., Grubb, B. R., Yankaskas, J. R., Cotton, C. U., McKenzie, A., and Boucher, R. C. (1994). Relationship of a non-cystic fibrosis transmembrane conductance regulator-mediated chloride conductance to organ-level disease in Cftr(−/−) mice. Proc. Natl. Acad. Sci. 91, 479–483. doi: 10.1073/pnas.91.2.479
Cogolludo, A., Moreno, L., and Villamor, E. (2007). Mechanisms controlling vascular tone in pulmonary arterial hypertension: implications for vasodilator therapy. Pharmacology 79, 65–75. doi: 10.1159/000097754
Cohen, M. H., Williams, G. A., Sridhara, R., Chen, G., and Pazdur, R. (2003). FDA drug approval summary: gefitinib (ZD1839) (Iressa) tablets. Oncologist 8, 303–306. doi: 10.1634/theoncologist.8-4-303
Corvol, H., Flamein, F., Epaud, R., Clement, A., and Guillot, L. (2009). Lung alveolar epithelium and interstitial lung disease. Int. J. Biochem. Cell Biol. 41, 1643–1651. doi: 10.1016/j.biocel.2009.02.009
Cowley, E. A., and Linsdell, P. (2002a). Characterization of basolateral K+ channels underlying anion secretion in the human airway cell line Calu-3. J. Physiol. 538, 747–757. doi: 10.1113/jphysiol.2001.013300
Cowley, E. A., and Linsdell, P. (2002b). Oxidant stress stimulates anion secretion from the human airway epithelial cell line Calu-3: implications for cystic fibrosis lung disease. J. Physiol. 543, 201–209. doi: 10.1113/jphysiol.2002.022400
Cummings, K. W., and Bhalla, S. (2015). Pulmonary vascular diseases. Clin. Chest Med. 36, 235–248. doi: 10.1016/j.ccm.2015.02.007
Cunningham, S. A., Worrell, R. T., Benos, D. J., and Frizzell, R. A. (1992). CAMP-stimulated ion currents in Xenopus oocytes expressing CFTR cRNA. Am. J. Phys. 262, C783–C788. doi: 10.1152/ajpcell.1992.262.3.C783
de Jong, I. E. M., and Jepps, T. A. (2018). Impaired Kv7 channel function in cerebral arteries of a tauopathy mouse model (rTg4510). Phys. Rep. 6:e13920. doi: 10.14814/phy2.13920
Dellaripa, P. F. (2018). Interstitial lung disease in the connective tissue diseases; a paradigm shift in diagnosis and treatment. Clin. Immunol. 186, 71–73. doi: 10.1016/j.clim.2017.09.015
Delmas, P., and Brown, D. A. (2005). Pathways modulating neural KCNQ/M (Kv7) potassium channels. Nat. Rev. Neurosci. 6, 850–862. doi: 10.1038/nrn1785
Duong-Quy, S., Bei, Y., Liu, Z., and Dinh-Xuan, A. T. (2013). Role of rho-kinase and its inhibitors in pulmonary hypertension. Pharmacol. Ther. 137, 352–364. doi: 10.1016/j.pharmthera.2012.12.003
Dupuis, D. S., Schrøder, R. L., Jespersen, T., Christensen, J. K., Christophersen, P., Jensen, B. S., et al. (2002). Activation of KCNQ5 channels stably expressed in HEK293 cells by BMS-204352. Eur. J. Pharmacol. 437, 129–137. doi: 10.1016/S0014-2999(02)01287-6
Eid, B. G., and Gurney, A. M. (2018). Zinc pyrithione activates K+ channels and hyperpolarizes the membrane of rat pulmonary artery smooth muscle cells. PLoS One 13:e0192699. doi: 10.1371/journal.pone.0192699
Evseev, A. I., Semenov, I., Archer, C. R., Medina, J. L., Dube, P. H., Shapiro, M. S., et al. (2013). Functional effects of KCNQ K(+) channels in airway smooth muscle. Front. Physiol. 4:277. doi: 10.3389/fphys.2013.00277
Fosmo, A. L., and Skraastad, Ø. B. (2017). The Kv7 channel and cardiovascular risk factors. Front. Cardiovasc. Med. 4:75. doi: 10.3389/fcvm.2017.00075
Frizzell, R. A., and Hanrahan, J. W. (2012). Physiology of epithelial chloride and fluid secretion. Cold Spring Harb. Perspect. Med. 2:a009563. doi: 10.1101/cshperspect.a009563
Gamper, N., Zaika, O., Li, Y., Martin, P., Hernandez, C. C., Perez, M. R., et al. (2006). Oxidative modification of M-type K(+) channels as a mechanism of cytoprotective neuronal silencing. EMBO J. 25, 4996–5004. doi: 10.1038/sj.emboj.7601374
Gao, H., Boillat, A., Huang, D., Liang, C., Peers, C., and Gamper, N. (2017). Intracellular zinc activates KCNQ channels by reducing their dependence on phosphatidylinositol 4,5-bisphosphate. Proc. Natl. Acad. Sci. U. S. A. 114, E6410–E6419. doi: 10.1073/pnas.1620598114
Girault, A., Privé, A., Trinh, N. T. N., Bardou, O., Ferraro, P., Joubert, P., et al. (2014). Identification of KvLQT1 K+ channels as new regulators of non-small cell lung cancer cell proliferation and migration. Int. J. Oncol. 44, 838–848. doi: 10.3892/ijo.2013.2228
Goodwill, A. G., Fu, L., Noblet, J. N., Casalini, E. D., Sassoon, D., Berwick, Z. C., et al. (2016). KV7 channels contribute to paracrine, but not metabolic or ischemic, regulation of coronary vascular reactivity in swine. Am. J. Physiol. Heart Circ. Physiol. 310, H693–H704. doi: 10.1152/ajpheart.00688.2015
Group (NINOS) (1997). Inhaled nitric oxide and hypoxic respiratory failure in infants with congenital diaphragmatic hernia. Pediatrics 99, 838–845. doi: 10.1542/peds.99.6.838
Grubb, B. R., Vick, R. N., and Boucher, R. C. (1994). Hyperabsorption of Na+ and raised Ca(2+)-mediated Cl− secretion in nasal epithelia of CF mice. Am. J. Phys. Cell Phys. 266, C1478–C1483. doi: 10.1152/ajpcell.1994.266.5.C1478
Grunnet, M., Jespersen, T., Rasmussen, H. B., Ljungstrøm, T., Jorgensen, N. K., Olesen, S.-P., et al. (2002). KCNE4 is an inhibitory subunit to the KCNQ1 channel. J. Physiol. 542, 119–130. doi: 10.1113/jphysiol.2002.017301
Gurney, A. M., Joshi, S., and Manoury, B. (2010). KCNQ potassium channels: new targets for pulmonary vasodilator drugs? Adv. Exp. Med. Biol. 661, 405–417. doi: 10.1007/978-1-60761-500-2_26
Haick, J. M., Brueggemann, L. I., Cribbs, L. L., Denning, M. F., Schwartz, J., and Byron, K. L. (2017). PKC-dependent regulation of Kv7.5 channels by the bronchoconstrictor histamine in human airway smooth muscle cells. Am. J. Phys. Lung Cell. Mol. Phys. 312, L822–L834. doi: 10.1152/ajplung.00567.2016
Haick, J. M., and Byron, K. L. (2016). Novel treatment strategies for smooth muscle disorders: targeting Kv7 potassium channels. Pharmacol. Ther. 165, 14–25. doi: 10.1016/j.pharmthera.2016.05.002
Haley, J. E., Abogadie, F. C., Delmas, P., Dayrell, M., Vallis, Y., Milligan, G., et al. (1998). The alpha subunit of Gq contributes to muscarinic inhibition of the M-type potassium current in sympathetic neurons. J. Neurosci. 18, 4521–4531. doi: 10.1523/JNEUROSCI.18-12-04521.1998
Hall, I. P. (2000). Second messengers, ion channels and pharmacology of airway smooth muscle. Eur. Respir. J. 15, 1120–1127. doi: 10.1034/j.1399-3003.2000.01523.x
Halpin, D. M. G., Faner, R., Sibila, O., Badia, J. R., and Agusti, A. (2020). Do chronic respiratory diseases or their treatment affect the risk of SARS-CoV-2 infection? Lancet Respir. Med. 5, 436–438. doi: 10.1016/S2213-2600(20)30167-3
Haworth, A. S., and Brackenbury, W. J. (2019). Emerging roles for multifunctional ion channel auxiliary subunits in cancer. Cell Calcium 80, 125–140. doi: 10.1016/j.ceca.2019.04.005
Hayabuchi, Y. (2017). The action of smooth muscle cell potassium channels in the pathology of pulmonary arterial hypertension. Pediatr. Cardiol. 38, 1–14. doi: 10.1007/s00246-016-1491-7
Herrmann, W. M., Kern, U., and Aigner, M. (1987). On the adverse reactions and efficacy of long-term treatment with flupirtine: preliminary results of an ongoing twelve-month study with 200 patients suffering from chronic pain states in arthrosis or arthritis. Postgrad. Med. J. 63, 87–103.
Higashida, H., Hoshi, N., Zhang, J.-S., Yokoyama, S., Hashii, M., Jin, D., et al. (2005). Protein kinase C bound with A-kinase anchoring protein is involved in muscarinic receptor-activated modulation of M-type KCNQ potassium channels. Neurosci. Res. 51, 231–234. doi: 10.1016/j.neures.2004.11.009
Hu, B., Zeng, W.-P., Li, X., Al-Sheikh, U., Chen, S.-Y., and Ding, J. (2019). A conserved arginine/lysine-based motif promotes ER export of KCNE1 and KCNE2 to regulate KCNQ1 channel activity. Channels 13, 483–497. doi: 10.1080/19336950.2019.1685626
Hynes, D., and Harvey, B. J. (2019). Dexamethasone reduces airway epithelial Cl− secretion by rapid non-genomic inhibition of KCNQ1, KCNN4 and KATP K+ channels. Steroids 151:108459. doi: 10.1016/j.steroids.2019.108459
Iannotti, F. A., Panza, E., Barrese, V., Viggiano, D., Soldovieri, M. V., and Taglialatela, M. (2010). Expression, localization, and pharmacological role of Kv7 potassium channels in skeletal muscle proliferation, differentiation, and survival after myotoxic insults. J. Pharmacol. Exp. Ther. 332, 811–820. doi: 10.1124/jpet.109.162800
Ivonnet, P., Salathe, M., and Conner, G. E. (2015). Hydrogen peroxide stimulation of CFTR reveals an Epac-mediated, soluble AC-dependent cAMP amplification pathway common to GPCR signalling. Br. J. Pharmacol. 172, 173–184. doi: 10.1111/bph.12934
Jang, S. H., Choi, S. Y., Ryu, P. D., and Lee, S. Y. (2011). Anti-proliferative effect of Kv1.3 blockers in A549 human lung adenocarcinoma in vitro and in vivo. Eur. J. Pharmacol. 651, 26–32. doi: 10.1016/j.ejphar.2010.10.066
Jentsch, T. J. (2000). Neuronal KCNQ potassium channels: physiology and role in disease. Nat. Rev. Neurosci. 1, 21–30. doi: 10.1038/35036198
Jepps, T. A., Carr, G., Lundegaard, P. R., Olesen, S.-P., and Greenwood, I. A. (2015). Fundamental role for the KCNE4 ancillary subunit in Kv7.4 regulation of arterial tone. J. Physiol. 593, 5325–5340. doi: 10.1113/JP271286
Jepps, T. A., Olesen, S. P., and Greenwood, I. A. (2013). One man’s side effect is another man’s therapeutic opportunity: targeting Kv7 channels in smooth muscle disorders. Br. J. Pharmacol. 168, 19–27. doi: 10.1111/j.1476-5381.2012.02133.x
Joshi, S., Sedivy, V., Hodyc, D., Herget, J., and Gurney, A. M. (2009). KCNQ modulators reveal a key role for KCNQ potassium channels in regulating the tone of rat pulmonary artery smooth muscle. J. Pharmacol. Exp. Ther. 329, 368–376. doi: 10.1124/jpet.108.147785
Jude, J. A., Wylam, M. E., Walseth, T. F., and Kannan, M. S. (2008). Calcium signaling in airway smooth muscle. Proc. Am. Thorac. Soc. 5, 15–22. doi: 10.1513/pats.200704-047VS
Jung, J., Lin, H., Koh, Y. I., Ryu, K., Lee, J. S., Rim, J. H., et al. (2019). Rare KCNQ4 variants found in public databases underlie impaired channel activity that may contribute to hearing impairment. Exp. Mol. Med. 51, 1–12. doi: 10.1038/s12276-019-0300-9
Kim, H.-J., Jeong, M.-H., Kim, K.-R., Jung, C.-Y., Lee, S.-Y., Kim, H., et al. (2016). Protein arginine methylation facilitates KCNQ channel-PIP2 interaction leading to seizure suppression. Elife 5:e17159. doi: 10.7554/eLife.17159
Kim, R. Y., Yau, M. C., Galpin, J. D., Seebohm, G., Ahern, C. A., Pless, S. A., et al. (2015). Atomic basis for therapeutic activation of neuronal potassium channels. Nat. Commun. 6:8116. doi: 10.1038/ncomms9116
Kroncke, B. M., Van Horn, W. D., Smith, J., Kang, C., Welch, R. C., Song, Y., et al. (2016). Structural basis for KCNE3 modulation of potassium recycling in epithelia. Sci. Adv. 2:e1501228. doi: 10.1126/sciadv.1501228
Kubisch, C., Schroeder, B. C., Friedrich, T., Lütjohann, B., El-Amraoui, A., Marlin, S., et al. (1999). KCNQ4, a novel potassium channel expressed in sensory outer hair cells, is mutated in dominant deafness. Cell 96, 437–446. doi: 10.1016/S0092-8674(00)80556-5
Lange, W., Geissendörfer, J., Schenzer, A., Grötzinger, J., Seebohm, G., Friedrich, T., et al. (2009). Refinement of the binding site and mode of action of the anticonvulsant retigabine on KCNQ K+ channels. Mol. Pharmacol. 75, 272–280. doi: 10.1124/mol.108.052282
Laumbach, R. J., and Kipen, H. M. (2012). Respiratory health effects of air pollution: update on biomass smoke and traffic pollution. J. Allergy Clin. Immunol. 129, 3–13. doi: 10.1016/j.jaci.2011.11.021
Lee, B. H., Ryu, P. D., and Lee, S. Y. (2014). Serum starvation-induced voltage-gated potassium channel Kv7.5 expression and its regulation by Sp1 in canine osteosarcoma cells. Int. J. Mol. Sci. 15, 977–993. doi: 10.3390/ijms15010977
Lehman, A., Thouta, S., Mancini, G. M. S., Naidu, S., van Slegtenhorst, M., and McWalter, K. (2017). Loss-of-function and gain-of-function mutations in KCNQ5 cause intellectual disability or epileptic encephalopathy. Am. J. Hum. Genet. 101, 65–74. doi: 10.1016/j.ajhg.2017.05.016
Leon, G., MacDonagh, L., Finn, S. P., Cuffe, S., and Barr, M. P. (2016). Cancer stem cells in drug resistant lung cancer: targeting cell surface markers and signaling pathways. Pharmacol. Ther. 158, 71–90. doi: 10.1016/j.pharmthera.2015.12.001
Li, H., Chen, Q., Moss, A. J., Robinson, J., Goytia, V., Perry, J. C., et al. (1998). New mutations in the KVLQT1 potassium channel that cause long-QT syndrome. Circulation 97, 1264–1269. doi: 10.1161/01.CIR.97.13.1264
Li, C., Huang, P., Lu, Q., Zhou, M., Guo, L., and Xu, X. (2014a). KCNQ/Kv7 channel activator flupirtine protects against acute stress-induced impairments of spatial memory retrieval and hippocampal LTP in rats. Neuroscience 280, 19–30. doi: 10.1016/j.neuroscience.2014.09.009
Li, S.-S., Ran, Y.-J., Zhang, D.-D., Li, S.-Z., and Zhu, D. (2014b). MicroRNA-190 regulates hypoxic pulmonary vasoconstriction by targeting a voltage-gated K+ channel in arterial smooth muscle cells. J. Cell. Biochem. 115, 1196–1205. doi: 10.1002/jcb.24771
Li, X., Xu, S., Yu, M., Wang, K., Tao, Y., Zhou, Y., et al. (2020). Risk factors for severity and mortality in adult COVID-19 inpatients in Wuhan. J. Allergy Clin. Immunol. S0091-6749(20)30495-4. doi: 10.1016/j.jaci.2020.04.006
Lin, Y., Wang, X., and Jin, H. (2014). EGFR-TKI resistance in NSCLC patients: mechanisms and strategies. Am. J. Cancer Res. 4, 411–435.
Lindman, J., Khammy, M. M., Lundegaard, P. R., Aalkjær, C., and Jepps, T. A. (2018). Microtubule regulation of Kv7 channels orchestrates cAMP-mediated vasorelaxations in rat arterial smooth muscle. Hypertension 71, 336–345. doi: 10.1161/HYPERTENSIONAHA.117.10152
Loussouarn, G., Demolombe, S., Mohammad-Panah, R., Escande, D., and Baró, I. (1996). Expression of CFTR controls cAMP-dependent activation of epithelial K+ currents. Am. J. Phys. 271, C1565–C1573. doi: 10.1152/ajpcell.1996.271.5.C1565
Lundby, A., Ravn, L. S., Svendsen, J. H., Hauns, S., Olesen, S.-P., and Schmitt, N. (2008). KCNE3 mutation V17M identified in a patient with lone atrial fibrillation. Cell. Physiol. Biochem. 21, 47–54. doi: 10.1159/000113746
Lundquist, A. L., Turner, C. L., Ballester, L. Y., and George, A. L. (2006). Expression and transcriptional control of human KCNE genes. Genomics 87, 119–128. doi: 10.1016/j.ygeno.2005.09.004
Mackie, A. R., Brueggemann, L. I., Henderson, K. K., Shiels, A. J., Cribbs, L. L., Scrogin, K. E., et al. (2008). Vascular KCNQ potassium channels as novel targets for the control of mesenteric artery constriction by vasopressin, based on studies in single cells, pressurized arteries, and in vivo measurements of mesenteric vascular resistance. J. Pharmacol. Exp. Ther. 325, 475–483. doi: 10.1124/jpet.107.135764
Maeno, E., Ishizaki, Y., Kanaseki, T., Hazama, A., and Okada, Y. (2000). Normotonic cell shrinkage because of disordered volume regulation is an early prerequisite to apoptosis. Proc. Natl. Acad. Sci. U. S. A. 97, 9487–9492. doi: 10.1073/pnas.140216197
Maljevic, S., Wuttke, T. V., Seebohm, G., and Lerche, H. (2010). KV7 channelopathies. Arch. Eur. J. Physiol. 460, 277–288. doi: 10.1007/s00424-010-0831-3
Mall, M., Gonska, T., Thomas, J., Schreiber, R., Seydewitz, H. H., Kuehr, J., et al. (2003). Modulation of Ca2+ activated Cl− secretion by basolateral K+ channels in human normal and cystic fibrosis airway epithelia. Pediatr. Res. 53, 608–618. doi: 10.1203/01.PDR.0000057204.51420.DC
Mall, M., Kunzelmann, K., Hipper, A., Busch, A. E., and Greger, R. (1996). CAMP stimulation of CFTR-expressing Xenopus oocytes activates a chromanol-inhibitable K+ conductance. Arch. Eur. J. Physiol. 432, 516–522. doi: 10.1007/s004240050164
Mall, M., Wissner, A., Schreiber, R., Kuehr, J., Seydewitz, H. H., Brandis, M., et al. (2000). Role of K(V)LQT1 in cyclic adenosine monophosphate-mediated Cl(−) secretion in human airway epithelia. Am. J. Respir. Cell Mol. Biol. 23, 283–289. doi: 10.1165/ajrcmb.23.3.4060
Mani, B. K., Robakowski, C., Brueggemann, L. I., Cribbs, L. L., Tripathi, A., Majetschak, M., et al. (2016). Kv7.5 potassium channel subunits are the primary targets for PKA-dependent enhancement of vascular smooth muscle Kv7 currents. Mol. Pharmacol. 89, 323–334. doi: 10.1124/mol.115.101758
Manville, R. W., and Abbott, G. W. (2018). Gabapentin is a potent activator of KCNQ3 and KCNQ5 potassium channels. Mol. Pharmacol. 94, 1155–1163. doi: 10.1124/mol.118.112953
Manville, R. W., and Abbott, G. W. (2020). Potassium channels act as chemosensors for solute transporters. Commun. Biol. 3:90. doi: 10.1038/s42003-020-0820-9
Manville, R. W., Papanikolaou, M., and Abbott, G. W. (2018). Direct neurotransmitter activation of voltage-gated potassium channels. Nat. Commun. 9:1847. doi: 10.1038/s41467-018-04266-w
Marx, S. O., Kurokawa, J., Reiken, S., Motoike, H., D’Armiento, J., Marks, A. R., et al. (2002). Requirement of a macromolecular signaling complex for beta adrenergic receptor modulation of the KCNQ1-KCNE1 potassium channel. Science 295, 496–499. doi: 10.1126/science.1066843
Mazzone, S. B., and Undem, B. J. (2016). Vagal afferent innervation of the airways in health and disease. Physiol. Rev. 96, 975–1024. doi: 10.1152/physrev.00039.2015
McLaughlin, V. V., Shah, S. J., Souza, R., and Humbert, M. (2015). Management of pulmonary arterial hypertension. J. Am. Coll. Cardiol. 65, 1976–1997. doi: 10.1016/j.jacc.2015.03.540
Melosky, B. (2014). Review of EGFRTKIs in metastatic NSCLC, including ongoing trials. Front. Oncol. 4:244. doi: 10.3389/fonc.2014.00244
Mondejar-Parreño, G., Morales-Cano, D., Barreira, B., Callejo, M., Ruiz-Cabello, J., Moreno, L., et al. (2018). HIV transgene expression impairs K+ channel function in the pulmonary vasculature. Am. J. Phys. Lung Cell. Mol. Phys. 315, L711–L723. doi: 10.1152/ajplung.00045.2018
Mondejar-Parreño, G., Moral-Sanz, J., Barreira, B., De la Cruz, A., Gonzalez, T., Callejo, M., et al. (2019). Activation of Kv7 channels as a novel mechanism for NO/cGMP-induced pulmonary vasodilation. Br. J. Pharmacol. 176, 2131–2145. doi: 10.1111/bph.14662
Morales-Cano, D., Callejo, M., Barreira, B., Mondejar-Parreño, G., Esquivel-Ruiz, S., Ramos, S., et al. (2019). Elevated pulmonary arterial pressure in Zucker diabetic fatty rats. PLoS One 14:e0211281. doi: 10.1371/journal.pone.0211281
Morales-Cano, D., Menendez, C., Moreno, E., Moral-Sanz, J., Barreira, B., Galindo, P., et al. (2014). The flavonoid quercetin reverses pulmonary hypertension in rats. PLoS One 9:e114492. doi: 10.1371/journal.pone.0114492
Morecroft, I., Murray, A., Nilsen, M., Gurney, A., and MacLean, M. (2009). Treatment with the Kv7 potassium channel activator flupirtine is beneficial in two independent mouse models of pulmonary hypertension. Br. J. Pharmacol. 157, 1241–1249. doi: 10.1111/j.1476-5381.2009.00283.x
Moser, S. L., Harron, S. A., Crack, J., Fawcett, J. P., and Cowley, E. A. (2008). Multiple KCNQ potassium channel subtypes mediate basal anion secretion from the human airway epithelial cell line Calu-3. J. Membr. Biol. 221, 153–163. doi: 10.1007/s00232-008-9093-9
Moudgil, R., Michelakis, E. D., and Archer, S. L. (2006). The role of K+ channels in determining pulmonary vascular tone, oxygen sensing, cell proliferation, and apoptosis: implications in hypoxic pulmonary vasoconstriction and pulmonary arterial hypertension. Microcirculation 13, 615–632. doi: 10.1080/10739680600930222
Neubauer, B. A., Waldegger, S., Heinzinger, J., Hahn, A., Kurlemann, G., Fiedler, B., et al. (2008). KCNQ2 and KCNQ3 mutations contribute to different idiopathic epilepsy syndromes. Neurology 71, 177–183. doi: 10.1212/01.wnl.0000317090.92185.ec
O’Grady, S. M., and Lee, S. Y. (2005). Molecular diversity and function of voltage-gated (Kv) potassium channels in epithelial cells. Int. J. Biochem. Cell Biol. 37, 1578–1594. doi: 10.1016/j.biocel.2005.04.002
Ohno, S., Toyoda, F., Zankov, D. P., Yoshida, H., Makiyama, T., Tsuji, K., et al. (2009). Novel KCNE3 mutation reduces repolarizing potassium current and associated with long QT syndrome. Hum. Mutat. 30, 557–563. doi: 10.1002/humu.20834
Oliveras, A., Roura-Ferrer, M., Solé, L., de la Cruz, A., Prieto, A., Etxebarria, A., et al. (2014). Functional assembly of Kv7.1/Kv7.5 channels with emerging properties on vascular muscle physiology. Arterioscler. Thromb. Vasc. Biol. 34, 1522–1530. doi: 10.1161/ATVBAHA.114.303801
Panchanathan, E., Ramanathan, G., and Lakkakula, B. V. K. S. (2013). Effect of flupirtine on the growth and viability of U373 malignant glioma cells. Cancer Biol. Med. 10, 142–147. doi: 10.7497/j.issn.2095-3941.2013.03.004
Pancrazio, J. J., Tabbara, I. A., and Kim, Y. I. (1993). Voltage-activated K+ conductance and cell proliferation in small-cell lung cancer. Anticancer Res. 13, 1231–1234.
Penn, R. B. (2008). Embracing emerging paradigms of G protein-coupled receptor agonism and signaling to address airway smooth muscle pathobiology in asthma. Naunyn Schmiedeberg's Arch. Pharmacol. 378, 149–169. doi: 10.1007/s00210-008-0263-1
Potet, F., Scott, J. D., Mohammad-Panah, R., Escande, D., and Baró, I. (2001). AKAP proteins anchor cAMP-dependent protein kinase to KvLQT1/IsK channel complex. Am. J. Physiol. Heart Circ. Physiol. 280, H2038–H2045. doi: 10.1152/ajpheart.2001.280.5.H2038
Preston, P., Wartosch, L., Günzel, D., Fromm, M., Kongsuphol, P., Ousingsawat, J., et al. (2010). Disruption of the K+ channel beta-subunit KCNE3 reveals an important role in intestinal and tracheal Cl− transport. J. Biol. Chem. 285, 7165–7175. doi: 10.1074/jbc.M109.047829
Prevarskaya, N., Skryma, R., and Shuba, Y. (2010). Ion channels and the hallmarks of cancer. Trends Mol. Med. 16, 107–121. doi: 10.1016/j.molmed.2010.01.005
Prevarskaya, N., Skryma, R., and Shuba, Y. (2018). Ion channels in cancer: are cancer hallmarks oncochannelopathies? Physiol. Rev. 98, 559–621. doi: 10.1152/physrev.00044.2016
Putnam, L. R., Tsao, K., Morini, F., Lally, P. A., Miller, C. C., and Lally, K. P. (2016). Evaluation of variability in inhaled nitric oxide use and pulmonary hypertension in patients with congenital diaphragmatic hernia. JAMA Pediatr. 170, 1188–1194. doi: 10.1001/jamapediatrics.2016.2023
Rao, V. R., Perez-Neut, M., Kaja, S., and Gentile, S. (2015). Voltage-gated ion channels in cancer cell proliferation. Cancer 7, 849–875. doi: 10.3390/cancers7020813
Richard, V., Nair, M. G., Santhosh Kumar, T. R., and Pillai, M. R. (2013). Side population cells as prototype of chemoresistant, tumor-initiating cells. Biomed. Res. Int. 2013:517237. doi: 10.1155/2013/517237
Roura-Ferrer, M., Etxebarria, A., Solé, L., Oliveras, A., Comes, N., Villarroel, A., et al. (2009). Functional implications of KCNE subunit expression for the Kv7.5 (KCNQ5) channel. Cell. Physiol. Biochem. 24, 325–334. doi: 10.1159/000257425
Roura-Ferrer, M., Solé, L., Oliveras, A., Dahan, R., Bielanska, J., Villarroel, A., et al. (2010). Impact of KCNE subunits on KCNQ1 (Kv7.1) channel membrane surface targeting. J. Cell. Physiol. 225, 692–700. doi: 10.1002/jcp.22265
Roura-Ferrer, M., Solé, L., Oliveras, A., Villarroel, A., Comes, N., and Felipe, A. (2012). Targeting of Kv7.5 (KCNQ5)/KCNE channels to surface microdomains of cell membranes. Muscle Nerve 45, 48–54. doi: 10.1002/mus.22231
Sabater-Lleal, M., Mälarstig, A., Folkersen, L., Soler Artigas, M., Baldassarre, D., Kavousi, M., et al. (2014). Common genetic determinants of lung function, subclinical atherosclerosis and risk of coronary artery disease. PLoS One 9:e104082. doi: 10.1371/journal.pone.0104082
Salata, J. J., Jurkiewicz, N. K., Wang, J., Evans, B. E., Orme, H. T., and Sanguinetti, M. C. (1998). A novel benzodiazepine that activates cardiac slow delayed rectifier K+ currents. Mol. Pharmacol. 54, 220–230. doi: 10.1124/mol.54.1.220
Salvi, S. (2014). Tobacco smoking and environmental risk factors for chronic obstructive pulmonary disease. Clin. Chest Med. 35, 17–27. doi: 10.1016/j.ccm.2013.09.011
Sands, T. T., Miceli, F., Lesca, G., Beck, A. E., Sadleir, L. G., Arrington, D. K., et al. (2019). Autism and developmental disability caused by KCNQ3 gain-of-function variants. Ann. Neurol. 86, 181–192. doi: 10.1002/ana.25522
Schenzer, A., Friedrich, T., Pusch, M., Saftig, P., Jentsch, T. J., Grötzinger, J., et al. (2005). Molecular determinants of KCNQ (Kv7) K+ channel sensitivity to the anticonvulsant retigabine. J. Neurosci. 25, 5051–5060. doi: 10.1523/JNEUROSCI.0128-05.2005
Schroeder, B. C., Waldegger, S., Fehr, S., Bleich, M., Warth, R., Greger, R., et al. (2000). A constitutively open potassium channel formed by KCNQ1 and KCNE3. Nature 403, 196–199. doi: 10.1038/35003200
Schwab, A., Hanley, P., Fabian, A., and Stock, C. (2008). Potassium channels keep mobile cells on the go. Physiology 23, 212–220. doi: 10.1152/physiol.00003.2008
Schwiebert, E. M., Benos, D. J., Egan, M. E., Stutts, M. J., and Guggino, W. B. (1999). CFTR is a conductance regulator as well as a chloride channel. Physiol. Rev. 79, S145–S166. doi: 10.1152/physrev.1999.79.1.S145
Sedivy, V., Joshi, S., Ghaly, Y., Mizera, R., Zaloudikova, M., Brennan, S., et al. (2015). Role of Kv7 channels in responses of the pulmonary circulation to hypoxia. Am. J. Phys. Lung Cell. Mol. Phys. 308, L48–L57. doi: 10.1152/ajplung.00362.2013
Serrano-Novillo, C., Capera, J., Colomer-Molera, M., Condom, E., Ferreres, J. C., and Felipe, A. (2019). Implication of voltage-gated potassium channels in neoplastic cell proliferation. Cancer 11:287. doi: 10.3390/cancers11030287
Sevilla-Montero, J., Labrousse-Arias, D., Fernández-Pérez, C., Barreira, B., Mondejar-Parreño, G., Cogolludo, A., et al. (2019). Direct effects of cigarette smoke in pulmonary arterial cells alter vascular tone through arterial remodeling and Kv7.4 channel dysregulation. BioRxiv 555953. [Preprint]. doi: 10.1101/555953
Shi, J., and Wei, L. (2013). Rho kinases in cardiovascular physiology and pathophysiology: the effect of fasudil. J. Cardiovasc. Pharmacol. 62, 341–354. doi: 10.1097/FJC.0b013e3182a3718f
Shimokawa, H., and Satoh, K. (2015). 2015 ATVB plenary lecture: translational research on rho-kinase in cardiovascular medicine. Arterioscler. Thromb. Vasc. Biol. 35, 1756–1769. doi: 10.1161/ATVBAHA.115.305353
Simonneau, G., Montani, D., Celermajer, D. S., Denton, C. P., Gatzoulis, M. A., Krowka, M., et al. (2019). Haemodynamic definitions and updated clinical classification of pulmonary hypertension. Eur. Respir. J. 53:1801913. doi: 10.1183/13993003.01913-2018
Singh, A., Wu, H., Zhang, P., Happel, C., Ma, J., and Biswal, S. (2010). Expression of ABCG2 (BCRP) is regulated by Nrf2 in cancer cells that confers side population and chemoresistance phenotype. Mol. Cancer Ther. 9, 2365–2376. doi: 10.1158/1535-7163.MCT-10-0108
Soler Artigas, M., Loth, D. W., Wain, L. V., Gharib, S. A., Obeidat, M., Tang, W., et al. (2011). Genome-wide association and large-scale follow up identifies 16 new loci influencing lung function. Nat. Genet. 43, 1082–1090. doi: 10.1038/ng.941
Splawski, I., Shen, J., Timothy, K. W., Lehmann, M. H., Priori, S., Robinson, J. L., et al. (2000). Spectrum of mutations in long-QT syndrome genes. KVLQT1, HERG, SCN5A, KCNE1, and KCNE2. Circulation 102, 1178–1185. doi: 10.1161/01.CIR.102.10.1178
Steinbichler, T. B., Dudás, J., Skvortsov, S., Ganswindt, U., Riechelmann, H., and Skvortsova, I.-I. (2018). Therapy resistance mediated by cancer stem cells. Semin. Cancer Biol. 53, 156–167. doi: 10.1016/j.semcancer.2018.11.006
Stott, J. B., Barrese, V., Jepps, T. A., Leighton, E. V., and Greenwood, I. A. (2015). Contribution of Kv7 channels to natriuretic peptide mediated vasodilation in normal and hypertensive rats. Hypertension 65, 676–682. doi: 10.1161/HYPERTENSIONAHA.114.04373
Stott, J. B., Jepps, T. A., and Greenwood, I. A. (2014). KV7 potassium channels: a new therapeutic target in smooth muscle disorders. Drug Discov. Today 19, 413–424. doi: 10.1016/j.drudis.2013.12.003
Strutz-Seebohm, N., Seebohm, G., Fedorenko, O., Baltaev, R., Engel, J., Knirsch, M., et al. (2006). Functional coassembly of KCNQ4 with KCNE-beta-subunits in Xenopus oocytes. Cell. Physiol. Biochem. 18, 57–66. doi: 10.1159/000095158
Sun, H., Lin, A.-H., Ru, F., Patil, M. J., Meeker, S., Lee, L.-Y., et al. (2019). KCNQ/M-channels regulate mouse vagal bronchopulmonary C-fiber excitability and cough sensitivity. JCI Insight 4:e124467. doi: 10.1172/jci.insight.124467
Sun, J., and MacKinnon, R. (2020). Structural basis of human KCNQ1 modulation and gating. Cell 180, 340.e9–347.e9. doi: 10.1016/j.cell.2019.12.003
Sylvester, J. T., Shimoda, L. A., Aaronson, P. I., and Ward, J. P. T. (2012). Hypoxic pulmonary vasoconstriction. Physiol. Rev. 92, 367–520. doi: 10.1152/physrev.00041.2010
Taylor, K. C., and Sanders, C. R. (2017). Regulation of KCNQ/Kv7 family voltage-gated K+ channels by lipids. Biochim. Biophys. Acta Biomembr. 1859, 586–597. doi: 10.1016/j.bbamem.2016.10.023
Tinel, N., Diochot, S., Lauritzen, I., Barhanin, J., Lazdunski, M., and Borsotto, M. (2000). M-type KCNQ2-KCNQ3 potassium channels are modulated by the KCNE2 subunit. FEBS Lett. 480, 137–141. doi: 10.1016/S0014-5793(00)01918-9
Tobin, M. J. (2005). Update in pulmonary diseases. Ann. Intern. Med. 142, 283–288. doi: 10.7326/0003-4819-142-4-200502150-00010
Trinh, N. T. N., Privé, A., Kheir, L., Bourret, J.-C., Hijazi, T., Amraei, M. G., et al. (2007). Involvement of KATP and KvLQT1 K+ channels in EGF-stimulated alveolar epithelial cell repair processes. Am. J. Phys. Lung Cell. Mol. Phys. 293, L870–L882. doi: 10.1152/ajplung.00362.2006
Undem, B. J., and Kollarik, M. (2005). The role of vagal afferent nerves in chronic obstructive pulmonary disease. Proc. Am. Thorac. Soc. 2, 355–360. doi: 10.1513/pats.200504-033SR
Van Horn, W. D., Vanoye, C. G., and Sanders, C. R. (2011). Working model for the structural basis for KCNE1 modulation of the KCNQ1 potassium channel. Curr. Opin. Struct. Biol. 21, 283–291. doi: 10.1016/j.sbi.2011.01.001
Vanoye, C. G., Welch, R. C., Daniels, M. A., Manderfield, L. J., Tapper, A. R., and Sanders, C. R. (2009). Distinct subdomains of the KCNQ1 S6 segment determine channel modulation by different KCNE subunits. J. Gen. Physiol. 134, 207–217. doi: 10.1085/jgp.200910234
Vigil, F. A., Bozdemir, E., Bugay, V., Chun, S. H., Hobbs, M., Sanchez, I., et al. (2020). Prevention of brain damage after traumatic brain injury by pharmacological enhancement of KCNQ (Kv7, «M-type») K+ currents in neurons. J. Cereb. Blood Flow Metab. 40, 1256–1273. doi: 10.1177/0271678X19857818
Wang, K., Zeng, J., Luo, L., Yang, J., Chen, J., Li, B., et al. (2013). Identification of a cancer stem cell-like side population in the HeLa human cervical carcinoma cell line. Oncol. Lett. 6, 1673–1680. doi: 10.3892/ol.2013.1607
WHO Global Health Estimates (2020). WHO; World Health Organization. Available at: http://www.who.int/healthinfo/global_burden_disease/en/ (Accessed March 1, 2020).
Wickenden, A. D., Krajewski, J. L., London, B., Wagoner, P. K., Wilson, W. A., Clark, S., et al. (2008). N-(6-chloro-pyridin-3-yl)-3,4-difluoro-benzamide (ICA-27243): a novel, selective KCNQ2/Q3 potassium channel activator. Mol. Pharmacol. 73, 977–986. doi: 10.1124/mol.107.043216
Xiong, Q., Sun, H., Zhang, Y., Nan, F., and Li, M. (2008). Combinatorial augmentation of voltage-gated KCNQ potassium channels by chemical openers. Proc. Natl. Acad. Sci. U. S. A. 105, 3128–3133. doi: 10.1073/pnas.0712256105
Yang, W. P., Levesque, P. C., Little, W. A., Conder, M. L., Ramakrishnan, P., Neubauer, M. G., et al. (1998). Functional expression of two KvLQT1-related potassium channels responsible for an inherited idiopathic epilepsy. J. Biol. Chem. 273, 19419–19423. doi: 10.1074/jbc.273.31.19419
Zaydman, M. A., and Cui, J. (2014). PIP2 regulation of KCNQ channels: biophysical and molecular mechanisms for lipid modulation of voltage-dependent gating. Front. Physiol. 5:195. doi: 10.3389/fphys.2014.00195
Zhang, X., An, H., Li, J., Zhang, Y., Liu, Y., Jia, Z., et al. (2016). Selective activation of vascular Kv7.4/Kv7.5 K+ channels by fasudil contributes to its vasorelaxant effect. Br. J. Pharmacol. 173, 3480–3491. doi: 10.1111/bph.13639
Zhang, Y., and Wu, S. (2017). Effects of fasudil on pulmonary hypertension in clinical practice. Pulm. Pharmacol. Ther. 46, 54–63. doi: 10.1016/j.pupt.2017.08.002
Zhou, L., Köhncke, C., Hu, Z., Roepke, T. K., and Abbott, G. W. (2019). The KCNE2 potassium channel β subunit is required for normal lung function and resilience to ischemia and reperfusion injury. FASEB J. 33, 9762–9774. doi: 10.1096/fj.201802519R
Keywords: Kv7 channels, KCNE, respiratory diseases, asthma, chronic obstructive pulmonary disease, pulmonary hypertension
Citation: Mondejar-Parreño G, Perez-Vizcaino F and Cogolludo A (2020) Kv7 Channels in Lung Diseases. Front. Physiol. 11:634. doi: 10.3389/fphys.2020.00634
Received: 18 March 2020; Accepted: 18 May 2020;
Published: 26 June 2020.
Edited by:
Francesco Miceli, University of Naples Federico II, ItalyReviewed by:
Núria Comes, University of Barcelona, SpainCopyright © 2020 Mondejar-Parreño, Perez-Vizcaino and Cogolludo. This is an open-access article distributed under the terms of the Creative Commons Attribution License (CC BY). The use, distribution or reproduction in other forums is permitted, provided the original author(s) and the copyright owner(s) are credited and that the original publication in this journal is cited, in accordance with accepted academic practice. No use, distribution or reproduction is permitted which does not comply with these terms.
*Correspondence: Angel Cogolludo, YWNvZ29sbHVkb0BtZWQudWNtLmVz
Disclaimer: All claims expressed in this article are solely those of the authors and do not necessarily represent those of their affiliated organizations, or those of the publisher, the editors and the reviewers. Any product that may be evaluated in this article or claim that may be made by its manufacturer is not guaranteed or endorsed by the publisher.
Research integrity at Frontiers
Learn more about the work of our research integrity team to safeguard the quality of each article we publish.