- Smidt Heart Institute, Cedars-Sinai Medical Center, Los Angeles, CA, United States
Heart disease remains an increasing major public health challenge in the United States and worldwide. A common end-organ feature in diseased hearts is myocardial fibrosis, which stiffens the heart and interferes with normal pump function, leading to pump failure. The development of cells for regenerative therapy has been met with many pitfalls on its path to clinical translation. Recognizing that regenerative cells secrete therapeutically bioactive vesicles has paved the way to circumvent many failures of cell therapy. In this review, we provide an overview of extracellular vesicles (EVs), with a focus on their utility as therapeutic agents for cardiac regeneration. We also highlight the engineering potential of EVs to enhance their therapeutic application.
Introduction
Despite significant advances in science and medicine, heart disease remains an increasing public health concern, and is a leading cause of morbidity and mortality worldwide (Roth et al., 2017). In adult mammals, the default response to cardiac insults or disease is scar formation which lowers myocardial compliance, decreases ventricular filling, interferes with electrical coupling, and ultimately leads to depressed cardiac performance (Sharma and Kass, 2014). Conventional therapies such as β-blockers are beneficial in patients with myocardial fibrosis; however, they do not directly treat the underlying causes of fibrosis (Jameel and Zhang, 2009; Members et al., 2012). There is ample evidence for the efficacy of cardiac cell therapy to treat myocardial fibrosis in preclinical models (Zwetsloot et al., 2016) and, to a lesser extent, in patients (Nigro et al., 2018). However, cells are fragile, living entities which can be difficult to manufacture and to handle (Dodson and Levine, 2015).
In recent years, the emphasis has shifted away from cell therapy toward a cell-free therapeutic paradigm. Although extracellular vesicles (EVs) have long been known to be produced by eukaryotic cells, only recently were EVs implicated as mediators of the paracrine benefits of cell therapy. Extensive evidence now supports the concept that EVs are vital for the benefits of numerous therapeutic cells such as neural progenitors (Marzesco et al., 2005), mesenchymal stem cells (Lai et al., 2010), CD34+ cells (Sahoo et al., 2011), and cardiosphere-derived cells (CDCs; Ibrahim et al., 2014). Importantly, EVs offer the potential to overcome key limitations of cell therapy. For example, advantages may include product stability (Akers et al., 2016), immune tolerability (Gallet et al., 2017; Aminzadeh et al., 2018), flexibility of dosing (not limited by microvascular plugging or loss of transplanted cell viability; Ibrahim and Marbán, 2016), and the potential for engineering to enhance efficacy (Conlan et al., 2017). In this review, we summarize EV biology, cellular and molecular players in myocardial fibrosis, the utility of EVs as therapeutic agents, and conclude with the promise of engineered EVs as next-generation therapeutic candidates.
Extracellular Vesicles Defined
Conserved through evolution, cellular release of EVs occurs in bacteria, fungi, plants, and animals (Barile et al., 2017). Based on studies of sheep reticulocytes, EV secretion was originally postulated to be a mechanism for elimination of cell waste including non-essential proteins (Johnstone et al., 1987). More recently, EVs have come to be viewed as key mediators of intercellular communication. EVs can deliver and exchange bioactive components from donor to recipient cells, regulating gene expression and altering cellular function (Stahl and Raposo, 2018). Several lines of evidence implicate EVs as important signaling mediators that carry proteins, lipids, and nucleic acids in physiological and pathophysiological conditions (Ibrahim et al., 2014; Ibrahim and Marbán, 2016).
Classification of Extracellular Vesicles
In the classical sense, the term EVs refers to all cell-secreted membrane vesicles. Based on their size, biogenesis, and secretory pathway, EVs can be broadly classified into three major classes: exosomes, microvesicles, and apoptotic bodies (Figure 1). The primary focus here will be on the first two classes of EVs.
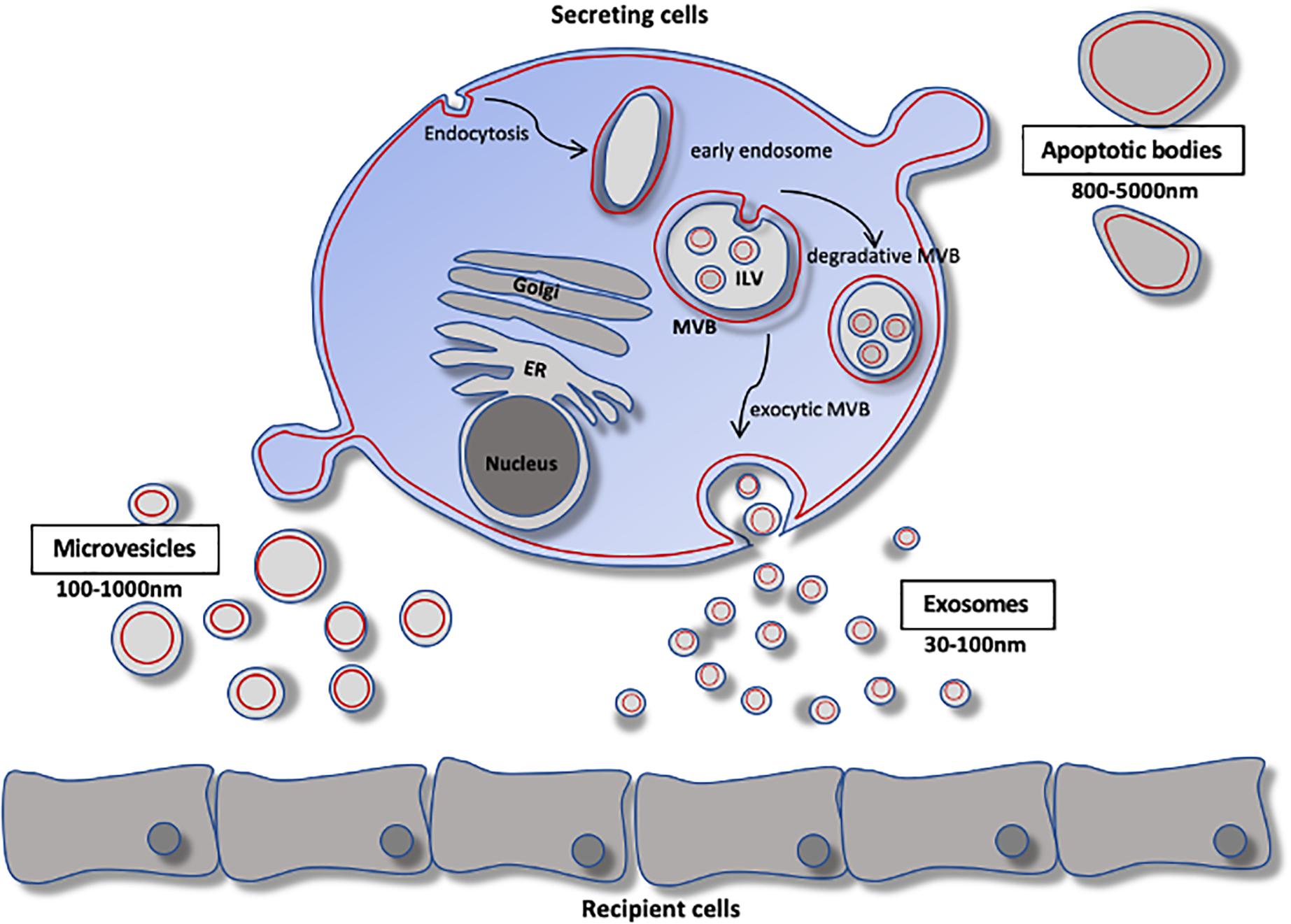
Figure 1. Extracellular vesicle biology. Schematic of extracellular vesicle biogenesis. Exosomes arise from the fusion of inward budding of multivesicular bodies. The resulting vesicles are either degraded by lysosomes or secreted as exsomes. Microvesicles arise by direct budding from the plasma membrane and enter the extracellular space. Apoptotic bodies arise from membrane protrusions fragmentation of an apoptotic cell. ER, endoplasmic reticulum; ILV, intraluminal vesicle; MVB, multivesicular body.
Exosomes are secreted and taken up by all eukaryotic cells and have been found abundantly in nearly all biological fluids. Exosomes are lipid-bilayer vesicles of endosomal origin that arise from inward budding of multivesicular bodies, and range in size from 30 to ∼100 nm in diameter. Multivesicular bodies can fuse with the plasma membrane to release their contents into the extracellular space, or can be trafficked to lysosomes for degradation (Colombo et al., 2014). Exosomal cargo contains non-random assortments of protein, RNA, and lipids, differing substantially from the cytoplasmic contents of the parent cells, but nevertheless reflecting the parent cell type and its metabolic state. According to ExoCarta1, at least 9,769 proteins, 4,946 mRNAs, 2,838 miRNAs, and 1,116 lipids have been identified in exosomes from various cell types. Though the diversity of exosomal cargo is vast, exosomes share common molecular markers including tetraspanins (CD9, CD63, and CD81). However, diversity exists among the expression of these tetraspanins – although the biological significance is currently unclear (Bobrie et al., 2012).
In contrast to exosomes, microvesicles are generally larger and range in size (100–1000 nm in diameter). The mechanism of microvesicle biogenesis is not well understood; however, it is thought to require cytoskeletal components such as actin and microtubules (along with the respective molecular motors), and fusion machinery (SNAREs and tethering proteins) (Cai et al., 2007). Microvesicles arise by direct budding from the plasma membrane and enter the extracellular space (He et al., 2018). Accordingly, microvesicle contents closely resemble the composition of the cytosol of the parent cell (Mohammadi et al., 2019).
We next consider the biology of cardiac fibrosis, then return to EVs as therapeutic candidates to offset fibrosis. For a detailed review of cardiac regeneration and EV biology, the reader is referred to recently published reviews (Balbi et al., 2020; Tikhomirov et al., 2020).
Origin of Cardiac Fibrosis
The deposition of collagen occurs in three forms: replacement, interstitial, and perivascular (Figure 2). As a result of cardiomyocyte loss, replacement fibrosis ensues to fill devoid space within the myocardium (Rogers and Otis, 2017). Dominating in acute myocardial infarction (MI), replacement fibrosis is critical to protecting the myocardium from rupture and dilative remodeling by preserving structural integrity and normalizing myocardial wall stress. Interstitial and perivascular fibrosis develop as excess collagen deposits in the myocardial interstitium and surrounding the peri-adventitia, respectively (Frangogiannis, 2019). The latter two are the unfortunate consequences of a number of insults, including myocardial inflammation, which lead to chronic heart disease. The extent of interstitial and perivascular fibrosis is closely associated with adverse clinical outcomes (Berk et al., 2007; Kong et al., 2014). As such, the development of therapeutic interventions to combat myocardial fibrosis remain a major focus of current research.
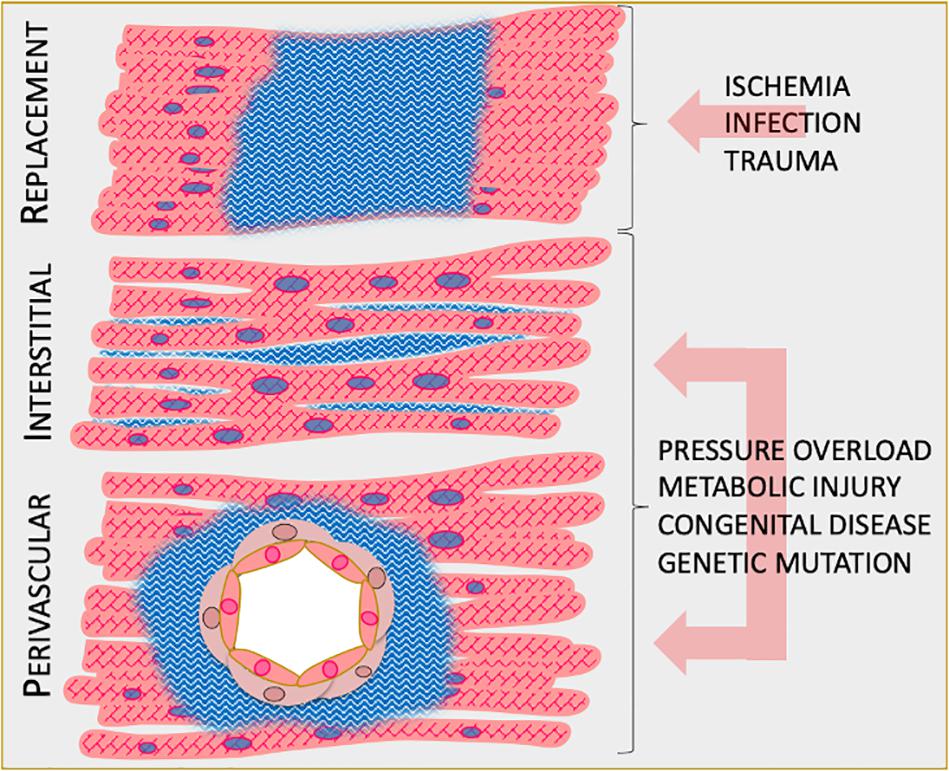
Figure 2. Manifestations of cardiac fibrosis. Schematic of the three main types of cardiac fibrosis. Replacement fibrosis develops to fill necrotic lesions within the myocardium as a result of ischemia, infection, or trauma. Interstitial fibrosis develops in the interstitial space of the myocardium as a result of non-ischemic cardiomyopathies. Perivascular fibrosis develops in the peri-adventitial space as a result of non-ischemic cardiomyopathies.
Cellular and Molecular Players in Cardiac Fibrosis
The hallmark of any cardiac insult remains the universal activation of the innate and adaptive immune system. For example, following acute myocardial infarction, dead cardiomyocytes release DNA (Roers et al., 2016) and cellular proteins (Scaffidi et al., 2002; Panayi et al., 2004) into the extracellular space which serve as damage-associated molecular patterns (Rubartelli and Lotze, 2007). These signals are sensed by leukocytes (Tang et al., 2012) and activate nuclear factor kappa-light-chain-enhancer of activated B cells (NFκB)-mediated transcription of pro-inflammatory cytokines, chemokines, and other mediators of the inflammatory response (Legrand et al., 2019). Mounting evidence suggests infiltrating macrophages are key mediators of the pro-fibrotic response to injury (Frangogiannis, 2019). In animal models of pressure overload-induced heart failure, recruited M1 macrophages communicate with CD4+ T lymphocytes and perpetuate the pro-inflammatory wave leading to fibrosis, cardiac dysfunction, and heart failure (Nevers et al., 2015; Patel et al., 2018). Moreover, macrophages can activate resident stromal cells which contribute directly to collagen deposition. For example, in response to angiotensin-induced hypertrophic cardiomyopathy, macrophages stimulate cardiac fibroblasts to produce IL-6 leading to TGFβ1 production and subsequent development of cardiac fibrosis (Ma et al., 2012).
Perhaps the most widely known macrophage-secreted cytokine to drive fibrosis is TGFβ, which binds to its cognate receptors on cardiac tissue (TGFβR2, ALK, and others) and mobilizes Smad2 and Smad3 transcription factors to initiate myofibroblast differentiation (Khalil et al., 2017; Pardali et al., 2017). The principal source of myofibroblasts in the heart are resident fibroblasts, and to a lesser degree, endothelial cells. However, not all macrophages are created equal. M2c macrophages, for example, phagocytose dead cells and debris and secrete matrix metalloproteinases, which can reabsorb collagen deposits and contribute to remodeling of the extracellular matrix (Cheng et al., 2018; Krzyszczyk et al., 2018). The interplay among macrophages, fibroblasts, and endothelial cells is now understood to be a major driving force of myocardial fibrosis. Thus, in the context of anti-fibrogenesis, EVs can intervene by direct stimulation of pro-inflammatory M1 macrophages to differentiate into an M2-like phenotype (Silva et al., 2017). This step is important for the resolution of inflammation and subsequent anti-fibrotic actions. We will next discuss, in more detail, immunomodulatory actions of EVs.
Extracellular Vesicles as Therapeutic Agents
Recently, EVs (and exosomes in particular) are attracting much interest – not only in physiological and pathological cell–cell communication, but also as a platform for therapeutic development (Sluijter et al., 2018). The recognition that progenitor cells secrete EVs that are bioactive ushered in the concept of EVs as cell-free therapeutic candidates (Figure 3). As the holy grail of regenerative medicine, restoring both cardiac structure and function are fundamental goals of any therapeutic candidate. Indeed, EVs harvested from cardiac stem/progenitor cells, mesenchymal stem/stromal cells (MSCs), embryonic stem cells (ESCs), induced pluripotent stem cells (iPSCs), and non-stem cell sources have demonstrated benefits in cardiac regeneration (Alibhai et al., 2018; Figure 4). By supplanting cell transplantation with administration of EVs, many concerns and limitations regarding safety and feasibility from cell therapy can be attenuated.
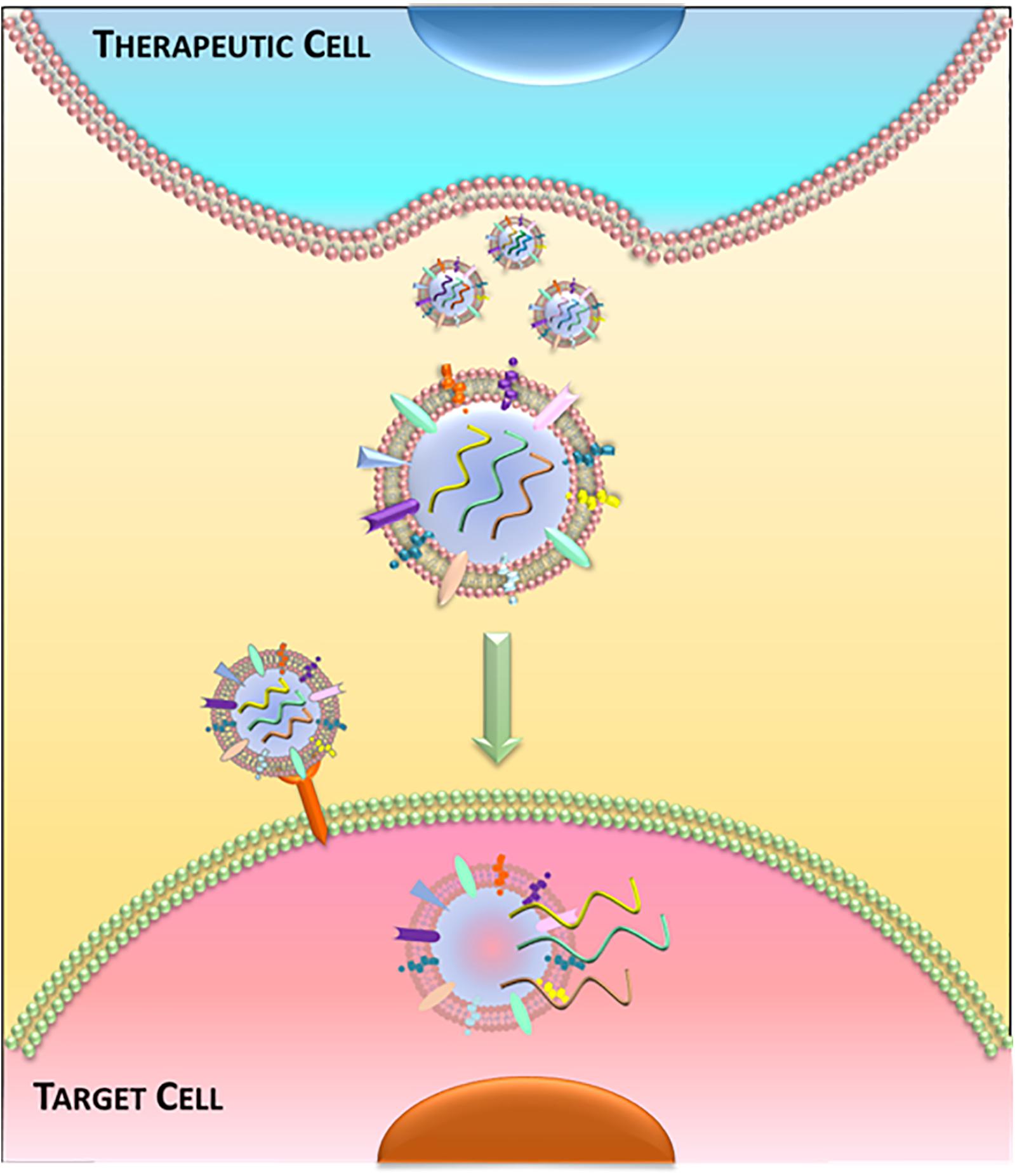
Figure 3. Extracellular vesicle-mediated communication. Schematic of release and uptake of extracellular vesicles. Therapeutic cells secrete bioactive extracellular vesicles, which are taken up by diseased cells to alter cell function.
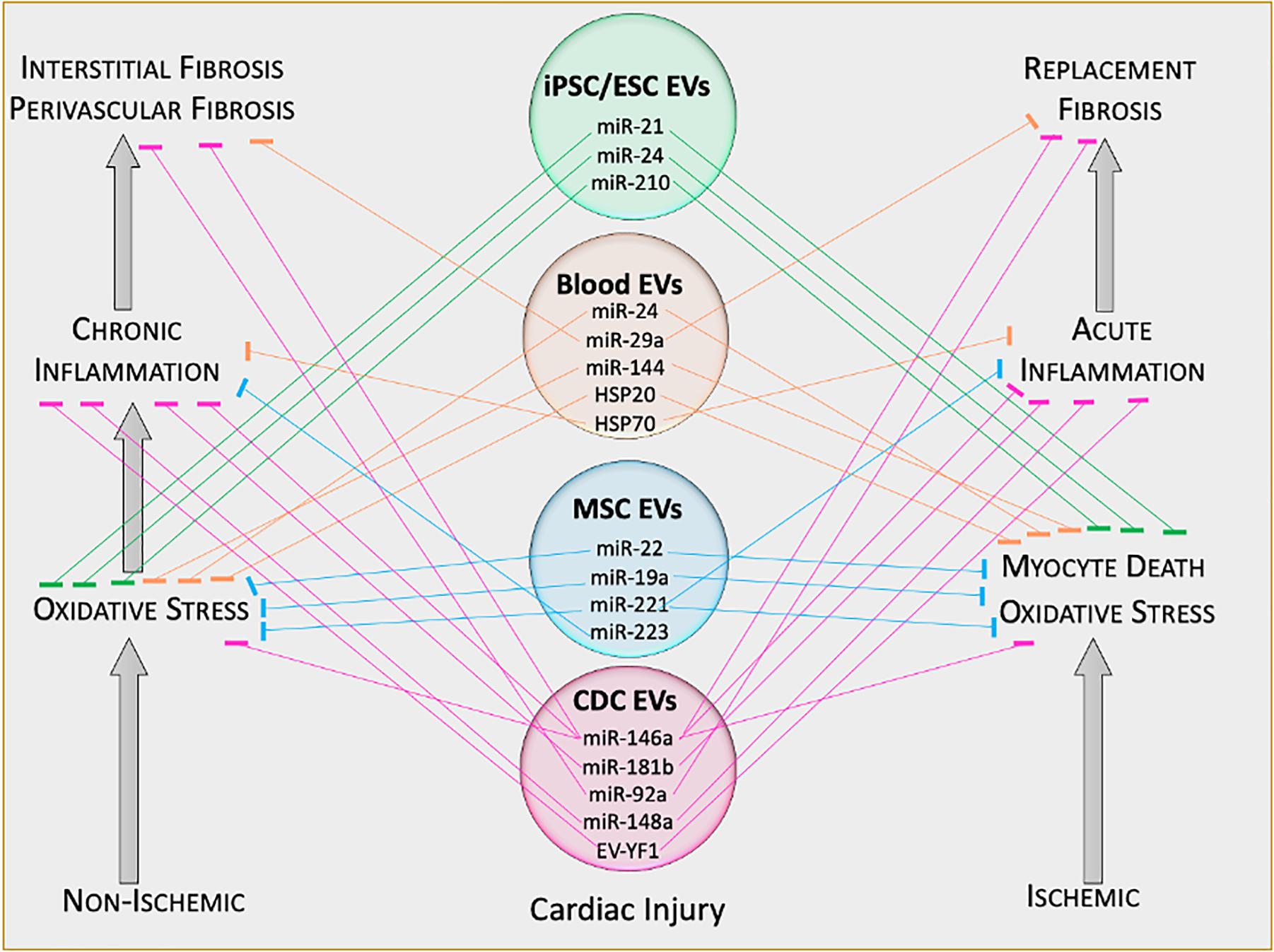
Figure 4. Therapeutic functions of extracellular vesicle cargo. Schematic of therapeutically active cargo found inside extracellular vesicles. Defined molecules exert specific biological functions in target tissues. EV, extracellular vesicle; ESC, embryonic stem cell; iPSC, induced pluripotent stem cell; miR, microRNA; EV-YF1, extracellular vesicle Y-RNA fragment.
Production of EVs for Therapeutic Applications
Therapeutic exosomes are of great potential interest, but it should be noted that there are no FDA-approved EV products. Nevertheless, some scholarship on the topic of isolating such exosomes has appeared (for example, Marbán, 2018b). Briefly, we start by culturing therapeutically potent cells in vitro in serum-free media (to exclude contaminating exosomes naturally found in serum), and allowing the cells to condition the media. The conditioning phase is variable and can be as brief as 24 h or as long as 15 days under normoxic or hypoxic conditions. Varying conditioning phase parameters can influence cargo loading into exosomes, which may impact their disease-modifying bioactivity. Conditioned media contains not only exosomes but also other EVs, proteins, and products of metabolism. To remove non-EV components, further processing by laboratory personnel typically involves ultrafiltration (i.e., by molecular weight exclusion). In order to separate exosomes from larger EVs such as microvesicles or apoptosomes, ultracentrifugation or size exclusion chromatography may be used (Baranyai et al., 2015). Finally, the purified product is aliquoted into dosage-defined units and packaged into vials for later use.
Cardiosphere-Derived Cell EVs
Cardiosphere-derived cells (CDCs) are stromal cells of intrinsic cardiac origin (White et al., 2013), and are multipotent and clonogenic (but not self-renewing; Davis et al., 2009). CDCs uniformly express CD105 and are negative for CD45 and other hematogenous markers. Since the isolation of CDCs was first reported in 2007 (Smith et al., 2007), >200 papers have been published using this cell type from >55 independent labs worldwide. CDCs secrete EVs (CDC-EVs) which transfer payloads into target cells, inducing epigenomic, transcriptomic, and phenotypic changes that underlie the benefits of CDC therapy (Marbán, 2018b). Indeed, therapeutic bioactivity by CDC-EVs has been demonstrated in several animal studies such as acute MI (Ibrahim et al., 2014; De Couto et al., 2017; Gallet et al., 2017), non-ischemic cardiomyopathy (Aminzadeh et al., 2015), and Duchenne muscular dystrophy (DMD)-related cardiomyopathy (Aminzadeh et al., 2018; Rogers et al., 2019b). In preclinical studies, CDC-EVs induce cardiomyogenesis and angiogenesis, reduce fibrosis, modulate the immune response, and generally improve cardiac function (Marbán, 2018b). The mechanism of benefit appears to hinge on their cargo, particularly non-coding ribonucleic acids (ncRNA). Of the numerous RNA species, microRNAs (miRs) are the best-described class of small ncRNA.
Several CDC-EV associated miRs impact on inflammation and fibrosis including miR-146a (Barile et al., 2014; Ibrahim et al., 2014), miR-210 (Barile et al., 2014), miR-181b (De Couto et al., 2017), miR-148a (Aminzadeh et al., 2018), and miR-92a (Ibrahim et al., 2019). miR-146a targets multiple pathways active in cardiac disease including inflammation and fibrosis. Indeed, miR-146a regulates NFκB (Saba et al., 2014) and TGFβ signaling (Geraldo et al., 2012). Specifically, miR-146a inhibits Smad4-mediated myofibroblast differentiation to attenuate myocardial fibrosis (Liu et al., 2012). Delivery of a miR-146a mimic in infarcted mouse hearts reproduced some, but not all the benefits observed with CDC-EV treatment, suggesting cooperative effects of other CDC-EV cargo in the overall benefits. Moreover, it is unclear if other CDC-EV miRs share overlapping or synergistic bioactivity. For example, miR-181b blunts pro-inflammatory cytokine production by targeting protein kinase Cδ in a rat model of acute MI (De Couto et al., 2017), while miR-148a attenuates NFκB phosphorylation in the mdx mouse model of DMD (Aminzadeh et al., 2018; Rogers et al., 2019b). We have recently demonstrated CDC-EVs modulate the mdx mouse macrophage toward a pro-regenerative phenotype with prominent secretion of tissue inhibitor of matrixmetalloproteinase 2 (TIMP-2; Rogers et al., 2019a). We speculate that enhanced TIMP-2 secretion may contribute to the anti-fibrotic effect of CDC-EVs. In TIMP-2 null mice, MI led to greater ventricular dilation and infarct expansion (Kandalam et al., 2010). In contrast, TIMP-2 overexpression reduced ventricular dilation and infarct expansion post-MI (Ramani et al., 2011), and TIMP-2 inhibits human fibroblast activation at high concentrations (Ngu et al., 2014).
In addition to miRs, other less described ncRNAs, such as Y-RNAs, have been shown to influence the transcriptome in cardiac tissue. Y-RNAs are components of the Ro60 ribonucleoproteins and play a key role in DNA replication by interacting with chromatin and replication initiation proteins (Christov et al., 2006; Zhang et al., 2011). EV-YF1, a Y-RNA fragment found abundantly in CDC-EVs, skews macrophage polarization toward a pro-regenerative phenotype, which provides cardioprotection against ischemia/reperfusion injury in rats (Cambier et al., 2017). Moreover, in a mouse model of angiotensin-induced hypertrophic cardiomyopathy, EV-YF1 treatment attenuated myocardial hypertrophy, inflammation, and fibrosis, which were mediated by IL-10 (Cambier et al., 2018). While much work has focused on well-known ncRNAs such as miRs, it is clear that other RNA species contained within EVs are therapeutically bioactive. Further work will be required to better understand the role other lesser-known ncRNAs play in the mechanistic basis of EV-based therapeutics.
Mesenchymal Stem Cell-Derived EVs
MSCs are multipotent stem/stromal cells found in loose connective tissue (e.g., areolar, reticular, and adipose), bone marrow, and lymph tissue (Bianco et al., 2008; Choi et al., 2019). While initial enthusiasm regarding MSCs originated from their capacity to differentiate into various cell types, it is now widely accepted that their engraftment and differentiation are negligible, and do not account for the therapeutic effects of MSC infusions (Iso et al., 2007; Leiker et al., 2008; Keating, 2012; Tokita et al., 2016). In congruency with CDCs (though a distinctly different cell type), MSCs mediate their disease-modifying bioactivity through the secretion of paracrine factors including EVs (MSC-EVs). Moreover, like CDC-EVs, MSC-EVs contain a plethora of RNA species, including miRs. For example, MSC-EVs from bone marrow-derived MSCs are enriched with miR-22, which confers anti-apoptotic and anti-fibrotic properties in a mouse model of acute MI (Feng et al., 2014). Further, cardioprotective miRs miR-19a and miR-221 are commonly found in MSC-EVs (Yu et al., 2013; Yu et al., 2015; Shi et al., 2018). Unlike MSCs, MSC-EVs are said to have no risk of tumorigenicity or extraosseous calcification, and a lower possibility of immune rejection following in vivo allogeneic administration (Stoltz et al., 2015). These features further support the notion of EVs as next-generation therapeutic candidates.
Embryonic Stem Cell- and Induced Pluripotent Stem Cell-Derived EVs
Embryonic stem cells (ESCs) and induced pluripotent stem cells (iPSCs), like CDCs and MSCs, secrete bioactive EVs against cardiac injury and fibrosis, although they are traditionally believed to work by engraftment and differentiation rather than by paracrine mechanisms (Marbán, 2018a). ESC-EVs are enriched in the miR-290/295 cluster; one member, miR-294, promotes cardiomyocyte survival and attenuates fibrosis in MI (Khan et al., 2015). Similarly, iPSC-EVs have also demonstrated cardioprotective properties, mediated in part by miR-21 and miR-210 (Wang et al., 2015).
Peripheral Blood-Derived EVs
Secreted mostly by platelets and the vascular endothelium, peripheral blood-derived EVs (PB-EVs) exhibit tissue-protective bioactivity. PB-EVs from healthy humans or rodents exert anti-oxidant (Vicencio et al., 2015), anti-apoptotic (Minghua et al., 2018), and anti-fibrotic (Yamaguchi et al., 2015) effects in animal models of MI. These therapeutic benefits appear to be, in part, mediated by miRs. The anti-fibrotic effects appear to be largely driven by miR-29, which has been validated to target col1a1, col1a2, col3a1, fbn1, and eln1 transcripts (Van Rooij et al., 2008). Moreover, PB-EVs were demonstrated to attenuate fibrosis and cardiac dysfunction in a streptozotocin-induced diabetic cardiomyopathy model, which was mediated, in part, by HSP20 (Wang et al., 2016).
The Promise of Extracellular Vesicle Engineering for Next-Generation Therapeutics
The nature and the physiological state of the EV-secreting cell affects the tropism and therapeutic bioactivity of the produced vesicles (Wiklander et al., 2015; Lai et al., 2016). In the circulation, the balance between EV production and clearance reflects the steady-state level. Upon systemic delivery, the detection window of labeled EVs is typically very short (Yáñez-Mó et al., 2015). Generally speaking, EVs are distributed to many body tissues including the liver, bone, skin, muscle, spleen, kidney, and lung. EV-specific expression of selective adhesion molecules may influence biodistribution. For example, CD169-expressing macrophages in the spleen and lymph nodes capture B cell-derived EVs. In contrast, EV trafficking to the lymphoid system is dysregulated in CD169 knockout mice (Saunderson et al., 2014). Other insights into cell-specific EV uptake indicate the potential influence of saccharides. In the presence of D-mannose or D-glucosamine, EV uptake by dendritic cells was blunted, suggesting an EV uptake mechanism based on C-type lectin interaction (Hao et al., 2007). However, other studies have demonstrated sugars do not seem to play a significant role in EV-cell interaction and uptake (Escrevente et al., 2011), suggesting cell- or condition-specific difference in EV uptake mechanisms.
Given the identification of EV-derived biomolecules that mediate many of the therapeutic benefits associated with EV therapy, selectively loading EVs with defined biomolecules is one goal which can be achieved using various approaches reviewed elsewhere (Kenari et al., 2020). Briefly, a non-invasive method for loading therapeutic molecules into EVs is via co-incubation (Zhuang et al., 2011). This method allows hydrophobic biomolecules to enter the EV lumen through passive diffusion without disrupting the lipid membrane. Other methods include, but not limited to, electroporation or sonication (Tian et al., 2014; Kim et al., 2016). Although these methods have been successfully used to load exogenous biomolecules into EVs, they disrupt the lipid membrane and may result in EV damage or lysis. Further research will be required to determine if these are viable methods to produce clinical-grade therapeutic EVs. In addition to loading defined molecules into EVs, engineering their delivery to specific target tissues would be a significant enhancement to such a therapeutic. As such, we have recently developed a platform using membrane cloaking and surface display technology to direct EVs to target tissues. Cloaking lends itself to utilizing any biotinylated antibody – to which countless are commercially available for testing. For example, we previously demonstrated that CDC-EV uptake by cardiac fibroblasts, which is ordinarily minimal, could be augmented in vitro by ligating a DDR2 antibody (Antes et al., 2018). Addition of an ischemic targeting peptide to the surface of CDC-EV conferred enhanced in vivo targeting to the myocardium. Such an advent may provide significant therapeutic value to target a major cell source contributing to the development of myocardial fibrosis.
Conclusion
Extracellular vesicles represent a mode of intercellular communication near and far. These tiny vesicles carry messages in the form of biomolecules to inform recipient cells of the current (patho)physiological state and direct the cell to respond appropriately. The recognition that EVs secreted from stem/progenitor cells are therapeutically bioactive when given to animals with heart disease represents a paradigm shift away from cell therapy toward a cell-free platform. Such a paradigm shift overcomes many key concerns and limitations of cell therapy, while conferring the amendable ability to modify vesicle cargo and tissue targeting. Indeed, we and others have demonstrated that payload RNAs, notably miRs, long non-coding RNAs, Y-RNAs, and piRNA, produce transcriptomic and epigenomic modifications that impart lasting effects on recipient cells. By targeting key cellular and molecular players in cardiac fibrosis, these defined molecules contained within therapeutically potent EVs provide insight into avenues for bioengineering. Time will certainly tell if EV-based therapeutics live up to their promise.
Author Contributions
RR drafted the manuscript. AI and AC generated the figures. EM approved the final version. All authors helped to write the manuscript.
Funding
Support for this research program was provided by NHLBI/NIH and the U.S. Department of Defense. EM holds the Mark S. Siegel Family Distinguished Chair of the Cedars-Sinai Medical Center.
Conflict of Interest
EM is a founding equity share-holder of Capricor Therapeutics.
The remaining authors declare that the research was conducted in the absence of any commercial or financial relationships that could be construed as a potential conflict of interest.
Footnotes
References
Akers, J. C., Ramakrishnan, V., Yang, I., Hua, W., Mao, Y., Carter, B. S., et al. (2016). Optimizing preservation of extracellular vesicular miRNAs derived from clinical cerebrospinal fluid. Cancer Biomark. 17, 125–132. doi: 10.3233/CBM-160609
Alibhai, F. J., Tobin, S. W., Yeganeh, A., Weisel, R. D., and Li, R.-K. (2018). Emerging roles of extracellular vesicles in cardiac repair and rejuvenation. Am. J. Physiol. Heart Circul. Physiol. 315, H733–H744. doi: 10.1152/ajpheart.00100.2018
Aminzadeh, M. A., Rogers, R. G., Fournier, M., Tobin, R. E., Guan, X., Childers, M. K., et al. (2018). Exosome-mediated benefits of cell therapy in mouse and human models of duchenne muscular dystrophy. Stem Cell Rep. 10, 942–955. doi: 10.1016/j.stemcr.2018.01.023
Aminzadeh, M. A., Tseliou, E., Sun, B., Cheng, K., Malliaras, K., Makkar, R. R., et al. (2015). Therapeutic efficacy of cardiosphere-derived cells in a transgenic mouse model of non-ischaemic dilated cardiomyopathy. Eur. Heart J. 36, 751–762. doi: 10.1093/eurheartj/ehu196
Antes, T. J., Middleton, R. C., Luther, K. M., Ijichi, T., Peck, K. A., Liu, W. J., et al. (2018). Targeting extracellular vesicles to injured tissue using membrane cloaking and surface display. J. Nanobiotechnol. 16, 1–15. doi: 10.1186/s12951-018-0388-4
Balbi, C., Costa, A., Barile, L., and Bollini, S. (2020). Message in a bottle: upgrading cardiac repair into rejuvenation. Cells 9:724. doi: 10.3390/cells9030724
Baranyai, T., Herczeg, K., Onódi, Z., Voszka, I., Módos, K., Marton, N., et al. (2015). Isolation of exosomes from blood plasma: qualitative and quantitative comparison of ultracentrifugation and size exclusion chromatography methods. PLoS One 10:e0145686. doi: 10.1371/journal.pone.0145686
Barile, L., Lionetti, V., Cervio, E., Matteucci, M., Gherghiceanu, M., Popescu, L. M., et al. (2014). Extracellular vesicles from human cardiac progenitor cells inhibit cardiomyocyte apoptosis and improve cardiac function after myocardial infarction. Cardiovasc. Res. 103, 530–541. doi: 10.1093/cvr/cvu167
Barile, L., Moccetti, T., Marbán, E., and Vassalli, G. (2017). Roles of exosomes in cardioprotection. Eur. Heart J. 38, 1372–1379.
Berk, B. C., Fujiwara, K., and Lehoux, S. (2007). ECM remodeling in hypertensive heart disease. J. Clin. Invest. 117, 568–575.
Bianco, P., Robey, P. G., and Simmons, P. J. (2008). Mesenchymal stem cells: revisiting history, concepts, and assays. Cell Stem Cell 2, 313–319. doi: 10.1016/j.stem.2008.03.002
Bobrie, A., Colombo, M., Krumeich, S., Raposo, G., and Théry, C. (2012). Diverse subpopulations of vesicles secreted by different intracellular mechanisms are present in exosome preparations obtained by differential ultracentrifugation. J. Extracell. Ves. 1:18397. doi: 10.3402/jev.v1i0.18397
Cai, H., Reinisch, K., and Ferro-Novick, S. (2007). Coats, tethers, Rabs, and SNAREs work together to mediate the intracellular destination of a transport vesicle. Dev. Cell 12, 671–682. doi: 10.1016/j.devcel.2007.04.005
Cambier, L., De Couto, G., Ibrahim, A., Echavez, A. K., Valle, J., Liu, W., et al. (2017). Y RNA fragment in extracellular vesicles confers cardioprotection via modulation of IL-10 expression and secretion. EMBO Mol. Med. 9, 337–352. doi: 10.15252/emmm.201606924
Cambier, L., Giani, J. F., Liu, W., Ijichi, T., Echavez, A. K., Valle, J., et al. (2018). Angiotensin II-induced end-organ damage in mice is attenuated by human exosomes and by an exosomal Y RNA fragment. Hypertension 72, 370–380. doi: 10.1161/HYPERTENSIONAHA.118.11239
Cheng, Z., Zhou, Y. Z., Wu, Y., Wu, Q. Y., Liao, X. B., Fu, X. M., et al. (2018). Diverse roles of macrophage polarization in aortic aneurysm: destruction and repair. J. Transl. Med. 16:354. doi: 10.1186/s12967-018-1731-0
Choi, Y. J., Koo, J. B., Kim, H. Y., Seo, J. W., Lee, E. J., Kim, W. R., et al. (2019). Umbilical cord/placenta-derived mesenchymal stem cells inhibit fibrogenic activation in human intestinal myofibroblasts via inhibition of myocardin-related transcription factor A. Stem Cell Res. Ther. 10, 1–16. doi: 10.1186/s13287-019-1385-8
Christov, C. P., Gardiner, T. J., Szuts, D., and Krude, T. (2006). Functional requirement of noncoding Y RNAs for human chromosomal DNA replication. Mol. Cell. Biol. 26, 6993–7004. doi: 10.1128/MCB.01060-06
Colombo, M., Raposo, G., and Théry, C. (2014). Biogenesis, secretion, and intercellular interactions of exosomes and other extracellular vesicles. Annu. Rev. Cell Dev. Biol. 30, 255–289. doi: 10.1146/annurev-cellbio-101512-122326
Conlan, R. S., Pisano, S., Oliveira, M. I., Ferrari, M., and Pinto, I. M. (2017). Exosomes as reconfigurable therapeutic systems. Trends Mol. Med. 23, 636–650. doi: 10.1016/j.molmed.2017.05.003
Davis, D. R., Zhang, Y., Smith, R. R., Cheng, K., Terrovitis, J., Malliaras, K., et al. (2009). Validation of the cardiosphere method to culture cardiac progenitor cells from myocardial tissue. PLoS One 4:e7195. doi: 10.1371/journal.pone.07195
De Couto, G., Gallet, R., Cambier, L., Jaghatspanyan, E., Makkar, N., Dawkins, J. F., et al. (2017). Exosomal MicroRNA transfer into macrophages mediates cellular postconditioning. Circulation 136, 200–214. doi: 10.1161/CIRCULATIONAHA.117.031555
Dodson, B. P., and Levine, A. D. (2015). Challenges in the translation and commercialization of cell therapies. BMC Biotechnol. 15:70. doi: 10.1186/s12896-015-0190-4
Escrevente, C., Keller, S., Altevogt, P., and Costa, J. (2011). Interaction and uptake of exosomes by ovarian cancer cells. BMC Cancer 11:108. doi: 10.1186/1471-2407-11-108
Feng, Y., Huang, W., Wani, M., Yu, X., and Ashraf, M. (2014). Ischemic preconditioning potentiates the protective effect of stem cells through secretion of exosomes by targeting Mecp2 via miR-22. PLoS One 9:e88685. doi: 10.1371/journal.pone.088685
Frangogiannis, N. G. (2019). Cardiac fibrosis: cell biological mechanisms, molecular pathways and therapeutic opportunities. Mol. Aspects Med. 65, 70–99. doi: 10.1016/j.mam.2018.07.001
Gallet, R., Dawkins, J., Valle, J., Simsolo, E., De Couto, G., Middleton, R., et al. (2017). Exosomes secreted by cardiosphere-derived cells reduce scarring, attenuate adverse remodelling, and improve function in acute and chronic porcine myocardial infarction. Eur. Heart J. 38, 201–211. doi: 10.1093/eurheartj/ehw240
Geraldo, M. V., Yamashita, A. S., and Kimura, E. T. (2012). MicroRNA miR-146b-5p regulates signal transduction of TGF-beta by repressing SMAD4 in thyroid cancer. Oncogene 31, 1910–1922. doi: 10.1038/onc.2011.381
Hao, S., Bai, O., Li, F., Yuan, J., Laferte, S., and Xiang, J. (2007). Mature dendritic cells pulsed with exosomes stimulate efficient cytotoxic T-lymphocyte responses and antitumour immunity. Immunology 120, 90–102. doi: 10.1111/j.1365-2567.2006.02483.x
He, C., Zheng, S., Luo, Y., and Wang, B. (2018). Exosome theranostics: biology and translational medicine. Theranostics 8:237. doi: 10.7150/thno.21945
Ibrahim, A., and Marbán, E. (2016). Exosomes: fundamental biology and roles in cardiovascular physiology. Annu. Rev. Physiol. 78, 67–83. doi: 10.1146/annurev-physiol-021115-104929
Ibrahim, A. G., Cheng, K., and Marban, E. (2014). Exosomes as critical agents of cardiac regeneration triggered by cell therapy. Stem Cell Rep. 2, 606–619. doi: 10.1016/j.stemcr.2014.04.006
Ibrahim, A. G. E., Li, C., Rogers, R., Fournier, M., Li, L., Vaturi, S. D., et al. (2019). Augmenting canonical Wnt signalling in therapeutically inert cells converts them into therapeutically potent exosome factories. Nat. Biomed. Eng. 3, 695–705. doi: 10.1038/s41551-019-0448-6
Iso, Y., Spees, J. L., Serrano, C., Bakondi, B., Pochampally, R., Song, Y.-H., et al. (2007). Multipotent human stromal cells improve cardiac function after myocardial infarction in mice without long-term engraftment. Biochem. Biophys. Res. Commun. 354, 700–706. doi: 10.1016/j.bbrc.2007.01.045
Jameel, M. N., and Zhang, J. (2009). Heart failure management: the present and the future. Antioxid. Redox Signal. 11, 1989–2010.
Johnstone, R. M., Adam, M., Hammond, J., Orr, L., and Turbide, C. (1987). Vesicle formation during reticulocyte maturation. Association of plasma membrane activities with released vesicles (exosomes). J. Biol. Chem. 262, 9412–9420.
Kandalam, V., Basu, R., Abraham, T., Wang, X., Soloway, P. D., Jaworski, D. M., et al. (2010). TIMP2 deficiency accelerates adverse post-myocardial infarction remodeling because of enhanced MT1-MMP activity despite lack of MMP2 activation. Circ. Res. 106:796. doi: 10.1161/CIRCRESAHA.109.209189
Keating, A. (2012). Mesenchymal stromal cells: new directions. Cell Stem Cell 10, 709–716. doi: 10.1016/j.stem.2012.05.015
Kenari, A. N., Cheng, L., and Hill, A. F. (2020). Methods for loading therapeutics into extracellular vesicles and generating extracellular vesicles mimetic-nanovesicles. Methods 177, 103–113.
Khalil, H., Kanisicak, O., Prasad, V., Correll, R. N., Fu, X., Schips, T., et al. (2017). Fibroblast-specific TGF-beta-Smad2/3 signaling underlies cardiac fibrosis. J. Clin. Invest. 127, 3770–3783. doi: 10.1172/JCI94753
Khan, M., Nickoloff, E., Abramova, T., Johnson, J., Verma, S. K., Krishnamurthy, P., et al. (2015). Embryonic stem cell-derived exosomes promote endogenous repair mechanisms and enhance cardiac function following myocardial infarction. Circ. Res. 117, 52–64. doi: 10.1161/CIRCRESAHA.117.305990
Kim, M. S., Haney, M. J., Zhao, Y., Mahajan, V., Deygen, I., Klyachko, N. L., et al. (2016). Development of exosome-encapsulated paclitaxel to overcome MDR in cancer cells. Nanomedicine 12, 655–664. doi: 10.1016/j.nano.2015.10.012
Kong, P., Christia, P., and Frangogiannis, N. G. (2014). The pathogenesis of cardiac fibrosis. Cell Mol. Life. Sci. 71, 549–574.
Krzyszczyk, P., Schloss, R., Palmer, A., and Berthiaume, F. (2018). The role of macrophages in acute and chronic wound healing and interventions to promote pro-wound healing phenotypes. Front. Physiol. 9:419. doi: 10.3389/fphys.2018.00419
Lai, R. C., Arslan, F., Lee, M. M., Sze, N. S. K., Choo, A., Chen, T. S., et al. (2010). Exosome secreted by MSC reduces myocardial ischemia/reperfusion injury. Stem Cell Res. 4, 214–222. doi: 10.1016/j.scr.2009.12.003
Lai, R. C., Tan, S. S., Yeo, R. W. Y., Choo, A. B. H., Reiner, A. T., Su, Y., et al. (2016). MSC secretes at least 3 EV types each with a unique permutation of membrane lipid, protein and RNA. J. Extracell. Ves. 5:29828. doi: 10.3402/jev.v5.29828
Legrand, A. J., Konstantinou, M., Goode, E. F., and Meier, P. (2019). The diversification of cell death and immunity: memento mori. Mol. Cell. 76, 232–242. doi: 10.1016/j.molcel.2019.09.006
Leiker, M., Suzuki, G., Iyer, V. S., John, M., and Lee, T. (2008). Assessment of a nuclear affinity labeling method for tracking implanted mesenchymal stem cells. Cell Transpl. 17, 911–922. doi: 10.3727/096368908786576444
Liu, Z., Lu, C.-L., Cui, L.-P., Hu, Y.-L., Yu, Q., Jiang, Y., et al. (2012). MicroRNA-146a modulates TGF-β1-induced phenotypic differentiation in human dermal fibroblasts by targeting SMAD4. Arch. Dermatol. Res. 304, 195–202. doi: 10.1007/s00403-011-1178-0
Ma, F., Li, Y., Jia, L., Han, Y., Cheng, J., Li, H., et al. (2012). Macrophage-stimulated cardiac fibroblast production of IL-6 is essential for TGF β/Smad activation and cardiac fibrosis induced by angiotensin II. PLoS One 7:e35144. doi: 10.1371/journal.pone.0035144
Marbán, E. (2018a). A mechanistic roadmap for the clinical application of cardiac cell therapies. Nat. Biomed. Eng. 2, 353–361. doi: 10.1038/s41551-018-0216-z
Marbán, E. (2018b). The secret life of exosomes: what bees can teach us about next-generation therapeutics. J. Am. Coll. Cardiol. 71, 193–200. doi: 10.1016/j.jacc.2017.11.013
Marzesco, A.-M., Janich, P., Wilsch-Bräuninger, M., Dubreuil, V., Langenfeld, K., Corbeil, D., et al. (2005). Release of extracellular membrane particles carrying the stem cell marker prominin-1 (CD133) from neural progenitors and other epithelial cells. J. Cell Sci. 118, 2849–2858. doi: 10.1242/jcs.02439
Members, A. T. F., Mcmurray, J. J., Adamopoulos, S., Anker, S. D., Auricchio, A., Böhm, M., et al. (2012). ESC guidelines for the diagnosis and treatment of acute and chronic heart failure 2012: the task force for the diagnosis and treatment of acute and chronic heart failure 2012 of the european society of cardiology. developed in collaboration with the heart failure association (HFA) of the ESC. Eur. J. Heart Fail. 14, 803–869. doi: 10.1093/eurjhf/hfs105
Minghua, W., Zhijian, G., Chahua, H., Qiang, L., Minxuan, X., Luqiao, W., et al. (2018). Plasma exosomes induced by remote ischaemic preconditioning attenuate myocardial ischaemia/reperfusion injury by transferring miR-24. Cell Death Dis. 9:320. doi: 10.1038/s41419-018-0274-x
Mohammadi, M. R., Riazifar, M., Pone, E. J., Yeri, A., Van Keuren-Jensen, K., Lässer, C., et al. (2019). Isolation and characterization of microvesicles from mesenchymal stem cells. Methods 177, 50–57. doi: 10.1016/j.ymeth.2019.10.010
Nevers, T., Salvador, A. M., Grodecki-Pena, A., Knapp, A., Velazquez, F., Aronovitz, M., et al. (2015). Left ventricular T-cell recruitment contributes to the pathogenesis of heart failure. Circ. Heart Fail. 8, 776–787. doi: 10.1161/CIRCHEARTFAILURE.115.002225
Ngu, J. M., Teng, G., Meijndert, H. C., Mewhort, H. E., Turnbull, J. D., Stetler-Stevenson, W. G., et al. (2014). Human cardiac fibroblast extracellular matrix remodeling: dual effects of tissue inhibitor of metalloproteinase-2. Cardiovasc. Pathol. 23, 335–343. doi: 10.1016/j.carpath.2014.06.003
Nigro, P., Bassetti, B., Cavallotti, L., Catto, V., Carbucicchio, C., and Pompilio, G. (2018). Cell therapy for heart disease after 15 years: unmet expectations. Pharmacol. Res. 127, 77–91. doi: 10.1016/j.phrs.2017.02.015
Panayi, G. S., Corrigall, V. M., and Henderson, B. (2004). Stress cytokines: pivotal proteins in immune regulatory networks. Opinion. Curr. Opin. Immunol. 16, 531–534. doi: 10.1016/j.coi.2004.05.017
Pardali, E., Sanchez-Duffhues, G., Gomez-Puerto, M. C., and Ten Dijke, P. (2017). TGF-beta-induced endothelial-mesenchymal transition in fibrotic diseases. Int. J. Mol. Sci. 18:2157. doi: 10.3390/ijms18102157
Patel, B., Bansal, S. S., Ismahil, M. A., Hamid, T., Rokosh, G., Mack, M., et al. (2018). CCR2(+) monocyte-derived infiltrating macrophages are required for adverse cardiac remodeling during pressure overload. JACC Basic Transl. Sci. 3, 230–244. doi: 10.1016/j.jacbts.2017.12.006
Ramani, R., Nilles, K., Gibson, G., Burkhead, B., Mathier, M., Mcnamara, D., et al. (2011). Tissue inhibitor of metalloproteinase-2 gene delivery ameliorates postinfarction cardiac remodeling. Clin. Transl. Sci. 4, 24–31. doi: 10.1111/j.1752-8062.2010.00252.x
Roers, A., Hiller, B., and Hornung, V. (2016). Recognition of endogenous nucleic acids by the innate immune system. Immunity 44, 739–754. doi: 10.1016/j.immuni.2016.04.002
Rogers, R. G., De Couto, G., Liu, W., Sanchez, L., and Marban, E. (2019a). Cardiosphere-derived cell exosomes modulate mdx macrophage phenotype and alter their secretome. FASEB J. 33, lb611–lb611.
Rogers, R. G., Fournier, M., Sanchez, L., Ibrahim, A. G., Aminzadeh, M. A., Lewis, M. I., et al. (2019b). Disease-modifying bioactivity of intravenous cardiosphere-derived cells and exosomes in mdx mice. JCI Insight 4:e130202. doi: 10.1172/jci.insight.130202
Rogers, R. G., and Otis, J. S. (2017). Resveratrol-mediated expression of KLF15 in the ischemic myocardium is associated with an improved cardiac phenotype. Cardiovasc. Drugs. Ther. 31, 29–38. doi: 10.1007/s10557-016-6707-9
Roth, G. A., Johnson, C., Abajobir, A., Abd-Allah, F., Abera, S. F., Abyu, G., et al. (2017). Global, regional, and national burden of cardiovascular diseases for 10 causes, 1990 to 2015. J. Am. Coll. Cardiol. 70, 1–25. doi: 10.1016/j.jacc.2017.04.052
Rubartelli, A., and Lotze, M. T. (2007). Inside, outside, upside down: damage-associated molecular-pattern molecules (DAMPs) and redox. Trends Immunol. 28, 429–436. doi: 10.1016/j.it.2007.08.004
Saba, R., Sorensen, D. L., and Booth, S. A. (2014). MicroRNA-146a: a dominant, negative regulator of the innate immune response. Front. Immunol. 5:578. doi: 10.3389/fimmu.2014.00578
Sahoo, S., Klychko, E., Thorne, T., Misener, S., Schultz, K. M., Millay, M., et al. (2011). Exosomes from human CD34+ stem cells mediate their proangiogenic paracrine activity. Circ. Res. 109, 724–728. doi: 10.1161/CIRCRESAHA.111.253286
Saunderson, S. C., Dunn, A. C., Crocker, P. R., and Mclellan, A. D. (2014). CD169 mediates the capture of exosomes in spleen and lymph node. Blood J. Am. Soc. Hematol. 123, 208–216. doi: 10.1182/blood-2013-03-489732
Scaffidi, P., Misteli, T., and Bianchi, M. E. (2002). Release of chromatin protein HMGB1 by necrotic cells triggers inflammation. Nature 418, 191–195. doi: 10.1038/nature00858
Sharma, K., and Kass, D. A. (2014). Heart failure with preserved ejection fraction: mechanisms, clinical features, and therapies. Circ. Res. 115, 79–96. doi: 10.1161/CIRCRESAHA.115.302922
Shi, B., Wang, Y., Zhao, R., Long, X., Deng, W., and Wang, Z. (2018). Bone marrow mesenchymal stem cell-derived exosomal miR-21 protects C-kit+ cardiac stem cells from oxidative injury through the PTEN/PI3K/Akt axis. PLoS One 13:e0191616. doi: 10.1371/journal.pone.0191616
Silva, A. M., Teixeira, J. H., Almeida, M. I., Goncalves, R. M., Barbosa, M. A., and Santos, S. G. (2017). Extracellular vesicles: immunomodulatory messengers in the context of tissue repair/regeneration. Eur. J. Pharm. Sci. 98, 86–95. doi: 10.1016/j.ejps.2016.09.017
Sluijter, J. P. G., Davidson, S. M., Boulanger, C. M., Buzas, E. I., De Kleijn, D. P. V., Engel, F. B., et al. (2018). Extracellular vesicles in diagnostics and therapy of the ischaemic heart: position paper from the working group on cellular biology of the heart of the european society of cardiology. Cardiovasc. Res. 114, 19–34. doi: 10.1093/cvr/cvx211
Smith, R. R., Barile, L., Cho, H. C., Leppo, M. K., Hare, J. M., Messina, E., et al. (2007). Regenerative potential of cardiosphere-derived cells expanded from percutaneous endomyocardial biopsy specimens. Circulation 115, 896–908. doi: 10.1161/CIRCULATIONAHA.106.655209
Stahl, P. D., and Raposo, G. (2018). Exosomes and extracellular vesicles: the path forward. Essays Biochem. 62, 119–124. doi: 10.1042/EBC20170088
Stoltz, J.-F., De Isla, N., Li, Y., Bensoussan, D., Zhang, L., Huselstein, C., et al. (2015). Stem cells and regenerative medicine: myth or reality of the 21th century. Stem Cells Intern. 2015:734731. doi: 10.1155/2015/734731
Tang, D., Kang, R., Coyne, C. B., Zeh, H. J., and Lotze, M. T. (2012). PAMPs and DAMPs: signal 0s that spur autophagy and immunity. Immunol. Rev. 249, 158–175. doi: 10.1111/j.1600-065X.2012.01146.x
Tian, Y., Li, S., Song, J., Ji, T., Zhu, M., Anderson, G. J., et al. (2014). A doxorubicin delivery platform using engineered natural membrane vesicle exosomes for targeted tumor therapy. Biomaterials 35, 2383–2390. doi: 10.1016/j.biomaterials.2013.11.083
Tikhomirov, R., Donnell, B. R.-O., Catapano, F., Faggian, G., Gorelik, J., Martelli, F., et al. (2020). Exosomes: from potential culprits to new therapeutic promise in the setting of cardiac fibrosis. Cells 9:592. doi: 10.3390/cells9030592
Tokita, Y., Tang, X.-L., Li, Q., Wysoczynski, M., Hong, K. U., Nakamura, S., et al. (2016). Repeated administrations of cardiac progenitor cells are markedly more effective than a single administration: a new paradigm in cell therapy. Circ. Res. 119, 635–651. doi: 10.1161/CIRCRESAHA.116.308937
Van Rooij, E., Sutherland, L. B., Thatcher, J. E., Dimaio, J. M., Naseem, R. H., Marshall, W. S., et al. (2008). Dysregulation of microRNAs after myocardial infarction reveals a role of miR-29 in cardiac fibrosis. Proc. Natl. Acad. Sci. U.S.A. 105, 13027–13032. doi: 10.1073/pnas.0805038105
Vicencio, J. M., Yellon, D. M., Sivaraman, V., Das, D., Boi-Doku, C., Arjun, S., et al. (2015). Plasma exosomes protect the myocardium from ischemia-reperfusion injury. J. Am. Coll. Cardiol. 65, 1525–1536. doi: 10.1016/j.jacc.2015.02.026
Wang, X., Gu, H., Huang, W., Peng, J., Li, Y., Yang, L., et al. (2016). Hsp20-mediated activation of exosome biogenesis in cardiomyocytes improves cardiac function and angiogenesis in diabetic mice. Diabetes Metab. Res. Rev. 65, 3111–3128. doi: 10.2337/db15-1563
Wang, Y., Zhang, L., Li, Y., Chen, L., Wang, X., Guo, W., et al. (2015). Exosomes/microvesicles from induced pluripotent stem cells deliver cardioprotective miRNAs and prevent cardiomyocyte apoptosis in the ischemic myocardium. Int. J. Cardiol. 192, 61–69. doi: 10.1016/j.ijcard.2015.05.020
White, A. J., Smith, R. R., Matsushita, S., Chakravarty, T., Czer, L. S., Burton, K., et al. (2013). Intrinsic cardiac origin of human cardiosphere-derived cells. Eur. Heart J. 34, 68–75. doi: 10.1093/eurheartj/ehr172
Wiklander, O. P., Nordin, J. Z., O’loughlin, A., Gustafsson, Y., Corso, G., Mäger, I., et al. (2015). Extracellular vesicle in vivo biodistribution is determined by cell source, route of administration and targeting. J. Extracell. Ves. 4:26316. doi: 10.3402/jev.v4.26316
Yamaguchi, T., Izumi, Y., Nakamura, Y., Yamazaki, T., Shiota, M., Sano, S., et al. (2015). Repeated remote ischemic conditioning attenuates left ventricular remodeling via exosome-mediated intercellular communication on chronic heart failure after myocardial infarction. Int. J. Cardiol. 178, 239–246. doi: 10.1016/j.ijcard.2014.10.144
Yáñez-Mó, M., Siljander, P. R.-M., Andreu, Z., Bedina Zavec, A., Borràs, F. E., Buzas, E. I., et al. (2015). Biological properties of extracellular vesicles and their physiological functions. J. Extracell. Ves. 4:27066. doi: 10.3402/jev.v4.27066
Yu, B., Gong, M., Wang, Y., Millard, R. W., Pasha, Z., Yang, Y., et al. (2013). Cardiomyocyte protection by GATA-4 gene engineered mesenchymal stem cells is partially mediated by translocation of miR-221 in microvesicles. PLoS One 8:e73304. doi: 10.1371/journal.pone.073304
Yu, B., Kim, H. W., Gong, M., Wang, J., Millard, R. W., Wang, Y., et al. (2015). Exosomes secreted from GATA-4 overexpressing mesenchymal stem cells serve as a reservoir of anti-apoptotic microRNAs for cardioprotection. Int. J. Cardiol. 182, 349–360. doi: 10.1016/j.ijcard.2014.12.043
Zhang, A. T., Langley, A. R., Christov, C. P., Kheir, E., Shafee, T., Gardiner, T. J., et al. (2011). Dynamic interaction of Y RNAs with chromatin and initiation proteins during human DNA replication. J. Cell Sci. 124, 2058–2069. doi: 10.1242/jcs.086561
Zhuang, X., Xiang, X., Grizzle, W., Sun, D., Zhang, S., Axtell, R. C., et al. (2011). Treatment of brain inflammatory diseases by delivering exosome encapsulated anti-inflammatory drugs from the nasal region to the brain. Mol. Ther. 19, 1769–1779. doi: 10.1038/mt.2011.222
Keywords: bioengineering, cardiac fibrosis, extracellular vesicles, progenitor cells, next generation therapeutics
Citation: Rogers RG, Ciullo A, Marbán E and Ibrahim AG (2020) Extracellular Vesicles as Therapeutic Agents for Cardiac Fibrosis. Front. Physiol. 11:479. doi: 10.3389/fphys.2020.00479
Received: 11 March 2020; Accepted: 20 April 2020;
Published: 21 May 2020.
Edited by:
Claudio de Lucia, Temple University, United StatesReviewed by:
Alexander E. Berezin, Zaporizhia State Medical University, UkraineRainer Schulz, University of Giessen, Germany
Ian Dixon, University of Manitoba, Canada
Copyright © 2020 Rogers, Ciullo, Marbán and Ibrahim. This is an open-access article distributed under the terms of the Creative Commons Attribution License (CC BY). The use, distribution or reproduction in other forums is permitted, provided the original author(s) and the copyright owner(s) are credited and that the original publication in this journal is cited, in accordance with accepted academic practice. No use, distribution or reproduction is permitted which does not comply with these terms.
*Correspondence: Eduardo Marbán, RWR1YXJkby5NYXJiYW5AY3NtYy5lZHU=