- 1College of Forestry, Northeast Forestry University, Harbin, China
- 2CAS Center for Excellence in Biotic Interactions, University of Chinese Academy of Sciences, Beijing, China
- 3Key Laboratory of Insect Developmental and Evolutionary Biology, CAS Center for Excellence in Molecular Plant Sciences, Shanghai Institute of Plant Physiology and Ecology, Chinese Academy of Sciences, Shanghai, China
Insect ion transport peptides (ITPs) are important regulators of many physiological processes and they exert their functions by interacting with their receptors (ITPRs). In the current study, we comprehensively investigated the physiological functions of ITPR in the lepidopteran model insect, the silkworm (Bombyx mori), using the clustered regularly interspaced short palindromic repeats (CRISPR)/CRISPR-associated protein-9 nuclease (Cas9) genome editing technique. Mutations in silkworm ITPR (BNGR-A2) resulted in a prolongnation of the larval stage by 3.5-day as well as failure in wing expansion of moths. The BNGR-A2 mutation accelerated food transition throughout the digestive tract, which is 1.55-fold that of wild type (WT) insects. Excretion was 1.56-fold of WT insects during the larval stage, resulting in the loss of body water content. Loss of BNGR-A2 function induced significant upregulation of nitric oxide synthase (NOS) enzyme activity and nitric oxide (NO) content, as well as downstream Ca2+/NO/cGMP signaling pathways. Key genes in insulin and ecdysone signaling pathways were also affected by BNGR-A2 disruption. Our data show that ITPR plays key roles in regulating insect water homeostasis and development.
Introduction
Ion transport peptides (ITPs) and its alternatively spliced homologous ITP-like (ITPL) products belong to the crustacean hyperglycemic hormone (CHH) family of peptides. They are homologs of the crustacean hyperglycemic hormones of molting-inhibiting hormone (MIH) and gonad/vitellogenesis-inhibiting hormone (GIH) (Audsley et al., 2006; Webster et al., 2012). In crustaceans, CHH family peptides play roles in energy metabolism, molting, reproduction, immune defense, and osmotic regulation, as well as in homeostatic regulation of stress responses (Sonobe et al., 2001; Webster et al., 2012). In insects, ITP and ITPL with Cl–, Na+, and Ca2+ transport, as well as fluid reabsorption functions, have been identified (Audsley et al., 1992; Drexler et al., 2007; Dircksen et al., 2008; Webster et al., 2012; Nagai et al., 2014; Yu et al., 2016). In Drosophila melanogaster, ITP plays a critical role in development and also participates in the control of locomotor rhythms (Johard et al., 2009; Hermann-Luibl et al., 2014; Gáliková et al., 2018), and the mutation of ITP is embryonically lethal (Park et al., 2004). Gáliková et al. (2018) work identified master regulatory roles of ITP in water homeostasis of Drosophila; ITP levels increase under desiccation stress and protect the fly from water loss by increasing thirst, reducing excretion rate, and promoting ingestion of water instead of food. Several studies have shown that ITPs function during ecdysis in Manduca sexta (Drexler et al., 2007). The insect ITPL also participates in ovarian maturation in Tribolium castaneum (Begum et al., 2009) and regulates wing expansion and cuticle melanism in Nilaparvata lugens (Yu et al., 2016).
Insect ITP and ITPL act through their receptors (ITPRs) to function in many physiological processes. However, no ITPRs have been identified in the model insect D. melanogaster thus far (Gáliková et al., 2018; Nässel and Zandawala, 2019). In the lepidopteran model insect, Bombyx mori, it has been reported that two Bombyx neuropeptide G protein-coupled receptors (BNGRs), BNGR-A2 and BNGR-A34, are native receptors for ITP (Nagai et al., 2014). The ITP receptor, BNGR-A34, is conserved across insect species, whereas BNGR-A2 may have a species-specific role in lepidopterans (Nagai et al., 2014). Although a positive correlation between response of the receptor and bioactivity of the ligand has been verified in vitro (Nagai et al., 2014), the physiological functions of both ITP/ITPR and their receptors in insects in vivo remain to be elucidated.
In this study, we investigated the physiological functions of BNGR-A2 using the CRISPR/Cas9 genome editing technique. Loss of BNGR-A2 function extended the larval developmental stage and caused failure of wing expansion in moths. The BNGR-A2 mutation also accelerated food transition throughout the digestive tract as well as excretion. Our data therefore reveals for the first time that ITPR plays critical roles in regulating water homeostasis and developement in B. mori.
Materials and Methods
Silkworm Strains
A multivoltine silkworm strain, Nistari, was used in all experiments. Larvae were reared with fresh mulberry leaves at 25°C and 75% relative humidity (RH) (Tan et al., 2013).
Plasmid Construction and Germline Transformation
The activator line of pBac [IE1-EGFP-Nos-Cas9] (Nos-Cas9) was used to construct the transgenic CRISPR/Cas9 system. Cas9 was driven by the germ-cell-specific promoter, Nos, as described previously (Xu et al., 2017b). The effector line pBac [IE1-DsRed × 2-U6-2 × BNGR-A2-sgRNAs] (BNGR-A2-sgRNAs) was under the control of the silkworm small nuclear RNA promoter U6. The plasmids targeting BNGR-A2 were constructed through a series of cloning steps (Xu et al., 2017a); primer sequences are listed in Supplementary Table S1.
For silkworm germline transformation, G0 Nistari embryos within 8 h of oviposition were separately injected with a plasmid mixture of Nos-Cas9 or BNGR-A2-sgRNAs. Hatched larvae were reared to the adult stage and G0 moths were sib-mated or backcrossed with wild-type (WT) moths. Using a fluorescence microscope (Nikon AZ100), G1 offspring were screened for the marker gene during the embryonic stage. The Nos-Cas9 line and the BNGR-A2-sgRNA line were crossed to produce heterozygous F1 progeny (mutant was obtained by indicator of two-color fluorescence), which was then used in subsequent experiments.
Genomic DNA Extraction and Mutagenesis Analysis
The BNGR-A2 mutant genomic DNA was extracted using a nucleic acid isolation system DP323 (TianGen, Beijing, China), and the DNA fragments, including the designed sgRNA targeting site, were amplified using EasyTaq® DNA Polymerase (TransGen Biotech, Beijing, China). The PCR conditions were as follows: 94°C for 5 min, 35 cycles at 94°C for 30 s, 60°C for 30 s, and 72°C for 1 min, followed by a final extension period of 72°C for 10 min. The PCR products were cloned into pMD19-T-Simple (Takara, Dalian, China) and subsequently sequenced. The primers that were designed to detect mutagenesis in targeted sites are listed in Supplementary Table S1.
Bioassays
The cumulative numbers of fecal pellets from 20 fifth instar silkworms (0 day of fifth instar larvae, L5D0) were counted in the breeding box every hour. Droppings were counted in three replicates. The statistical significance was analyzed by two-way ANOVA, and the number of fresh fecal masses that were deposited within 2 h (the given period) was used.
To measure water content, L5D0 silkworm larvae were dehydrated at 80°C until a constant weight. The weight of 10 larvae was recorded before and after dehyration using a Mettler MT5 analytical microbalance (Columbus, OH, United States). The difference in water content between the fresh and dry weights was calculated and is expressed as the percent of the fresh body weight. Three replicates (each consisting of 10 silkworms) were tested per WT and mutant silkworm.
Ecdysteroid Titer and NOS Activity Determination
Haemolymph was extracted from transgenic or WT larvae (L5D3). Total ecdysteroids in the haemolymph samples were extracted as previously described (Mirth et al., 2005). Concentrations of total ecdysteroids were quantified using a 20-Hydroxyecdysone EIA kit (Cayman Chemicals, Ann Arbor, MI, United States) according to the manufacturer’s instructions.
The nitric oxide synthase (NOS) kit (Nanjing Jiancheng Bioengineering Institute, Nanjing, China) was used to detect the total NOS activity in silkworm hindgut. The total nitric oxide (NO) was determined using a Nitric Oxide Assay Kit (Beyotime, Shanghai, China). Protein concentrations were measured using the Bradford Protein Assay Kit (Beyotime, Shanghai, China). Each measurement was from at least four biological replicates including two males and two females. Data were normalized to the protein concentration.
RNA Isolation, cDNA Synthesis, and Quantitative Real-Time PCR (qRT-PCR) Analysis
Total RNA was extracted from the fat body and hindgut of L5D3 animals using Trizol reagent (Invitrogen, Carlsbad, CA, United States) in accordance with the manufacturer’s instructions. Then, 0.5 μg of RNA was reverse-transcribed for cDNA using TransScript® RT/RI Enzyme Mix (TransGen Biotech, Beijing, China). qRT-PCR was performed using a SYBR Green PCR Kit (Toyobo, Osaka, Japan) and an ABI Stepone plus real-time PCR system (Applied Biosystems, United States). Each 20 μL reaction contained 10 μL of SYBR Green real-time PCR master mix (Toyobo, Osaka, Japan), 0.5 μM of each forward and reverse primer, and 2 μL of cDNA template (equivalent to 100 ng of total RNA). Primers used for amplification are listed in Supplementary Table S1. Thermal cycling parameters were 94°C for 5 min followed by 40 cycles at 94°C for 30 s and 60°C for 1 min. For each sample, a melting curve was generated at the end of each run to allow assessment of product purity. Expression levels were calculated according to the 2–ΔΔCT method (Pfaffl et al., 2002), and relative expression levels were calculated by dividing mutant transcription levels by levels recorded in wild type, WT insects.
RNA-Seq Analysis
Hindguts from WT and mutant L5D3 animals were separately collected and used to construct a transcriptome library. Each type of sample was collected from four animals (two males and two females). The cDNA libraries were sequenced using an Illumina BGISEQ-500 platform (BGI, Wuhan, China). Total RNA was isolated as described previously, and cDNA libraries were prepared according to Illumina’s protocol. The raw data were filtered using Tophat software, corrected, and mapped to the silkworm genome sequence. The total reading was normalized by a plurality of normalization factors. Transcript levels were calculated using standard readings per petaflop of mapping readings. The difference between the mutant and the control groups is represented by the P value and fold change (ΔBNGR-A2/WT). Differentially expressed genes with a significance level of P < 0.001 and fold change (ΔBNGR-A2/WT) > 2 were enriched in each comparison.
Statistical Analyses
Two-tailed Student’s t-test and one-way ANOVA were used to analyze measurement variables. Asterisk symbols reflect significant differences (∗P < 0.05, ∗∗P < 0.01, ∗∗∗P < 0.001, ****P < 0.0001). Error bars represent SEM. Measurement variable data was analyzed using Excel Workbook (version 2016) and data related to nominal variables were analyzed by GraphPad Prism (version 7.0).
Results
Generation of BNGR-A2 Targeted Mutations Using the Transgenic CRISPR/Cas9 System
A binary transgenic CRISPR/Cas9 system (Li et al., 2015) was used to generate somatic BNGR-A2 mutants. This system included two transgenic silkworm lines. One line expressed Cas9 under the control of Nos promoter and the other line expressed two sequence-specific sgRNAs under the control of U6 promoter to target BNGR-A2 exon 1 (Figures 1A,B). Transgenic animals carrying nos-Cas9 or U6-BNGR-A2–sgRNA were fully viable and fertile, indicating that the accumulation of neither Cas9 nor BNGR-A2-sgRNA had deleterious effects on silkworm physiology. The offspring crossed by Nos-Cas9 and U6-BNGR-A2-sgRNA lines specifically expressed the active Cas9-sgRNA complex in the germ line. The mutations were located at the targeted genomic loci of the BNGR-A2 gene (Figure 1B). To assess mutation efficiency, five newly hatched silkworms with both red and green fluoresence were randomly selected to extract the genomic DNA. The DNA samples were run through PCR amplification. PCR-amplified fragments were subcloned and sequenced. The results showed that various deletion mutations located at two sgRNA targets and deletions ranged from 215 to 349 bp, indicating that successful mutations were produced using the transgenic CRISPR/Cas9 system. Moreover, the BNGR-A2 gene was successfully disrupted (Figure 1C).
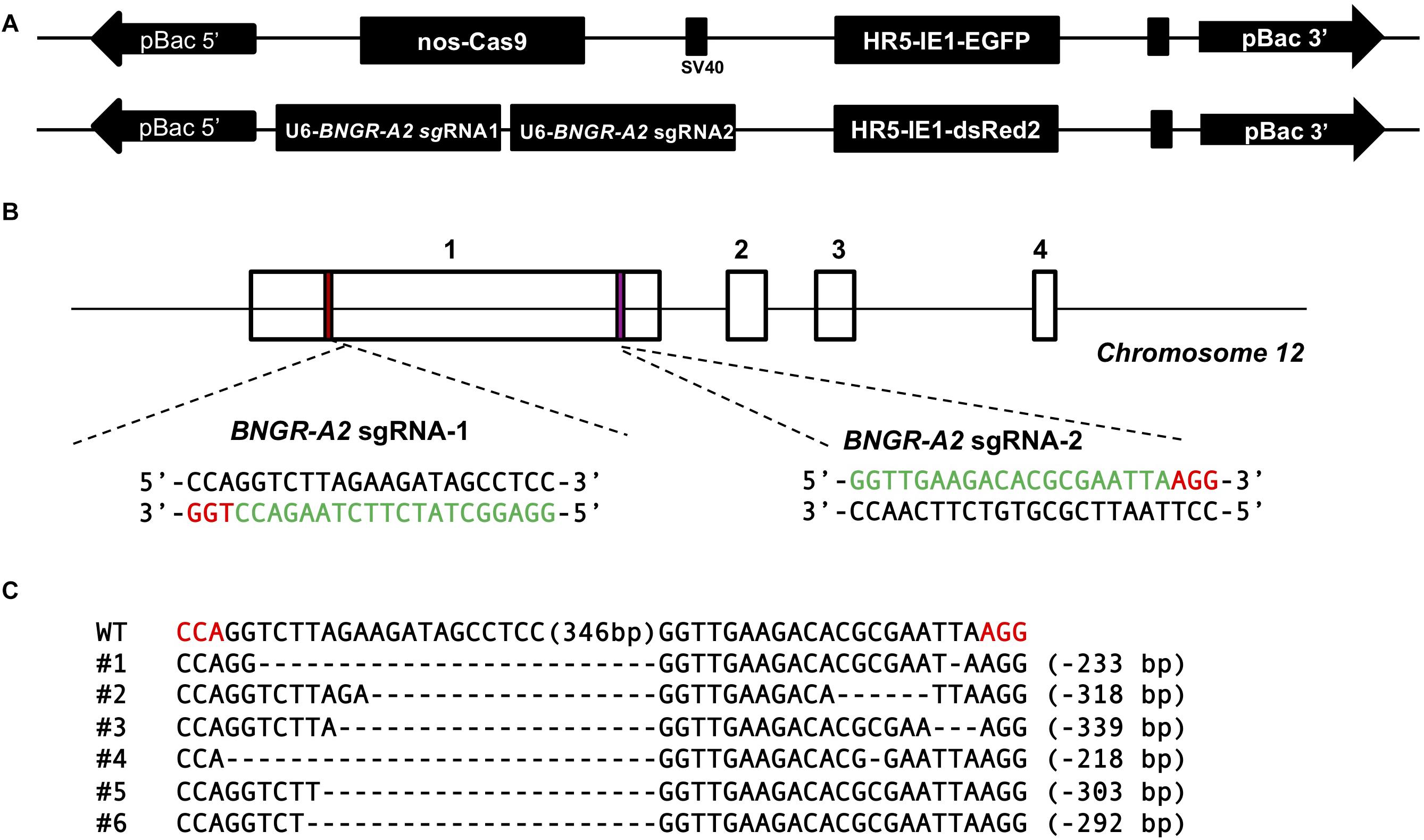
Figure 1. Schematic diagram of BNGR-A2 sgRNA targeting sites and genomic mutagenesis induced by the transgenic CRISPR/Cas9 system. (A) Binary transgenic CRISPR/Cas9 system. (B) Schematic diagram of sgRNA-targeting sites. The boxes indicate the four exons of BNGR-A2, and the black line represents the gene locus. The sgRNA-targeting sites, BNGR-A2 sgRNA-1 and BNGR-A2 sgRNA-2, are located of the exon 1. The sgRNA targeting sequence is in green, and the protospacer adjacent motif (PAM) sequence is in red. (C) Various deletion mutageneses were detected in heterozygous Nos-Cas9: BNGR-A2-sgRNAs (△BNGR-A2) offspring. The dashes in every sequence line represent deleted residues and the detailed indel size is shown on the right. The numbers in brackets in the middle of each sequence refer to the 349 bp interspace fragment that was found between the targeting sites. The red sequence indicates the PAM sequence.
Water Content and Excretion Rate of BNGR-A2 Mutant Larvae
Previous studies showed that ITP has a positive effect on the water balance in Drosophila (Gáliková et al., 2018). However, whether ITPR affects the water balance in insects is unclear. In order to investigate whether BNGR-A2 is involved in water homeostasis in B. mori, the water contents of WT and mutant 5th instar larvae were determined. The results showed that the water contents of ΔBNGR-A2 animals was (87.34 ± 0.03)%, which was significantly lower than that of WT animals (88.76 ± 0.05)%; P < 0.0001), indicating that the loss of BNGR-A2 function affected the water homeostasis regulation in B. mori (Figure 2A).
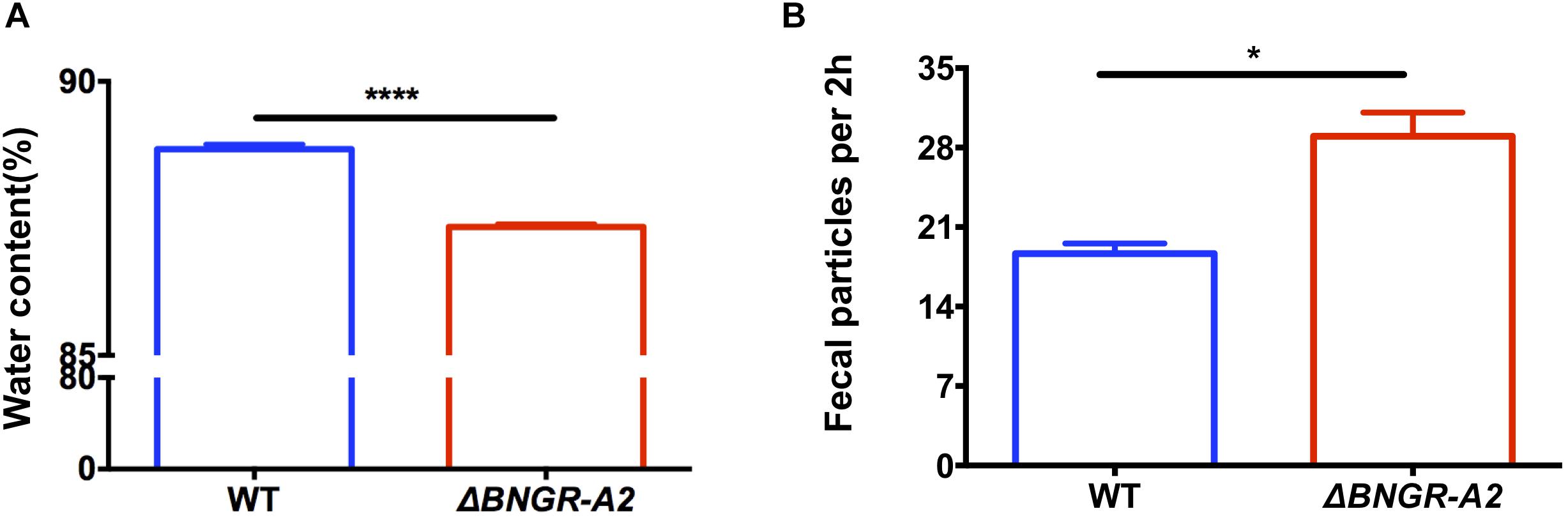
Figure 2. ITP regulates water content, the speed of transit through the digestive tract and the number of defecation events. (A) BNGR-A2 regulates water content. Two-tailed Student’s t-test: P < 0.0001. (B) BNGR-A2-loss increases the defecation rate.
In insects, the primary urete enters the hindgut, and water that is not reabsorbed by the posterior intestinal epithelium is usually excreted in the same way as feces (Gäde, 2004). We investigated whether BNGR-A2 knockout affects excretion. The excretion rate of the 5th instar larvae was calculated by counting the number of fecal particles produced every 2 h. The results showed that each mutant larvae produced 29 fecal particles per 2 h period, which was significantly higher than in WT animals (18.67 fecal particles per 2 h, P < 0.05). Loss of BNGR-A2 function accelerated food transition throughout the digestive tract (Figure 2B), resulting in more frequent defecation events.
Phenotypic Defects in BNGR-A2 Mutants
The BNGR-A2 mutant has no deleterious phenotype during embryonic development. From the end of the 2nd larval instar, mutant larvae exhibited a significant delay in growth rate relative to WT animals (Figures 3A,B). Compared with WT animals, the development of mutant larvae was delayed by half a day during the 3rd larval stage. The weight of mutant larvae was 57.41% of WT animals at L3D2. During the 4th instar larval stage, the weight of the mutant larvae was 55.58% that of WT animals, and development was delayed by 2.5 days. The weight of the 5th instar mutant larvae was 73.84% of the WT animals at L5D0, and the weight of the L5D4 mutant was similar to that of the L5D2 WT animals (Figures 3A,B). The half pupation time (PT50) of the mutant silkworm was delayed by 3 days relative to WT silkworms (Figure 3A). Pupal weight did not show significant differences between mutant and WT animals. After eclosion, the fore and hindwings of mutant moths became crumpled (Figure 3C).
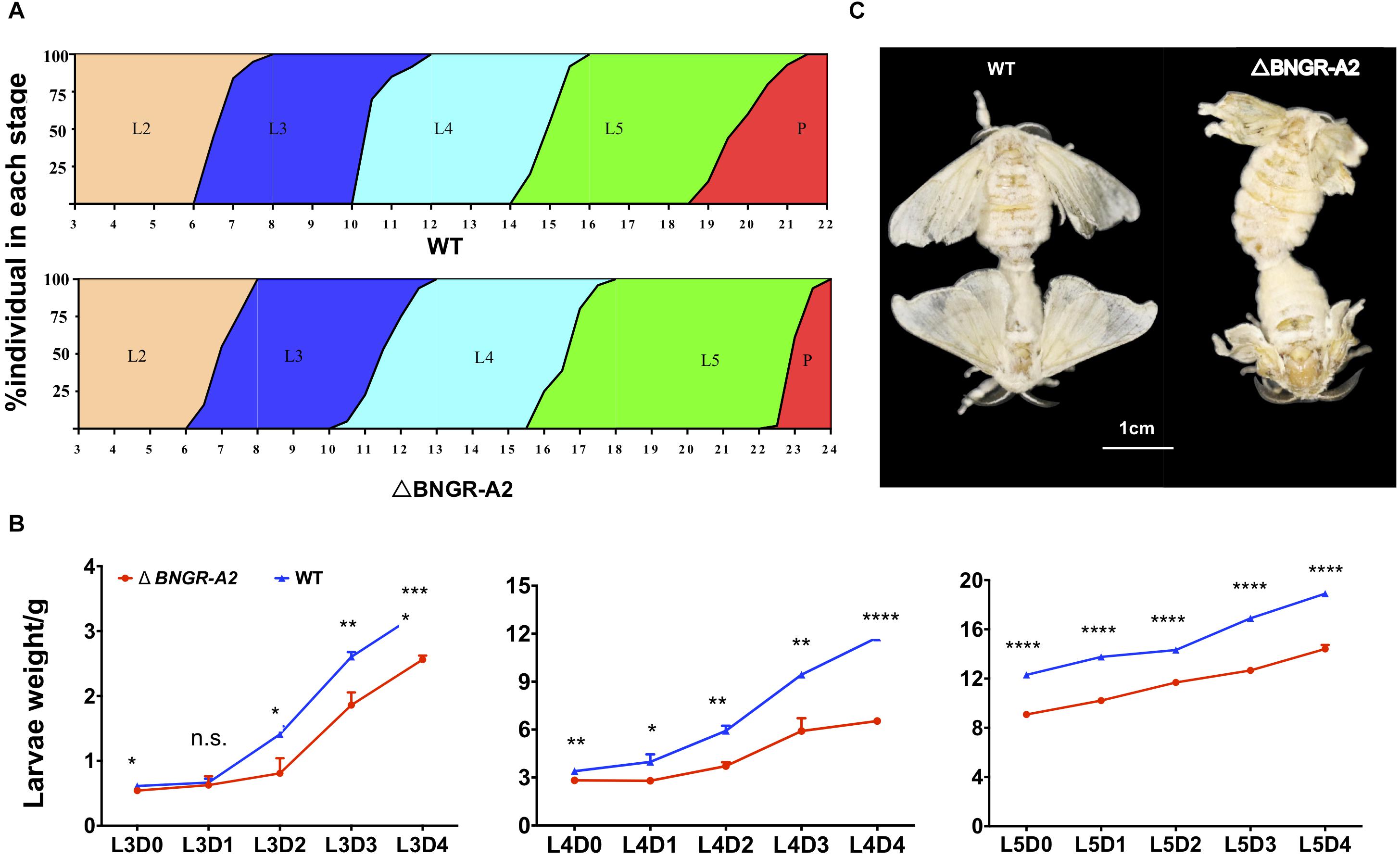
Figure 3. BNGR-A2 gene mutation affects larval growth and development. (A) The stages of larval and pupal development in WT and △BNGR-A2 (L2–L5 represent 2nd to 5th instar, respectively; P, pupae). (B) The L3, L4, and L5 weight of WT and △BNGR-A2 (n = 3 samples per treatment, 10 animals/sample). (C) Wing expansion failed in △BNGR-A2 insects. The left graph shows the △BNGR-A2 insects, the right graph shows the WT insects.
Ca2+/NO/cGMP Signaling in BNGR-A2 Mutants
To explore the molecular mechanisms underlying defective phenotypes induced by BNGR-A2 mutations, we perfomed RNA-seq analysis by using hindgut tissues between WT and mutant larvae. A total of 1,288 differentially expressed genes (612 up-regulated and 676 down-regulated) were identified (Figure 4A and Supplementary Figure 1SA). The up- and down-regulated genes were allocated into three categories according to the Gene Ontology (GO) terms: molecular function, cellular component, and biological process (Supplementary Figure 1SB). Among GO categories, the “catalytic activity” in “molecular function,” “membrane” and “membrane part” in “cellular component,” and “metabolic process” in “biological process” were the most abundant protein classes. Kyoto Encyclopedia of Genes and Genomes (KEGG) enrichment analysis revealed that water-transport-related pathways, such as the calcium signaling pathway, cGMP-PKG signaling pathway and cAMP signaling pathway, were annotated (Table 1 and Supplementary Figure 1SC).
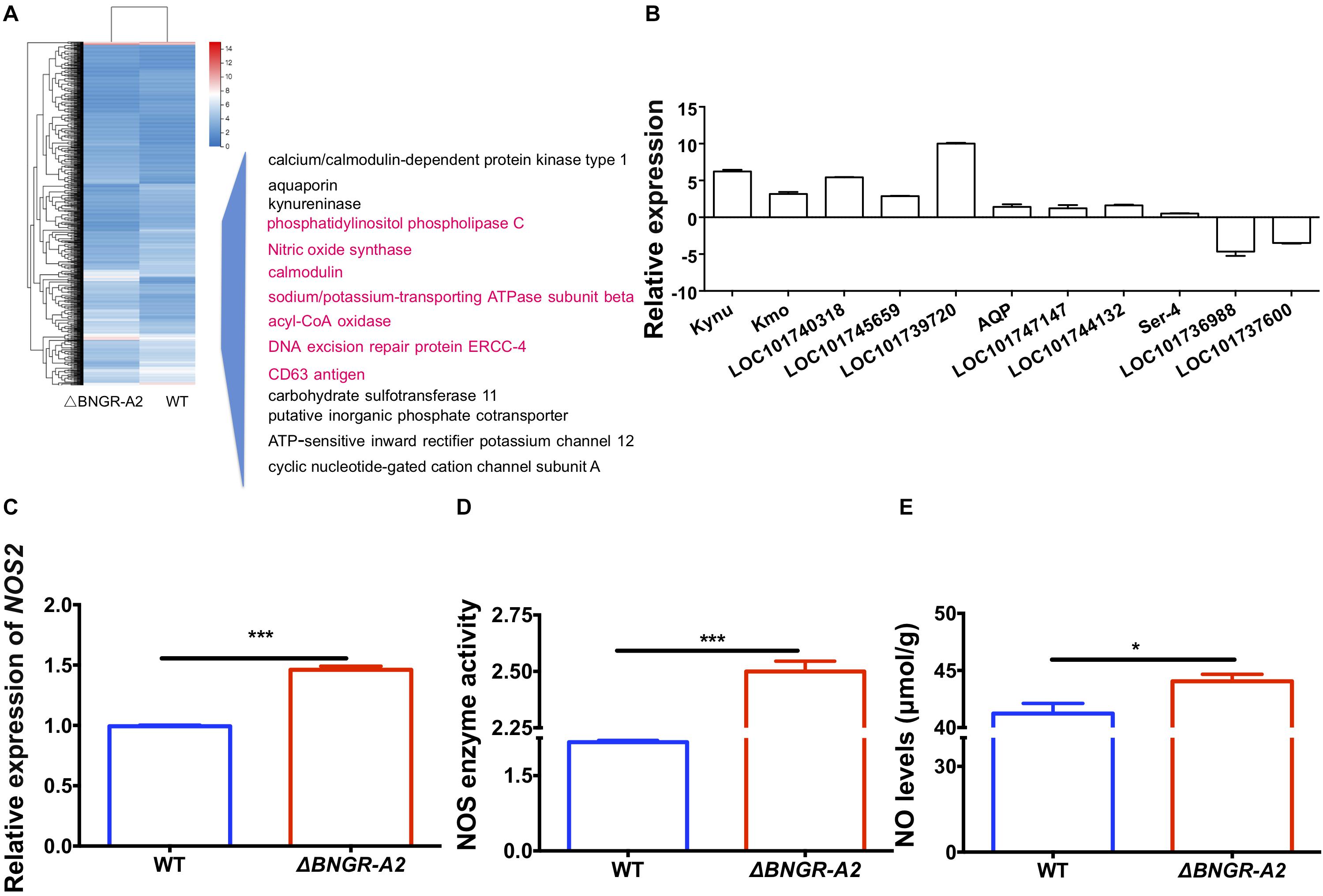
Figure 4. Cluster analysis of RNA-Seq data leads to the identification of Ca2+/NO/cGMP as downstream of the BNGR-A2 pathway. (A) Cluster analysis of differentially expressed genes (DEGs) in the transcriptome (in hindguts, HG). Several important genes (highlighted pink) were involved in Ca2+/NO/cGMP signaling and display expression patterns after the loss of BNGR-A2 transcription levels. Logarithmic fold alteration of △BNGR-A2 versus WT is shown in the heat map. Red and blue colors indicate up- and down-regulation, respectively. (B) Transcriptome verification of genes involved in water transport, Log2. (C) Change in the expression of NOS2 genes in HG (n = three samples per treatment, four (two males and two females) silkworms/sample). (D) Enzyme activity change of NOS in HG (n = three samples per treatment, four (two males and two females) silkworms/sample). (E) Changes in the levels of NO in HG [n = two samples per treatment, four (two males and two females) silkworms/sample].
Among these differentially expressed genes (DEGs), several classes of genes and inorganic salt ion transporters, ATP-sensitive inward rectifier potassium channels, uridine nucleosides, potassium voltage-gated channel proteins, calcium/calmodulin-dependent proteins, solute carriers, and organic anions were identified. A large of DEGs were associated with transporters, aquaporins, and water transport genes were heavily annotated, and a class of important signaling molecular genes were annotated. Using qRT-PCR, several types of genes were confirmed in two comparisons (Figure 4A and Table 2), showing elevated transcriptional levels in the hindgut of mutants. The expression patterns of these genes, including inorganic salt ion transporters, calcium/calmodulin-dependent proteins, kynureninase, aquaporins and NOS, were confirmed in mutants and WT comparisons (Figure 4B and Table 2).
In D. melanogaster tubules, as in vertebrate epithelia, convergence of distinct but interacting signal transduction pathways (Ca2+/NO/cGMP) stimulates calcium entry into principal cells via several classes of calcium channels, resulting in increased fluid transport (Choi et al., 1995; Davies, 2000). In this study, we examined whether the NOS pathway could be influenced after BNGR-A2 mutation. In mutant animals, the transcriptional level of NOS2 was significantly up-regulated by 143% (Figure 4C), NOS enzyme activity increased by 15.39% (Figure 4D), and NO concentration increased by 6.85% (Figure 4E), as compared with WT animals. These results supported that the NOS pathway was accelerated in mutant animals.
Insulin and Ecdysone Signaling Pathways in BNGR-A2 Mutants
To further investigate the molecular mechanisms underlying phenotypic defects in the growth of BNGR-A2 animals, transcription levels of key genes in the insulin and ecdysone signaling pathways were investigated in L5D3 larval fat body of WT and BNGR-A2 mutants. Relative mRNA expressions of key genes in insulin signaling pathways including PI3K, AKT, S6K, and 4EBP were down-regulated, suggesting that knockout of BNGR-A2 affected the insulin signaling pathway (Figure 5A). Lower ecdysteroid titers and downregulation of 20E-related key genes, including ECRA, E75A, E75B and HR3, suggested that the 20E signaling pathway was affected after BNGR-A2 disruption (Figures 5B,C).
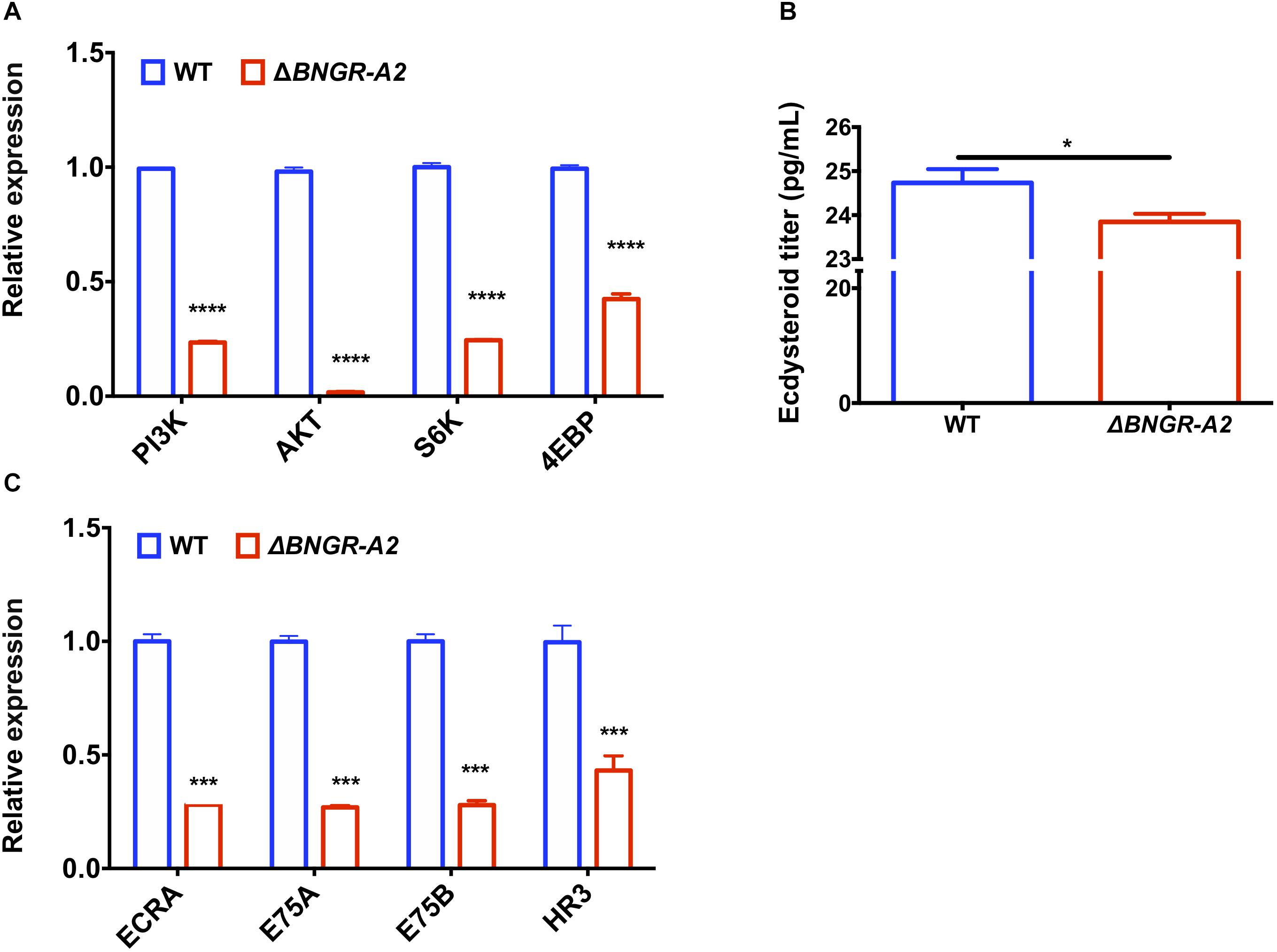
Figure 5. BNGR-A2 regulates transcriptional levels of genes in insulin signaling pathway and 20E signaling pathway. (A) Change in expression of genes in insulin signaling pathway in fat body (FB). (B) Change in expression of ecdysone responsive genes in fat body (FB). The data show mean ± SEM (n = 3). (C) Relative ecdysteriod titers in the hemolymph at L5D3 stage of WT and △BNGR-A2 animals. Hemolymph was collected from five larvae, and the pooled samples were used to determine ecdysteriod titers.
Discussion
ITP belongs to the class of CHH family neuropeptides and has been functionally characterized in many insect species (Drexler et al., 2007; Dircksen et al., 2008; Webster et al., 2012; Nagai et al., 2014; Yu et al., 2016). However, little is known regarding ITP signaling pathways in insects, and no insect ITPRs have been identified thus far, except in B. mori (Nagai et al., 2014). Here, we comprehensively investigated physiological functions of BNGR-A2 in B. mori by using CRISPR/Cas9-mediated gene disruption. The NOS enzyme activity, NO content, and calmodulin transcription levels were significantly higher when BNGR-A2 was knocked out. The downstream Ca2+/NO/cGMP signaling pathways were activated, resulting in the loss of water in the body. The decrease in the water content and concurrent increase in the amount of excretion in the mutant animals resulted in insufficient pressure in the adult body after emergence and ultimately led to the failure of wing expansion (Figure 6). BNGR-A2 deficiency affected silkworm growth and development by inhibiting ecdysone and insulin signaling. These results reveal the important role BNGR-A2 plays in insect larval growth rate, wing expansion, and water homeostasis (Figure 6).
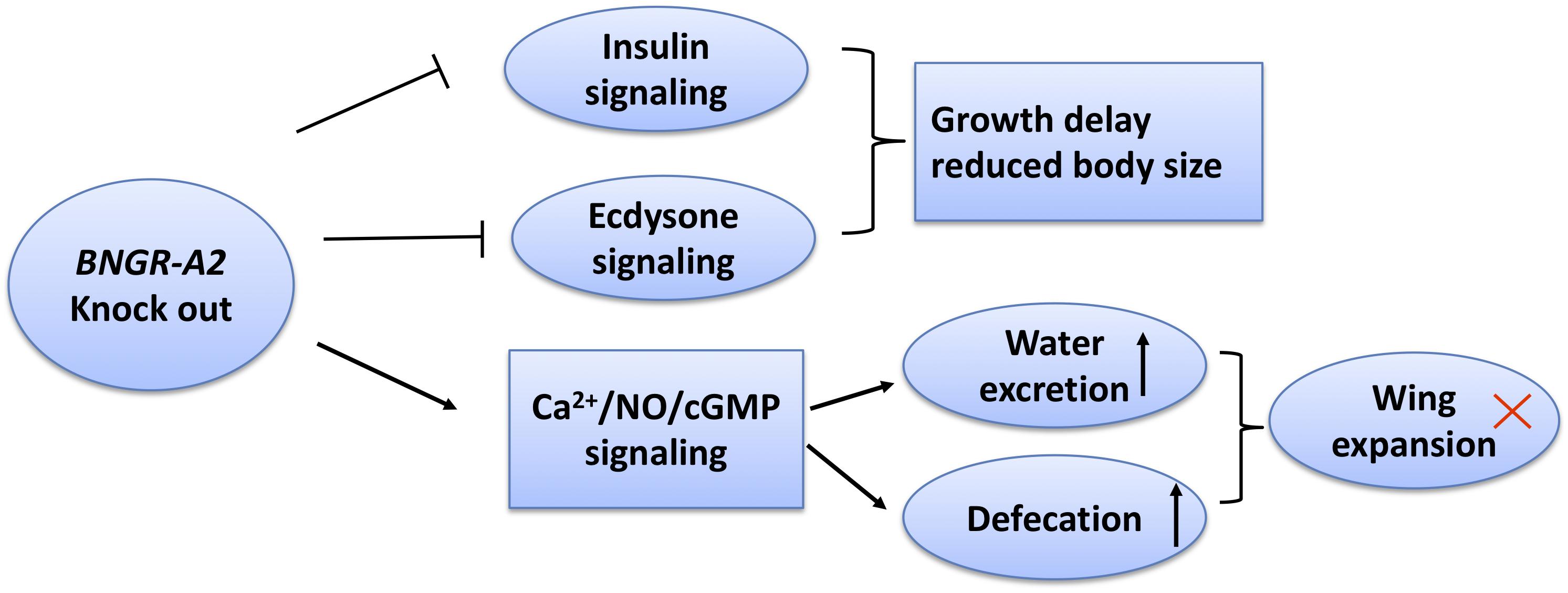
Figure 6. Scheme summarizing the roles of BNGR-A2 in Bombyx mori. In BNGR-A2 knock-out silkworm larvae, insulin signaling is reduced and ecdysone signaling inhibition causes growth delay. Through the Ca2+/NO/cGMP signaling pathway, body water decreases and the rate of excretion increases. Eventually, the body pressure drops to critical levels and the adult wings fail to expand.
In the insect excretory system, the Malpighian tubules and hindgut are involved in water preservation and absorption, which aid in maintaining water balance, allowing the organism to tolerate external hypertonicity or dryness (Meredith et al., 1996). This process is strictly regulated by neuropeptides, including diuretic hormone 31 (DH31) (Coast et al., 2001), diuretic hormone 44 (DH44), leucokinin (Cannell et al., 2016), and capa (Terhzaz et al., 2015), as well as antidiuretic hormones such as ITP. In insects, the diuretic peptide acts as a scavenging hormone, promoting the formation of primary urine in malpighian tubules and inducing rapid circulation of hemolymph and frequent hypoperistalsis of the digestive tract. Diuretic peptides promote the entry of raw urine into the hindgut, aid in moving dry food residue through the intestine (Gäde, 2004). The antidiuretic hormone acts on the hindgut to promote water reabsorption and plays an important role in insect water retention (Gäde, 2004). In previous studies, the NO signaling pathway was reported to be involved in the preservation of water in Diptera (Davies, 2000). NO is produced from the amino acid L-arginine by the enzyme action of nitric oxide synthase (NOS). NOS is encoded by a large multigene family, and occurs several isoforms. NOS isoforms have been classified into either calcium-dependent or calcium-independent enzymes, and belong to constitutive (ecNOS and ncNOS) or inducible NOS (iNOS) (Davies, 2000). Liquid transport was found to be controlled by NOS in Drosophila Malpighian tubules (Davies, 2000). In the late stage of Plasmodium berghei infection of A. stephensi in Diptera, NOS activity was significantly increased, likely due to the increase of hemolymph clearance (Dimopoulos et al., 1998). The NOS isoform in mosquitoes also contains Ca2+/calmodulin binding sites, indicating that NOS activation is associated with a regulatory calcium signaling process (Davies, 2000). In B. mori, two types of NO synthase were found in the malpighian tube. During the gut purging phase of B. mori larvae, here considered the excretion phase, the activity of NOS in malpighian tubule was significantly increased (Choi et al., 1995). We also found NOS activity and NO content to be significantly higher in the mutant B. mori than in WT animals. Based on hindgut transcriptome and qPCR data, downstream Ca2+/NO/cGMP signaling pathways were activated and 46 DEGs were annotated in the pathways named above when BNGR-A2 was knocked out (Table 2). The transcription levels of the DEGs in mutant B. mori, including those encoding aquaporins, ion cotransporters, calmodulin (CaM), and NOS, were significantly higher than those of the WT animals. This is the first work to show that knockout of BNGR-A2 in silkworms caused a (1.42 ± 0.035)% reduction in body water. Previous research showed that in humans, 2% dehydration leads to diminished mental activity and cognitive ability (Grandjean and Grandjean, 2007). Our results revealed that the Ca2+/NO/cGMP signaling pathway was involved in the transportation of water in B. mori, indicating that BNGR-A2 affects the resorption of the hindgut water.
BNGR-A2 was also found to play a critical role in the development of B. mori. The mutant larvae had significantly lower body weights than WT larvae at the same stage. The mutants showed slower preadult development, and larval development was extended by 3 days. Although development was prolonged, the pupal body weight showed no significant difference between mutant and WT B. mori. The size of adults was primarily determined by two factors: duration of growth and growth rate. The ecdysone and juvenile hormones synergistically regulate insect developmental metamorphosis and determine the duration of insect growth. The growth and death signals, such as insulin, nutrition, and cell contact inhibition, as well as their conduction pathways, control cell division, growth, differentiation, death, and ultimately determine the insect’s growth rate (Colombani et al., 2005; OrmeSally and Leevers, 2005). We discovered that the ecdysone titer is lower and the ecdysone pathway genes were down-regulated in the Δ BNGR-A2. The key genes of the insulin signaling pathway in the mutant were down-regulated, and the results indicated that the mutation of BNGR-A2 disrupted the expression of other genes in the insulin signaling pathway, affecting the nutritional signaling pathway of the silkworm. This ultimately led to changes in the duration of development of mutant silkworms. However, the regulatory interaction of BNGR-A2 between water homeostasis and ecdysone, as well as the insulin signaling pathways, needs further investigation.
Data Availability Statement
All datasets generated for this study are included in the article/Supplementary Material.
Author Contributions
LS and AT designed the research and wrote the manuscript. ZZ, RZ, YY, and FY performed the experiments and analyzed the data. AT revised the manuscript. All authors listed have approved the manuscript for publication.
Funding
This work was supported by grants from the National Science Foundation of China (31925007 and U1738110).
Conflict of Interest
The authors declare that the research was conducted in the absence of any commercial or financial relationships that could be construed as a potential conflict of interest.
Supplementary Material
The Supplementary Material for this article can be found online at: https://www.frontiersin.org/articles/10.3389/fphys.2020.00424/full#supplementary-material
FIGURE S1 | Transcriptomic profiles influenced by BNGR-A2-loss in silkworm hindguts by RNA-seq. (A) M-versus-A plot, MA plot diagram of DEGs in the transcriptome. (B) Gene Ontology (GO) term of DEGs in the transcriptome. (C) Kyoto Encyclopedia of Genes and Genomes (KEGG) annotations of differentially expressed genes (DEGs) in the transcriptome.
TABLE S1 | List of primers used in this study.
References
Audsley, N., Mcintosh, C., and Phillips, J. E. (1992). Actions of Ion-transport peptide from locust corpus cardiacum on several hindgut transport processes. J. Exp. Biol. 173, 275–288.
Audsley, N., Meredith, J., and Phillips, J. E. (2006). Haemolymph levels of Schistocerca gregaria ion transport peptide and ion transport-like peptide. Physiol. Entomol. 31, 154–163. doi: 10.1111/j.1365-3032.2006.00500.x
Begum, K., Li, B., Beeman, R. W., and Park, Y. (2009). Functions of ion transport peptide and ion transport peptide-like in the red flour beetle Tribolium castaneum. Insect Biochem. Mol.Biol. 39, 717–725. doi: 10.1016/j.ibmb.2009.08.005
Cannell, E., Dornan, A. J., Halberg, K. A., Terhzaz, S., Dow, J. A. T., and Davies, S. A. (2016). The corticotropin-releasing factor- like diuretic hormone 44 (DH44) and kinin neuropeptides modulate desiccation and starvation tolerance in Drosophila melanogaster. Peptides 80, 96–107. doi: 10.1016/j.peptides.2016.02.004
Choi, S. K., Choi, H. K., Kadono-Okuda, K., Taniai, K., Kato, Y., Yamamoto, M., et al. (1995). Occurrence of novel types of nitric oxide synthase in the silkworm, Bombyx mori. Biochem. Biophys. Res. Commun. 207, 452–459. doi: 10.1006/bbrc.1995.1209
Coast, G. M., Webster, S. G., Schegg, K. M., Tobe, S. S., and Schooley, D. A. (2001). The Drosophila melanogaster homologue of an insect calcitonin-like diuretic peptide stimulates V-ATPase activity in fruit fly Malpighian tubules. J. Exp. Biol. 204, 1795–1804.
Colombani, J., Bianchini, L., Layalle, S., Pondeville, E., Dauphin-Villemant, C., Antoniewski, C., et al. (2005). Antagonistic actions of ecdysone and insulins determine final size in Drosophila. Science 310, 667–670. doi: 10.1126/science.1119432
Davies, S. A. (2000). Nitric oxide signalling in insects. Insect Biochem. Mol. Biol. 30, 1123–1138. doi: 10.1016/s0965-1748(00)00118-1
Dimopoulos, G., Seeley, D., Wolf, A., and Kafatos, F. C. (1998). Malaria infection of the mosquito Anopheles gambiae activates immune-responsive genes during critical transition stages of the parasite life cycle. EMBO J. 17, 6115–6123. doi: 10.1093/emboj/17.21.6115
Dircksen, H., Tesfai, L. K., Albus, C., and Nässel, D. R. (2008). Ion transport peptide splice forms in central and peripheral neurons throughout postembryogenesis of Drosophila melanogaster. J. Comp. Neurol. 509, 23–41. doi: 10.1002/cne.21715
Drexler, A. L., Harris, C. C., delaPena, M. G., Asuncion-Uchi, M., Chung, S., Webster, S., et al. (2007). Molecular characterization and cell-specific expression of an ion transport peptide in the tobacco hornworm, Manduca sexta. Cell Tissue Res. 329, 391–408. doi: 10.1007/s00441-007-0391-9
Gäde, G. (2004). Regulation of intermediary metabolism and water balance of insects by neuropeptides. Annu. Rev. Entomol. 49, 93–113.
Gáliková, M., Dircksen, H., and Nässel, D. R. (2018). The thirsty fly: ion transport peptide (ITP) is a novel endocrine regulator of water homeostasis in Drosophila. PLoS Genet. 14:e1007618. doi: 10.1371/journal.pgen.1007618
Grandjean, A. C., and Grandjean, N. R. (2007). Dehydration and cognitive performance. J. Am. Coll. Nutr. 26, 549S–544S.
Hermann-Luibl, C., Yoshii, T., Senthilan, P. R., Dircksen, H., and Helfrich-Förster, C. (2014). The ion transport peptide is a new functional clock neuropeptide in the fruit fly Drosophila melanogaster. J. Neurosci. 34, 9522–9536. doi: 10.1523/JNEUROSCI.0111-14.2014
Johard, H. A. D., Yoishii, T., Dircksen, H., Cusumano, P., Rouyer, F., Helfrich-Förster, C., et al. (2009). Peptidergic clock neurons in Drosophila: ion transport peptide and short neuropeptide F in subsets of dorsal and ventral lateral neurons. J. Comp. Neurol. 516, 59–73. doi: 10.1002/cne.22099
Li, Z. Q., You, L., Zeng, B. S., Ling, L., Xu, J., Chen, X., et al. (2015). Ectopic expression of ecdysone oxidase impairs tissue degeneration in Bombyx mori. Proc. Biol. Sci. 282:20150513. doi: 10.1098/rspb.2015.0513
Meredith, J., Ring, M., Macins, A., Marschall, J., Cheng, N. N., Theilmann, D., et al. (1996). Locust ion transport peptide (ITP): primary structure, cDNA and expression in a baculovirus system. J. Exp. Biol. 199, 1053–1061.
Mirth, C., Truman, J. W., and Riddiford, L. M. (2005). The role of the prothoracic gland in determining critical weight for metamorphosis in Drosophila melanogaster. Curr. Biol. 15, 1796–1807. doi: 10.1016/j.cub.2005.09.017
Nagai, C., Mabashi-Asazuma, H., Nagasawa, H., and Nagata, S. (2014). Identification and characterization of receptors for ion transport peptide (ITP) and ITP-like (ITPL) in the silkworm Bombyx mori. J. Biol. Chem. 289, 32166–32177. doi: 10.1074/jbc.M114.590646
Nässel, D. R., and Zandawala, M. (2019). Recent advances in neuropeptide signaling in Drosophila, from genes to physiology and behavior. Prog. Neurobiol. 179:101607. doi: 10.1016/j.pneurobio.2019.02.003
OrmeSally, M. H., and Leevers, J. (2005). Flies on steroids: the interplay between ecdysone and insulin signaling. Cell Metab. 2, 277–278. doi: 10.1016/j.cmet.2005.10.005
Park, Y., Kim, H., Li, D., and Adams, M. (2004). “A Novel function of ion transport peptide in Drosophila ecdysis,” in Proceedings of the Program and Abstracts 45th Annual Drosophila Research Conference, Washington, DC.
Pfaffl, M. W., Horgan, G. W., and Dempfle, L. (2002). Relative expression software tool (REST) for group-wise comparison and statistical analysis of relative expression results in real-time PCR. Nucleic Acids Res. 30:e36.
Sonobe, H., Nishimura, T., Sonobe, M., Nakatsuji, T., Yanagihara, R., Kawakami, T., et al. (2001). The molt-inhibiting hormone in the American crayfish Procambarus clarkii. its chemical and biological activity. Gen. Comp. Endocrinol. 121, 196–204. doi: 10.1006/gcen.2000.7586
Tan, A., Fu, G., Jin, L., Guo, Q., Li, Z., Niu, B., et al. (2013). Transgene-based, female-specific lethality system for genetic sexing of the silkworm, Bombyx mori. Proc. Natl. Acad. Sci. U.S.A. 110, 6766–6770. doi: 10.1073/pnas.1221700110
Terhzaz, S., Teets, N. M., Cabrero, P., Henderson, L., Ritchie, M. G., Nachman, R. J., et al. (2015). Insect capa neuropeptides impact desiccation and cold tolerance. Proc. Natl. Acad. Sci. U.S.A. 112, 2882–2887. doi: 10.1073/pnas.1501518112
Webster, S. G., Keller, R., and Dircksen, H. (2012). The CHH-superfamily of multifunctional peptide hormones controlling crustacean metabolism, osmoregulation, moulting, and reproduction. Gen. Comp. Endocr. 175, 217–233. doi: 10.1016/j.ygcen.2011.11.035
Xu, J., Chen, S. Q., Zeng, B. S., James, A. A., Tan, A. J., and Huang, Y. P. (2017a). Bombyx mori P-element somatic inhibitor (BmPSI) is a key auxiliary factor for silkworm male sex determination. PLoS Genet. 13:e1006576. doi: 10.1371/journal.pgen.1006576
Xu, J., Zhan, S., Chen, S. Q., Zeng, B. S., Li, Z. Q., James, A. A., et al. (2017b). Sexually dimorphic traits in the silkworm, Bombyx mori, are regulated by doublesex. Insect Biochem. Mol. Biol. 80, 42–51. doi: 10.1016/j.ibmb.2016.11.005
Keywords: Bombyx mori, ion transport peptide receptor, CRISPR/Cas9, water homeostasis, development
Citation: Sun L, Zhang Z, Zhang R, Yu Y, Yang F and Tan A (2020) Molecular Disruption of Ion Transport Peptide Receptor Results in Impaired Water Homeostasis and Developmental Defects in Bombyx mori. Front. Physiol. 11:424. doi: 10.3389/fphys.2020.00424
Received: 13 December 2019; Accepted: 07 April 2020;
Published: 20 May 2020.
Edited by:
Bin Tang, Hangzhou Normal University, ChinaCopyright © 2020 Sun, Zhang, Zhang, Yu, Yang and Tan. This is an open-access article distributed under the terms of the Creative Commons Attribution License (CC BY). The use, distribution or reproduction in other forums is permitted, provided the original author(s) and the copyright owner(s) are credited and that the original publication in this journal is cited, in accordance with accepted academic practice. No use, distribution or reproduction is permitted which does not comply with these terms.
*Correspondence: Anjiang Tan, dGFuYW5qaWFuZ0BjZW1wcy5hYy5jbg==