- 1Department of Infectious Diseases, The First Affiliated Hospital of Wenzhou Medical University, Zhejiang Provincial Key Laboratory for Accurate Diagnosis and Treatment of Chronic Liver Diseases, Hepatology Institute of Wenzhou Medical University, Wenzhou, China
- 2Department of Gastroenterology, Peking University First Hospital, Beijing, China
Impaired intestinal barrier function and oxidative stress injury play critical roles in the pathogenesis of alcoholic liver disease (ALD), and recent investigations have revealed a role for dietary copper in the liver and intestinal barrier function. Therefore, the current study investigates the mechanisms and role of dietary copper in alcohol induced liver diseases. C57BL/6 mice were used to create an alcoholic liver disease model with a Lieber-DeCarli diet containing 5% alcohol and were fed with different concentrations of dietary copper of adequate (6 ppm, CuA), marginal (1.5 ppm, CuM), or supplemental (20 ppm, CuS) amounts. Caco-2 cells were also exposed to ethanol and different concentrations of copper. Damages of the liver and intestine were evaluated by transaminases, histology staining, and protein and mRNA level, as well as cell proliferation, oxidative stress, and mitochondrial membrane potential. In animal experiments, the results indicate that an alcohol diet causes liver injury and disruption of intestinal barrier function as well as decreasing the expression of genes such as HIF-1α, occludin, SOD1, and GPX1. Supplemental dietary copper can revert these changes except for SOD1, but marginal dietary copper can worsen these changes. The in vitro cell experiments showed that proper copper supplementation can promote cell growth and reduce reactive oxygen species (ROS) production. In conclusion, supplemental dietary copper has beneficial effects on alcohol-induced intestine and liver injury, and marginal dietary copper shows detrimental effects on these parameters.
Introduction
Alcoholic liver disease (ALD) arises due to excess use of alcohol for a long period of time and ranges from hepatic steatosis to steatohepatitis, cirrhosis, and even hepatocellular carcinoma (Seitz et al., 2018). ALD is a leading cause of morbidity and mortality worldwide, but its targeted therapy is limited (Bajaj, 2019; Ge et al., 2019). Therefore, research on mechanisms and treatment methods is urgently needed. Although the pathogenesis of ALD may involve many aspects (Rao, 2009; Gao and Bataller, 2011; Orman et al., 2013; Chen et al., 2016), recent studies have suggested that gut barrier dysfunction induced by alcohol, such as increased intestinal permeability, bacterial translocation, and release of bacteria-derived endotoxin into circulation, may play an important role in ALD development.
The barrier function of intestinal epithelial cells is regulated by a variety of factors, including oxygen (Rath et al., 2018). Moreover, alcohol-induced oxidative stress and inflammatory reactions can aggravate tissue hypoxia (Louvet and Mathurin, 2015). Since hypoxia-inducible factor-1α (HIF-1α) is a regulatory subunit of the hypoxia-inducible factor (HIF), which regulates a wide range of genes involved in cellular responses to hypoxia and other tissue environmental cues (Prabhakar and Semenza, 2012), HIF-1α should play an important role in maintaining barrier functions of intestinal epithelial cells. In addition, HIF-1α was significantly increased in hepatic fibrotic tissues and activated hepatic stellate cells (HSCs) (Moon et al., 2009), which are the consequences of alcoholic diseases. However, the role of HIF-1α in alcoholic diseases still remains to be investigated.
An adequate supply of copper is essential for healthy life, as chronic copper deficiency can elicit anemia, leucopenia, myelopathy, or skin abnormalities (Altarelli et al., 2019). Moreover, copper deficiency in rodent shows an adverse effect on lipid metabolism (Al-Othman et al., 1992, 1993). Furthermore, several investigations revealed that copper might be involved in the regulation of gut microbiota and gut barrier function (Song et al., 2018). For example, copper has been used as an antimicrobial agent throughout the ages (Hodgkinson and Petris, 2012), and the response to copper stress varies greatly among different bacterial species (Pontel and Soncini, 2009; Solioz et al., 2010). However, there are no copper-related drugs for the clinical treatment of alcoholic liver. In addition, low copper availability was observed in NAFLD patients, and a copper-deficient diet induces fatty liver in rodents (Aigner et al., 2008, 2010). However, whether and how dietary copper contributes to the development of ALD through altering gut barrier function and oxidative stress remain unknown. Therefore, in this research, we employed mice and Caco-2 cells to investigate the role of dietary copper in the development of alcoholic hepatic steatosis and intestinal epithelial functions.
Materials and Methods
Animal Experiments
As shown in Figure 1, seventy-two C57BL/6 wild type (WT) mice (6 weeks of age, male) from the Shanghai Laboratory Animal Center (Shanghai, China) were fed (ad lib.) with the Lieber-DeCarli control diet (Trophic Animal Feed High-tech Co., Jiangsu, China) with a defined copper content in the form of cupric carbonate (Bertola et al., 2013). In order to adapt the animals from solid feed to liquid feed, the mice received 1.5, 6.0, or 20 ppm of copper as marginal, adequate, or supplemental doses, respectively, for 5 days. They were then divided into two groups: a control group (C) and an alcoholic steatosis model group (M). For the purpose of reducing the impact of alcohol on animal function and decreasing mortality, mice were fed a mixed diet of which the ratio (Lieber-DeCarli alcohol diet: Lieber-DeCarli control diet) changed from 2:1 to 1:1 to 1:2 at days 2, 4, and 6, respectively, for 1 week in the model group and were then fed with the Lieber-DeCarli alcohol diet to general steatosis model. In the control group, mice were fed with the Lieber-DeCarli control diet with the same copper content as mice in the model group for 6 weeks. On the 28th day, a bolus of ethanol (5 g/kg body weight, 40%) or isocaloric dextrin (8.9 g/kg body weight) was gavaged in the model and control groups, respectively. After 10 h of gavage, all mice were euthanized to collect blood, liver, and intestinal tissue. Mice were treated according to the protocols reviewed and approved by the Institutional Ethics Committee of Wenzhou Medical University, which were in accordance with the Guiding Principles for the Care and Use of Laboratory Animals in China.
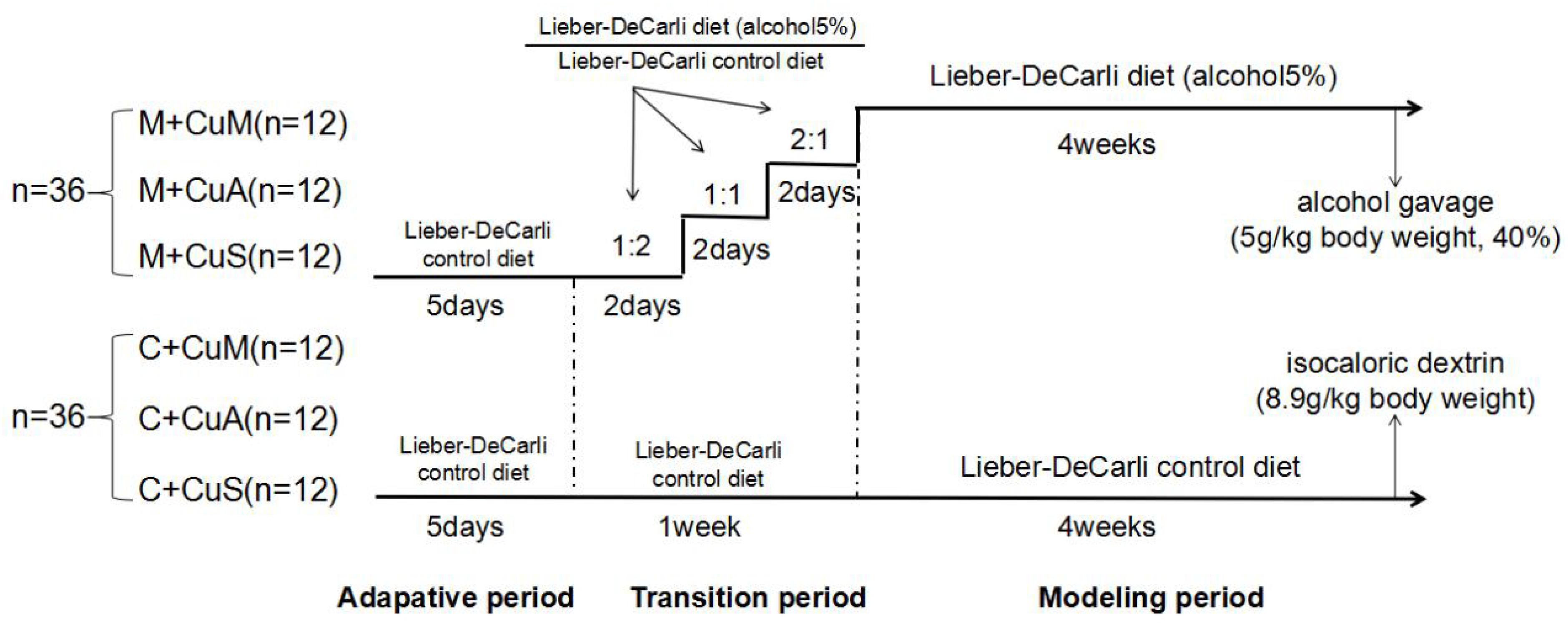
Figure 1. Animal feeding schedule. M + CuM: model group + marginal Cu level, M + CuA: model group + adequate Cu level, M + CuS: model group + supplemental Cu level, C + CuM: control group + marginal Cu level, C + CuA: control group + adequate Cu level, C + CuS: control group + supplemental Cu level.
Measurement of Serum Copper, ALT, and AST Levels
The collected blood was centrifuged at 1500 rpm for 10 min to obtain serum. The levels of serum copper, ALT, and AST were measured by using an automated biochemical analyzer (Abbott Laboratories, Chicago, IL, United States) in the clinical biochemical laboratory of the First Affiliated Hospital of Wenzhou Medical University.
Liver and Small Intestine Histopathology
Harvested liver and upper small intestine samples were fixed in 4% paraformaldehyde and embedded in paraffin, then cut into 4-μm sections and processed for staining with hematoxylin and eosin (H&E). Moreover, sections of the liver were quickly frozen and then cut into 4-μm sections and stained with Oil Red O. Both were analyzed by light microscopy.
Real-Time Reverse Transcriptase-Polymerase Chain Reaction (RT-PCR) Assay
Total RNA in tissues was separately extracted according to the instructions of the RNA extraction kit (Aidlab Biotechnologies Co., Beijing, China), and 1 μg total RNA was reverse-transcribed into cDNA using the PrimeScriptTM RTreagent Kit (Perfect Real Time; Aidlab Biotechnologies Co., Beijing, China). Genes related to HIF-1α and small intestine functions (Occludin, SOD1, and GPX1) were examined. The relevant cDNA was then amplified using the SYBR PremixExTaq II (TOYOBO, Osaka, Japan) with specific primers as listed in Table 1. RT-PCR was performed by a 7500 Real-Time PCR System (Applied Biosystems, Life Technologies, Waltham, MA, United States). Each mRNA expression was calculated based on glyceraldehyde-3-phosphate dehydrogenation (GAPDH) as a reference gene. The final mRNA expression was analyzed by the 2−ΔΔCt method.
Western Blot Analysis
Total protein from tissues was extracted in a mixture of ice-cold RIPA (Beyotime Biotechnology, China) and protease inhibitor. Proteins were separated by 10% SDS-PAGE and electro-transferred to PVDF membrane (Millipore, Burlington, MA, United States). The membranes were blocked for 2 h at room temperature with 5% BSA in TBST solution and then incubated with primary antibodies of anti-HIF-1α (1:1000, Abcam), anti-Occludin (1:1000, Proteintech), anti-SOD1 (1:1000, Proteintech), anti-GPX1 (1:1000, Affity) and anti-βactin (1:1000, Abcam), respectively, at 4°C overnight. After being washed with TBST, the membranes were incubated with the secondary antibody (goat anti-rabbit) (1:5000, Biosharp) for 1 h and were then washed three times with TBST. The bands were then detected and analyzed by Western Bright ECL (Advansta, San Jose, CA, United States) and Image Lab 4.1 software (Bio-Rad).
Caco-2 Cell Culture
Caco-2 cells were obtained from the Cell Bank of the Chinese Academy of Sciences (Shanghai, China) and cultured in DMEM (Gibco, United States) containing 10% fetal bovine serum (Gibco, United States), penicillin (100 U/ml), and streptomycin (100 U/ml) in a 5% CO2 humidified incubator at 37°C. The medium was changed every 2 days, and the growth of the cells was observed with an inverted microscope. The cell monolayer was used after 24 days of culture, at which time the cells have differentiated and matured. The cells were exposed to 100 mmol/L alcohol and Cu (added as CuSO4.5H2O; Macklin, Shanghai, China) with different concentrations (0, 3.125, 6.25, 12.5, and 25 μM).
Proliferation Assay
The effect of different concentrations of copper on Caco-2 proliferation was determined using real-time cellular analysis (RTCA). After 24 h stimulation, cells (8 × 103 cells/well) were seeded in several E-Plate 16 dishes (ACEA Biosciences Inc., CA, United States) for proliferation assays. The plates were kept in the cell incubator at 37°C with 5% CO2 for 3–4 days. The cell index and growth curves were automatically recorded on the xCELLigence RTCA System (ACEA Biosciences Inc., CA, United States).
Mitochondrial Membrane Potential Determination
The mitochondrial membrane potential (ΔΨm) was detected according to the manual of the JC-1 Assay Kit (Beyotime, Jiangsu, China). Briefly, the cells were suspended in 1 ml PBS after 48 h of stimulation at approximately 1 × 106 cells/mL and were incubated with 2 μM of JC-1 for 15 min at 37°C. The cells were washed and resuspended in 500 μL PBS and then analyzed on a flow cytometer with 488 nm excitation and emission at 590 nm (red) and 540 nm (green).
Cellular Total ROS Level Measurement
The relative level of cellular total reactive oxygen species (ROS) level was detected according to the Reactive Oxygen Species Assay Kit (Beyotime, Jiangsu, China). The cells were collected and incubated with DCFH-DA probe solution in a 37°C, 5% CO2 incubator for 20 min (final concentration is 10 μM). Next, they were centrifuged at 1000 rpm for 4 min and then washed three times with FBS-free DMEM to remove the residual probe. Finally, cell samples were resuspended in FBS-free DMEM and subjected to flow cytometry analysis. The mean value of fluorescence intensity indicates the relative level of cellular total ROS level.
Both mitochondrial membrane potential and cellular total ROS level were examined by using a BD Accuri C6 (BD Biosciences, Franklin Lakes, NJ, United States) flow cytometer.
Statistics and Data Analysis
Comparisons between multiple sets of data were performed using one-way analysis of variance (ANOVA), LSD, and Dunnett T3 tests in SPSS 22.0 software. Data are expressed as mean ± SD. P < 0.05 was considered statistically significant.
Results
Effects of Different Doses of Dietary Copper on Body Weight, Liver Weight, and Liver/Body Weight Ratio in Mice
As shown in Figure 2, alcohol feeding resulted in a significant decrease in body weight but a significant increase in liver weight and liver/body weight ratio. In addition, although the marginal copper diet significantly increased liver weight and the liver/body weight ratio in the control group, this has little practical significance. However, there was no obvious effect of copper on body weight or the liver/body weight ratio in the model group. The results showed that alcohol could damage the liver and cause liver fat deposition but that a beneficial effect of copper supplementation in the diet was not obvious. In the control group, the marginal copper diet was harmful to the liver.
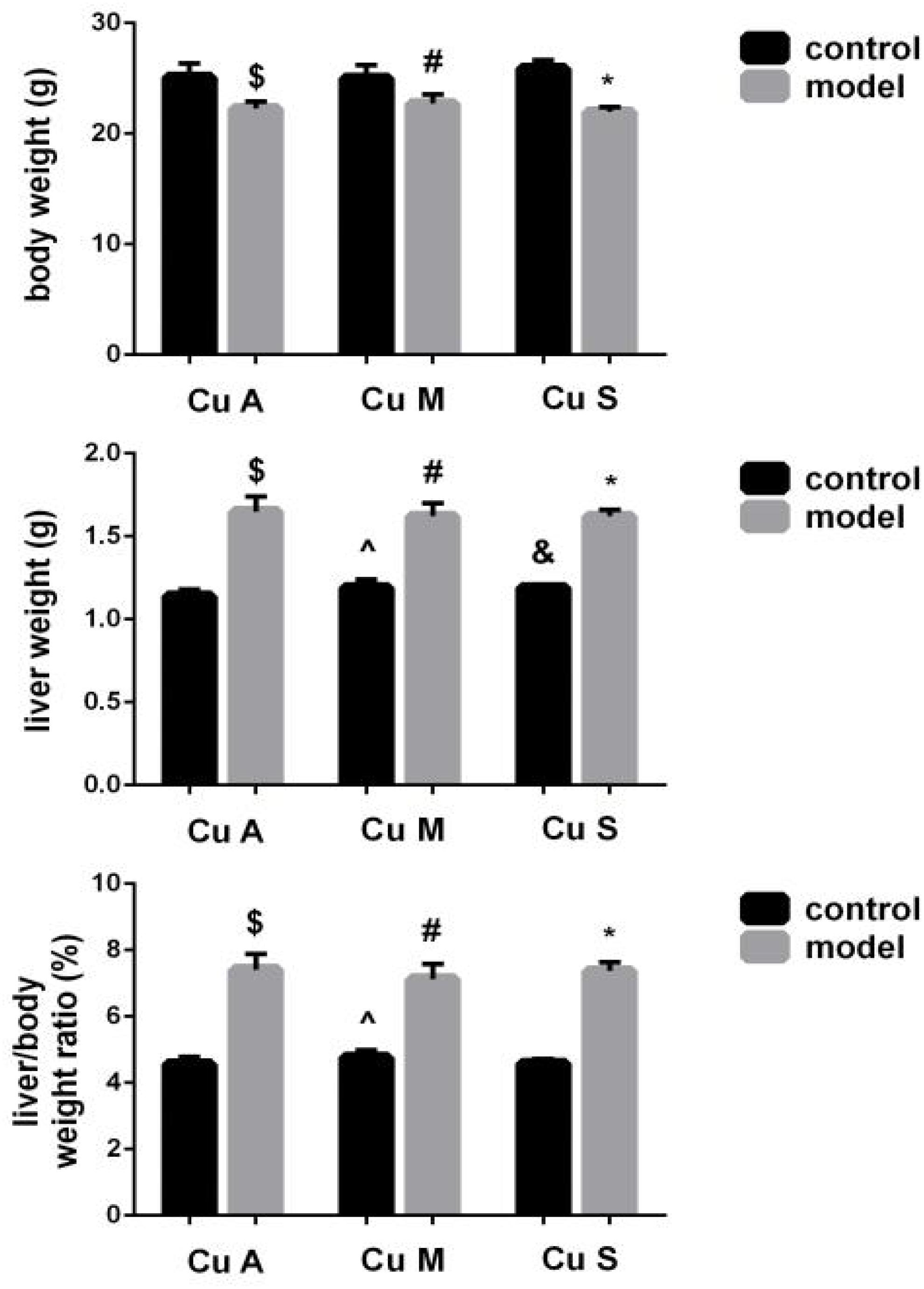
Figure 2. Effects of different doses of dietary copper on body weight, liver weight, and liver/body weight ratio in mice. Comparisons between multiple sets of data were performed using one-way analysis of variance (ANOVA). Data are presented as the mean ± SD (n = 12) of three independent experiments. Statistical significance was set at p < 0.05. $: M + CuA versus C + Cu A; #: M + CuM versus C + CuM; *: C + CuS versus C + CuS; ^: C + CuM versus C + CuA; &: C + CuS versus C + CuA; + : M + CuM versus M + CuA; ± : M + CuS versus M + CuA.
Serum Copper, ALT, and AST Levels in Response to Different Doses of Dietary Copper in Mice
Liver ALT and AST were significantly increased and the serum copper was decreased in the model group (Figure 3). Whether in the control group or the model group, the marginal copper diet can increase ALT and AST and reduce serum copper compared to an adequate copper diet. In contrast, in the model group, copper-supplemented mice had lower ALT and AST values, and serum copper increased. Therefore, alcohol could disrupt the homeostasis of serum copper, damage the liver, and cause elevated aminotransferases. In the model group and the control group, supplemental levels of copper in the diet caused a significant improvement, while marginal copper had a harmful effect.
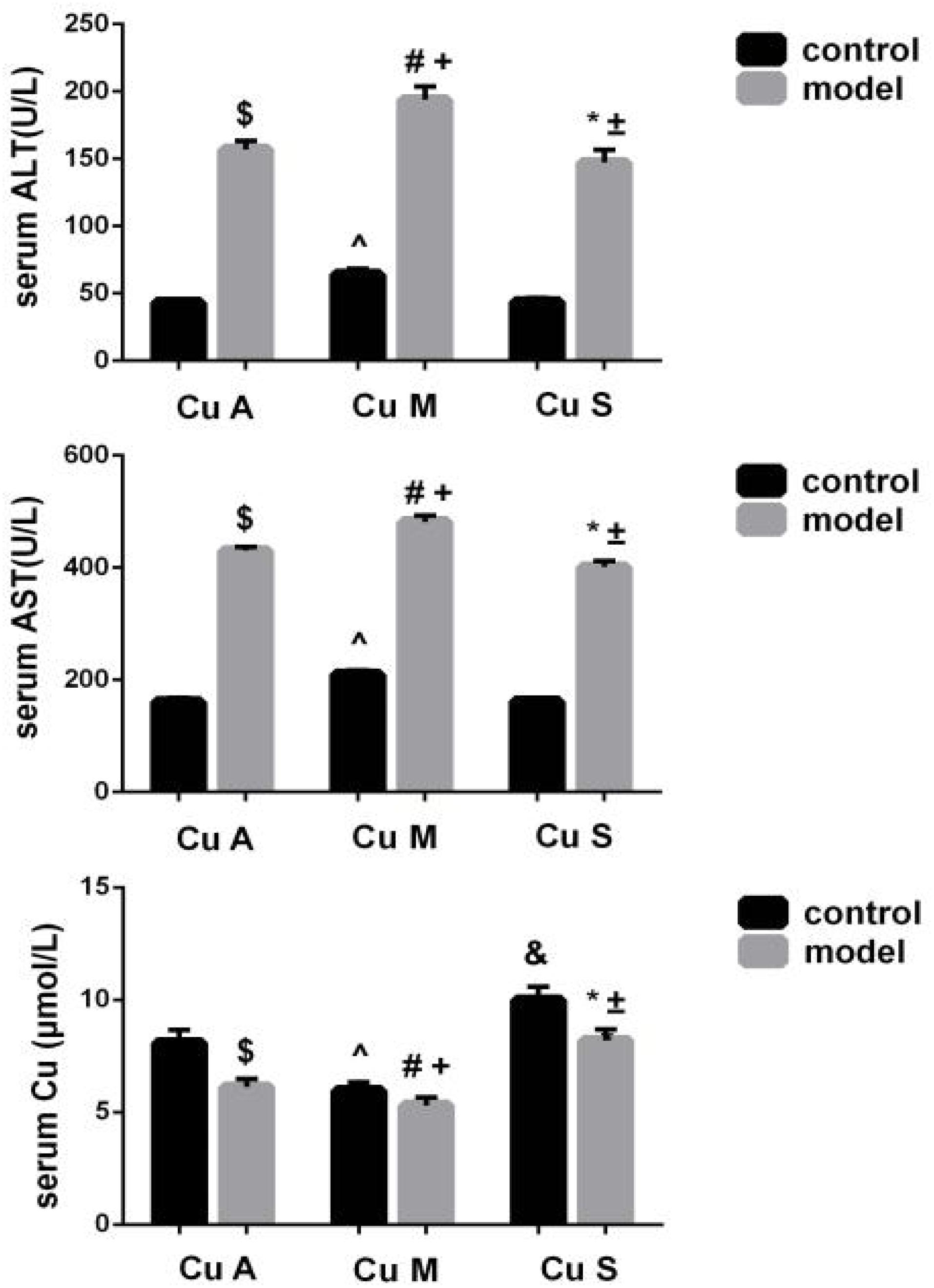
Figure 3. Serum copper, ALT, and AST levels in response to different dietary doses of copper feeding, with or without alcohol. Comparisons between multiple sets of data were performed using one-way analysis of variance (ANOVA). Data are presented as the mean ± SD (n = 12) of three independent experiments. Statistical significance was set at p < 0.05. $: M + CuA versus C + CuA; #: M + CuM versus C + CuM; *: C + CuS versus C + Cu S; ^: C + CuM versus C + CuA; &: C + CuS versus C + CuA; + : M + CuM versus M + CuA; ± : M + CuS versus M + CuA.
Effect of Different Doses of Dietary Copper on Liver Histology in Mice
Liver injury was evaluated by liver histology in mice, as shown in Figure 4. Significant liver injury and lipid deposition were observed in the liver of model mice as compared with that in control mice. Moreover, copper supplementation could slightly alleviate liver lipid deposition, while marginal copper exacerbated this change in the control and model groups. The results showed that the damage done by alcohol to the liver was obvious and that copper supplementation in the diet could appropriately alleviate this change, whereas a marginal copper diet exacerbated the situation.
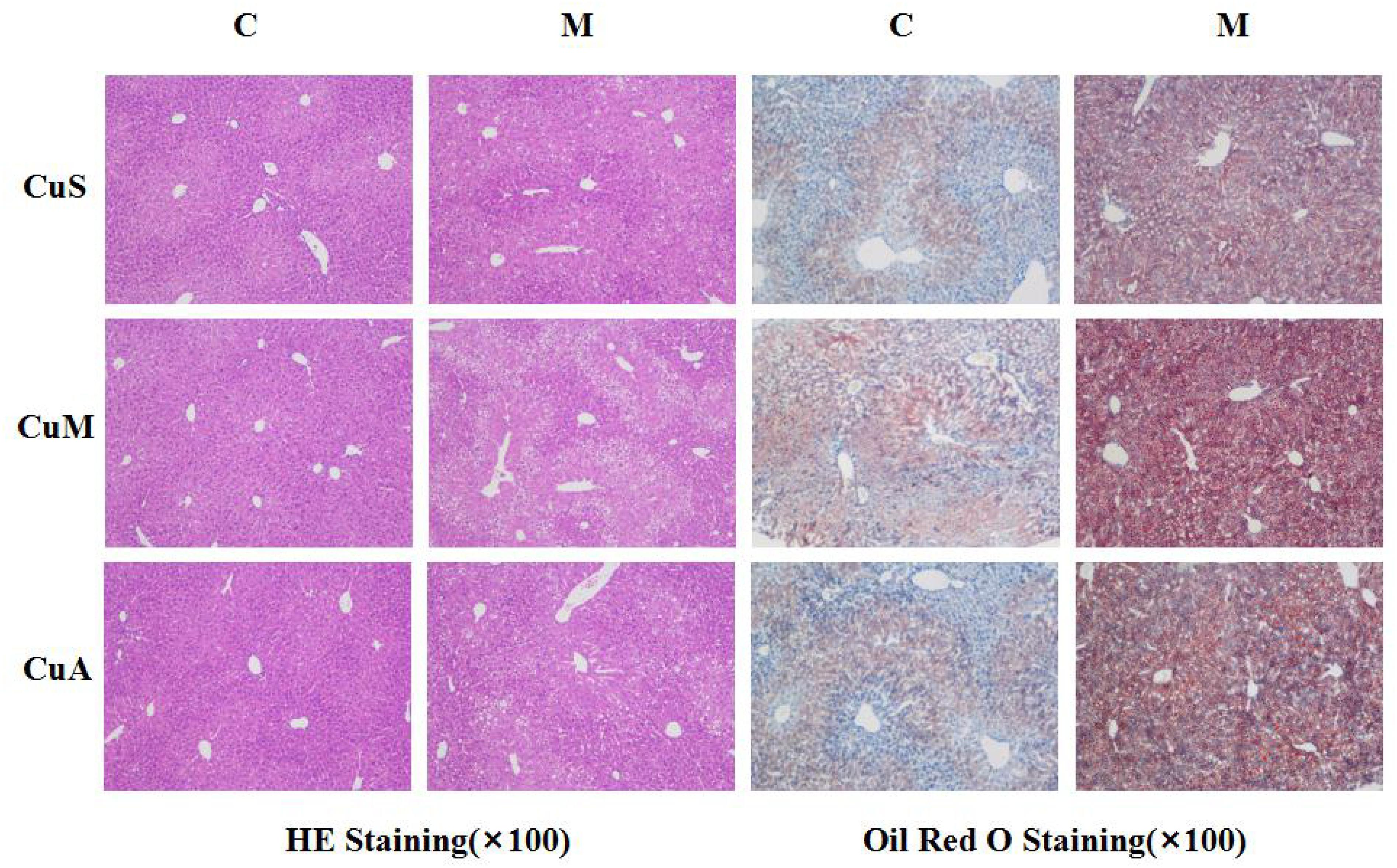
Figure 4. Histology of liver stained with HE and Oil Red O. CuM: marginal Cu level (1.5 ppm); CuA: adequate Cu level (6 ppm); CuS: supplemental Cu level (20 ppm). C: control group; M: model group.
Effect of Different Doses of Dietary Copper on the Histology and Gene Expression of Small Intestine
The histology of small intestine and gene expression in small intestine are shown in Figures 5–7. As shown in Figure 5, alcohol intake caused the intestinal villi to become thinner, shorter, and more irregular. Moreover, in the model group, the marginal copper diet exacerbated the alcohol-induced intestinal villus destruction, while the supplemental copper diet alleviated it. In the control group, the marginal copper diet also induced changes in intestinal villi, but the supplemental copper diet had no obvious impact on intestinal villi. The genes related to intestinal barrier function and HIF-1α were evaluated, as shown in Figures 6, 7. Alcohol intake resulted in a significant decrease in protein and mRNA levels of occludin, GPX1, and SOD1. The marginal copper diet exacerbated the change, and the supplemental copper diet ameliorated it, except for SOD1 protein expression in the model group. Moreover, alcohol decreased the HIF-1α gene expression in intestine, which suggests a role for HIF-1α in alcohol-induced intestinal injury. The mRNA and protein levels of HIF-1α were lowest in mice fed with the marginal copper diet in both the control and model groups, while the supplemental copper diet appeared to restore the HIF-1α level. The above research results showed that alcohol could damage the intestine by down-regulating the HIF-1α pathway and reducing occludin, SOD1, and GPX1 expression. Copper supplementation in the diet could alleviate these changes, except for that in SOD1, and the marginal copper diet would aggravate all these changes.
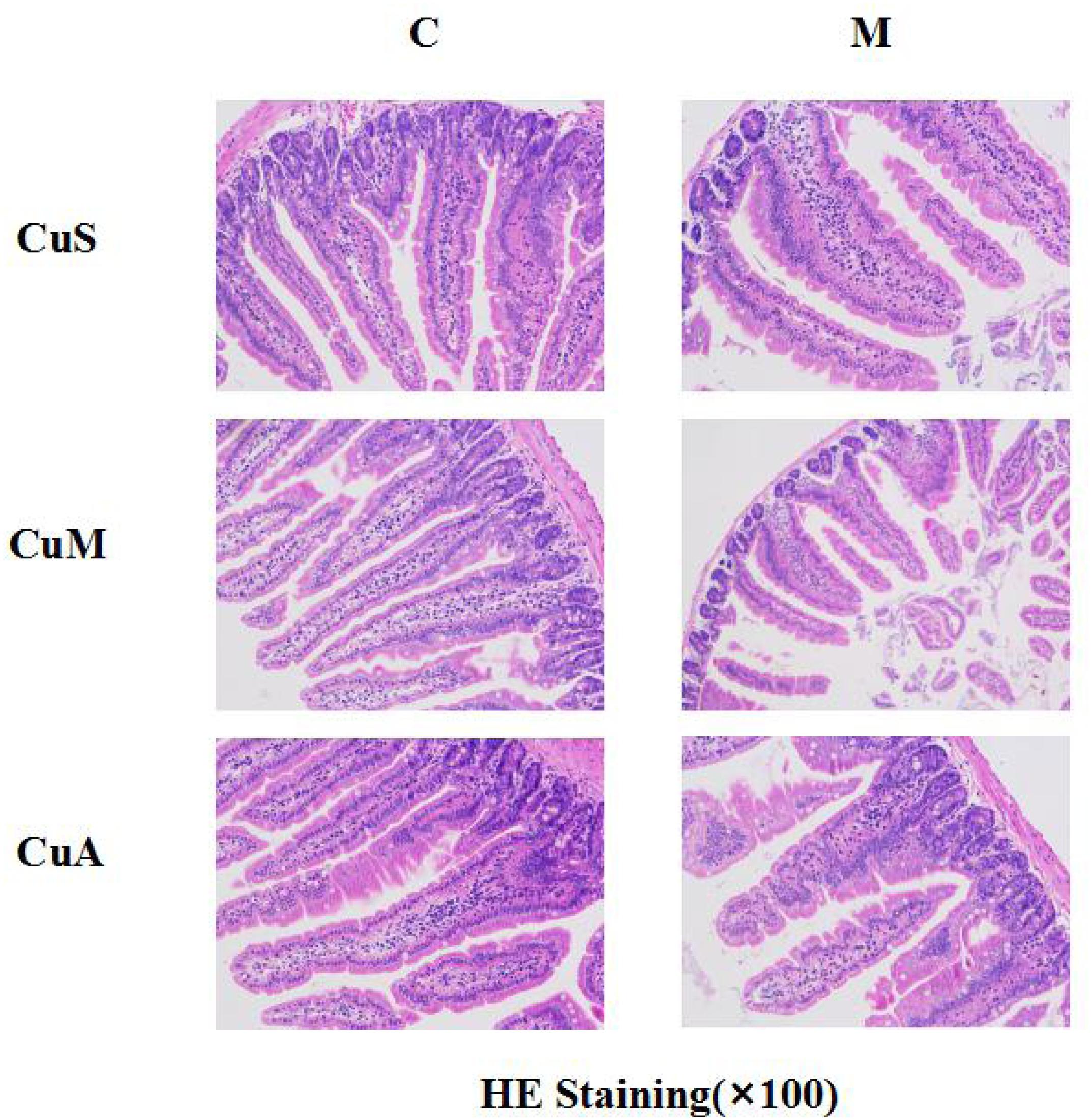
Figure 5. Histology of small intestine stained with HE. CuM: marginal Cu level (1.5 ppm); CuA: adequate Cu level (6 ppm); CuS: supplemental Cu level (20 ppm). C: control group; M: model group.
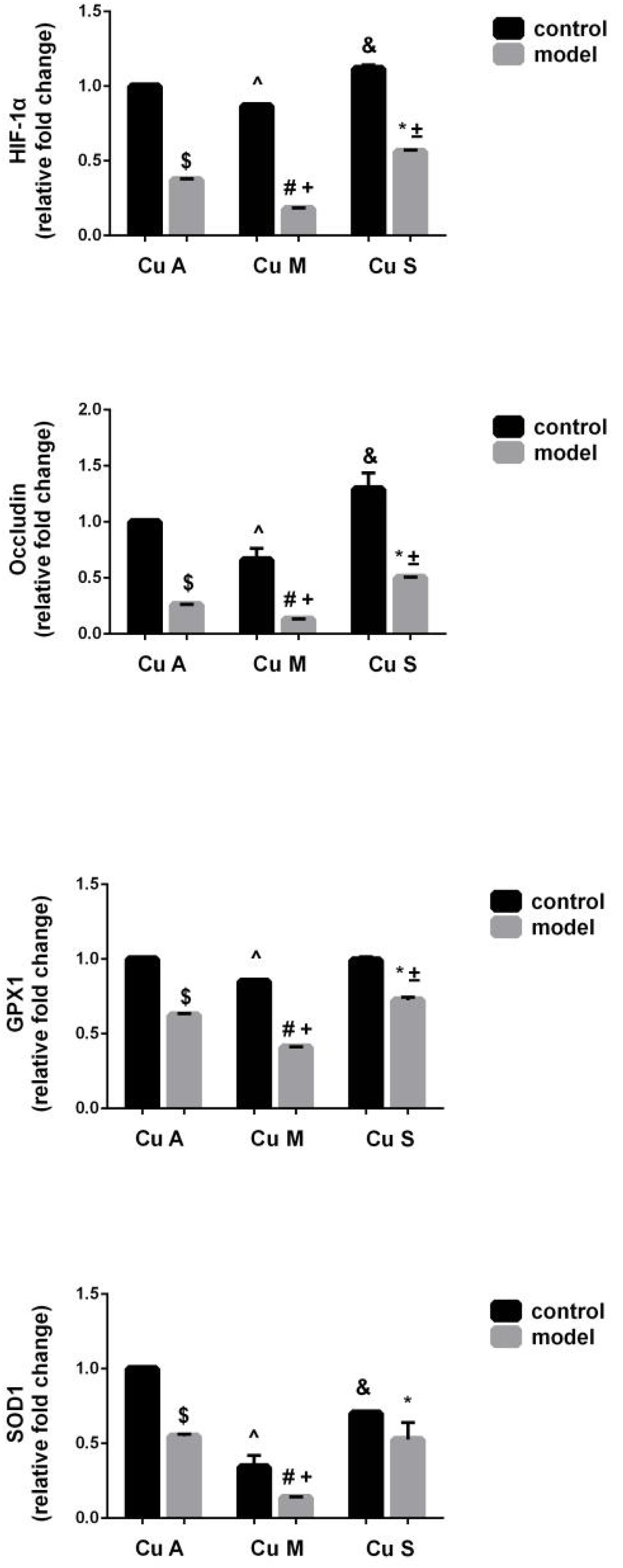
Figure 6. mRNA levels of intestinal HIF-1α, Occludin, GPX1, and SOD1 evaluated by RT-PCR, normalized to the levels of GAPDH. Comparisons between multiple sets of data were performed using one-way analysis of variance (ANOVA). Data are presented as the mean ± SD (n = 12) of three independent experiments. Statistical significance was set at p < 0.05. $: M + CuA versus C + CuA; #: M + CuM versus C + CuM; *: C + CuS versus C + CuS; ^: C + CuM versus C + CuA; &: C + CuS versus C + CuA; + : M + CuM versus M + CuA; ± : M + CuS versus M + CuA.
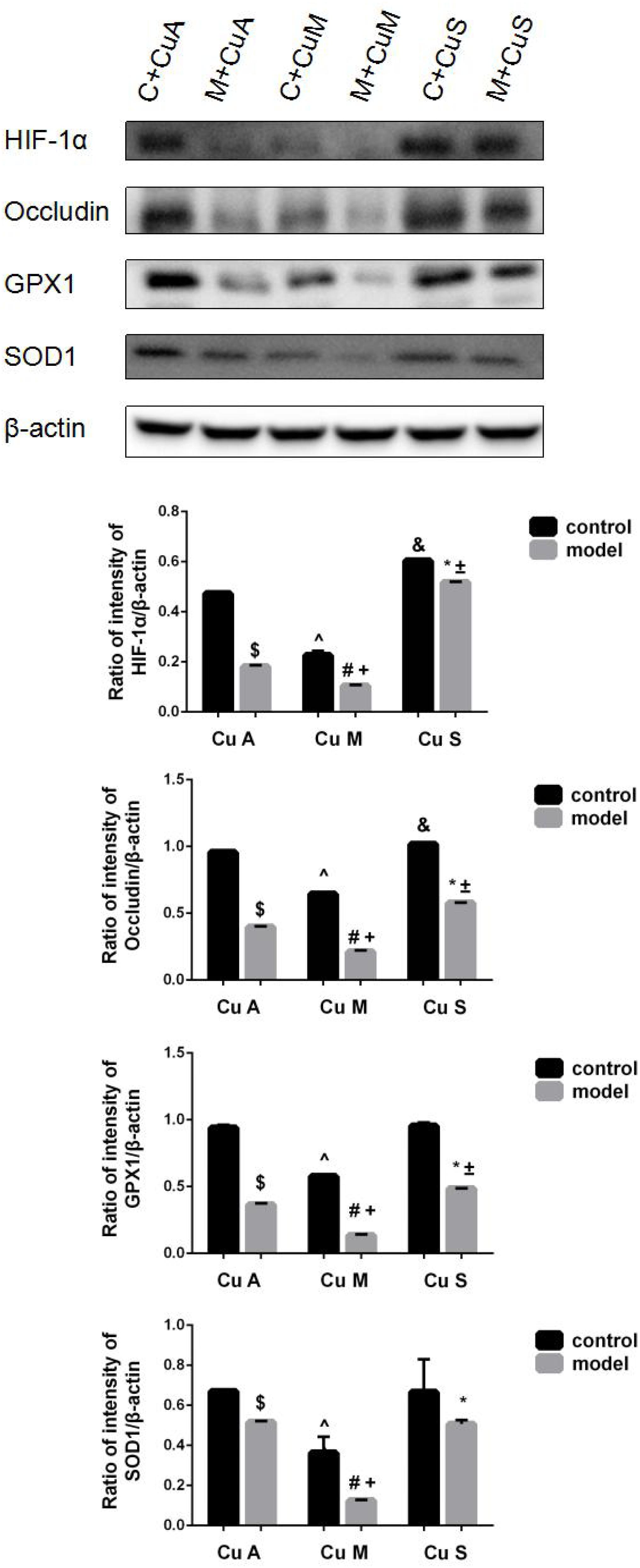
Figure 7. Protein levels of intestinal HIF-1α, Occludin, GPX1, and SOD1 evaluated by WB, normalized to the levels of β-actin. Comparisons between multiple sets of data were performed using one-way analysis of variance (ANOVA). Data are presented as the mean ± SD (n = 12) of three independent experiments. Statistical significance was set at p < 0.05. $: M + CuA versus C + CuA; #: M + CuM versus C + CuM; *: C + CuS versus C + CuS; ^: C + CuM versus C + CuA; &: C + CuS versus C + CuA; + : M + CuM versus M + CuA; ± : M + CuS versus M + CuA.
Effect of Different Doses of Dietary Copper on Caco-2 Cells
Since different concentrations of dietary copper can affect liver steatosis and small intestinal barrier function, further investigation of the effects of dietary copper was performed on small intestinal cells (Caco-2 cell line). As shown in Figure 8A, with an increase in the concentration of dietary copper, there was an increase in cell proliferation in an almost dose-dependent manner. Copper also affects ROS production and mitochondrial membrane potential in Caco-2 cells. As shown in Figure 8B, copper decreased ROS production in Caco-2 cells in an almost dose-dependent manner. The same observation was found in mitochondrial membrane potential (Figure 8C). The above results showed that in vitro experiments, proper copper supplementation was beneficial for small intestinal cells (Caco-2 cell line) but harmful to mitochondrial membrane potential.
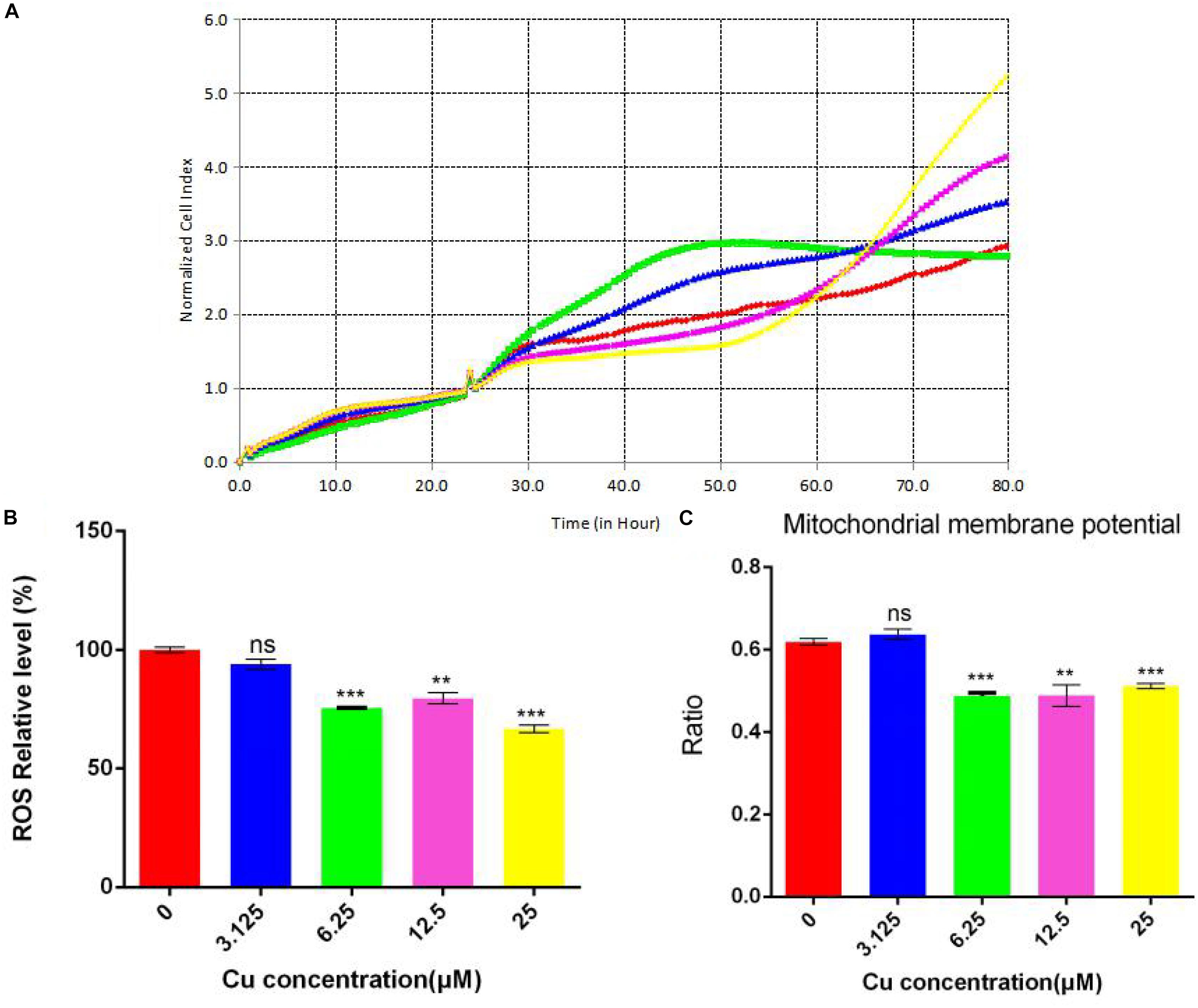
Figure 8. Evaluation of functional mitochondria. (A) Proliferation assay real-time cellular analysis (RTCA), (B) Cellular total ROS level measurement, (C) Mitochondrial membrane potential determination. Comparisons between multiple sets of data were performed using one-way analysis of variance (ANOVA). Data are presented as the mean ± SD (n = 12) of three independent experiments. Statistical significance was set at **P < 0.05, ***P < 0.01. ns: not significant.
Discussion
The liver is one of the most important organs of the human body and plays an extremely important role in metabolism (Lackner and Tiniakos, 2019). Since Marshall proposed the concept of the intestinal hepatic axis in 1998, research on the relationship between intestinal and liver diseases has attracted more and more attention (Tripathi et al., 2018). It is currently believed that the intestine and the liver interact with each other, and protection of intestinal mucosal barrier integrity has been a major focus for the development of new treatments for liver diseases (Bird, 2012; Llopis et al., 2016).
The intestines are rich in blood vessels and are therefore susceptible to diseases associated with reduced blood flow and concomitant tissue hypoxia (Taylor and Colgan, 1999; Furuta et al., 2001). Hypoxia-inducible factor 1α (HIF-1α) has been implicated in transcriptional regulation of intestinal barrier integrity and inflammation (Shao et al., 2018). Disruption of epithelial HIF-1α can lead to increased epithelial permeability (Karhausen et al., 2004). Metabolism in the intestine is very active, and thus it is highly vulnerable to oxidative stress (Glover et al., 2016; Rath et al., 2018). Superoxide dismutase 1 (SOD1) and glutathione peroxidase 1 (GPX1) are the most important antioxidant enzymes in cells (Weydert and Cullen, 2010). Tight junction (TJ) is the main means of connection between intestinal epithelial cells and has a major effect on maintaining the polarity of epithelial cells and regulating the permeability of the intestinal barrier (Umeda et al., 2006). Occludin is one of the most important protein molecules in TJ (Du et al., 2010).
In the current study, we found that alcohol intake could induce liver injury and impair gut barrier function. Alcohol caused intestinal villi to become thinner and disordered, decreased the expression of genes such as HIF-1α, occludin, GPX1, and SOD1, and impaired intestinal barrier function. Disorders in intestinal morphology and functions induced by alcohol can be alleviated with supplemental dietary copper and exacerbated with marginal dietary copper. However, supplementation with copper did not produce the expected ameliorative effect on SOD1; it may not be sensitive to increased copper content and may only be more sensitive to copper reduction.
Copper is a necessary mineral element in the diet and has been shown to play roles in antioxidant defense, lipid peroxidation, and mitochondrial function (Al-Othman et al., 1992, 1993). Copper deficiency has been linked to atherogenic dyslipidemia (Aigner et al., 2010). Systemic copper deficiency can also cause mitochondrial dysfunction in mice (Nose et al., 2006), and similar morphological and functional alterations have also been described in human NAFLD (Wei et al., 2008). In the current study, we also found that mice fed with marginal dietary copper also showed damage to the liver and gut without alcohol intake. However, the effect of supplemental dietary copper on control mice was still unclear; it might have a positive effect on the intestine but had no effect on oxidative stress. All of these findings indicated that dietary copper could alter intestinal barrier function through regulation of the intestinal HIF-1α signaling pathway and oxidative stress in mice.
ROS is formed as a natural by-product of the normal metabolism of oxygen and plays an important role in cell signaling and homeostasis. When cell homeostasis is disrupted, ROS levels increase dramatically. This can cause severe damage to the cell structure, which is called oxidative stress. Normal MMP is a prerequisite for maintaining oxidative phosphorylation of mitochondria and producing adenosine triphosphate. The stability of MMP is conducive to maintaining the normal physiological functions of cells. The in vitro cell experiments demonstrated an important role of copper in cell proliferation, ROS production, and mitochondrial membrane potential. With an increase in copper concentration, there was an increase in cell proliferation but a decrease in cellular total ROS level. This means that copper could reduce ROS production and promote cell proliferation. Although there is a slight increase in mitochondrial membrane potential at low copper concentration (3.125 μM), a significant decrease in mitochondrial membrane potential was observed with increases in copper concentration. However, the functioning of mitochondria remained intact, which suggested that the membrane potential was more sensitive to changes in the concentration of copper. However, further investigations are needed to understand the relationship between copper and mitochondrial functions.
In summary, our current study provides novel discovery of the pathogenesis of ALD, which indicates that copper homeostasis is critical in maintaining gut barrier integrity and plays an important role in the prevention of ALD. The mechanism by which copper prevents ALD may be related to its regulation of intestinal HIF-1α gene expression and oxidative stress.
Data Availability Statement
All datasets generated for this study are included in the article/supplementary material.
Ethics Statement
All mice were treated according to the protocols reviewed and approved by the institutional ethics committee of Wenzhou Medical University.
Author Contributions
LX and Y-PC designed the protocol. HL and DC performed the experiments and edited the manuscript. QD and TP directed and participated in the sample detection. HT, YX, TT, and JT analyzed the data. HL wrote the manuscript, which was also edited by DC. All authors read and approved the final manuscript.
Funding
This work was supported by the National Natural Science Foundation of China (grant numbers 81770585, 81570514, and 81600466) and the National Science and Technology Major Project (grant numbers 2017ZX10203201-002-003, 2017ZX10202201, and 2018ZX10725506-001).
Conflict of Interest
The authors declare that the research was conducted in the absence of any commercial or financial relationships that could be construed as a potential conflict of interest.
Acknowledgments
We heartily appreciate all those who contributed to this research.
References
Aigner, E., Strasser, M., Haufe, H., Sonnweber, T., Hohla, F., Stadlmayr, A., et al. (2010). A role for low hepatic copper concentrations in nonalcoholic Fatty liver disease. Am. J. Gastroenterol. 105, 1978–1985.
Aigner, E., Theurl, I., Haufe, H., Seifert, M., Hohla, F., Scharinger, L., et al. (2008). Copper availability contributes to iron perturbations in human nonalcoholic fatty liver disease. Gastroenterology 135, 680–688.
Al-Othman, A. A., Rosenstein, F., and Lei, K. Y. (1992). Copper deficiency alters plasma pool size, percent composition and concentration of lipoprotein components in rats. J. Nutr. 122, 1199–1204.
Al-Othman, A. A., Rosenstein, F., and Lei, K. Y. (1993). Copper deficiency increases in vivo hepatic synthesis of fatty acids, triacylglycerols, and phospholipids in rats. Proc. Soc. Exp. Biol. Med. 204, 97–103.
Altarelli, M., Ben-Hamouda, N., Schneider, A., and Berger, M. M. (2019). Copper deficiency: causes, manifestations, and treatment. Nutr. Clin. Pract. 34, 504–513.
Bajaj, J. S. (2019). Alcohol, liver disease and the gut microbiota. Nat. Rev. Gastroenterol. Hepatol. 16, 235–246.
Bertola, A., Mathews, S., Ki, S. H., Wang, H., and Gao, B. (2013). Mouse model of chronic and binge ethanol feeding (the NIAAA model). Nat. Protoc. 8, 627–637.
Chen, R. C., Xu, L. M., Du, S. J., Huang, S. S., Wu, H., Dong, J.-J., et al. (2016). Lactobacillus rhamnosus GG supernatant promotes intestinal barrier function, balances Treg and TH17 cells and ameliorates hepatic injury in a mouse model of chronic-binge alcohol feeding. Toxicol. Lett. 241, 103–110.
Du, D., Xu, F., Yu, L., Zhang, C., Lu, X., Yuan, H., et al. (2010). The tight junction protein, occludin, regulates the directional migration of epithelial cells. Dev. Cell 18, 52–63.
Furuta, G. T., Turner, J. R., Taylor, C. T., Hershberg, R. M., Comerford, K., Narravula, S., et al. (2001). Hypoxia-inducible factor 1-dependent induction of intestinal trefoil factor protects barrier function during hypoxia. J. Exp. Med. 193, 1027–1034.
Gao, B., and Bataller, R. (2011). Alcoholic liver disease: pathogenesis and new therapeutic targets. Gastroenterology 141, 1572–1585.
Ge, L., Chen, D., Chen, W., Cai, C., Tao, T., Lin, Z., et al. (2019). Pre-activation of TLR3 enhances the therapeutic effect of BMMSCs through regulation the intestinal HIF-2alpha signaling pathway and balance of NKB cells in experimental alcoholic liver injury. Int. Immunopharmacol. 70, 477–485.
Glover, L. E., Lee, J. S., and Colgan, S. P. (2016). Oxygen metabolism and barrier regulation in the intestinal mucosa. J. Clin. Invest. 126, 3680–3688.
Hodgkinson, V., and Petris, M. J. (2012). Copper homeostasis at the host-pathogen interface. J. Biol. Chem. 287, 13549–13555.
Karhausen, J., Furuta, G. T., Tomaszewski, J. E., Johnson, R. S., Colgan, S. P., Haase, V. H., et al. (2004). Epithelial hypoxia-inducible factor-1 is protective in murine experimental colitis. J. Clin. Invest. 114, 1098–1106.
Lackner, C., and Tiniakos, D. (2019). Fibrosis and alcohol-related liver disease. J. Hepatol. 70, 294–304.
Llopis, M., Cassard, A. M., Wrzosek, L., Boschat, L., Bruneau, A., Ferrere, G., et al. (2016). Intestinal microbiota contributes to individual susceptibility to alcoholic liver disease. Gut 65, 830–839.
Louvet, A., and Mathurin, P. (2015). Alcoholic liver disease: mechanisms of injury and targeted treatment. Nat. Rev. Gastroenterol. Hepatol. 12, 231–242.
Moon, J. O., Welch, T. P., Gonzalez, F. J., and Copple, B. L. (2009). Reduced liver fibrosis in hypoxia-inducible factor-1alpha-deficient mice. Am. J. Physiol. Gastrointest. Liver Physiol. 296, G582–G592.
Nose, Y., Kim, B. E., and Thiele, D. J. (2006). Ctr1 drives intestinal copper absorption and is essential for growth, iron metabolism, and neonatal cardiac function. Cell Metab. 4, 235–244.
Orman, E. S., Odena, G., and Bataller, R. (2013). Alcoholic liver disease: pathogenesis, management, and novel targets for therapy. J. Gastroenterol. Hepatol. 28(Suppl. 1), 77–84.
Pontel, L. B., and Soncini, F. C. (2009). Alternative periplasmic copper-resistance mechanisms in Gram negative bacteria. Mol. Microbiol. 73, 212–225.
Prabhakar, N. R., and Semenza, G. L. (2012). Adaptive and maladaptive cardiorespiratory responses to continuous and intermittent hypoxia mediated by hypoxia-inducible factors 1 and 2. Physiol. Rev. 92, 967–1003.
Rao, R. (2009). Endotoxemia and gut barrier dysfunction in alcoholic liver disease. Hepatology 50, 638–644.
Rath, E., Moschetta, A., and Haller, D. (2018). Mitochondrial function - gatekeeper of intestinal epithelial cell homeostasis. Nat. Rev. Gastroenterol. Hepatol. 15, 497–516.
Seitz, H. K., Bataller, R., Cortez-Pinto, H., Gao, B., Gual, A., Lackner, C., et al. (2018). Alcoholic liver disease. Nat. Rev. Dis. Primers 4:16.
Shao, T., Zhao, C., Li, F., Gu, Z., Liu, L., Zhang, L., et al. (2018). Intestinal HIF-1alpha deletion exacerbates alcoholic liver disease by inducing intestinal dysbiosis and barrier dysfunction. J. Hepatol. 69, 886–895.
Solioz, M., Abicht, H. K., Mermod, M., and Mancini, S. (2010). Response of gram-positive bacteria to copper stress. J. Biol. Inorg. Chem. 15, 3–14.
Song, M., Li, X., Zhang, X., Shi, H., Vos, M. B., Wei, X., et al. (2018). Dietary copper-fructose interactions alter gut microbial activity in male rats. Am. J. Physiol. Gastrointest. Liver Physiol. 314, G119–G130.
Taylor, C. T., and Colgan, S. P. (1999). Therapeutic targets for hypoxia-elicited pathways. Pharm. Res. 16, 1498–1505.
Tripathi, A., Debelius, J., Brenner, D. A., Karin, M., Loomba, R., Schnabl, B., et al. (2018). The gut-liver axis and the intersection with the microbiome. Nat. Rev. Gastroenterol. Hepatol. 15, 397–411.
Umeda, K., Ikenouchi, J., Katahira-Tayama, S., Furuse, K., Sasaki, H., Nakayama, M., et al. (2006). ZO-1 and ZO-2 independently determine where claudins are polymerized in tight-junction strand formation. Cell 126, 741–754.
Wei, Y., Rector, R. S., Thyfault, J. P., and Ibdah, J. A. (2008). Nonalcoholic fatty liver disease and mitochondrial dysfunction. World J. Gastroenterol. 14, 193–199.
Keywords: copper, HIF-1α, alcoholic liver injury, intestinal barrier, Caco-2
Citation: Lin H, Chen D, Du Q, Pan T, Tu H, Xu Y, Teng T, Tu J, Li J, Lin Z, Wang X, Xu L and Chen Y-P (2020) Dietary Copper Plays an Important Role in Maintaining Intestinal Barrier Integrity During Alcohol-Induced Liver Disease Through Regulation of the Intestinal HIF-1α Signaling Pathway and Oxidative Stress. Front. Physiol. 11:369. doi: 10.3389/fphys.2020.00369
Received: 02 December 2019; Accepted: 30 March 2020;
Published: 08 May 2020.
Edited by:
Kusum K. Kharbanda, University of Nebraska Medical Center, United StatesReviewed by:
Pradeep Kumar Shukla, The University of Tennessee Health Science Center (UTHSC), United StatesValentina Medici, University of California, Davis, United States
Copyright © 2020 Lin, Chen, Du, Pan, Tu, Xu, Teng, Tu, Li, Lin, Wang, Xu and Chen. This is an open-access article distributed under the terms of the Creative Commons Attribution License (CC BY). The use, distribution or reproduction in other forums is permitted, provided the original author(s) and the copyright owner(s) are credited and that the original publication in this journal is cited, in accordance with accepted academic practice. No use, distribution or reproduction is permitted which does not comply with these terms.
*Correspondence: Lanman Xu, MTM1ODc2NDYzMTVAMTYzLmNvbQ==; Yong-Ping Chen, MTM1MDU3NzcyODFAMTYzLmNvbQ==
†These authors have contributed equally to this work