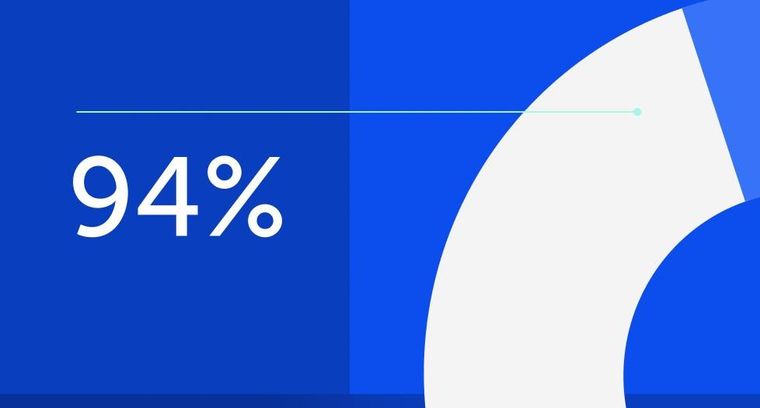
94% of researchers rate our articles as excellent or good
Learn more about the work of our research integrity team to safeguard the quality of each article we publish.
Find out more
REVIEW article
Front. Physiol., 10 March 2020
Sec. Exercise Physiology
Volume 11 - 2020 | https://doi.org/10.3389/fphys.2020.00199
This article is part of the Research TopicExploration of the Physiological Effects of Exercise in Cardiovascular DiseasesView all 10 articles
Exercise training has been reported to ameliorate heart dysfunction in both humans and animals after myocardial infarction (MI). Exercise-induced cardioprotective factors have been implicated in mediating cardiac repair under pathological conditions. These protective factors secreted by or enriched in the heart could exert cardioprotective functions in an autocrine or paracrine manner. Extracellular vesicles, especially exosomes, contain key molecules and play an essential role in cell-to-cell communication via delivery of various factors, which may be a novel target to study the mechanism of exercise-induced benefits, besides traditional signaling pathways. This review is designed to demonstrate the function and underlying protective mechanism of exercise-induced cardioprotective factors in MI, with an aim to offer more potential therapeutic targets for MI.
Myocardial infarction (MI) is a serious result of cardiovascular disease and the leading cause of mortality and morbidity (Andersson et al., 2018). Approaches to inhibit the pathological process of MI are essential for its treatment and prognosis. To date, well-documented evidences have proved that appropriate exercise training can alleviate MI, which reduces mortality and improves the net clinical benefit in patients with MI (Anderson et al., 2016; Lear et al., 2017; Moholdt et al., 2018). Therefore, exercise training has been widely recommended as a therapeutic strategy for MI.
Various factors, including multiple polypeptides, nucleic acids, and similar substances are induced during exercise, which, in part, exert protective biological effects against several diseases (Whitham et al., 2018). Parts of cardioprotective factors are largely secreted by or enriched in the heart, which facilitate direct communication between the myocardium and other organs, and induce repair of cardiac injury under pathologic conditions (Shimano et al., 2012). Recently, it has been reported that the level of some cardioprotective factors is significantly increased during exercise training (Sanchis-Gomar et al., 2016; Whitham et al., 2018). Discovery and characterization of exercise-induced cardioprotective factors are of great interest because they may lead to a better understanding of the alterations in cell-to-cell communication in MI and help identify new therapeutic targets.
However, the secretory mechanisms of exercise-induced cardioprotective factors and their network regulation in MI are complex. Extracellular vesicles (EVs) released by cells are composed of a lipid bilayer enclosing soluble cytosolic material and nuclear components, which could act in an autocrine or paracrine manner to play a role in intercellular communication (Kowal et al., 2014). EVs divide into apoptotic bodies, microvesicles, and exosomes (EXs) depending on their size (Kowal et al., 2014). EVs, especially the most widely studied EXs, can be induced by exercise to facilitate the exchange of peptides, non-coding RNAs (ncRNAs), mRNA, DNA, and metabolites between cells and tissues, and are considered to play a key role in intercellular communication under physiological and pathological conditions, such as MI (Kowal et al., 2014; Davidson et al., 2017).
Intriguingly, circulatory EVs/EXs content varies in an intensity-dependent manner in response to exercise training (Bei et al., 2017). The heart is the main endocrine organ affected during exercise, and some cardiac-derived protective factors can be induced by exercise and carried by EVs/EXs to ameliorate the pathological processes of MI (Ogawa and de Bold, 2014; Sanchis-Gomar et al., 2016). Thus, this review summarizes the protective role and underlying mechanism of cardiac-derived protective factors that can be induced by exercise in MI, with an aim to demonstrate their potential as therapeutic targets (Figure 1).
Figure 1. Exercise-induced cardioprotective factors on cardiac repair after MI. The heart is an endocrine organ that produces and releases cardioprotective factors during exercise training. Cardioprotective factors mainly derived from cardiomyocytes, endothelial cells, and cardiac fibroblasts, which not only secreted by traditional signaling pathways but also delivered via EVs/EXs that changed components during exercise. Subsequently, these factors exert important regulatory functions for cardiac repair after MI in autocrine or paracrine manners, which may partially explain exercise-induced beneficial mechanism of MI. BDNF, brain-derived neurotrophic factor; CTRP9, C1q/TNF-related protein 9; EVs, extracellular vesicles; EXs, exosomes; FSTL1, follistatin-like 1; FGF21, fibroblast growth factor 21; GDF15, growth differentiation factor 15; ILs, interleukins; lncRNA, long non-coding RNA; MI, myocardial infarction; MIF, migration inhibitory factor; miR, microRNA; MALAT1, metastasis associated lung adenocarcinoma transcript 1; NRG, neuregulin; ncRNAs, non-coding RNAs. ↑ means upregulate, ↓ means downregulate, N means not clarified or controversial.
Certain cardiac-derived peptides exert multiple biological functions under pathophysiological states. It has been reported that exercise can induce the secretion of various cardioprotective factors, which exert protective effects in MI (Safdar et al., 2016) (Table 1).
Growth differentiation factor 15 (GDF15), also known as macrophage inhibitory cytokine 1, is a polypeptide molecule that could be synthesized and secreted by cardiomyocytes (Shimano et al., 2012; Emmerson et al., 2017). GDF15 expression is induced under various pathophysiological states and significantly increased during MI onset (Khan et al., 2009). It has also been found that higher levels of GDF-15 in patients with acute coronary syndrome are associated with raised risks of spontaneous MI, as well as cardiovascular and total mortality (Hagström et al., 2016). Thus, GDF15 could serve as both a biomarker and predictor for the prognosis of MI.
Moreover, GDF15 expression can be induced by exercise. A recent study reported that plasma GDF15 levels in healthy male individuals significantly increased from 215 pg/mL at rest to 295 pg/mL at the end of the exercise bout, and further increased to about 350 pg/mL at the end of recovery (Kleinert et al., 2018). More interestingly, GDF15 from femoral artery and femoral vein has been, respectively, tested before, during, and after exercise and found no difference. This finding suggests that exercise-induced GDF15 may not be secreted by the skeletal muscle (Kleinert et al., 2018), but possibly be derived from other organs, especially the cardiac muscle.
Growth differentiation factor 15 has been found to play important roles in regulating the pathophysiological process of MI via its vital anti-inflammatory function (Kempf et al., 2011). Excessive inflammatory cell recruitment to the infarcted area after MI aggravates myocardial remodeling and leads to fatal complications. Kempf et al. (2011) reported that GDF15-deficient mice showed a significant increase in the recruitment of polymorphonuclear leukocytes (PMNs) to the infarcted myocardium and an increase in the incidence of cardiac rupture. Conversely, infusion of recombinant GDF15 in MI mice repressed PMN recruitment by directly inhibiting chemokine signaling and integrin activation, indicating that GDF15 protects against fatal cardiac rupture after MI though controlling inflammatory cell recruitment. Rainer et al. (2014) further showed that suppression of cardiomyocyte-specific transforming growth factor (TGF)-β promoted GDF15 expression, which inhibited neutrophil integrin activation and heart remodeling after infarction (Rainer et al., 2014). Although GDF15 is increasing after MI and its higher level can predict a worse prognosis, it may be a promising exercise-induced therapeutic target for MI.
FSTL1, a member of the follistatin family, is abundantly expressed in the skeletal and cardiac muscle, and has been shown to mediate multiple aspects of MI (Wei et al., 2015; Shen et al., 2019). Maruyama et al. (2016) found that FSTL1 expression significantly increased after MI, and inhibiting its expression increased mortality due to cardiac rupture in the acute phase of MI. Moreover, FSTL1 deficiency-induced cardiac rupture was mainly associated with attenuation of the migratory and proliferative capabilities of cardiac fibroblasts and reduction of extracellular matrix proteins mediated via the extracellular signal-regulated kinase (ERK)1/2 pathway (Maruyama et al., 2016). Shen et al. (2019) showed that FSTL1 expression declined dramatically in hypoxia-induced mesenchymal stem cells (MSCs), while overexpression of FSTL1 significantly prolonged MSC retention after implantation in the ischemic myocardium, thereby preserving heart function after MI by limiting scar formation, reducing inflammatory response, and enhancing neovascularization. These findings suggest that FSTL1 might serve as a key therapeutic target for MI.
Exercise training has been shown to induce FSTL1 expression (Xi et al., 2016; Kon et al., 2019a). In healthy individuals, 60 min of cycling dramatically increased the serum FSTL1 level by 22%, with a concentration increase from 16.9 ng/mL (pre-exercise) to 20.1 ng/mL (immediately after exercise), which further increased to 21.9 ng/mL at 30 min after completing the exercise [23]. More interestingly, exercise training further increases ischemia-induced FSTL1 expression. A previous study showed that FSTL1 expression in MI mice was 1.96-fold higher than that in control mice, and it further increased by 4.04-fold after intermittent aerobic exercise (Xi et al., 2016). In addition, exercise-induced FSTL1 has been shown to improve cardiac remodeling and prognosis after MI via promoting angiogenesis and reducing cardiac fibrosis (Xi et al., 2016).
Interestingly, it was previously detected by a luciferase-based reporter gene assay that FSTL1 as an upstream regulator of GDF-15. It has been found that treatment with FSTL1 activated GDF15 production in cultured cardiomyocytes. More importantly, transgenic production of FSTL1 stimulated GDF15 production in the murine heart, whereas cardiomyocyte selective deletion of FSTL1 decreased production of GDF15 in cardiomyocytes, suggests that these proteins function as components of an interactive network (Widera et al., 2012). Moreover, the circulating concentration of FSTL1 has been shown to be dependently related to GDF15 and act as a biomarker to predict cardiovascular mortality in patients with acute coronary syndrome (Widera et al., 2012). Overall, the co-expression of FSTL1 and GDF15 provides an explanation for the mechanism of exercise-induced cardioprotection in MI, at least in part.
Irisin, a new type of muscle factor highly expressed in the myocardium, has been considered as a novel exercise-induced cardioprotective factors (Tsuchiya et al., 2015; Wang H. et al., 2017). Irisin levels have been shown to be significantly induced by various exercise types. Plasma irisin levels were increased by 65% in mice after 3 weeks of free wheel running exercise, while circulating irisin levels were increased by twofold in healthy adult humans after 10 weeks of supervised endurance exercise training compared to the non-exercising group (Bostrom et al., 2012). More interestingly, the effects of different exercise duration and models on irisin levels are distinct. Irisin levels were shown to increase significantly after acute strenuous exercise and a 30 min bout of intensive exercise in children and young adults, but remained unchanged after 6 weeks of chronic exercise training (Loffler et al., 2015). Endurance exercise significantly increased irisin levels, which began to decrease after 2 h of exercise (Nygaard et al., 2015).
Recently, irisin has been found to protect against the pathologic process of MI via its anti-apoptotic, pro-angiogenetic, and cardiac regenerative functions (Wang H. et al., 2017; Liao et al., 2019; Zhao et al., 2019). Wang H. et al., 2017 showed that irisin treatment induced remarkable improvements in ventricular functional recovery and reduction of infarct size in the Langendorff perfused ischemia/reperfusion (I/R) injury heart of mice via suppressing the opening of mitochondrial permeability transition pore, which results in mitochondrial swelling, and protecting mitochondrial function to reduce cardiomyocyte apoptosis. Liao et al. (2019) reported that treatment with irisin for 2 weeks significantly reduced infarct size and fibrosis in MI mice, significantly increased angiogenesis in the ischemic area, and decreased cardiomyocyte apoptosis via activating the ERK signaling pathway. Besides, it has been recently reported that irisin can induce cardiac regeneration and functional improvement of MI mice via promoting the function of cardiac progenitor cells (CPCs) (Zhao et al., 2019). Thus, irisin is a key exercise-induced cardioprotective factor exerting multiple functions in MI.
FGF21 has been found to ameliorate the pathological progression of MI (Joki et al., 2015; Hu S. et al., 2018; Tang et al., 2018). It has been shown that injection of MI mice with recombinant interleukin (IL)-22 in the 1st week after acute MI effectively prevents left ventricular (LV) dysfunction and attenuates ventricular remodeling via markedly increasing FGF21 expression in a signal transducer and activator of transcription (STAT)3-dependent manner, indicating that FGF21 might be a promising therapeutic target for MI treatment (Tang et al., 2018). Another recent study showed that increased FGF21 expression can ameliorate cardiac remodeling in MI mice via increasing capillary density around the infarct area and reducing cardiomyocyte apoptosis together with decreasing pro-inflammatory cytokine level in an adiponectin-dependent manner (Joki et al., 2015). Besides, FGF21 has been reported to protect against I/R-injury via decreasing miR-145-mediated autophagy (Hu S. et al., 2018).
Circulating FGF21 levels have been shown to significantly increase with exercise training (Geng et al., 2019). In healthy subjects, the level of FGF21 increases from 276.8 to 460.8 ng/L after 2 weeks of physical activity (Cuevas-Ramos et al., 2012). Another random cross-sectional study showed that 5 weeks of endurance exercise significantly increased circulating FGF21 levels in the elderly, and decreased their liver fat content (Taniguchi et al., 2016). It has also been speculated that exercise may induce cardiac-specific FGF21 expression via the sirtuin1 (Sirt1)/peroxisome proliferator-activated receptor gamma coactivator-1α (PGC-1α) signaling pathway (Guo et al., 2016). Thus, FGF21 is considered as an important exercise-induced cardioprotective factor for MI treatment.
It has been widely considered that irisin is widely expressed in cardiac and skeletal muscle, while FGF21 is also abundantly exist in the liver, adipose tissue, skeletal and cardiac muscle to mediate lipid metabolism. A previous study identified that exercise-induced irisin secretion could interact with FGF21 to exert biological functions, suggesting that they may regulate muscle–adipose crosstalk during exercise (Lee et al., 2014). Thus, their regulatory network may be a way to explain muscle–adipose or organ-organ crosstalk during exercise training, and thereby indicating further mechanisms of exercise-induced benefits in MI.
Several members of the IL family have been identified as cardioprotective factors, some of which are induced by exercise. IL-33, a cytokine belonging to the IL-1 family, is abundantly expressed in the heart, and attenuates inflammatory response and acts as the specific ligand for soluble ST2 (Shimano et al., 2012; Chen et al., 2018). IL-33 is mainly secreted by cardiac fibroblasts, exerting its function in a paracrine manner to exchange information with cardiomyocytes, and thereby playing an important role in the pathophysiological process of MI (Chen et al., 2018).
It has been reported that IL-33/ST2 pathway activation results in inhibition of apoptosis and inflammation, reduction of cardiac fibrosis, and improvement of cardiac function (Chen et al., 2018). Yin et al. (2014) found that IL-33 effectively suppressed macrophage infiltration and production of inflammatory cytokines in the myocardium after MI, and injection of recombinant IL-33 in MI mice reduced infarct size, attenuated cardiac remodeling, and improved cardiac function by inhibiting nuclear factor-κB (NF-κB) and p38 mitogen-activated protein kinase (MAPK) signaling pathways. Besides, Li et al. (2019) showed that IL-33 reduced infarct area and prevented the progression of fibrosis by inducing M2 macrophage polarization in MI mice model via activating the Janus kinase (JAK)/STAT pathway. Thus, IL-33 exerts anti-inflammatory effects in MI.
Various factors, including exercise training, have be shown to stimulate IL-33 expression. Further, it has been demonstrated that long-term medium-intensity exercise not only decreases the expression of pro-inflammatory factors, like Toll-like receptor 4 (TLR4), NF-κB, and IL-18, but also significantly increases the level of the anti-inflammatory factor IL-33 in patients with diabetes mellitus (Liu et al., 2015). Hence, IL-33 is considered as an exercise-induced cardioprotective factor that exerts important protective functions in MI.
Besides, IL-6 and IL-1β have also been identified as cardioprotective factors, as they aggravate MI. Distinctly, exercise training has been shown to reduce their expression, which may be a potential approach to ameliorate MI (Pedersen, 2017). Although not all IL family members are widely expressed in the heart and induced by exercise, they exert vital functions to regulate the course of MI. Several members of this family have been identified as exercise-induced cardioprotective factors, and may serve as promising therapeutic targets for MI.
Neuregulin (NRG) is expressed in human cardiac endothelial cells and acts as an endothelial cell-derived molecule that exerts cardioprotective effects (Hedhli et al., 2011). NRG has been found to protect against cardiomyocyte apoptosis induced by hypoxia-reoxygenation, and in an in vivo study it was shown to reduce infarct size and cardiomyocyte apoptosis after myocardial I/R-injury (Hedhli et al., 2011). Furthermore, the cardioprotective effect of NRG was shown to be mediated via attenuation of endoplasmic reticulum stress and cardiomyocyte apoptosis by activating the phosphatidylinositol-3-kinase/protein kinase B (PI3K/AKT) signaling pathway under I/R states (Fang et al., 2017). More interestingly, exercise can upregulate NGR and its ligand expression to promote cardiac repair, indicating that NRG is an exercise-induced cardioprotective factor and serves as a promising therapeutic target for MI (Cai et al., 2016).
Migration inhibitory factor (MIF) is a macrophage factor that regulates inflammation and immunity, and is secreted by cardiomyocytes and cardiac fibroblasts to promote cardiomyocyte survival and regulate inflammation after MI (Voss et al., 2019). Besides, it has been reported that MIF promotes cardiac stem cell survival, proliferation, and endothelial differentiation by targeting its receptor CD74 via activation of the PI3K/AKT/mammalian target of rapamycin (mTOR) and adenosine monophosphate-activated protein kinase (AMPK) signaling pathways. This finding suggests a potential therapeutic role of MIF in the treatment of MI (Cui et al., 2016). Additionally, MIF expression can also be induced by exercise, and it has been identified as an exercise-induced cardioprotective factor that protects against MI (Chang et al., 2019).
Brain-derived neurotrophic factor (BDNF), widely expressed in various non-neural tissues, such as vascular endothelial cells and myocardial cells, plays a protective role in MI via targeting its functional receptor, tyrosine receptor kinase B (Zhang et al., 2019). Recent studies have found that BDNF can promote angiogenesis, inhibit inflammatory response, and attenuate cardiac remodeling, thereby improving cardiac function and prognosis after MI (Wang et al., 2018). Well-documented evidences have shown that BDNF expression is significantly induced by exercise training, and thereby it can be considered as a beneficial exercise-induced cardioprotective factor (Wang et al., 2018; Zhang et al., 2019).
C1q/TNF-related protein-9 (CTRP9) is a novel cardioprotective factor primarily secreted by the adipose tissue and cardiac endothelial cells (Zhao et al., 2018). CTRP9 has been shown to enhance adipose-derived mesenchymal stem cell (ADSC) proliferation and survival after implantation to MI mice. Further, CTRP9 stimulates ADSC migration and attenuates cardiomyocyte cell death after MI via binding with N-cadherin, activation of ERK/MMP-9 and ERK/Nrf2 signaling, and upregulation/secretion of anti-oxidative proteins (Yan et al., 2017). Besides, CTRP9 expression is also induced by exercise, and a single bout of high intensity interval training is sufficient to stimulate CTRP9 secretion in healthy men (Kon et al., 2019b). Thus, CTRP9 has been identified as a novel exercise-induced cardioprotective factor exerting potential therapeutic effects in MI.
Non-coding RNAs, which do not code for proteins but functionally regulate protein expression, have been identified as critical regulators of cell function and important candidates that protect against MI (Guo et al., 2017). MicroRNAs (miRNAs, miRs) are a class of short-chain ncRNAs that have been reported to regulate MI via negatively modulating their target genes (Chistiakov et al., 2016). Cardiac-derived miRNAs induced by exercise may explain the exercise-induced beneficial effects on MI (Gomes et al., 2014) (Table 2).
miR-1 and miR-133 are highly expressed in cardiomyocytes and play an important role in regulating myocardial autonomy, conduction, contraction, and myocyte differentiation and proliferation (Chistiakov et al., 2016). Previous studies have reported that miR-1 and miR-133 expression is regulated by exercise. It was shown that miR-1 and miR-133 expression significantly increased in marathon athletes after exercise (Gomes et al., 2014). However, another study demonstrated that miR-1 and miR-133 expression was significantly downregulated after exercise (Pietrangelo et al., 2015). These paradoxical results may be attributed to individual variations and differences in exercise duration. More interestingly, both miR-1 and miR-133 have been shown to exert important regulatory functions in MI (Duan et al., 2018; Yu et al., 2019).
While the regulatory role of miR-1 in MI remains controversial, studies suggest that transplantation of stem cells with miR-1 overexpression exerts important protective effects after MI. It has been reported that transplantation of embryonic stem cells with miR-1 overexpression can significantly reduce apoptosis and improve cardiac function via phosphatase and tensin homolog deleted on chromosome 10 (PTEN)/AKT pathway activation (Glass and Singla, 2011). Additionally, transplantation of MSCs overexpressing miR-1 into the infarcted myocardium of mice was shown to improve cell survival rate, promote cardiomyogenic differentiation, and improve cardiac function, indicating that miR-1 has a protective effect on MI (Huang et al., 2013). However, the direct function of miR-1 in MI requires further investigation.
miR-133 plays a cardioprotective function in MI. Li et al. (2015) found that overexpression of exogenous miR-133 significantly attenuated cardiomyocyte apoptosis, both in vitro and in vivo, in a hypoxia-reoxygenation injury model via inhibition of death associated protein kinase 2 (DAPK2). Izarra et al. (2014) further reported that miR-133 can attenuate myocardiocyte apoptosis through inhibiting the expression of pro-apoptotic genes, including caspase-9, apoptotic protease activating factor, DAPK2, Bcl2-like 11, and Bcl-2-modifying factor. In an MI rat model, it was reported that overexpression of miR-133 significantly promotes angiogenesis and cardiomyocyte proliferation, and inhibits cardiac hypertrophy and fibrosis, thereby improving cardiac function (Izarra et al., 2014). Another study showed that transplantation of miR-133-overexpressing MSCs in ischemic area in MI rat model markedly improved cardiac function (Chen et al., 2017). Therefore, overexpression of miR-133 may be an important therapeutic strategy for MI treatment.
The cardiac-enriched miRNAs, miR-208 and miR-499, are mainly involved in modulation of differentiation and development of cardiac fibroblasts and cardiomyocytes, and play a vital role in maintaining normal cardiac function (Chistiakov et al., 2016). It has been shown that the expression of miR-208 is significantly downregulated, while that of miR-499 is markedly upregulated after exercise training, which may partly explain the benefits of exercise (Baggish et al., 2014; Soci et al., 2016).
miR-208 promotes cardiomyocyte apoptosis and cardiac remodeling after MI. Yan et al. (2016) found that inhibition of miR-208 expression in neonatal rat cardiomyocytes under hypoxia condition could relieve cardiomyocyte injury. Bian et al. (2015) showed that overexpression of miR-208 markedly aggravated I/R-induced myocardial injury in rats and promoted hypoxia-induced cardiomyocyte apoptosis in vitro, while knockdown of miR-208 suppressed cardiomyocyte apoptosis. Further, they found that miR-208 induced apoptosis via its target gene Ets1. The expression of miR-208 is directly correlated with β-MHC levels and cardiac collagen capacity. Therefore, inhibiting miR-208 expression may be a potential therapeutic approach for MI.
miR-499 has important regulatory effects on the pathological process of MI. Wang et al. (2014) found that miR-499 protected cardiomyocytes against H2O2-induced apoptosis and overexpression of miR-499 in rat cardiomyocytes increased cell survival rate by inhibiting the mitochondrial apoptosis pathway and pro-apoptotic gene expression. The pro-apoptotic gene Dyrk2 promoted apoptosis by increasing the level of p53 phosphorylation, whereas miR-499 significantly inhibited Dyrk2 expression, preventing the transfer of activated p53 to the mitochondria and inhibiting apoptosis. miR-499 has also been shown to suppress calcineurin-mediated dephosphorylation of dynamin-related protein-1 (Drp1) to inhibit cardiomyocyte apoptosis, thereby reducing Drp1 accumulation in the mitochondria and mitochondrial fission (Wang et al., 2011). Furthermore, Shi et al. (2019) found that miR-499 reduced H9C2 cell injury by inhibiting the expression of Sox6, and suppressed apoptosis by increasing Bcl-2 and decreasing Bax and caspase-3 expression under hypoxia-reoxygenation condition. Therefore, miR-499 can target multiple genes to protect against apoptosis after MI.
miR-126, mainly expressed in endothelial cells, significantly promotes angiogenesis around the infarct area after MI (Jiang et al., 2014). The expression of cardiac-enriched miR-126 is significantly changed after MI, exerting pro-angiogenetic effects to maintain vascular wall integrity by negatively regulating its target gene Spred1 (Guo et al., 2018). Exercise can increase miR-126 expression. It has been reported that miR-126 expression was upregulated by 2.1- and 4.6-fold in healthy subjects after a maximal symptom-limited exercise test and 4 h of cycling, respectively (Uhlemann et al., 2014). Thus, miR-126 is an important exercise-induced factor that protects against MI mainly by promoting angiogenesis.
miR-21, a miRNA transcribed by RNA polymerase II, is widely expressed in endothelial cells and exerts protective functions after MI. A previous study demonstrated that miR-21 exerts anti-fibrotic effects via promoting cardiac fibroblast-to-myofibroblast transformation by regulating its target gene Jagged1 (Zhou X.L. et al., 2018). Another study showed that miR-21 attenuated inflammation, cardiac dysfunction, and maladaptive remodeling post-MI through targeting kelch repeat and BTB (POZ) domain containing 7 and inhibiting p38 and NF-κB pathway activation (Yang et al., 2018). Intriguingly, a recent study showed that the serum level of miR-21 was upregulated in elderly patients with acute MI, and that miR-21 suppressed TNF-α-induced apoptosis in human cardiomyocytes via stimulating the activation of JNK/p38/caspase-3 signaling pathway (Wang Z.H. et al., 2017). Additionally, miR-21 expression has been shown to be regulated by exercise, indicating a novel mechanism underlying exercise-induced benefits in MI (Wahl et al., 2016).
lncRNAs are transcripts longer than 200 nucleotides that regulate various biological processes by interacting with multiple transcription factors (Wang et al., 2015). Recently, it has been identified that lncRNAs play a crucial regulatory function in MI. The lncRNAs H19 and metastasis associated lung adenocarcinoma transcript 1 (MALAT1) have been widely studied, and their levels are shown to be regulated by exercise. Exercise-induced lncRNA H19 expression has been shown to involve in epigenetic modifications (Xu et al., 2017). lncRNA MALAT1 expression can be induced by swimming via inhibition of apoptotic functions, thereby protecting hippocampal neurons against ischemic diseases (Shang et al., 2018).
The lncRNA H19 has been reported to play multiple roles after MI. Wang et al. (2015) reported that lncRNA H19 directly binds to miR-103/107 and reduces its expression, which promotes Fas-associated protein with death domain expression, and participates in H2O2-induced necrosis in H9C2 cells. This finding revealed that lncRNA H19 prevents cardiomyocyte necrosis in MI. Furthermore, lncRNA H19 was shown to alleviate myocardial cell injury through reduction of miR-139 expression and further upregulation of its target gene Sox8 via PI3K/AKT/mTOR and MAPK pathway activation (Gong et al., 2017). Additionally, lncRNA H19 has been shown to enhance angiogenesis, prevent cardiomyocyte apoptosis, and improve cardiac function after MI via targeting miR-675-5p (Huang P. et al., 2019). Besides, overexpression of lncRNA H19 in mice was shown to reduce infarct size and improve cardiac function via activating autophagy (Zhou M. et al., 2018). These findings demonstrate that lncRNA H19 exerts essential protective functions in MI.
lncRNA MALAT1 is highly expressed in vascular endothelial cells and has a complex function in MI (Li L. et al., 2018). It was initially reported that genetic deletion or pharmacological inhibition of MALAT1 reduced vascular growth in vivo, suggesting that MALAT1 promoted angiogenesis in ischemic diseases (Michalik et al., 2014). Besides, it has been shown that MALAT1 protects against cardiomyocyte apoptosis after MI by sponging miR-558, thereby inducing Unc-51-like autophagy-activating kinase 1-dependent protective autophagy (Guo et al., 2019). However, a recent study reported that MALAT1 promoted cardiac fibrosis and deteriorated cardiac function post-MI by increasing TGF-β1 activity and inhibiting miR-145 expression (Huang S. et al., 2019). Additionally, MALAT1 has been shown to increase cell apoptosis in MI via acting as a competing endogenous RNA to sponge miR-200a-3p (Sun and Zhang, 2019). Thus, lncRNA MALAT1 exerts critical regulatory functions in various biological processes during MI, including angiogenesis, cell proliferation, apoptosis, and cardiac fibrosis. Nevertheless, whether it plays a protective or detrimental role in MI requires further investigation.
Recent researchers have identified EVs as an important cell-to-cell communication way (Doroudgar and Glembotski, 2011). EVs, secreted by cells in the form of vesicles containing various signaling molecules, play a role in cell-to-cell communication under pathophysiological conditions (Safdar et al., 2016). As previously described, EXs are one of the most widely studied EVs. Generally, EXs act in an endocrine, autocrine, or paracrine manner to exert their biological functions (Safdar et al., 2016).
The biogenesis and release of EXs is related to complex regulatory factors. As previously described, EXs biogenesis begins within the endosome system and is matured in an endosomal sorting complex required for transport (ESCRT)-dependent or independent manner (Colombo et al., 2014). Molecular regulators implicated in exosome release include multiple factors, especially Soluble NSF Attachment Protein Receptor (SNARE) and Rabs GTPases (Colombo et al., 2014). Thus, EXs carries not only secreted factors but also a cluster of scaffold proteins, which may be used to derive their source cells. It has been found that EXs originated from cardiomyocytes mainly express caveolin-3 and Troponin T, from cardiac fibroblast express CD90.2, and from endothelial cells express CD31 (Loyer et al., 2018).
At present, many kinds of cells have been shown to secrete EXs, including various stem cells, endothelial cells, cardiofibroblasts, and cardiomyocytes (Barile et al., 2017). EXs derived from different cells exhibit different functions. A recent study reported that cardiomyocytes release EVs under ischemic stress; and cardiomyocytes as the main source of bioactive EXs in coronary serum (Li H. et al., 2018). Interestingly, certain cells exert various biological functions by releasing EXs with different components, which varies under different culture conditions and stimuli (Gallet et al., 2017; Ribeiro-Rodrigues et al., 2017; Huang P. et al., 2019).
Previous studies have shown that EVs/EXs stimulated by ischemia exert important cardioprotective functions after MI via changing their contents (Gallet et al., 2017; Ribeiro-Rodrigues et al., 2017; Gao et al., 2018). Ribeiro-Rodrigues et al. (2017) found that EXs secreted by cardiomyocytes under ischemic conditions contained high levels of matrix metalloproteinases (MMP), miR-222, and miR-143, and stimulated the formation of new functional vessels following MI. Barile et al. (2014) reported that infarcted hearts injected with EVs from CPCs showed reduced cardiomyocyte apoptosis, enhanced angiogenesis, and improved LV ejection fraction compared with those injected with control medium, and this effect was induced by the changed levels of miR-210, miR-132, and miR-146a-3p in EVs. Li H. et al., 2018 also found that, compared to EXs from healthy controls, EXs from patients with myocardial ischemia enhanced endothelial cell proliferation, migration, and tube formation via downregulation of miR-939-5p, and thereby increased endothelial nitric oxide production, eventually promoting angiogenesis.
More interestingly, exercise can induce various organs to release EVs/EXs that are enriched in various peptides, ncRNAs, and other substances. The release of EXs was shown to significantly increase immediately after cycling exercise and then decline again within 90 min at rest, while release of EXs was moderate but appeared more sustained after treadmill running. Moreover, release of EXs into the circulation has been shown to be initiated in the aerobic phase, suggesting that it is independent of the metabolic changes during exercise (Fruhbeis et al., 2015). Further, in another study, after a 1 h bout of cycling exercise in healthy humans, an increase in the circulation of over 300 proteins was observed, and most of them were secreted by EXs, suggesting that exercise exerts systemic biological effects (Whitham et al., 2018). Thus, EXs may provide a new approach to explain the multiple protective mechanisms of exercise in MI.
Additionally, it has been found that exercise can induce cardiomyocytes to release EVs/EXs, which exert a cardioprotective function in MI. A recent study showed that the serum level of EVs was increased by about 1.85-fold in mice after 3 weeks of swimming (Bei et al., 2017). Furthermore, intramyocardial injection of EVs induced by exercise exerted additional anti-apoptotic effects on H2O2-treated H9C2 cardiomyocytes compared to exercise alone. The protective effects on acute ischemia in mice were mediated by the activation of the ERK1/2 and HSP27 pathways (Bei et al., 2017). Thus, exercise-induced EVs/EXs released by cardiomyocytes exert important protective functions in MI.
Cardioprotective factors carried by EXs have been found to regulate MI pathology (Hu M. et al., 2018). In an MI mice model, it was reported that the level of cardiac-enriched miRNAs, such as miR-1 and miR-499 was predominantly increased in circulating EXs, subsequently mediating functional crosstalk between the ischemic heart and bone marrow for repair of cardiac injury (Cheng et al., 2019). Recently, the exosomal lncRNA H19 derived from MSCs has been reported to mediate the cardioprotective effects in infarcted hearts via promoting endothelial cell function and angiogenesis (Huang P. et al., 2019). Besides, polypeptide molecules, like MIF and BNDF can also be delivered by EXs to exert biological functions (Suire et al., 2017; Amosse et al., 2018). Thus, EXs may serve as important vehicles to deliver exercise-induced cardioprotective factors and as mediators of intercellular communication in MI, which may be a possible mechanism underlying exercise-induced benefits.
In summary, exercise has beneficial effects on MI, but its mechanisms are complex and need to be fully elucidated. It has been found that exercise can induce the secretion of a variety of polypeptide molecules, ncRNAs, and other substances derived from the myocardium and other organs. Several recent studies have reported that EVs/EXs are enriched in a large number of cardioprotective factors, and their secretion is regulated by exercise. This review summarizes the effects of newly discovered exercise-induced cardiogenic peptides and ncRNAs on MI and their potential mechanisms, thus aiming to provide a new theoretical basis for the application of exercise training in the clinical treatment of MI.
YG drafted the manuscript. JC and HQ checked and revised the manuscript. All authors read and approved the final manuscript.
The authors declare that the research was conducted in the absence of any commercial or financial relationships that could be construed as a potential conflict of interest.
Amosse, J., Durcin, M., Malloci, M., Vergori, L., Fleury, A., Gagnadoux, F., et al. (2018). Phenotyping of circulating extracellular vesicles (EVs) in obesity identifies large EVs as functional conveyors of Macrophage Migration Inhibitory Factor. Mo.l Metab. 18, 134–142. doi: 10.1016/j.molmet.2018.10.001
Anderson, L., Oldridge, N., Thompson, D. R., Zwisler, A. D., Rees, K., Martin, N., et al. (2016). Exercise-based cardiac rehabilitation for coronary heart disease: cochrane systematic review and meta-analysis. J. Am. Coll. Cardiol. 67, 1–12. doi: 10.1016/j.jacc.2015.10.044
Andersson, H. B., Pedersen, F., Engstrøm, T., Helqvist, S., Jensen, M. K., Jørgensen, E., et al. (2018). Long-term survival and causes of death in patients with ST-elevation acute coronary syndrome without obstructive coronary artery disease. Eur. Heart J. 39, 102–110. doi: 10.1093/eurheartj/ehx491
Baggish, A. L., Park, J., Min, P. K., Isaacs, S., Parker, B. A., Thompson, P. D., et al. (2014). Rapid upregulation and clearance of distinct circulating microRNAs after prolonged aerobic exercise. J. Appl. Physiol. 116, 522–531. doi: 10.1152/japplphysiol.01141.2013
Barile, L., Lionetti, V., Cervio, E., Matteucci, M., Gherghiceanu, M., Popescu, L. M., et al. (2014). Extracellular vesicles from human cardiac progenitor cells inhibit cardiomyocyte apoptosis and improve cardiac function after myocardial infarction. Cardiovasc. Res. 103, 530–541. doi: 10.1093/cvr/cvu167
Barile, L., Moccetti, T., Marban, E., and Vassalli, G. (2017). Roles of exosomes in cardioprotection. Eur. Heart J. 38, 1372–1379. doi: 10.1093/eurheartj/ehw304
Bei, Y., Xu, T., Lv, D., Yu, P., Xu, J., Che, L., et al. (2017). Exercise-induced circulating extracellular vesicles protect against cardiac ischemia-reperfusion injury. Basic Res. Cardiol. 112:38. doi: 10.1007/s00395-017-0628-z
Bian, C., Xu, T., Zhu, H., Pan, D., Liu, Y., Luo, Y., et al. (2015). Luteolin inhibits ischemia/reperfusion-induced myocardial injury in rats via downregulation of microRNA-208b-3p. PLoS One 10:e0144877. doi: 10.1371/journal.pone.0144877
Bostrom, P., Wu, J., Jedrychowski, M. P., Korde, A., Ye, L., Lo, J. C., et al. (2012). A PGC1-alpha-dependent myokine that drives brown-fat-like development of white fat and thermogenesis. Nature 481, 463–468. doi: 10.1038/nature10777
Cai, M. X., Shi, X. C., Chen, T., Tan, Z. N., Lin, Q. Q., Du, S. J., et al. (2016). Exercise training activates neuregulin 1/ErbB signaling and promotes cardiac repair in a rat myocardial infarction model. Life Sci. 149, 1–9. doi: 10.1016/j.lfs.2016.02.055
Chang, M. C., Park, C. R., Rhie, S. H., Shim, W. H., and Kim, D. Y. (2019). Early treadmill exercise increases macrophage migration inhibitory factor expression after cerebral ischemia/reperfusion. Neural Regen. Res. 14, 1230–1236. doi: 10.4103/1673-5374.251330
Chen, B., Geng, J., Gao, S. X., Yue, W. W., and Liu, Q. (2018). Eplerenone modulates interleukin-33/sST2 signaling and IL-1beta in left ventricular systolic dysfunction after acute myocardial infarction. J. Interferon Cytokine Res. 38, 137–144. doi: 10.1089/jir.2017.0067
Chen, Y., Zhao, Y., Chen, W., Xie, L., Zhao, Z. A., Yang, J., et al. (2017). MicroRNA-133 overexpression promotes the therapeutic efficacy of mesenchymal stem cells on acute myocardial infarction. Stem Cell Res. Ther. 8:268. doi: 10.1186/s13287-017-0722-z
Cheng, M., Yang, J., Zhao, X., Zhang, E., Zeng, Q., Yu, Y., et al. (2019). Circulating myocardial microRNAs from infarcted hearts are carried in exosomes and mobilise bone marrow progenitor cells. Nat. Commun. 10:959. doi: 10.1038/s41467-019-08895-7
Chistiakov, D. A., Orekhov, A. N., and Bobryshev, Y. V. (2016). Cardiac-specific miRNA in cardiogenesis, heart function, and cardiac pathology (with focus on myocardial infarction). J. Mol. Cell. Cardiol. 94, 107–121. doi: 10.1016/j.yjmcc.2016.03.015
Colombo, M., Raposo, G., and Thery, C. (2014). Biogenesis, secretion, and intercellular interactions of exosomes and other extracellular vesicles. Annu. Rev. Cell Dev. Biol. 30, 255–289. doi: 10.1146/annurev-cellbio-101512-122326
Cuevas-Ramos, D., Almeda-Valdes, P., Meza-Arana, C. E., Brito-Cordova, G., Gomez-Perez, F. J., Mehta, R., et al. (2012). Exercise increases serum fibroblast growth factor 21 (FGF21) levels. PLoS One 7:e38022. doi: 10.1371/journal.pone.0038022
Cui, J., Zhang, F., Wang, Y., Liu, J., Ming, X., Hou, J., et al. (2016). Macrophage migration inhibitory factor promotes cardiac stem cell proliferation and endothelial differentiation through the activation of the PI3K/Akt/mTOR and AMPK pathways. Int. J. Mol. Med. 37, 1299–1309. doi: 10.3892/ijmm.2016.2542
Davidson, S. M., Takov, K., and Yellon, D. M. (2017). Exosomes and cardiovascular protection. Cardiovasc. Drugs Ther. 31, 77–86. doi: 10.1007/s10557-016-6698-6
Doroudgar, S., and Glembotski, C. C. (2011). The cardiokine story unfolds: ischemic stress-induced protein secretion in the heart. Trends Mol. Med. 17, 207–214. doi: 10.1016/j.molmed.2010.12.003
Duan, M.-J., Yan, M.-L., Wang, Q., Mao, M., Su, D., Sun, L.-L., et al. (2018). Overexpression of miR-1 in the heart attenuates hippocampal synaptic vesicle exocytosis by the posttranscriptional regulation of SNAP-25 through the transportation of exosomes. Cell Commun. Signal. 16:91. doi: 10.1186/s12964-018-0303-5
Emmerson, P. J., Wang, F., Du, Y., Liu, Q., Pickard, R. T., Gonciarz, M. D., et al. (2017). The metabolic effects of GDF15 are mediated by the orphan receptor GFRAL. Nat. Med. 23, 1215–1219. doi: 10.1038/nm.4393
Fang, S. J., Li, P. Y., Wang, C. M., Xin, Y., Lu, W. W., Zhang, X. X., et al. (2017). Inhibition of endoplasmic reticulum stress by neuregulin-1 protects against myocardial ischemia/reperfusion injury. Peptides 88, 196–207. doi: 10.1016/j.peptides.2016.12.009
Fruhbeis, C., Helmig, S., Tug, S., Simon, P., and Kramer-Albers, E. M. (2015). Physical exercise induces rapid release of small extracellular vesicles into the circulation. J. Extracell. Vesicles 4:28239. doi: 10.3402/jev.v4.28239
Gallet, R., Dawkins, J., Valle, J., Simsolo, E., De Couto, G., Middleton, R., et al. (2017). Exosomes secreted by cardiosphere-derived cells reduce scarring, attenuate adverse remodelling, and improve function in acute and chronic porcine myocardial infarction. Eur. Heart J. 38, 201–211. doi: 10.1093/eurheartj/ehw240
Gao, L., Gregorich, Z. R., Zhu, W., Mattapally, S., Oduk, Y., and Lou, X. (2018). Large cardiac muscle patches engineered from human induced-pluripotent stem cell-derived cardiac cells improve recovery from myocardial infarction in swine. Circulation 137, 1712–1730. doi: 10.1161/CIRCULATIONAHA.117.030785
Geng, L., Liao, B., Jin, L., Huang, Z., Triggle, C. R., Ding, H., et al. (2019). Exercise alleviates obesity-induced metabolic dysfunction via enhancing FGF21 sensitivity in adipose tissues. Cell Rep. 26:e2734. doi: 10.1016/j.celrep.2019.02.014
Glass, C., and Singla, D. K. (2011). ES cells overexpressing microRNA-1 attenuate apoptosis in the injured myocardium. Mol. Cell. Biochem. 357, 135–141. doi: 10.1007/s11010-011-0883-5
Gomes, C. P., Oliveira, GP Jr, Madrid, B., Almeida, J. A., Franco, O. L., and Pereira, R. W. (2014). Circulating miR-1, miR-133a, and miR-206 levels are increased after a half-marathon run. Biomarkers 19, 585–589. doi: 10.3109/1354750X.2014.952663
Gong, L. C., Xu, H. M., Guo, G. L., Zhang, T., Shi, J. W., and Chang, C. (2017). Long non-coding RNA H19 protects H9c2 cells against hypoxia-induced injury by targeting MicroRNA-139. Cell. Physiol. Biochem. 44, 857–869. doi: 10.1159/000485354
Guo, X., Wu, X., Han, Y., Tian, E., and Cheng, J. (2019). LncRNA MALAT1 protects cardiomyocytes from isoproterenol-induced apoptosis through sponging miR-558 to enhance ULK1-mediated protective autophagy. J. Cell. Physiol. 234, 10842–10854. doi: 10.1002/jcp.27925
Guo, Y., Liu, Q., Gui, Y., Liao, C., and Xu, D. (2016). Exercise promotes cardiac-specific fibroblast growth factor 21 expression. Int J Cardiol 203, 532–533. doi: 10.1016/j.ijcard.2015.10.231
Guo, Y., Luo, F., Liu, Q., and Xu, D. (2017). Regulatory non-coding RNAs in acute myocardial infarction. J. Cell. Mol. Med. 21, 1013–1023. doi: 10.1111/jcmm.13032
Guo, Y., Luo, F., Zhang, X., Chen, J., Shen, L., Zhu, Y., et al. (2018). TPPU enhanced exercise-induced epoxyeicosatrienoic acid concentrations to exert cardioprotection in mice after myocardial infarction. J. Cell. Mol. Med. 22, 1489–1500. doi: 10.1111/jcmm.13412
Hagström, E., James, S. K., Bertilsson, M., Becker, R. C., Himmelmann, A., and Husted, S. (2016). Growth differentiation factor-15 level predicts major bleeding and cardiovascular events in patients with acute coronary syndromes: results from the PLATO study. Eur. Heart J. 37, 1325–1333. doi: 10.1093/eurheartj/ehv491
Hedhli, N., Huang, Q., Kalinowski, A., Palmeri, M., Hu, X., Russell, R. R., et al. (2011). Endothelium-derived neuregulin protects the heart against ischemic injury. Circulation 123, 2254–2262. doi: 10.1161/CIRCULATIONAHA.110.991125
Hu, M., Guo, G., Huang, Q., Cheng, C., Xu, R., Li, A., et al. (2018). The harsh microenvironment in infarcted heart accelerates transplanted bone marrow mesenchymal stem cells injury: the role of injured cardiomyocytes-derived exosomes. Cell Death Dis. 9:357. doi: 10.1038/s41419-018-0392-5
Hu, S., Cao, S., Tong, Z., and Liu, J. (2018). FGF21 protects myocardial ischemia-reperfusion injury through reduction of miR-145-mediated autophagy. Am. J. Transl. Res. 10, 3677–3688.
Huang, F., Li, M. L., Fang, Z. F., Hu, X. Q., Liu, Q. M., Liu, Z. J., et al. (2013). Overexpression of MicroRNA-1 improves the efficacy of mesenchymal stem cell transplantation after myocardial infarction. Cardiology 125, 18–30. doi: 10.1159/000347081
Huang, P., Wang, L., Li, Q., Tian, X., Xu, J., Xu, J., et al. (2019). Atorvastatin enhances the therapeutic efficacy of mesenchymal stem cells derived exosomes in acute myocardial infarction via up-regulating long non-coding RNA H19. Cardiovasc. Res. doi: 10.1093/cvr/cvz139 [Epub ahead of print].
Huang, S., Zhang, L., Song, J., Wang, Z., Huang, X., Guo, Z., et al. (2019). Long noncoding RNA MALAT1 mediates cardiac fibrosis in experimental postinfarct myocardium mice model. J. Cell. Physiol. 234, 2997–3006. doi: 10.1002/jcp.27117
Izarra, A., Moscoso, I., Levent, E., Canon, S., Cerrada, I., Diez-Juan, A., et al. (2014). miR-133a enhances the protective capacity of cardiac progenitors cells after myocardial infarction. Stem Cell Rep. 3, 1029–1042. doi: 10.1016/j.stemcr.2014.10.010
Jiang, C., Ji, N., Luo, G., Ni, S., Zong, J., Chen, Z., et al. (2014). The effects and mechanism of miR-92a and miR-126 on myocardial apoptosis in mouse ischemia-reperfusion model. Cell Biochem. Biophys. 70, 1901–1906. doi: 10.1007/s12013-014-0149-4
Joki, Y., Ohashi, K., Yuasa, D., Shibata, R., Ito, M., Matsuo, K., et al. (2015). FGF21 attenuates pathological myocardial remodeling following myocardial infarction through the adiponectin-dependent mechanism. Biochem. Biophys. Res. Commun. 459, 124–130. doi: 10.1016/j.bbrc.2015.02.081
Kempf, T., Zarbock, A., Widera, C., Butz, S., Stadtmann, A., Rossaint, J., et al. (2011). GDF-15 is an inhibitor of leukocyte integrin activation required for survival after myocardial infarction in mice. Nat. Med. 17, 581–588. doi: 10.1038/nm.2354
Khan, S. Q., Ng, K., Dhillon, O., Kelly, D., Quinn, P., Squire, I. B., et al. (2009). Growth differentiation factor-15 as a prognostic marker in patients with acute myocardial infarction. Eur. Heart J. 30, 1057–1065. doi: 10.1093/eurheartj/ehn600
Kleinert, M., Clemmensen, C., Sjoberg, K. A., Carl, C. S., Jeppesen, J. F., Wojtaszewski, J. F. P., et al. (2018). Exercise increases circulating GDF15 in humans. Mol. Metab. 9, 187–191. doi: 10.1016/j.molmet.2017.12.016
Kon, M., Ebi, Y., and Nakagaki, K. (2019a). Effects of acute sprint interval exercise on follistatin-like 1 and apelin secretions. Arch. Physiol. Biochem. doi: 10.1080/13813455.2019.1628067 [Epub ahead of print].
Kon, M., Ebi, Y., and Nakagaki, K. (2019b). Effects of a single bout of high-intensity interval exercise on C1q/TNF-related proteins. Appl. Physiol. Nutr. Metab. 44, 47–51. doi: 10.1139/apnm-2018-0355
Kowal, J., Tkach, M., and Thery, C. (2014). Biogenesis and secretion of exosomes. Curr. Opin. Cell Biol. 29, 116–125. doi: 10.1016/j.ceb.2014.05.004
Lear, S. A., Hu, W., Rangarajan, S., Gasevic, D., Leong, D., Iqbal, R., et al. (2017). The effect of physical activity on mortality and cardiovascular disease in 130 000 people from 17 high-income, middle-income, and low-income countries: the PURE study. Lancet 390, 2643–2654. doi: 10.1016/S0140-6736(17)31634-3
Lee, P., Linderman, J. D., Smith, S., Brychta, R. J., Wang, J., Idelson, C., et al. (2014). Irisin and FGF21 are cold-induced endocrine activators of brown fat function in humans. Cell Metab. 19, 302–309. doi: 10.1016/j.cmet.2013.12.017
Li, H., Liao, Y., Gao, L., Zhuang, T., Huang, Z., Zhu, H., et al. (2018). Coronary serum exosomes derived from patients with myocardial ischemia regulate angiogenesis through the miR-939-mediated nitric oxide signaling pathway. Theranostics 8, 2079–2093. doi: 10.7150/thno.21895
Li, J., Shen, D., Tang, J., Wang, Y., Wang, B., Xiao, Y., et al. (2019). IL33 attenuates ventricular remodeling after myocardial infarction through inducing alternatively activated macrophages ethical standards statement. Eur. J. Pharmacol. 854, 307–319. doi: 10.1016/j.ejphar.2019.04.046
Li, L., Wang, Q., Yuan, Z., Chen, A., Liu, Z., Wang, Z., et al. (2018). LncRNA-MALAT1 promotes CPC proliferation and migration in hypoxia by up-regulation of JMJD6 via sponging miR-125. Biochem. Biophys. Res. Commun. 499, 711–718. doi: 10.1016/j.bbrc.2018.03.216
Li, S., Xiao, F. Y., Shan, P. R., Su, L., Chen, D. L., Ding, J. Y., et al. (2015). Overexpression of microRNA-133a inhibits ischemia-reperfusion-induced cardiomyocyte apoptosis by targeting DAPK2. J. Hum. Genet. 60, 709–716. doi: 10.1038/jhg.2015.96
Liao, Q., Qu, S., Tang, L. X., Li, L. P., He, D. F., Zeng, C. Y., et al. (2019). Irisin exerts a therapeutic effect against myocardial infarction via promoting angiogenesis. Acta Pharmacol. Sin. 40, 1314–1321. doi: 10.1038/s41401-019-0230-z
Liu, Y., Liu, S. X., Cai, Y., Xie, K. L., Zhang, W. L., and Zheng, F. (2015). Effects of combined aerobic and resistance training on the glycolipid metabolism and inflammation levels in type 2 diabetes mellitus. J. Phys. Ther. Sci. 27, 2365–2371. doi: 10.1589/jpts.27.2365
Loffler, D., Muller, U., Scheuermann, K., Friebe, D., Gesing, J., Bielitz, J., et al. (2015). Serum irisin levels are regulated by acute strenuous exercise. J. Clin. Endocrinol. Metab. 100, 1289–1299. doi: 10.1210/jc.2014-2932
Loyer, X., Zlatanova, I., Devue, C., Yin, M., Howangyin, K. Y., Klaihmon, P., et al. (2018). Intra-cardiac release of extracellular vesicles shapes inflammation following myocardial infarction. Circ. Res. 123, 100–106. doi: 10.1161/CIRCRESAHA.117.311326
Maruyama, S., Nakamura, K., Papanicolaou, K. N., Sano, S., Shimizu, I., Asaumi, Y., et al. (2016). Follistatin-like 1 promotes cardiac fibroblast activation and protects the heart from rupture. EMBO Mol. Med. 8, 949–966. doi: 10.15252/emmm.201506151
Michalik, K. M., You, X., Manavski, Y., Doddaballapur, A., Zornig, M., Braun, T., et al. (2014). Long noncoding RNA MALAT1 regulates endothelial cell function and vessel growth. Circ. Res. 114, 1389–1397. doi: 10.1161/CIRCRESAHA.114.303265
Moholdt, T., Lavie, C. J., and Nauman, J. (2018). Sustained physical activity, not weight loss, associated with improved survival in coronary heart disease. J. Am. Coll. Cardiol. 71, 1094–1101. doi: 10.1016/j.jacc.2018.01.011
Nygaard, H., Slettalokken, G., Vegge, G., Hollan, I., Whist, J. E., Strand, T., et al. (2015). Irisin in blood increases transiently after single sessions of intense endurance exercise and heavy strength training. PLoS One 10:e0121367. doi: 10.1371/journal.pone.0121367
Ogawa, T., and de Bold, A. J. (2014). The heart as an endocrine organ. Endocr. Connect. 3, R31–R44. doi: 10.1530/EC-14-0012
Pedersen, B. K. (2017). Anti-inflammatory effects of exercise: role in diabetes and cardiovascular disease. Eur. J. Clin. Invest. 47, 600–611. doi: 10.1111/eci.12781
Pietrangelo, T., Di Filippo, E. S., Mancinelli, R., Doria, C., Rotini, A., Fano-Illic, G., et al. (2015). Low intensity exercise training improves skeletal muscle regeneration potential. Front. Physiol. 6:399. doi: 10.3389/fphys.2015.00399
Rainer, P. P., Hao, S., Vanhoutte, D., Lee, D. I., Koitabashi, N., Molkentin, J. D., et al. (2014). Cardiomyocyte-specific transforming growth factor beta suppression blocks neutrophil infiltration, augments multiple cytoprotective cascades, and reduces early mortality after myocardial infarction. Circ. Res. 114, 1246–1257. doi: 10.1161/CIRCRESAHA.114.302653
Ribeiro-Rodrigues, T. M., Laundos, T. L., Pereira-Carvalho, R., Batista-Almeida, D., Pereira, R., Coelho-Santos, V., et al. (2017). Exosomes secreted by cardiomyocytes subjected to ischaemia promote cardiac angiogenesis. Cardiovasc. Res. 113, 1338–1350. doi: 10.1093/cvr/cvx118
Safdar, A., Saleem, A., and Tarnopolsky, M. A. (2016). The potential of endurance exercise-derived exosomes to treat metabolic diseases. Nat. Rev. Endocrinol. 12, 504–517. doi: 10.1038/nrendo.2016.76
Sanchis-Gomar, F., Perez-Quilis, C., and Lucia, A. (2016). Overexpressing FSTL1 for heart repair. Trends Mol. Med. 22, 353–354. doi: 10.1016/j.molmed.2016.03.003
Shang, J. L., Cheng, Q., Duan, S. J., Li, L., and Ly, J. (2018). Cognitive improvement following ischemia/reperfusion injury induced by voluntary running-wheel exercise is associated with LncMALAT1-mediated apoptosis inhibition. Int. J. Mol. Med. 41, 2715–2723. doi: 10.3892/ijmm.2018.3484
Shen, H., Cui, G., Li, Y., Ye, W., Sun, Y., Zhang, Z., et al. (2019). Follistatin-like 1 protects mesenchymal stem cells from hypoxic damage and enhances their therapeutic efficacy in a mouse myocardial infarction model. Stem Cell Res. Ther. 10:17. doi: 10.1186/s13287-018-1111-y
Shi, Y., Han, Y., Niu, L., Li, J., and Chen, Y. (2019). MiR-499 inhibited hypoxia/reoxygenation induced cardiomyocytes injury by targeting SOX6. Biotechnol. Lett. 41, 837–847. doi: 10.1007/s10529-019-02685-3
Shimano, M., Ouchi, N., and Walsh, K. (2012). Cardiokines: recent progress in elucidating the cardiac secretome. Circulation 126, e327–e332.
Soci, U. P. R., Fernandes, T., Barauna, V. G., Hashimoto, N. Y., De Fatima Alves Mota, G., Rosa, K. T., et al. (2016). Epigenetic control of exercise training-induced cardiac hypertrophy by miR-208. Clin. Sci. 130, 2005–2015. doi: 10.1042/CS20160480
Suire, C. N., Eitan, E., Shaffer, N. C., Tian, Q., Studenski, S., Mattson, M. P., et al. (2017). Walking speed decline in older adults is associated with elevated pro-BDNF in plasma extracellular vesicles. Exp. Gerontol. 98, 209–216. doi: 10.1016/j.exger.2017.08.024
Sun, R., and Zhang, L. (2019). Long non-coding RNA MALAT1 regulates cardiomyocytes apoptosis after hypoxia/reperfusion injury via modulating miR-200a-3p/PDCD4 axis. Biomed. Pharmacother. 111, 1036–1045. doi: 10.1016/j.biopha.2018.12.122
Tang, T. T., Li, Y. Y., Li, J. J., Wang, K., Han, Y., Dong, W. Y., et al. (2018). Liver-heart crosstalk controls IL-22 activity in cardiac protection after myocardial infarction. Theranostics 8, 4552–4562. doi: 10.7150/thno.24723
Taniguchi, H., Tanisawa, K., Sun, X., Kubo, T., and Higuchi, M. (2016). Endurance exercise reduces hepatic fat content and serum fibroblast growth factor 21 levels in Elderly Men. J. Clin. Endocrinol. Metab. 101, 191–198. doi: 10.1210/jc.2015-3308
Tsuchiya, Y., Ando, D., Takamatsu, K., and Goto, K. (2015). Resistance exercise induces a greater irisin response than endurance exercise. Metabolism 64, 1042–1050. doi: 10.1016/j.metabol.2015.05.010
Uhlemann, M., Mobius-Winkler, S., Fikenzer, S., Adam, J., Redlich, M., Mohlenkamp, S., et al. (2014). Circulating microRNA-126 increases after different forms of endurance exercise in healthy adults. Eur. J. Prev. Cardiol. 21, 484–491. doi: 10.1177/2047487312467902
Voss, S., Krüger, S., Scherschel, K., Warnke, S., Schwarzl, M., Schrage, B., et al. (2019). Macrophage migration inhibitory factor (MIF) expression increases during myocardial infarction and supports pro-inflammatory signaling in cardiac fibroblasts. Biomolecules 9:38. doi: 10.3390/biom9020038
Wahl, P., Wehmeier, U. F., Jansen, F. J., Kilian, Y., Bloch, W., Werner, N., et al. (2016). Acute effects of different exercise protocols on the circulating vascular microRNAs -16, -21, and -126 in trained subjects. Front. Physiol. 7:643. doi: 10.3389/fphys.2016.00643
Wang, B. L., Jin, H., Han, X. Q., Xia, Y., and Liu, N. F. (2018). Involvement of brain-derived neurotrophic factor in exerciseinduced cardioprotection of post-myocardial infarction rats. Int. J. Mol. Med. 42, 2867–2880. doi: 10.3892/ijmm.2018.3841
Wang, H., Zhao, Y. T., Zhang, S., Dubielecka, P. M., Du, J., Yano, N., et al. (2017). Irisin plays a pivotal role to protect the heart against ischemia and reperfusion injury. J. Cell. Physiol. 232, 3775–3785. doi: 10.1002/jcp.25857
Wang, Z. H., Sun, X. Y., Li, C. L., Sun, Y. M., Li, J., Wang, L. F., et al. (2017). miRNA-21 expression in the serum of elderly patients with acute myocardial infarction. Med. Sci. Monit. 23, 5728–5734. doi: 10.12659/msm.904933
Wang, J., Jia, Z., Zhang, C., Sun, M., Wang, W., Chen, P., et al. (2014). miR-499 protects cardiomyocytes from H2O2-induced apoptosis via its effects on Pdcd4 and Pacs2. RNA Biol. 11, 339–350. doi: 10.4161/rna.28300
Wang, J. X., Jiao, J. Q., Li, Q., Long, B., Wang, K., Liu, J. P., et al. (2011). miR-499 regulates mitochondrial dynamics by targeting calcineurin and dynamin-related protein-1. Nat. Med. 17, 71–78. doi: 10.1038/nm.2282
Wang, J. X., Zhang, X. J., Li, Q., Wang, K., Wang, Y., Jiao, J. Q., et al. (2015). MicroRNA-103/107 regulate programmed necrosis and myocardial ischemia/reperfusion injury through targeting FADD. Circ. Res. 117, 352–363. doi: 10.1161/CIRCRESAHA.117.305781
Wei, K., Serpooshan, V., Hurtado, C., Diez-Cunado, M., Zhao, M., Maruyama, S., et al. (2015). Epicardial FSTL1 reconstitution regenerates the adult mammalian heart. Nature 525, 479–485. doi: 10.1038/nature15372
Whitham, M., Parker, B. L., Friedrichsen, M., Hingst, J. R., Hjorth, M., Hughes, W. E., et al. (2018). Extracellular vesicles provide a means for tissue crosstalk during exercise. Cell Metab. 27, 237–251.e4. doi: 10.1016/j.cmet.2017.12.001
Widera, C., Giannitsis, E., Kempf, T., Korf-Klingebiel, M., Fiedler, B., Sharma, S., et al. (2012). Identification of follistatin-like 1 by expression cloning as an activator of the growth differentiation factor 15 gene and a prognostic biomarker in acute coronary syndrome. Clin. Chem. 58, 1233–1241. doi: 10.1373/clinchem.2012.182816
Xi, Y., Gong, D. W., and Tian, Z. (2016). FSTL1 as a potential mediator of exercise-induced cardioprotection in post-myocardial infarction rats. Sci. Rep. 6:32424. doi: 10.1038/srep32424
Xu, W. H., Wu, H., Xia, W. L., Lan, H., Wang, Y., Zhang, Y., et al. (2017). Physical exercise before pregnancy helps the development of mouse embryos produced in vitro. Mitochondrion 34, 36–42. doi: 10.1016/j.mito.2016.12.004
Yan, W., Guo, Y., Tao, L., Lau, W. B., Gan, L., Yan, Z., et al. (2017). C1q/Tumor necrosis factor-related protein-9 regulates the fate of implanted mesenchymal stem cells and mobilizes their protective effects against ischemic heart injury via multiple novel signaling pathways. Circulation 136, 2162–2177. doi: 10.1161/CIRCULATIONAHA.117.029557
Yan, X., Liu, J., Wu, H., Liu, Y., Zheng, S., Zhang, C., et al. (2016). Impact of miR-208 and its target gene nemo-like kinase on the protective effect of ginsenoside Rb1 in hypoxia/ischemia injuried cardiomyocytes. Cell. Physiol. Biochem. 39, 1187–1195. doi: 10.1159/000447825
Yang, L., Wang, B., Zhou, Q., Wang, Y., Liu, X., Liu, Z., et al. (2018). MicroRNA-21 prevents excessive inflammation and cardiac dysfunction after myocardial infarction through targeting KBTBD7. Cell Death Dis. 9:769. doi: 10.1038/s41419-018-0805-5
Yin, H., Li, P., Hu, F., Wang, Y., Chai, X., and Zhang, Y. (2014). IL-33 attenuates cardiac remodeling following myocardial infarction via inhibition of the p38 MAPK and NF-κB pathways. Mol. Med. Rep. 9, 1834–1838. doi: 10.3892/mmr.2014.2051
Yu, Y., Liu, H., Yang, D., He, F., Yuan, Y., Guo, J., et al. (2019). Aloe-emodin attenuates myocardial infarction and apoptosis via up-regulating miR-133 expression. Pharmacol. Res. 146:104315. doi: 10.1016/j.phrs.2019.104315
Zhang, Z., Wang, B., and Fei, A. (2019). BDNF contributes to the skeletal muscle anti-atrophic effect of exercise training through AMPK-PGC1alpha signaling in heart failure mice. Arch. Med. Sci. 15, 214–222. doi: 10.5114/aoms.2018.81037
Zhao, D., Feng, P., Sun, Y., Qin, Z., Zhang, Z., Tan, Y., et al. (2018). Cardiac-derived CTRP9 protects against myocardial ischemia/reperfusion injury via calreticulin-dependent inhibition of apoptosis. Cell Death Dis. 9:723. doi: 10.1038/s41419-018-0726-3
Zhao, Y. T., Wang, J., Yano, N., Zhang, L. X., Wang, H., Zhang, S., et al. (2019). Irisin promotes cardiac progenitor cell-induced myocardial repair and functional improvement in infarcted heart. J. Cell. Physiol. 234, 1671–1681. doi: 10.1002/jcp.27037
Zhou, M., Zou, Y. G., Xue, Y. Z., Wang, X. H., Gao, H., and Dong, H. W. (2018). Long non-coding RNA H19 protects acute myocardial infarction through activating autophagy in mice. Eur. Rev. Med. Pharmacol. Sci. 22, 5647–5651. doi: 10.26355/eurrev_201809_15831
Keywords: exercise, cardioprotective factors, exosomes, extracellular vesicles, myocardial infarction
Citation: Guo Y, Chen J and Qiu H (2020) Novel Mechanisms of Exercise-Induced Cardioprotective Factors in Myocardial Infarction. Front. Physiol. 11:199. doi: 10.3389/fphys.2020.00199
Received: 12 October 2019; Accepted: 21 February 2020;
Published: 10 March 2020.
Edited by:
Markos Klonizakis, Sheffield Hallam University, United KingdomReviewed by:
Mohsin Khan, Temple University, United StatesCopyright © 2020 Guo, Chen and Qiu. This is an open-access article distributed under the terms of the Creative Commons Attribution License (CC BY). The use, distribution or reproduction in other forums is permitted, provided the original author(s) and the copyright owner(s) are credited and that the original publication in this journal is cited, in accordance with accepted academic practice. No use, distribution or reproduction is permitted which does not comply with these terms.
*Correspondence: Yuan Guo, Z3VveXVhbjgxNDFAY3N1LmVkdS5jbg==
Disclaimer: All claims expressed in this article are solely those of the authors and do not necessarily represent those of their affiliated organizations, or those of the publisher, the editors and the reviewers. Any product that may be evaluated in this article or claim that may be made by its manufacturer is not guaranteed or endorsed by the publisher.
Research integrity at Frontiers
Learn more about the work of our research integrity team to safeguard the quality of each article we publish.