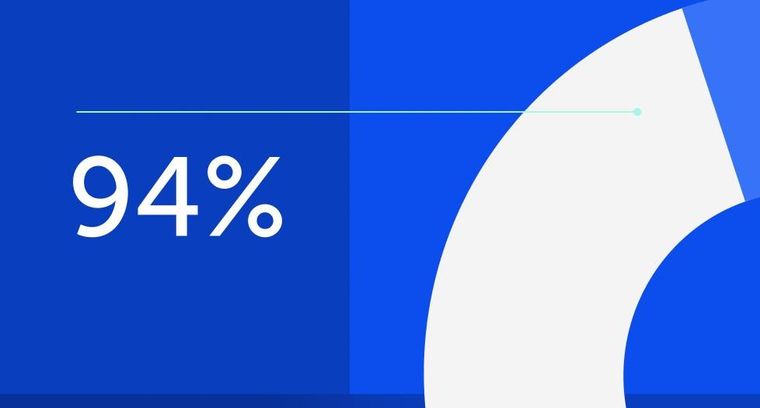
94% of researchers rate our articles as excellent or good
Learn more about the work of our research integrity team to safeguard the quality of each article we publish.
Find out more
ORIGINAL RESEARCH article
Front. Physiol., 21 January 2020
Sec. Invertebrate Physiology
Volume 10 - 2019 | https://doi.org/10.3389/fphys.2019.01623
Adaptations to low temperature play a critical role in restricting the geographical distribution of insects. Decreasing day lengths and temperatures trigger seasonal cold adaptations in insects. These adaptions include changes in expression at the miRNA, mRNA and protein levels. The rice water weevil (RWW), Lissorhoptrus oryzophilus, introduced from the Mississippi River, is a globally invasive pest of wetland rice that can survive at the northern border of China. To investigate the changes in expression at the protein level in overwintering female RWW adults, 6-plex tandem mass tags (TMTs) were used in overwintering and summer adults. By using a proteome database available for Curculionidae, 1077 proteins were quantified, 183 of which differed significantly between the overwintering and summer samples. To further understand these differentially expressed proteins (DEPs), bioinformatics analyses such as gene ontology (GO) enrichment analyses were performed. DEPs associated with the terms binding, structural molecule activity, catalytic activity, multicellular organismal process, extracellular region, chitin binding, metabolic process, intracellular part and organic cyclic compound binding were altered by selection during winter. The changes in the expression of these proteins suggest that the proteins are important for RWW survival in winter.
As an international quarantine species, rice water weevil (RWW; Lissorhoptrus oryzophilus Kuschel) causes severe economic problems in wetland rice agriculture, resulting in losses of up to 25% in untreated fields (Reay-Jones et al., 2008). The parthenogenetic female weevils invade rapidly in temperate rice growing regions around the world (Aghaee and Godfrey, 2014). In China, RWW was first identified in 1988; it is now distributed in 78% of the provinces and has become the most widespread invasive pest (Zhuo et al., 2018). This insect is located in areas with seasonal and environmental variability and must address periodic ecological adversity (Aghaee and Godfrey, 2014; Wan and Yang, 2016). Generally, RWW adults undergo prolonged exposure to subzero temperatures from October to March in the winter season in northern China, and the extremely low temperatures can even reach −30°C such as in Harbin (Li et al., 2018). To date, there has been little research on how RWW overcomes the physiological challenges in extreme winter conditions (Yang et al., 2018).
During overwintering, insects are exposed to fluctuating, freezing temperatures accompanied by adversities such as a risk of dehydration, lack of food and reduction in oxygen levels (Holmstrup et al., 2002). Among environmental factors, low temperature is a critical factor that affects the metabolic rate, distribution and ultimately the survival ability of insects (Wilches et al., 2016). As the metabolic rate of insects is temperature-dependent, low temperature causes indirect injuries such as persistent suppression of cellular metabolism, loss of ion homeostasis and accumulation of toxic metabolic end products (Dollo et al., 2010; Koštál et al., 2011; Teets and Denlinger, 2013; Sinclair, 2015). Direct injuries also lead to membrane damage, which is generally caused by phase transition, restructuring of the cytoskeleton caused by actin depolymerization and deleterious aggregations of denatured proteins. The crystallization of water also causes mechanical damage to cell membranes (Steponkus, 1984; Tursman and Duman, 1995; Feder and Hofmann, 1999; Kim et al., 2006; Teets and Denlinger, 2013).
Various aspects of the mechanism via which overwintering insects survive low temperature conditions have been studied (Rinehart et al., 2007; Clark and Worland, 2008; Fu et al., 2019; Hao et al., 2019). Insects enhance their cold resistance by means of behavioral, biochemical, and physiological strategies. In Japan, beetle larvae migrate to 10 cm below the surface of the soil, which provides a thermal buffer equivalent to moving 100 km south, and snow is another kind of thermal buffer (Sinclair et al., 2003). A variety of small molecules, such as polyhydric alcohols (glycerol, sorbitol, etc.), sugars (trehalose and glucose) and amino acids (proline and alanine) are thought to be cryoprotectants in insects (Bale and Hayward, 2010). These molecules are involved in stabilizing cell membranes and proteins, enhancing the supercooling ability of insects, and preventing infiltration damage to cells (Teets and Denlinger, 2013). Proline has been suggested to participate in the protection of membranes against dehydration during multigelation (Neufeld and Leader, 1998; Ramløv, 1999; Wharton, 2011). Unpredictable temperatures may greatly influence the cold hardiness of insects. Many freeze-avoiding insects (which die before they are frozen) have developed a rapid cold-hardening (RCH) mechanism, which instantaneously responds to severe fluctuations in temperature (Sinclair et al., 2003). Another strategy is diapause, a form of hormone-regulated dormancy that prepares the insect for expected periodic adverse conditions by stunting development and significant metabolic suppression (Lester and Irwin, 2012).
To have the minimal amount of energy needed to survive the winter and reemerge in the warm spring, overwintering insects must store enough energy prior to overwintering, as no food is available in winter (Sinclair, 2015). The main food stores are lipids, which offer the most dense energy storage. Sinclair et al. (2011) speculated that insects that do not feed during the winter rely on lipid consumption. Carbohydrates and storage proteins may also be used as fuel, and storage proteins may facilitate amino acid transfer between insect larvae and adults (Brien et al., 2002; Sinclair, 2015).
Proteomics methods have been developed successfully to investigate overwintering insects (Carrasco et al., 2011a, b; Teets and Denlinger, 2016). Tandem mass tag (TMT) technology is a powerful tool for precise and accurate quantitative proteomics. This method has been widely used to characterize protein expression profiles and investigate and compare functional changes at the protein level in vertebrates and invertebrates (Thingholm et al., 2010; Bogle et al., 2017). The 6-plex TMT method is a labeling strategy with six (126, 127, 128, 129, 130, 131) isobaric tags. Theoretically, these six labels have the same mass, and all the peptides in a sample are labeled with one chemical tag. The identical peptides from different samples produce identical precursor ions, and the same ions are used to compute quantity (Liu et al., 2016).
Using 6-plex TMT labeling-based proteomics approach, we identified and quantified differentially expressed proteins (DEPs) in overwintering and summer female RWW adults. The results offer insight into the differences in metabolic processes between the two stages and enhance our understanding of RWW overwintering diapause. The parthenogenetic female adult weevils are active in terms of feeding and reproduction in summer and can be used as control for overwintering responses. In addition, the results are crucial for predicting future outbreaks and their long-term effects.
Lissorhoptrus oryzophilus female adults were collected in mid-June 2017 (summer) and mid-November 2017 (overwintering), from paddies and ridges around Changchun (43°88′N, 125°35′E), Jilin Province, China. Both samples were immediately immersed in liquid nitrogen (one insect per tube), then transported to laboratory and stored at −80°C. Local temperature was shown in Supplementary Figure S1.
The summer and overwintering RWW (four adults and replicated three times each) were subjected to protein extraction by using the phenol extraction method, and proteins were precipitated by methanol/ammonium acetate as described previously by our laboratory (Yiou et al., 2013). The extracted samples were solubilized with lysis buffer. Protein concentrations were determined with the Modified Bradford Protein Assay Kit according to the manufacturer’s instructions (Liu et al., 2018).
One hundred micrograms of each protein was treated with 1 M DL-dithiothreitol (DTT) to obtain a final concentration of 50 mM and incubated for 1 h at 37°C. Then, 1 M iodoacetamide (IAA) was added to obtain a concentration of 120–150 mM, and the mixture was incubated for 30 min at room temperature (RT) in the dark. The samples were digested with trypsin at a ratio of 1:20 for 12–16 h. Then, the pH was adjusted to 8.5 with 1 M TEAB (pH 8.5). The samples were labeled using TMT six-plex Isobaric Label Reagent Set (Thermo Fisher Scientific, San Jose, CA, United States) according to the manufacturer’s protocols. Each peptide solution was incubated for 1 h at RT and quenched for 15 min with 8 μL of 5% hydroxylamine solution in water. After labeling, the samples were desalted by a SEP-PAK Kit. Sample loading was performed by eluting cartridges with 0.6 mL of 0.1% acetonitrile-miscible trifluoro acetic acid (TFA) twice and then with 0.6 mL of 0.1% water-miscible TFA. Then the samples were eluted with 0.1% formic acid and eluted with a mixed solvent of containing 80% acetonitrile and 0.1% formic acid (1.2 mL) for three times. The sample was then dried by using a vacuum centrifuge. To reduce the complexity and improve the separation, protein characterization and confidence level of the tested sample, the lyophilized TMT-labeled peptides were divided into 12 fractions by using a high performance liquid chromatography (HPLC) system (Shimadzu). Then, fractions were dried in a vacuum centrifuge and dissolved in 1% formic acid before mass spectrometry (MS) analysis.
Samples were introduced using an Easy-nLC 1000 system at a constant rate of 280 nL/min. The resulting samples were analyzed by a Q ExactiveTM hybrid quadrupole-Orbitrap mass spectrometer. Then the obtained peptides were loaded onto a nanospray ion (NSI) source, and tandem mass spectrometry (MS/MS) was performed in a Q Exactive spectrometer connected to an Ultra Performance LC (UPLC) system. Using normalized collision energy (NCE), the intact peptides were then selected for MS/MS with settings of 27, 30, and 33 to detect ion fragments in the Orbitrap. In an MS survey scan with a dynamic exclusion of 30.0 s, a data-dependent process was applied alternately between one MS scan and 20 MS/MS scans for the first 20 precursor ions above a threshold of 1.0E4. The fixed mass parameter was set to 100 m/z, and the electrospray voltage is 2.0 kV. Automatic gain control (AGC) was used to prevent overload of the ion trap; a total of 5E4 ions were accumulated for production of MS/MS spectra. For MS scans, the m/z scan range was set to 350–1800.
SEQUEST was used to extract MS/MS spectra. Charge state deconvolution and isotope removal were not performed. Using SEQUEST HT, the MS/MS spectra were searched against the Dendroctonus ponderosae proteome database with a common contaminant list. The fragment ion mass tolerance was 0.020 Da and the parent ion tolerance was 10.0 PPM. Carbamido methylation was specified as a fixed modification. Oxidation of methionine and acetylation of the N-terminus were specified as variable modifications. The final criterion was at least two peptide matches, and the false discovery rate (FDR) threshold was specified as 0.01. All contaminant and decoy proteins were removed from the data sets before downstream analysis. The overall workflow for quantitative comparison of proteomes between the overwintering and summer samples is shown in Figure 1.
Figure 1. Experiment strategy for the overwintering proteomic analysis in Lissorhoptrus oryzophilus. The strategy mainly involved protein extraction, TMT labeling and mass spectrometry analysis.
Using information provided by the UniProtKB/Swiss-Prot website1, the proteins in this study were classified according to subcellular localization and biological functions. The bioinformatics tool DAVID v6.72 (Database for Annotation, Visualization and Integrated Discovery) was used to determine the gene ontology (GO) terms and identify the active biological pathways. The EASE score was used to test the gene-enrichment, and the modified Fisher’s exact P-value (P < 0.05) was significant.
The significantly upregulated proteins [fold change (FC) > 1.5] between the overwintering and summer RWW samples were selected for protein-protein interaction network construction. First, using the annotated protein names, a list of gene symbols for these proteins was retrieved from FlyBase3. Second, the gene symbols were transferred to GeneMANIA for network visualization with the default parameters and algorithms, and proteins that failed to be incorporated into the network were deleted (Telegina et al., 2015; Yang et al., 2018). Third, the FC level was assigned to the corresponding proteins, and the overall pathways and functional clusters were identified in Cytoscape (ver. 3.4.0).
For glycogen content measurement, each sample containing four individuals was weighed and homogenized in 1 ml of ice-cold phosphate-buffered saline (PBS) and centrifuged at 3,000 × g for 30 min at 4°C. The supernatants were used to estimate the glycogen content according to the manufacturer’s instructions for the Glycogen Assay Kit. For glycerol content measurement, samples were weighed and homogenized in 0.05% v/v Tween 20. Free glycerol reagent (Sigma Aldrich, Inc., St. Louis, MO, United States) was added to the experimental samples, and the samples were incubated on ice for 15 min. The liquids were then centrifuged at 16,000 × g for 2 min. Absorbance was tested by spectrophotometry at 540 nm and calculated by comparison with a standard curve (Boychuk et al., 2015). The results from the above experiments are reported as milligrams per gram of fresh weight (FW).
To obtain information complementary to the TMT results, we selected examined the expression levels of genes involved in synthesis and degradation of triglycerides and glycogen. Total RNA from the overwintering and summer RWW samples was extracted using TRIzol (TaKaRa, China). The primers used for qPCR are listed in Supplementary Table S4, and GAPDH was used as an internal reference control. The qPCR conditions used were as described by Wang et al. (2016), and the relative expression levels were calculated using the 2–ΔΔCt method (Pfaffl, 2001). Statistical analysis was conducted by GraphPad Prism software (San Diego, CA, United States) (Wang et al., 2016).
Our proteomic analysis identified 1077 proteins, which were annotated by using the D. ponderosae genomic information in the NCBI non-redundant (Nr) protein database (Supplementary Table S1). Of these proteins, 183 were significant DEPs with abundances that changed >1.5-fold (overwintering/summer) and P-values of <0.05. A total of 79 proteins were upregulated, and 104 proteins were downregulated (orange and green background colors, respectively, in Supplementary Table S1). In addition, there were 16 and 2 uncharacterized proteins among the up- and downregulated DEPs, respectively.
The up- and downregulated DEPs were annotated by GO with Fisher’s exact test to better understand the roles that these proteins may play in cold adaptation. The significantly up- and downregulated DEPs were classified into three categories using GO terms: biological process (BP), cellular component (CC), and molecular function (MF). The downregulated DEPs were clustered into 50 BP terms (the most representative term was “metabolic process”), 18 MF terms (the most representative term was “organic cyclic compound binding”) and 20 CC terms (the most representative term was “intracellular part”). Each of the first eight terms in BP, MF and CC determined based on P-values, are listed in Figure 2.
Figure 2. Gene ontology enrichment of downregulated differentially expressed proteins in summer and overwintering samples.
As the upregulated DEPs may play important roles in winter acclimatization, we have listed detailed information regarding these upregulated DEPs in Table 1. Surprisingly, 25 DEPs were related to the “binding” term, which suggests that these proteins have binding characteristics. The other 22 DEPs belonged to the “structural molecule activity,” “catalytic activity,” and “signaling” terms. The “metal ion binding” term contained the most (12) proteins and was related to the binding of ferric, calcium, zinc and other ions. From the proteins with significant fold change above 1.5, the one with the highest FC was “N6U3H6,” which was a “DNA-directed RNA polymerase II subunit RPB1,” with an FC of 6.36. The protein with the highest molecular weight was “N6TY73,” which was an uncharacterized protein with a “calcium ion binding” function and a molecular weight of 788 kDa.
Table 1. Detailed information regarding upregulated differentially expressed proteins in L. oryzophilus under overwintering conditions.
Ribosomal proteins (RPs) are known for their importance in regulating protein synthesis and maintaining the stability of ribosomal complexes. Among the 1077 quantified proteins, 44 RPs were identified including 23 40S RPs and 21 60S RPs. Of the 183 significant DEPs, 22 RPs were downregulated (9 40S and 13 60S, Figure 3). All of these RPs had a significant score based on FC, and the very large number indicates that most RPs were likely downregulated in winter, indicating that the DEPs that were RPs play important roles in RWW overwintering. The four most strongly downregulated RPs were L15, L3, L7 and L27a, which were downregulated to 0.37, 0.37, 0.41, and 0.42 times of their previous levels (Supplementary Table S1).
Figure 3. Schematic representation of the differentially expressed proteins involved in the ribosome pathway. The green boxes indicate the downregulated proteins, the specific value of the fold change is supplemented in Supplementary Table S1.
Herein, we constructed a metabolic network of the main energy sources, including the citrate cycle, glycolysis/gluconeogenesis, fatty acid degradation and main amino acid (valine, leucine, and isoleucine) degradation, to reveal the energy change (EC) profiles of summer and overwintering adults (Figure 4). Some of these EC profiles contained several proteins, and the preferred upregulated proteins are shown. Overall, 39 proteins were identified and quantified in the constructed energy metabolism network, and detailed information regarding all of these proteins is provided in Supplementary Table S2. In total, 28 of the proteins (71.8%) showed a downregulated trend (FC < 1), including 16 (20) TCA, 4 (7) fatty acid degradation, 5 (7) main amino acid and 9 (15) glycolysis/gluconeogenesis proteins. In contrast, pyruvate carboxylase, mitochondrial (EC 6.4.1.1), pyruvate dehydrogenase (PDH) E1 component subunit β, mitochondrial (E1β, EC 1.2.4.1), and long-chain-fatty-acid-CoA ligase 5 (EC 6.2.1.3) were slightly upregulated (1.2 < FC < 1.5, P < 0.05), and retinal dehydrogenase 1 (EC 1.2.1.3) was significantly upregulated (FC ≥ 1.5, P < 0.05). In addition, retinal dehydrogenase 1 and 3-ketoacyl-CoA thiolase participated in several metabolic processes including fatty acid degradation and degradation of valine, leucine and isoleucine. In summary, citrate cycle and glycolysis/gluconeogenesis were decreased while fatty acid degradation was activated in overwintering female RWW.
Figure 4. The metabolism pathway of the main energy sources. Different colors indicate the ranges of the fold change (overwintering/summer) values. The numbers indicate the EC No. of the proteins, and the color of these numbers indicates differential extent changes. The specific value of the fold change is given in Supplementary Table S2.
The network contains 51 proteins, 35 of which were identified in the present study, and the remaining proteins were provided by GeneMANIA (Figure 5). Based on previous studies (Aerts et al., 2010; Guruharsha et al., 2011; Leader et al., 2018), the protein interaction networks were divided into three relation types, co-expression had the single largest share of constituents (62.46%), followed by physical interactions (35.96%) and co-localization (1.58%). Morphogenesis of embryonic epitheliumactin and cell junction were significantly overrepresented compared to the rest of the processes based on statistical results. The two proteins Fer1HCH (ferritin 1 heavy chain homolog) and Nrx-IV (neurexin IV) represented the hub with the most interactions in the network (Supplementary Table S3). Fer1HCH interacts with other proteins including cib, Jheh1, Hsp68, Arr1, Tina-1, Mgstl, and the GeneMANIA provided proteins Fer2LCH and pch2. The former proteins are associated with increased tolerance to oxidative stress and starvation. Fer2LCH provides iron nucleation sites and is essential for embryogenesis and the functions of pch2 in the monitoring the synaptonemal complex assembly and recombination during female meiosis. Up-regulation of these proteins may not only increase resistance to cold temperatures, but also be involved in parthenogenesis.
Figure 5. PPI network: significantly upregulated differentially expressed proteins between overwintering and summer RWW samples (Supplementary Table S3). The proteins upregulated in RWWs are colored in a gradient color from white to red, 1.52 ≤ FC ≤ 6.36.
We selectively tested the levels of glycogen and glycerol in summer and overwintering RWW female adults. Glycogen was significantly downregulated in overwintering adults while glycerol was significantly upregulated. Next, we tested the expression levels of four genes, namely, lipid storage droplet 1 (Lsd1), lipid storage droplet2 (Lsd2), glycogen phosphorylase (Gp), and glycogen synthase (Gs). The first two genes are involved in lipid storage droplet homeostasis (Teets and Denlinger, 2016; Tan et al., 2017). Lsd1 participates in the activation of triglyceride lipolysis (Fraser et al., 2017), while the role of Lsd2 is to help the accumulation of triglycerides in insect lipid droplets (Bonnett et al., 2012). The expression patterns are summarized in Figure 6. These proteins showed different expression patterns; Lsd1 was regulated in winter adults, while Lsd2 was downregulated. The latter two genes (Gp and Gs), involved in the synthesis and degradation of glycogen, also showed the same trend as Lsd1 and Lsd2.
Figure 6. Contents of glycogen and glycerol and qPCR data for expression levels of genes involved in synthesis and degradation of triglycerides and glycogen between overwintering and summer RWW samples. Statistically significant differences in gene expression are indicated with asterisks: ∗∗P < 0.01.
Cold adaptation is defined as the process of improving cold tolerance through prolonged exposure to subzero temperatures in the field or laboratory (Teets and Denlinger, 2013). Little attention is given to how RWW cope with subzero temperatures. In this study, we intended to reveal how protein expression changes in RWW under the influence of a long-term cold period in winter through quantitative proteomics and bioinformatics.
Because RWWs stop feeding during the winter, their limited energy reserves must be allocated between the maintenance of basal metabolism and production of cryoprotectants. We detected 28 downregulated proteins and 11 upregulated protiens related to energy metabolism. The levels of most of the enzymes functioning in the maintenance of basal metabolism through TCA and glycolysis were decreased in overwintering adults compared to summer adults, as shown in Figure 4. Inhibition of citrate cycle activity was also observed in flesh flies, in which the levels of the intermediate products fumaric acid and citric acid were decreased during diapause (Michaud and Denlinger, 2007).
Downregulation of the key enzymes hexokinase, 6-phosphofructokinase and pyruvate kinase indicated similar results in glycolysis (Smolinski et al., 2017). Hexokinase showed a significant decrease in activity and substrate affinity under freezing conditions (Penning de Vries, 1975). Furthermore, the inhibition of phosphofructokinase at low temperatures leads to decreased glycolysis (Storey, 1987). Pyruvate kinase is a primary regulator in both glycolysis and carbohydrate metabolism. The inhibition of pyruvate kinase was accompanied by an increase in the rate of gluconeogenesis, which indicates the accumulation of glucose (Smolinski et al., 2017; Petchampai et al., 2019).
Long-chain-fatty-acid–CoA ligase 5 (ACSL5) activates free long-chain fatty acids from exogenous sources to synthesize triacylglycerol for intracellular storage and degradation via β-oxidation (Rajkumar et al., 2018). In fatty acid degradation, with a magnesium ion cofactor, ACSL5 catalyzes the conversion of a long-chain fatty acid and an acyl-CoA to the corresponding fatty acyl-CoA (Zhou et al., 2007). Upregulation of ACSL5 is a sign that the metabolic rate of fatty acid β-oxidation is increased (Rajkumar et al., 2018).
Glycerol is important for supercooling of the bodily fluids of insects, thus protecting them from low temperatures (Michaud and Denlinger, 2007). Glycerol is generally thought to accumulate from the degradation of fat or from synthetic pathways that utilize glycogen (Fraser et al., 2017). The up regulation of Lsd1 and down regulation of Lsd2 indicated that glycerol production in overwintering RWW probably occurs through a lipolytic source. The glycogen content was significantly downregulated in overwintering RWW adults, and qPCR results indicated that glycogen synthase was downregulated and glycogen phosphorylase was significantly upregulated. It was reported that fructose-1,6-diphosphatase and pyruvate kinase were inhibited during the glycerol accumulation by activation of glycogen phosphorylase (Kojić et al., 2018). The levels of both these enzymes were decreased (0.82 and 0.81) in overwintering adults, indicating that this route is also closely related to glycerol accumulation.
At low temperatures, certain proteins are selectively degraded to meet the needs of the cell (Guy et al., 2008; Zhang et al., 2019). In the present study, 20 PRs were identified as being downregulated. RPs are responsible for ribosome biogenesis and protein translation and play important roles in controlling cell growth, division and development (Barakat et al., 2001). Studies have indicated that RPs affect fertility, viability and certain phenotypes in Drosophila (Alonso and Santaren, 2006; Paik et al., 2012). At present, there are a limited number of reports on the role of ribosome genes in stress response in plants, and few studies have been conducted on insects. The low temperature degradation of RPs suggests an adjustment of translation in cell metabolism and promotes the process of polypeptide synthesis (Carrasco et al., 2011a). The most strongly downregulated RPs in this study were the 60S RPs L15, L3, and L13 and the 40S ribosomal protein S8. A cross-species reference related to cold stress identified by bioinformatic approaches revealed the downregulation of the 3S ribosomal protein S12 and a 5S ribosomal protein in response to different cold exposures (Carrasco et al., 2011c).
The kinetic properties of RPs are different at low temperatures than at other temperatures, and a large reduction in ribosome proteins may lead to translation inefficiency. Therefore, RWW may elevate the expression of L23 and S27 to compensate for this loss or for another purpose. Upregulated RPs at low temperature may enhance proper translation or function in ribosome assembly in response to growth demands. Based on this situation, ribosomes that are more active in winter might be very important for insect overwintering.
The levels of cytoskeletal elements, including cuticle proteins, tropomyosin, microtubule-associated proteins (MAPs), intermediate filaments, and microfilaments, were shown to be increased in insects during winter (Viswanathan and Zhu, 2002; Say, 2008; Carrasco et al., 2011b). Insects that exhibit upregulation of cytoskeletal elements during cold exposure and diapause include Culex pipiens, Sarcophaga crassipalpis, and Aphidius colemani (Robich et al., 2007; Yi et al., 2007; Shang et al., 2015). Upregulation of cytoskeleton-related proteins during diapause is a common response in insects, suggesting that remodeling of cytoskeletal elements plays a central role during diapause (Robich et al., 2007; Carrasco et al., 2011a).
According to the comparative proteomic analysis, MAPs showed 1.4-fold upregulation in the overwintering adults compared with summer adults. Structural MAPs are significant in the nervous system and function in both the control of microtubule cytoskeleton stability and interactions with postsynaptic proteins (Lepicard et al., 2014). Studies in Drosophila found that the gene futsch, an important gene for the whole MAP family, is involved in axon growth and neuronal development (Hummel et al., 2000), and this gene is expressed in the nervous system with the microtubule cytoskeleton at the neuromuscular junction in Drosophila larvae (Drewes et al., 1997).
Tropomyosin-1 is the main isoform of tropomyosin that binds and stabilizes actin cables and fibers (Singer and Shaw, 2003). This isoform plays important roles in the dynamic regulation and organization of actin filaments by inhibiting both depolymerization and polymerization of actin (Gunning et al., 2008). A previous study found elevated expression levels of tropomyosin (tropomyosin-2-like protein) in S. crassipalpis in response to RCH (Li and Denlinger, 2008). Furthermore, tropomyosin was shown to be important for the stable arrangement of actin filaments in C. pipiens at low temperatures (Kim et al., 2006; Carrasco et al., 2011b).
Increasing evidence has suggested that the levels of cuticle proteins increase in response to abiotic stress, such as in A. colemani during cold stress and the Colorado potato beetle, Leptinotarsa decemlineata, during desiccation stress (Zhang et al., 2008). These lines of evidence indicate that cuticular proteins may be involved in the prevention of cross-cuticular freezing, and may be so in RWW, representing a critical source of protection for these insects, as any ice formation outside or within the insect cuticle tends to be lethal.
Insect neurons are protected from ions in the hemolymph by the tight septate junctions of glial cells that compose the blood–brain barrier (Stork et al., 2008). Thus, if most of the body fluid is transformed to ice, the concentrations of the remaining fluids including metal ions and other solutes are significantly increased (Storey and Storey, 2012). Ion sequestering is needed in this process. Long-term cold exposure causes cellular depolarization in insects, which is accompanied by an increase in K+ levels in the hemolymph (Overgaard and MacMillan, 2017). This would depolarize neuronal membranes, leading to initial hyperactivity followed by complete nervous system silencing. Thus, K+ homeostasis is critical for preservation of a negative resting membrane potential (Dawson et al., 1989; Fitzgerald et al., 1996). In addition, low temperature also impairs the activation of the L-type Ca2+ channels responsible for the action potential (AP) in larval Drosophila (Frolov and Singh, 2013). Cold-induced reductions in muscle AP amplitude and muscle force production seem to result from the effects of both temperature and membrane depolarization on excitation-contraction coupling (Frolov and Singh, 2013; Findsen et al., 2014, 2016).
A relationship between insect cold resistance and metal ion binding ability has been indicated (Storey and Storey, 2012). In our findings, ferritin was identified as a significantly upregulated ion binding protein, which is consistent with cold treatment studies in many insects. The ferritin level was significantly increased in Epiblema scudderiana after short-term cold exposure (Storey and Storey, 2010), and this protein was upregulated in response to cold in D. ponderosae (Bonnett et al., 2012). Transferrin was also induced by cold stress, showing up regulation at 4°C in the mulberry longhorn beetle (Sik et al., 2006). These proteins isolate free iron in bodily fluids to reduce the potential damage from the production of toxic free radicals, especially in freeze-tolerant insects with high concentrations of free metal in bodily fluids under freezing conditions (Sik et al., 2006). Transferrin is also known to be an antibiotic agent in insects which may reduce the risk of bacterial infections during the cold winter (Geiser and Winzerling, 2012). These factors may account for the multiple forms of iron-binding protein enhancement in RWW during the winter.
The main objective of this 6-plex TMT proteomic experiment was successfully attained, as DEPs between female adults of overwintering and summer RWWs were found. The carbohydrate metabolism rate was depressed during the low temperate according to the TCA and glycolysis while lipid metabolism (fatty acid degradation) was increased. RWW show significantly increased levels of some proteins: ACSL5 involved in fatty acid β-oxidation; RpL23 and RpS18 involved in translation; MAPs and Tropomyosin function in microtubule cytoskeleton ferritin, involved in iron ion homeostasis. This research comprehensively identified and analyzed DEPs in overwintering and summer female RWW adults. A better understanding of the DEPs could help determine their functional roles during cold tolerance and provide new targets for bio-tech strategies development for this globally important rice pest.
The raw data supporting the conclusions of this article will be made available by the authors, without undue reservation, to any qualified researcher.
ZXi, YS, and XJ conceived the study. YS and ZXi undertook the experiment, analyzed the data, and drafted the manuscript. ZXu and WS provided the insects. ZJ and XJ contributed to the manuscript revisions.
This research was supported by the National Key R&D Program of China (2018YFD0201000 and 2017YFD0200600).
The authors declare that the research was conducted in the absence of any commercial or financial relationships that could be construed as a potential conflict of interest.
The Supplementary Material for this article can be found online at: https://www.frontiersin.org/articles/10.3389/fphys.2019.01623/full#supplementary-material
FIGURE S1 | Field temperature during the study period.
TABLE S1 | Detailed information of all identified proteins.
TABLE S2 | Proteins in mainly energy source metabolism network.
TABLE S3 | Interacting upregulated DEPs and their network types based on the GeneMANIA network analysis.
TABLE S4 | Primers sequences used for qPCR amplification of selected genes for validation.
Aerts, S., Quan, X.-J., Claeys, A., Naval Sanchez, M., Tate, P., Yan, J., et al. (2010). Robust target gene discovery through transcriptome perturbations and genome-wide enhancer predictions in drosophila uncovers a regulatory basis for sensory specification. PLoS Biol. 8:e1000435. doi: 10.1371/journal.pbio.1000435
Aghaee, M., and Godfrey, L. D. (2014). A century of rice water weevil (Coleoptera: Curculionidae): a history of research and management with an emphasis on the United States. J. Integr. Pest Manag. 1958, 1–14. doi: 10.1603/ipm14011
Alonso, J., and Santaren, J. F. (2006). Characterization of the Drosophila melanogaster ribosomal proteome. J. Proteome Res. 5, 2025–2032.
Bale, J. S., and Hayward, S. A. L. (2010). Insect overwintering in a changing climate. J. Exp. Biol. 213, 980–994. doi: 10.1242/jeb.037911
Barakat, A., Szick-Miranda, K., Chang, I. F., Guyot, R., Blanc, G., Cooke, R., et al. (2001). The organization of cytoplasmic ribosomal protein genes in the Arabidopsis genome. Plant Physiol. 127, 398–415. doi: 10.1104/pp.127.2.398
Bogle, O. A., Kumar, K., Attardo-Parrinello, C., Lewis, S. E. M., Estanyol, J. M., Ballescà, J. L., et al. (2017). Identification of protein changes in human spermatozoa throughout the cryopreservation process. Andrology 5, 10–22. doi: 10.1111/andr.12279
Bonnett, T. R., Robert, J. A., Pitt, C., Fraser, J. D., Keeling, C. I., Bohlmann, J., et al. (2012). Global and comparative proteomic profiling of overwintering and developing mountain pine beetle, Dendroctonus ponderosae (Coleoptera: Curculionidae), larvae. Insect Biochem. Mol. Biol. 42, 890–901. doi: 10.1016/j.ibmb.2012.08.003
Boychuk, E. C., Smiley, J. T., Dahlhoff, E. P., Bernards, M. A., Rank, N. E., and Sinclair, B. J. (2015). Cold tolerance of the montane Sierra leaf beetle, Chrysomela aeneicollis. J. Insect Physiol. 81, 157–166. doi: 10.1016/j.jinsphys.2015.07.015
Brien, D. M. O., Fogel, M. L., and Boggs, C. L. (2002). Renewable and nonrenewable resources: amino acid turnover and allocation to reproduction in Lepidoptera. Proc. Natl. Acad. Sci. U.S.A. 99, 4413–4418. doi: 10.1073/pnas.072346699
Carrasco, M. A., Buechler, S. A., Arnold, R. J., Sformo, T., Barnes, B. M., and Duman, J. G. (2011a). Elucidating the biochemical overwintering adaptations of Larval Cucujus clavipes puniceus, a nonmodel organism, via high throughput proteomics. J. Proteome Res. 10, 4634–4646. doi: 10.1021/pr200518y
Carrasco, M. A., Buechler, S. A., Arnold, R. J., Sformo, T., Barnes, B. M., and Duman, J. G. (2011b). Investigating the deep supercooling ability of an Alaskan beetle, Cucujus clavipes puniceus, via high throughput proteomics. J. Proteomics 75, 1220–1234. doi: 10.1016/j.jprot.2011.10.034
Carrasco, M. A., Tan, J. C., and Duman, J. G. (2011c). A cross-species compendium of proteins / gene products related to cold stress identified by bioinformatic approaches. J. Insect Physiol. 57, 1127–1135. doi: 10.1016/j.jinsphys.2011.04.021
Clark, M. S., and Worland, M. R. (2008). How insects survive the cold: molecular mechanisms - a review. J. Comp. Physiol. B Biochem. Syst. Environ. Physiol. 178, 917–933. doi: 10.1007/s00360-008-0286-4
Dawson, J., Djamgoz, M. B. A., Hardie, J., and Irving, S. N. (1989). Components of resting membrane electrogenesis in Lepidopteran skeletal muscle. J. Insect Physiol. 35, 659–666. doi: 10.1016/0022-1910(89)90085-1
Dollo, V. H., Yi, S. X., and Lee, R. E. (2010). High temperature pulses decrease indirect chilling injury and elevate ATP levels in the flesh fly, Sarcophaga crassipalpis. Cryobiology 60, 351–353. doi: 10.1016/j.cryobiol.2010.03.002
Drewes, G., Ebneth, A., Preuss, U., Mandelkow, E. M., and Mandelkow, E. (1997). MARK, a novel family of protein kinases that phosphorylate microtubule- associated proteins and trigger microtubule disruption. Cell 89, 297–308. doi: 10.1016/s0092-8674(00)80208-1
Feder, M. E., and Hofmann, G. E. (1999). Heat-shock proteins, molecular chaperones, and the stress response: evolutionary and ecological physiology. Annu. Rev. Physiol. 61, 243–282. doi: 10.1146/annurev.physiol.61.1.243
Findsen, A., Overgaard, J., and Pedersen, T. H. (2016). Reduced L-type Ca 2 + current and compromised excitability induce loss of skeletal muscle function during acute cooling in locust. J. Exp Biol. 219(Pt 15), 2340–2348. doi: 10.1242/jeb.137604
Findsen, A., Pedersen, T. H., Petersen, A. G., Nielsen, O. B., and Overgaard, J. (2014). Why do insects enter and recover from chill coma? Low temperature and high extracellular potassium compromise muscle function in Locusta migratoria. J. Exp. Biol. 217, 1297–1306. doi: 10.1242/jeb.098442
Fitzgerald, E. M., Mba, D. S. D., and Stuart, J. D. (1996). Maintenance of the k+ activity gradient in insect muscle compared in Diptera and Lepidoptera- contributions of metabolic and exchanger mechanisms. J. Exp. Biol. 199, 1857–1872.
Fraser, J. D., Bonnett, T. R., Keeling, C. I., and Huber, D. P. (2017). Seasonal shifts in accumulation of glycerol biosynthetic gene transcripts in mountain pine beetle, Dendroctonus ponderosae Hopkins (Coleoptera: Curculionidae), larvae. Peer J. 5:e3284. doi: 10.7717/peerj.3284
Frolov, R. V., and Singh, S. (2013). Temperature and functional plasticity of L-type Ca2+ channels in Drosophila. Cell Calcium 54, 287–294. doi: 10.1016/j.ceca.2013.07.005
Fu, D., Dai, L., Gao, H., Sun, Y., Liu, B., and Chen, H. (2019). Identification, expression patterns and RNA interference of aquaporins in Dendroctonus armandi (Coleoptera: Scolytinae) larvae during overwintering. Front. Physiol. 10:967. doi: 10.3389/fphys.2019.00967
Geiser, D. L., and Winzerling, J. J. (2012). Insect transferrins: multifunctional proteins. Biochim. Biophys. Acta Gen. Subj. 1820, 437–451. doi: 10.1016/j.bbagen.2011.07.011
Gunning, P., O’neill, G., and Hardeman, E. (2008). Tropomyosin-based regulation of the actin cytoskeleton in time and space. Physiol. Rev. 88, 1–35. doi: 10.1152/physrev.00001.2007
Guruharsha, K. G., Rual, J. F., Zhai, B., Mintseris, J., Vaidya, P., Vaidya, N., et al. (2011). A protein complex network of Drosophila melanogaster. Cell 147, 690–703. doi: 10.1016/j.cell.2011.08.047
Guy, C., Kaplan, F., Kopka, J., Selbig, J., and Hincha, D. K. (2008). Metabolomics of temperature stress. Physiol. Plant 132, 220–235. doi: 10.1111/j.1399-3054.2007.00999.x
Hao, K., Ullah, H., Jarwar, A. R., Nong, X., Tu, X., and Zhang, Z. (2019). Molecular identification and diapause-related functional characterization of a novel dual-specificity kinase gene, MPKL, in Locusta migratoria. FEBS Lett. 593, 3064–3074. doi: 10.1002/1873-3468.13544
Holmstrup, M., Bayley, M., and Ramlov, H. (2002). Supercool or dehydrate? an experimental analysis of overwintering strategies in small permeable arctic invertebrates. Proc. Natl. Acad. Sci. U.S.A. 99, 5716–5720. doi: 10.1073/pnas.082580699
Hummel, T., Krukkert, K., Roos, J., Davis, G., Kla, C., and Mu, D. (2000). Drosophila Futsch / 22C10 Is a MAP1B-like protein required for dendritic and axonal development. Neuron 26, 357–370. doi: 10.1016/s0896-6273(00)81169-1
Kim, M., Robich, R. M., Rinehart, J. P., and Denlinger, D. L. (2006). Upregulation of two actin genes and redistribution of actin during diapause and cold stress in the northern house mosquito, Culex pipiens. J. Insect Physiol. 52, 1226–1233. doi: 10.1016/j.jinsphys.2006.09.007
Kojić, D., Popović, ŽD., Orčić, D., Purać, J., Orčić, S., Vukašinović, E. L., et al. (2018). The influence of low temperature and diapause phase on sugar and polyol content in the European corn borer Ostrinia nubilalis (Hbn.). J. Insect Physiol. 109, 107–113. doi: 10.1016/j.jinsphys.2018.07.007
Koštál, V., Korbelová, J., Rozsypal, J., Zahradníèková, H., Cimlová, J., Tomèala, A., et al. (2011). Long-term cold acclimation extends survival time at 0°C and modifies the metabolomic profiles of the larvae of the fruit fly Drosophila melanogaster. PLoS One 6:e25025. doi: 10.1371/journal.pone.0025025
Leader, D. P., Krause, S. A., Pandit, A., Davies, S. A., and Dow, J. A. T. (2018). FlyAtlas 2: a new version of the Drosophila melanogaster expression atlas with RNA-Seq, miRNA-Seq and sex-specific data. Nucleic Acids Res. 46, D809–D815. doi: 10.1093/nar/gkx976
Lepicard, S., Franco, B., de Bock, F., and Parmentier, M.-L. (2014). A presynaptic role of microtubule-associated protein 1/Futsch in Drosophila: regulation of active zone number and neurotransmitter release. J. Neurosci. 34, 6759–6771. doi: 10.1523/JNEUROSCI.4282-13.2014
Lester, J. D., and Irwin, J. T. (2012). Metabolism and cold tolerance of overwintering adult mountain pine beetles (Dendroctonus ponderosae): evidence of facultative diapause? J. Insect Physiol. 58, 808–815. doi: 10.1016/j.jinsphys.2012.03.003
Li, A., and Denlinger, D. L. (2008). Rapid cold hardening elicits changes in brain protein profiles of the flesh fly, Sarcophaga crassipalpis. Insect Mol. Biol. 17, 565–572. doi: 10.1111/j.1365-2583.2008.00827.x
Li, S., Jiang, D., Lian, Y., and Yin, L. (2018). Circulation characteristics of extreme cold events in Northeast China during wintertime. Chin. J. Atmos. Sci. 42, 963–976.
Liu, J. M., Sweredoski, M. J., and Hess, S. (2016). Improved 6-Plex tandem mass tags quantification throughput using a linear ion trap-high-energy collision induced dissociation MS3 scan. Anal. Chem. 88, 7471–7475. doi: 10.1021/acs.analchem.6b01067
Liu, J. Y., Chang, M. C., Meng, J. L., Feng, C. P., and Wang, Y. (2018). A comparative proteome approach reveals metabolic changes associated with Flammulina velutipes mycelia in response to cold and light stress. J. Agric. Food Chem. 66, 3716–3725. doi: 10.1021/acs.jafc.8b00383
Michaud, M. R., and Denlinger, D. L. (2007). Shifts in the carbohydrate, polyol, and amino acid pools during rapid cold-hardening and diapause-associated cold-hardening in flesh flies (Sarcophaga crassipalpis): a metabolomic comparison. J. Comp. Physiol. B Biochem. Syst. Environ. Physiol. 177, 753–763. doi: 10.1007/s00360-007-0172-5
Neufeld, D. S., and Leader, L. P. (1998). Freezing survival by isolated Malpighian tubules of the New Zealand alpine weta Hemideina maori. J. Exp. Biol. 201, 227–236.
Overgaard, J., and MacMillan, H. A. (2017). The integrative physiology of insect chill tolerance. Annu. Rev. Physiol. 79, 187–208. doi: 10.1146/annurev-physiol-022516-034142
Paik, D., Jang, Y. G., Lee, Y. E., Lee, Y. N., Yamamoto, R., Gee, H. Y., et al. (2012). Misexpression screen delineates novel genes controlling Drosophila lifespan. Mech. Ageing Dev. 133, 234–245. doi: 10.1016/j.mad.2012.02.001
Penning de Vries, F. W. T. (1975). The cost of maintenance processes in plant cells. Ann. Bot. 39, 77–92. doi: 10.1093/jxb/erw472
Petchampai, N., Murillo-solano, C., Isoe, J., Pizarro, J. C., and Scaraffia, P. Y. (2019). Distinctive regulatory properties of pyruvate kinase 1 from Aedes aegypti mosquitoes. Insect Biochem. Mol. Biol. 104, 82–90. doi: 10.1016/j.ibmb.2018.12.010
Pfaffl, M. W. (2001). A new mathematical model for relative quantification in real-time RT-PCR. Nucleic Acids Res. 29:e45.
Rajkumar, A., Liaghati, A., Chan, J., Lamothe, G., Dent, R., and Doucet, É, et al. (2018). ACSL5 genotype in fluence on fatty acid metabolism: a cellular, tissue, and whole-body study. Metabolism 83, 271–279. doi: 10.1016/j.metabol.2018.03.019
Ramløv, H. (1999). Microclimate and variations in haemolymph composition in the freezing-tolerant New Zealand alpine weta Hemideina maori Hutton (Orthoptera: Stenopelmatidae). J. Comp. Physiol. B Biochem. Syst. Environ. Physiol. 169, 224–235. doi: 10.1007/s003600050215
Reay-Jones, F. P. F., Way, M. O., and Tarpley, L. (2008). Nitrogen fertilization at the rice panicle differentiation stage to compensate for rice water weevil (Coleoptera: Curculionidae) injury. Crop Prot. 27, 84–89. doi: 10.1016/j.cropro.2007.04.009
Rinehart, J. P., Li, A., Yocum, G. D., Robich, R. M., Hayward, S. A. L., and Denlinger, D. L. (2007). Up-regulation of heat shock proteins is essential for cold survival during insect diapause. Proc. Natl. Acad. Sci. U.S.A. 104, 11130–11137. doi: 10.1073/pnas.0703538104
Robich, R. M., Rinehart, J. P., Kitchen, L. J., and Denlinger, D. L. (2007). Diapause-specific gene expression in the northern house mosquito, Culex pipiens L., identified by suppressive subtractive hybridization. J. Insect Physiol. 53, 235–245. doi: 10.1016/j.jinsphys.2006.08.008
Say, L. (2008). Environmental stresses induce the expression of putative glycine-rich insect cuticular protein genes in adult Leptinotarsa decemlineata (Say). Insect Mol. Biol. 17, 209–216. doi: 10.1111/j.1365-2583.2008.00796.x
Shang, Q., Pan, Y., Peng, T., Yang, S., Lu, X., Wang, Z., et al. (2015). Proteomics analysis of overexpressed plasma proteins in response to cold acclimation in Ostrinia furnacalis. Arch. Insect Biochem. Physiol. 90, 195–208. doi: 10.1002/arch.21302
Sik, K., Yeon, B., Ja, H., Jae, S., Joo, H., Soo, Y., et al. (2006). Transferrin inhibits stress-induced apoptosis in a beetle. Free Radic. Biol. Med. 41, 1151–1161. doi: 10.1016/j.freeradbiomed.2006.07.001
Sinclair, B. J. (2015). Linking energetics and overwintering in temperate insects. J. Therm. Biol. 54, 5–11. doi: 10.1016/j.jtherbio.2014.07.007
Sinclair, B. J., Bretman, A., Tregenza, T., Tomkins, J. L., and Hosken, D. J. (2011). Metabolic rate does not decrease with starvation in Gryllus bimaculatus when changing fuel use is taken into account. Physiol. Entomol. 36, 84–89. doi: 10.1111/j.1365-3032.2010.00765.x
Sinclair, B. J., Vernon, P., Klok, C. J., and Chown, S. L. (2003). Insects at low temperatures: an ecological perspective. Trends Ecol. Evol. 18, 257–262. doi: 10.1016/j.jtherbio.2016.06.026
Singer, J. M., and Shaw, J. M. (2003). Mdm20 protein functions with Nat3 protein to acetylate Tpm1 protein and regulate tropomyosin-actin interactions in budding yeast. Proc. Natl. Acad. Sci. U.S.A. 100, 7644–7649. doi: 10.1073/pnas.1232343100
Smolinski, M. B., Mattice, J. J. L., and Storey, K. B. (2017). Cryobiology regulation of pyruvate kinase in skeletal muscle of the freeze tolerant wood frog, Rana sylvatica. Cryobiology 77, 25–33. doi: 10.1016/j.cryobiol.2017.06.002
Steponkus, P. L. (1984). Role of the plasma membrane in freezing injury and cold acclimation. Annu. Rev. Plant Biol. 35, 543–584. doi: 10.1146/annurev.pp.35.060184.002551
Storey, K. B. (1987). Glycolysis and the regulation of cryoprotectant synthesis in liver of the freeze tolerant wood frog. J. Comp. Physiol. B 157, 373–380. doi: 10.1007/bf00693364
Storey, K. B., and Storey, J. M. (2010). “Oxygen: stress and adaptation in cold-hardy insects,” in Low Temperature Biology of Insects, eds D. L. Denlinger and R. E. Lee Jr. (Cambridge: Cambridge University Press), 141–165. doi: 10.1017/cbo9780511675997.007
Storey, K. B., and Storey, J. M. (2012). Insect cold hardiness: metabolic, gene, and protein adaptation. Can. J. Zool. 90, 456–475. doi: 10.1139/z2012-011
Stork, T., Engelen, D., Krudewig, A., Silies, M., Bainton, R. J., and Klambt, C. (2008). Organization and function of the blood brain barrier in Drosophila. J. Neurosci. 28, 587–597. doi: 10.1523/JNEUROSCI.4367-07.2008
Tan, Q. Q., Liu, W., Zhu, F., Lei, C. L., Hahn, D. A., and Wang, X. P. (2017). Describing the diapause-preparatory proteome of the beetle Colaphellus bowringi and identifying candidates affecting lipid accumulation using isobaric tags for mass spectrometry-based proteome quantification (iTRAQ). Front. Physiol. 8:251. doi: 10.3389/fphys.2017.00251
Teets, N. M., and Denlinger, D. L. (2013). Physiological mechanisms of seasonal and rapid cold-hardening in insects. Physiol. Entomol. 38, 105–116. doi: 10.1111/phen.12019
Teets, N. M., and Denlinger, D. L. (2016). Quantitative phosphoproteomics reveals signaling mechanisms associated with rapid cold hardening in a chill-tolerant fly. J. Proteome Res. 15, 2855–2862. doi: 10.1021/acs.jproteome.6b00427
Telegina, D. V., Korbolina, E. E., Ershov, N. I., Kolosova, N. G., and Kozhevnikova, O. S. (2015). Identification of functional networks associated with cell death in the retina of OXYS rats during the development of retinopathy. Cell Cycle 14, 3544–3556. doi: 10.1080/15384101.2015.1080399
Thingholm, T. E., Palmisano, G., Kjeldsen, F., and Larsen, M. R. (2010). Undesirable charge-enhancement of isobaric tagged phosphopeptides leads to reduced identification efficiency. J Proteome Res. 9, 4045–4052. doi: 10.1021/pr100230q
Tursman, D., and Duman, J. G. (1995). Cryoprotective effects of thermal hysteresis protein on survivorship of frozen gut cells from the freeze-tolerant centipede Lithobius forficatus. J. Exp. Zool. 272, 249–257. doi: 10.1002/jez.1402720402
Viswanathan, C., and Zhu, J. K. (2002). Molecular genetic analysis of cold-regulated gene transcription. Philos. Trans. R. Soc. B Biol. Sci. 357, 877–886. doi: 10.1098/rstb.2002.1076
Wan, F.-H., and Yang, N.-W. (2016). Invasion and management of agricultural alien insects in China. Annu. Rev. Entomol. 61, 77–98. doi: 10.1146/annurev-ento-010715-023916
Wang, Y., Chen, Q., Zhao, H., and Ren, B. (2016). Identification and comparison of candidate olfactory genes in the olfactory and non-olfactory organs of elm pest Ambrostoma quadriimpressum (coleoptera: Chrysomelidae) based on transcriptome analysis. PLoS One 11:e0147144. doi: 10.1371/journal.pone.0147144
Wharton, D. A. (2011). Cold tolerance of New Zealand alpine insects. J. Insect Physiol. 57, 1090–1095. doi: 10.1016/j.jinsphys.2011.03.004
Wilches, D. M., Laird, R. A., Floate, K. D., and Fields, P. G. (2016). A review of diapause and tolerance to extreme temperatures in dermestids (Coleoptera). J. Stored Prod. Res. 68, 50–62. doi: 10.1016/j.jspr.2016.04.004
Yang, S., Zhang, X., Wang, J., Wang, S., Pan, Y., Zhang, J., et al. (2018). Identification and analysis of up-regulated proteins in Lissorhoptrus oryzophilus adults for rapid cold hardening. Gene 642, 9–15. doi: 10.1016/j.gene.2017.11.002
Yi, S. X., Moore, C. W., and Lee, R. E. (2007). Rapid cold-hardening protects Drosophila melanogaster from cold-induced apoptosis. Apoptosis 12, 1183–1193. doi: 10.1007/s10495-006-0048-2
Yiou, P., Shaoli, A., Kebin, L., Tao, W., Kui, F., Hua, Z., et al. (2013). Evaluation of extraction procedures for 2-DE analysis of aphid proteins. J. Sep. Sci. 36, 532–539. doi: 10.1002/jssc.201200642
Zhang, C., Wei, D., Shi, G., Huang, X., Cheng, P., Liu, G., et al. (2019). Understanding the regulation of overwintering diapause molecular mechanisms in Culex pipiens pallens through comparative proteomics. Sci. Rep. 9, 1–12. doi: 10.1038/s41598-019-42961-w
Zhang, J., Goyer, C., and Pelletier, Y. (2008). Environmental stresses induce the expression of putative glycine-rich insect cuticular protein genes in adult Leptinotarsa decemlineata (Say). Insect Mol. Biol. 17, 209–216. doi: 10.1111/j.1365-2583.2008.00796.x
Zhou, Y., Abidi, P., Kim, A., Chen, W., Huang, T., Kraemer, F. B., et al. (2007). Transcriptional activation of hepatic ACSL3 and ACSL5 by oncostatin m reduces hypertriglyceridemia through enhanced beta-oxidation. Arterioscler. Thromb. Vasc. Biol. 27, 2198–2205. doi: 10.1161/atvbaha.107.148429
Keywords: insect proteomics, Lissorhoptrus oryzophilus, overwintering, DEP, TMT
Citation: Xinxin Z, Shuang Y, Xunming Z, Shang W, Juhong Z and Jinghui X (2020) TMT-Based Quantitative Proteomic Profiling of Overwintering Lissorhoptrus oryzophilus. Front. Physiol. 10:1623. doi: 10.3389/fphys.2019.01623
Received: 07 October 2019; Accepted: 24 December 2019;
Published: 21 January 2020.
Edited by:
Bin Tang, Hangzhou Normal University, ChinaReviewed by:
Suresh Kumar, Indian Agricultural Research Institute (ICAR), IndiaCopyright © 2020 Xinxin, Shuang, Xunming, Shang, Juhong and Jinghui. This is an open-access article distributed under the terms of the Creative Commons Attribution License (CC BY). The use, distribution or reproduction in other forums is permitted, provided the original author(s) and the copyright owner(s) are credited and that the original publication in this journal is cited, in accordance with accepted academic practice. No use, distribution or reproduction is permitted which does not comply with these terms.
*Correspondence: Zhang Juhong, emhqaHFoZEAxMjYuY29t; Xi Jinghui, amh4aTE5NjVAamx1LmVkdS5jbg==
Disclaimer: All claims expressed in this article are solely those of the authors and do not necessarily represent those of their affiliated organizations, or those of the publisher, the editors and the reviewers. Any product that may be evaluated in this article or claim that may be made by its manufacturer is not guaranteed or endorsed by the publisher.
Research integrity at Frontiers
Learn more about the work of our research integrity team to safeguard the quality of each article we publish.