- 1Department of Conservation Ecology & Entomology, Faculty of AgriSciences, Centre for Invasion Biology, Stellenbosch University, Stellenbosch, South Africa
- 2Department of Zoology, Stockholm University, Stockholm, Sweden
- 3South African Sugarcane Research Institute, Mount Edgecombe, South Africa
- 4CT Scanner Facility, Central Analytical Facilities, Stellenbosch University, Stellenbosch, South Africa
- 5Physics Department, Stellenbosch University, Stellenbosch, South Africa
Temperature has a profound impact on insect fitness and performance via metabolic, enzymatic or chemical reaction rate effects. However, oxygen availability can interact with these thermal responses in complex and often poorly understood ways, especially in hypoxia-adapted species. Here we test the hypothesis that thermal limits are reduced under low oxygen availability – such as might happen when key life-stages reside within plants – but also extend this test to attempt to explain that the magnitude of the effect of hypoxia depends on variation in key respiration-related parameters such as aerobic scope and respiratory morphology. Using two life-stages of a xylophagous cerambycid beetle, Cacosceles (Zelogenes) newmannii we assessed oxygen-limitation effects on metabolic performance and thermal limits. We complement these physiological assessments with high-resolution 3D (micro-computed tomography scan) morphometry in both life-stages. Results showed that although larvae and adults have similar critical thermal maxima (CTmax) under normoxia, hypoxia reduces metabolic rate in adults to a greater extent than it does in larvae, thus reducing aerobic scope in the former far more markedly. In separate experiments, we also show that adults defend a tracheal oxygen (critical) setpoint more consistently than do larvae, indicated by switching between discontinuous gas exchange cycles (DGC) and continuous respiratory patterns under experimentally manipulated oxygen levels. These effects can be explained by the fact that the volume of respiratory anatomy is positively correlated with body mass in adults but is apparently size-invariant in larvae. Thus, the two life-stages of C. newmannii display key differences in respiratory structure and function that can explain the magnitude of the effect of hypoxia on upper thermal limits.
Introduction
Temperature is a key environmental driver of insect population dynamics since it directly affects life-history traits (Angilletta, 2009). In the context of climate change, geographic range shifts of insects are tightly linked with climate variability at a range of spatial and temporal scales (Rosenzweig et al., 2008). Understanding insect responses to temperature is therefore crucial in estimating how global warming will affect terrestrial arthropods (Bale et al., 2002; Huey et al., 2012). This is particularly true for insect pests, whose distribution and population dynamics are likely to be modified with changing climate (Battisti and Larsson, 2015; Lehmann et al., 2019a). For these reasons, estimating thermal limits is critical, since they represent the environmental limits of key traits, such as activity, survival, development and reproduction of ectothermic organisms (Angilletta, 2009). Yet thermal tolerances are affected by a range of methodological and environmental factors (Kovacevic et al., 2019) at diverse time-scales, from evolutionary to more recent thermal history. Thermal limits can be influenced, for example, by a large number of endogenous and exogenous parameters (Colinet and Boivin, 2011) including sex (Colinet and Hance, 2009; Nyamukondiwa and Terblanche, 2009), the rate of change in temperature (Kelty and Lee, 1999), acclimation or acclimatization history (Sejerkilde et al., 2003; Hoffmann et al., 2005; Koštál et al., 2011), reproductive status (Marshall and Sinclair, 2010; Javal et al., 2016), stage of development (Zhang et al., 2015), age (Bowler and Terblanche, 2008), body condition (Mitchell et al., 2017), humidity (Terblanche et al., 2011; Bubliy et al., 2012), or photoperiod (Kim and Song, 2000; Fischer et al., 2012).
One major factor of interest is atmospheric oxygen levels, and how this interacts with – or perhaps jointly determines – organismal thermal tolerance. For example, using a microarray approach, Boardman et al. (2018) recently found a novel candidate gene with unknown functions underlying high temperature stress resistance during hypoxia in Drosophila melanogaster. The “oxygen-and capacity-limited thermal tolerance” (OCLTT) hypothesis proposes to explain variation in heat tolerance in ectotherms under oxygen-limited conditions. This hypothesis states that insufficient capacity to supply oxygen to meet the oxygen demand of tissues can cause a progressive decline in animal performance as temperatures deviate from the optimum (Giomi et al., 2014). Many aquatic species conform to one or several predictions derived from the OCLTT hypothesis (Pörtner, 2010). The results for terrestrial insects, however, remain somewhat equivocal with some species showing strong support (Woods and Hill, 2004; Boardman and Terblanche, 2015), some showing partial support (Stevens et al., 2010) and others showing little or no support (Klok et al., 2004; Lehmann et al., 2019b; reviewed in Verberk et al., 2016b). This hypothesis has proven controversial and is the focus of several recent discussions questioning, in particular, the appropriate methods for falsifying predictions thereof (Clark et al., 2013; Jutfelt et al., 2014; Pörtner, 2014) and how widespread the evidence might be supporting OCLTT across diverse taxa (Verberk et al., 2016b; Jutfelt et al., 2018). In cases where strong or obvious support was not found for OCLTT in terrestrial insects, the reasons are typically unclear and not the focus of current investigation. Most commonly, structure-function dynamics of the respiratory system is widely expected to drive variation in support for the OCLTT hypothesis even in air-breathing insects (Verberk et al., 2016b). Differences in respiratory media (gas vs. liquid physico-chemistry, reviewed in Verberk et al., 2016a; Terblanche and Woods, 2018) may also account for variation in support for the OCLTT hypothesis among diverse arthropods.
The longhorned beetle Cacosceles (Zelogenes) newmannii Thomson (1877) (Coleoptera: Cerambycidae) is native to Mozambique, Eswatini and South Africa. Larvae of this beetle were found feeding on sugarcane (Saccharum sp. hybrids) for the first time in 2015 in the KwaZulu-Natal Province of South Africa. They dig galleries into the sugarcane stool and upwards into the stalks, causing considerable crop loss, as sucrose is stored in the lower nodes of mature sugarcane stalks. Although the level of hypoxia expected inside living plant tissues can vary with many different parameters (Pincebourde and Casas, 2016), this species is expected to show hypoxia adaptations, at least at the larval stage, owing to the wood-boring nature of larvae (Way et al., 2017), although it is possible the sugarcane plant is more aerated than the woody natural host plants in the region.
We explored the potential connection between thermal tolerance and oxygen limitation in this cerambycid beetle using diverse experimental laboratory approaches. We investigated whether oxygen availability influenced upper critical (=lethal) temperature through thermolimit respirometry (TLR; Lighton and Turner, 2004) in larvae and adults under normoxia and hypoxia. Our first prediction was that hypoxia reduced upper critical temperature (CTmax) in both life stages. Our second prediction was that the magnitude of the oxygen limitation effect on CTmax would differ between adults and larvae, and that this was related to variation in respiratory structure and function. Adult beetles are expected to have greater aerobic scope (i.e., the excess capacity of the respiratory system to deliver oxygen) than larvae, because of more energetically demanding behaviors such as flight and/or mating. Adults are therefore expected to be able to maintain a more consistent respiratory pattern with decreasing oxygen level. Larvae on the other hand are expected to be more hypoxia-tolerant due to their saproxylic or wood-boring nature but to perhaps have limited aerobic scope since their activities do not imply large energy expenditure at this stage (e.g., they lack flight ability). Overall, these differences are expected to be associated with structural differences in the tracheal system (i.e., respiratory anatomy): in conditions of constant energy demand, adults would have a more developed tracheal system (estimated as e.g., total air volume) relative to their body size compared to larvae, indicative of greater potential upper capacity for oxygen flux (supply).
Using a combination of experimental respirometry and 3D-imaging of respiratory anatomy, we investigate functional hypoxia and temperature tolerance in a member of a previously unexamined group of terrestrial arthropods.
Materials and Methods
Experimental Animals
Cacosceles newmannii larvae and adults of both sexes were collected by hand on sugarcane farms in the Entumeni District (28° 55′S; 31°19′E), KwaZulu-Natal, South Africa (Way et al., 2017). Sampling of C. newmannii larvae included digging up sugarcane stalks, by prioritizing the ones looking unhealthy. Specimens were usually found in the base of the stalks, which had to be carefully split open to obtain the larvae. These were brought back to the laboratory at the South African Sugarcane Research Institute (SASRI) in 4 L plastic trays with perforated lids, containing moist peat in order to avoid stress or excessive desiccation. In the laboratory they were placed individually into 3 L plastic bottles with perforated lids, 30% filled with moist autoclaved peat, and a 22 cm long piece of sugarcane, with a 14 mm diameter hole bored 6 cm deep into one end to facilitate the larva’s entrance into the stalk to feed. The piece of sugarcane was replaced with fresh sugarcane every 3 weeks. The old stalk was carefully dissected to remove the feeding larva which was transfered into a new stalk. Larvae were then kept individually at 25°C in a 16L:8D regime, in an environmentally controlled room in the SASRI Insect Unit, before being couriered overnight, singly placed in plastic 250–600 ml jars filled with freshly crushed sugarcane, to the Department of Conservation Ecology and Entomology (ConsEnt), Stellenbosch University, Western Cape Province, South Africa. Adults were found flying (mainly males) or walking (mainly females) in sugarcane fields, mainly in January and February. They were collected by hand and transported individually in 250–600 ml plastic jars with perforated lids to ConsEnt. Shredded paper was placed in the bottle to provide a perching substrate and some protection from excessive movement for the adults, and a damp a piece of cotton to provide water in transit.
Thermolimit Respirometry
Larvae and adults were placed individually in flow-through chambers to perform TLR. All individuals were weighed before and after respirometry on a microbalance (to 0.1 mg; AB104-S/Fact, Mettler Toledo International, Inc., Columbus, OH, United States), and the average value of the two measurements was used for statistical analyses. Thermal limits from metabolic rate data (V̇CO2 CTmax) under normoxia (21 kPa O2) and hypoxia (2.5 kPa O2, balance N2) were assessed following methods adapted from Boardman and Terblanche (2015). Briefly, air flow was regulated at 200 ml min–1 (STPD) [controlled by a mass flow control valve (SideTrak, Sierra International, United States)] into a Li-7000 infra-red CO2/H2O analyzer fully calibrated for CO2 at 386 ppm (balanced nitrogen) and water at 5°C dew point. The standard LiCor software (Li-Cor, Lincoln, NE, United States) recorded the CO2 production differentially (V̇CO2) in ppm. Activity was monitored using an infra-red activity detector (AD-2, Sable Systems International, Las Vegas, NV, United States). Animals were given a 15-min equilibration period at 25°C, after which the temperature in the chamber was ramped up at a rate of 0.06°C min–1 to 55°C using a programmable circulating and heating water bath (CC410wl, Huber, Berching, Germany). Baseline recordings were taken before and after each run to correct for potential drift, which was typically negligible. Sample sizes for each treatment are given in Table 1. Oxygen availability levels were chosen according to what is commonly used in similar work on terrestrial arthropods (e.g., Van Voorhies, 2009; Basson and Terblanche, 2010; Stevens et al., 2010; Owings et al., 2014; Boardman and Terblanche, 2015; Boardman et al., 2015, 2016) and in order to mimic extreme environmental levels (Pincebourde and Casas, 2016) as well as to maximize insects’ responses over the time-scales examined here.
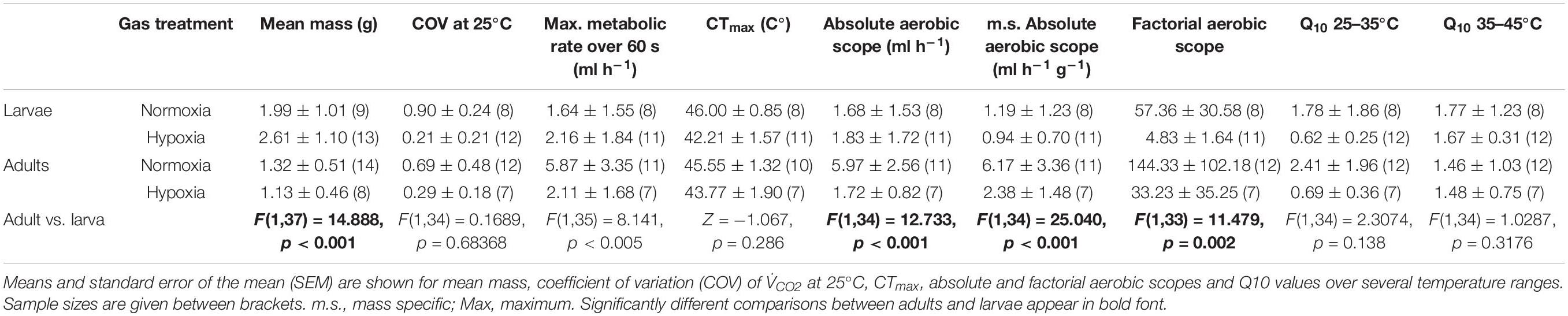
Table 1. Summary data for adults and larvae under hypoxia and normoxia assessed in C. newmannii, and results of statistical comparison between adults and larvae for all variables.
Determination of Respiratory Patterns and Ambient Oxygen Effects
Assuming that discontinuous gas exchange cycles (DGC, i.e., showing a clear prolonged spiracle-closed phase) are undertaken to defend a particular tracheal respiratory pO2 setpoint of 4–5 kPa (as argued for moth pupae in Hetz and Bradley, 2005), we investigated whether respiration patterns (continuous or discontinuous gas exchange cycles) were affected by variation in ambient oxygen availability. For determination of respiratory patterns, the same methodology as described for TLR was used. Five adults and eight larvae were placed sequentially in individual chambers maintained at 25°C. Chambers were then measured for 40 min at each of five randomly assigned oxygen partial pressures (0, 2.5, 5, 8, 10.5 kPa O2, balance N2), starting and ending with a measurement at 20.9 kPa O2 and pattern-type classified according to spiracle behavior (openings and closings). The levels of oxygen partial pressure were chosen in order to target a wide range of hypoxia levels, and are similar to what is commonly found in the insect literature (e.g., Basson and Terblanche, 2010; Owings et al., 2014; Boardman et al., 2016).
Respirometry Data Extraction and Analysis
Expedata (v. 1.9.10, Sable Systems International) was used to transform recorded CO2 values in ppm to V̇CO2 in ml CO2/h, to correct for potential baseline drift for all respirometry files (TLR and respiratory patterns data), and to record activity (body movement) data.
In addition, minimum and maximum metabolic rates over 60 s, and mean CO2 were extracted from the normoxia data of the TLR using Expedata. Effects of life stage and mean mass on these values, as well as on normoxia CTmax (for the specimens used in the TLR experiment only), were computed using ANCOVAs.
Critical temperatures were assessed from the TLR data for normoxia and for hypoxia using Expedata 1.9.10 (Sable Systems International). The coefficient of variation (COV) at 25°C, CTmax (defined as the point at which spiracles switched from high to low variability and indicating that spiracle control was lost, reliably seen as a smooth curve of V̇CO2, Supplementary Figure S1), absolute aerobic scope (i.e., the difference between resting and maximum metabolic rates) and Q10 values over different temperature ranges [as defined by Bělehrádek (1930), the factor by which a reaction rate increases for a 10°C increase in temperature] were computed according to Boardman and Terblanche (2015). Statistical analyses of the TLR data were conducted in Statistica (StatSoft Inc., Tulsa, OK, United States). Normality of data was checked and, when violated, non-parametric tests were used. Larval and adult mass differences between oxygen treatments were analyzed with t-tests, and comparisons between groups were made with ANOVAs or Mann–Whitney U-tests, followed by Tukey post hoc tests.
Expedata 1.9.10 (Sable Systems International) was used to visualize the effect of ambient oxygen on the proportion of individuals showing DGC.
Computation of the Total Internal Air Volume of Respiratory Anatomy of Adults and Larvae
X-ray micro computed tomography (μCT) was performed with scan parameters optimized according to du Plessis et al. (2017). For these measurements, 4 adults (2 males and 2 females) and 4 larvae were selected based on their size, such that a wide spectrum of available morphologies was represented. All samples were scanned with identical settings in order to ensure direct comparison, specifically 120 kV and 220 μA for X-ray generation, magnification was set to achieve a voxel size of 30 μm, and each image was acquired in 131 ms, with averaging and skipping of images to enhance image quality. A total of 2200 step positions were used in one full rotation of the sample.
Images were segmented and analyzed in Volume Graphics VGSTUDIO MAX 3.1 software. The segmentation was achieved using a combination of image morphological operations, with slight modifications depending on the size of the sample. The main challenge was to achieve a closed surface including the whole tracheal system including the connected internal air cavities, while not including exterior air spaces, despite the entire system being open. The final result was manually checked and potential errors were corrected by modifying the segmentation. The segmentation process was manual but started with an automated surface determination function, guiding the selection of void spaces. The final segmented volume was checked manually to ensure no exterior air was included in the segmentation, and only interior air was included. A skin of two voxels all around the outside of the sample was then eroded, to leave only the interior of the sample including material and air. This was used to calculate internal air volumes. These were transformed into scaling relationships of air volume against body mass with fitted linear regression. Data fitted better to the regression without log scaling and therefore statistical testing was done on untransformed data.
For analyzing the scaling relationships between the traits, we used the general equation Y = aMb where Y is the air volume, a is a scaling coefficient, M is the body mass, and b is the scaling value (Huxley, 1932; LaBarbera, 1989). The slopes, i.e., b, were derived by using ordinary least squares regression estimates and then compared to the commonly used theoretical scaling values 1, 0.67, and 0.75 (Chown et al., 2007). When comparing air volume to mass, the value 1 reflects isometry and is often used as a null hypothesis in anatomy scaling studies (LaBarbera, 1989). The value 0.67 is also isometry that reflects surface area to volume geometry (Kozłowski et al., 2003). The value 0.75 reflects isometry under fractal similarity of nutrient supply which also means that an organ grows less than body size (West et al., 1997, 1999). We used slope tests with a t-statistic computed as t = slope1-slope2/SE1 (following e.g., Chown et al., 2007). This approach assumes no variance in the theoretical slope value but employs the estimated slope and standard error of the empirical data (Zar, 1997). However, while scaling patterns of respiratory structures are expected to vary among tracheated arthropod species, they may also be highly variable across and within stages of the same species due to the discontinuous growth of the tracheal system and, in some cases, complex life histories (e.g., occurrence of diapause) (Helm and Davidowitz, 2013; Vogt and Dillon, 2013; Greenlee et al., 2014). Therefore, no specific allometry model or anatomical scaling hypothesis has been adequately developed yet regarding the scaling relationship of the tracheal volume in this study with body size to test, and we instead sought here to statistically compare the estimated scaling slopes (regression exponents) with other scaling values commonly reported in the literature across a wide range of species (Chown et al., 2007) and between the two main life-stages investigated.
Visualizations of segmented volume data were performed using isosurface views in VGSTUDIO MAX. Tracheal volumes were colored according to their local volume, which is equivalent to local diameter, using wall thickness analysis (sphere method, in mm). This function calculated the largest sphere that fitted in any given segmented region, and is usually applied to analysis of objects with varying wall thickness, but in this case the local thickness of the tracheal volumes were colored for visual clarity of large vs. small areas of connected volumes.
Results
Thermolimit Respirometry
Larvae were significantly heavier than adults [F(1,37) = 14.888, p < 0.001, Table 1]. Under normoxia, CTmax was significantly influenced by life-stage and by the interaction between life-stage and mean body mass (Table 2). CTmax increased with mass for adults (R2 = 0.135) but decreased with mass for larvae (R2 = 0.811) (Figure 1). Mass and life stage did not have any significant impact on mean CO2 production nor on minimum or maximum metabolic rates (Table 2). Normoxic metabolism of both life stages of this species was independent of body mass in our data.
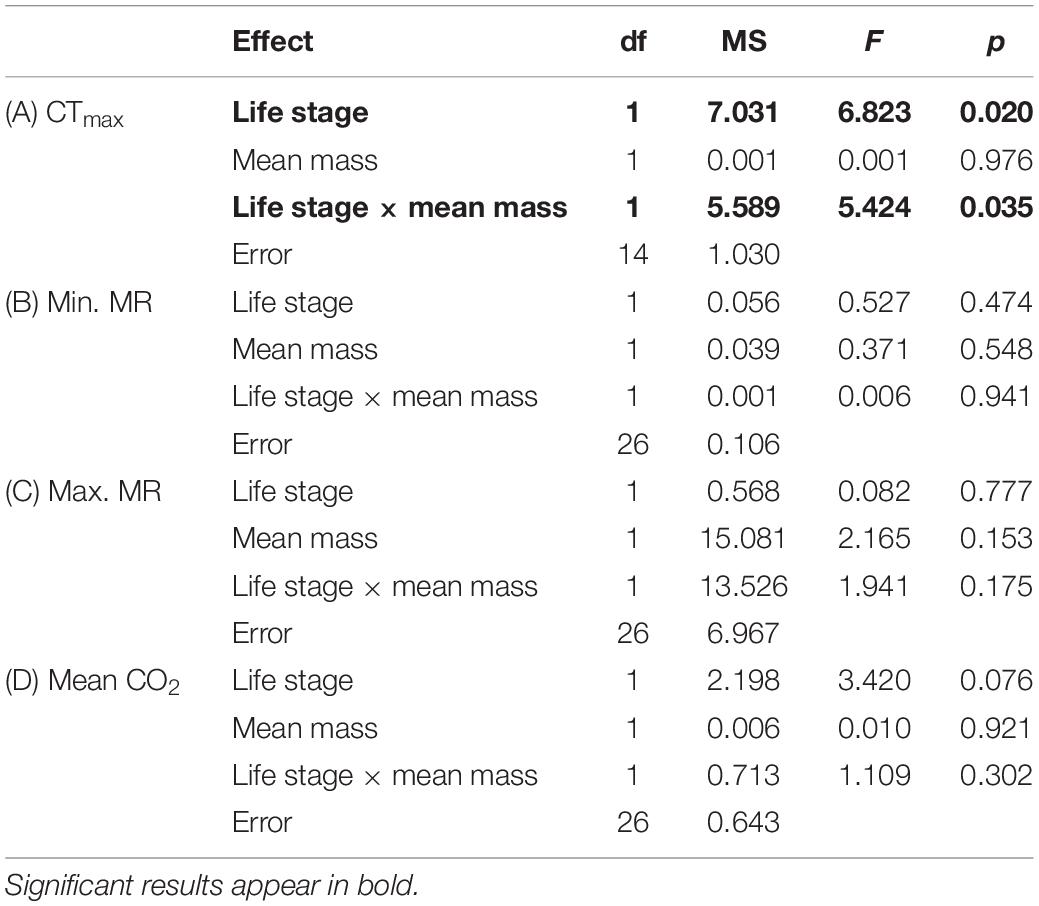
Table 2. Results of factorial ANCOVAs with CTmax (A), minimum metabolic rate (Min MR) (B), maximum metabolic rate (Max MR) (C), and mean CO2 production at 25°C (D) of C. newmannii life stages at normoxia as dependant variables.
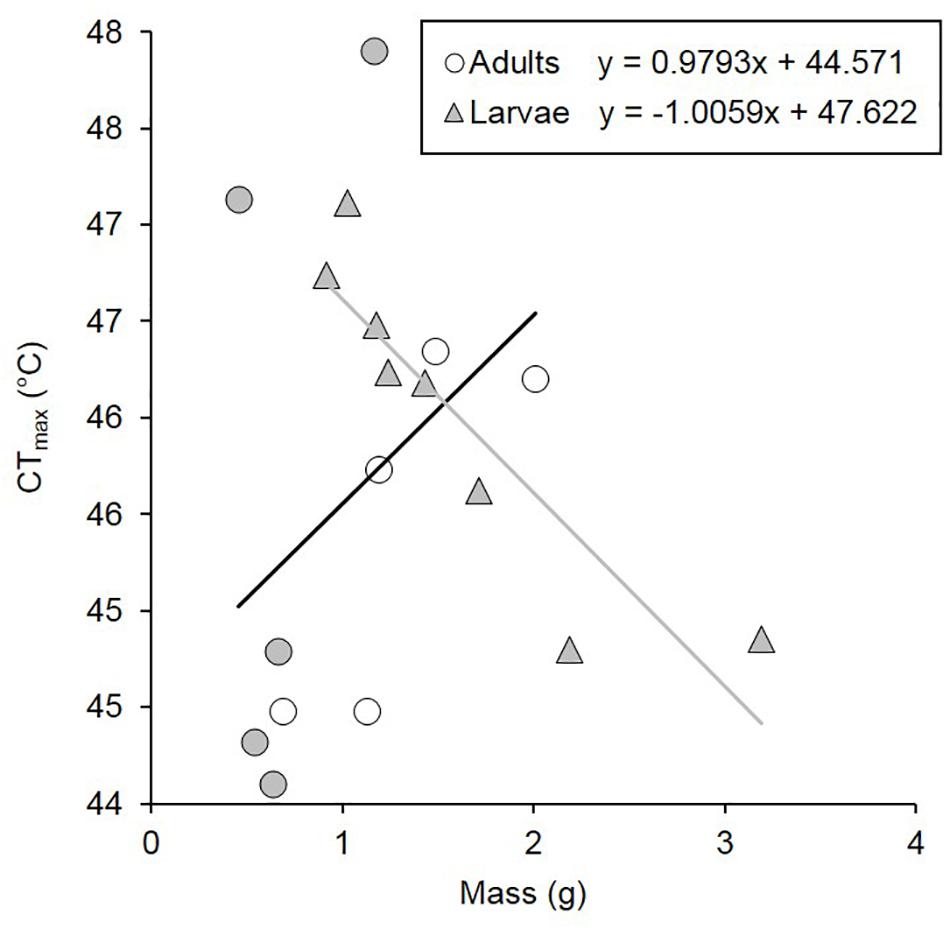
Figure 1. CTmax under normoxia of C. newmannii adults (males: white circles; females: gray circles) and larvae (gray triangles) of different masses. Regression equations are displayed on the figure (for adults, r2 = 0.135; for larvae, r2 = 0.811).
Individuals used for normoxia and hypoxia did not differ in mass (t = −1.26, p = 0.216 and t = 0.610, p = 0.550 for larvae and adults, respectively). Hypoxia lead to a significant decline in thermal tolerance for both larvae and adults: CTmax decreased by 3.8 and 1.8°C, respectively (Table 1).
Variation in Metabolic Rates
Both mass-specific absolute aerobic scope and maximum metabolic rate were significantly higher for adults than for larvae regardless of the gas treatment [F(1,34) = 25.040, p < 0.001 and F(1,34) = 9.302, p = 0.004, respectively, Table 1]. The effect of hypoxia on maximum metabolic rate depended on life-stage: for larvae, hypoxia did not alter the maximum metabolic rate (Tables 1, 3 and Supplementary Figure S2), while in adults hypoxia decreased maximum metabolic rate to only 36% of normoxic levels (Tables 1, 3 and Supplementary Figure S2).
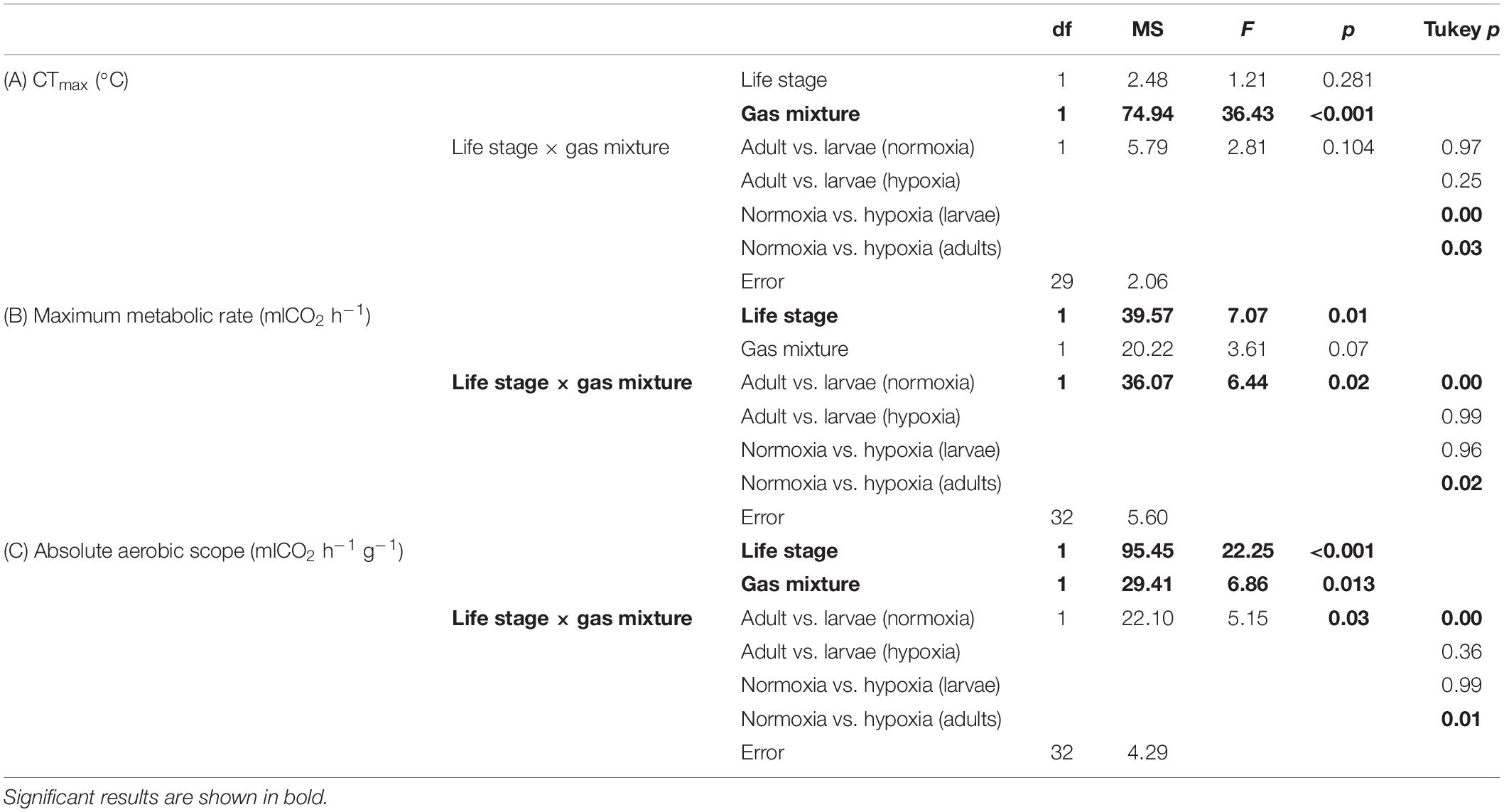
Table 3. Results of factorial ANOVAs with CTmax (A), maximum metabolic rate (B), and mass-specific absolute aerobic scope (C) of C. newmannii life stages as dependent variables, and the associated Tukey post hoc tests.
Respiratory pattern switching was monitored at the individual level as a test of innate hypoxia sensitivity. Both life-stage and O2 partial pressure affected the proportion of individuals displaying DGC (life-stage: χ2 = 8.971, p = 0.003; O2 partial pressure: χ2 = 16.37, p < 0.001). More adults showed DGC than larvae across all O2 partial pressure treatments (Figure 2). Within life-stages, adult DGC proportion increased dramatically above 5 kPa O2. Only two larvae showed DGC, at 10.5 kPa O2 and at normoxia, the rest displayed continuous respiration patterns. At 0 and 2.5 kPa O2, V̇CO2 was generally stable, in both adults and larvae, suggesting steady-state conditions had been achieved.
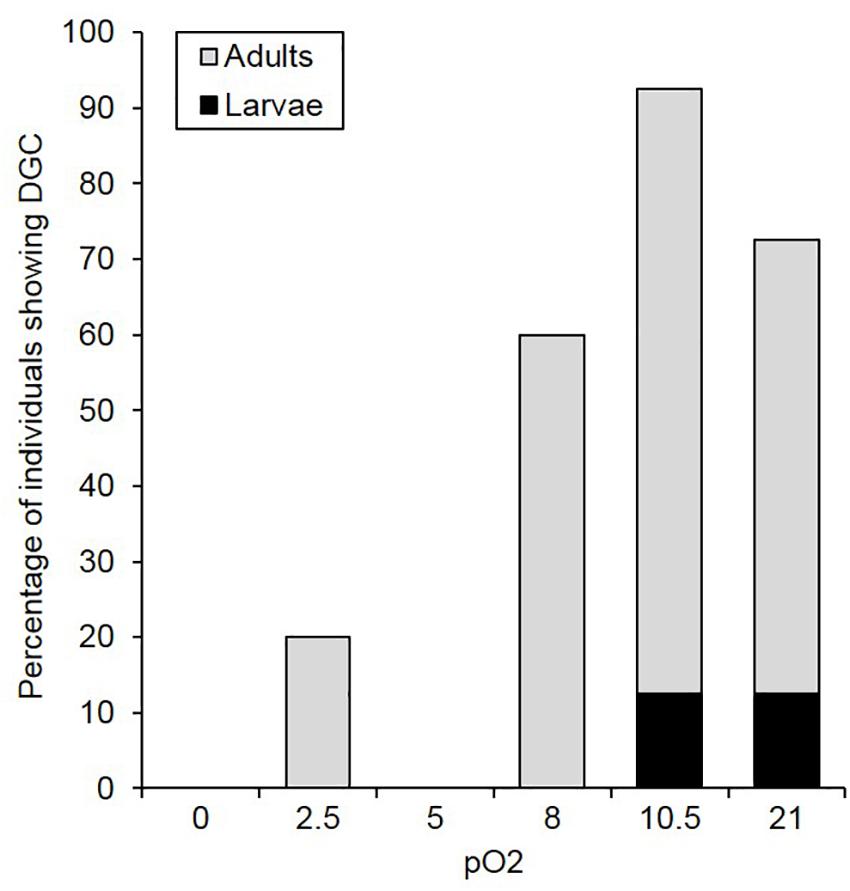
Figure 2. Percentage of C. newmannii individuals showing DGC as a function of oxygen concentration (pO2) for adults (light gray, n = 5) and larvae (black, n = 8).
Respiratory Anatomy
The entire respiratory structure was taken into account down to the minimum voxel resolution achieved in the CT scans. Smaller air spaces than the voxel size might not be included in the analysis, but this is expected to be negligible at the high resolution used here, given the large volume fraction of air space and the clearly visible tracheal systems in the datasets. In addition, the underestimation of the total tracheal volume due to the voxel size used in this study is similar for adults and larvae (Greco et al., 2014; Iwan et al., 2015), therefore allowing comparison of the two life stages.
Total internal air volume was greater in adults than in larvae (t = 5.859, p = 0.009; Figures 3, 4). The internal air volume of adults increased with body mass (R2 = 0.791), while larval air volume remained constant even though body mass increased over threefold (R2 = 0.021) (Figure 4). However, even if the life-cycle and duration of each larval stage is not completely described yet for C. newmannii, individuals in this mass range are believed to belong to the same instar, according to preliminary observations. Neither larvae nor adults showed isometric growth (LaBarbera, 1989) between mass and respiratory structure air volume (t = 43.079, p < 0.001 and t = 2.456, p < 0.05, respectively), in both cases the scaling showed a negative allometry. We could not distinguish between scaling of 0.67 and 0.75 statistically for adults (t = 0.739, p > 0.25 and t = 1.155, p > 0.05, respectively). Interestingly, adults of both sexes showed a relatively large air sac in their abdomen, which was connected to the rest of the tracheal system (Figures 3A,C and Supplementary Figure S3).
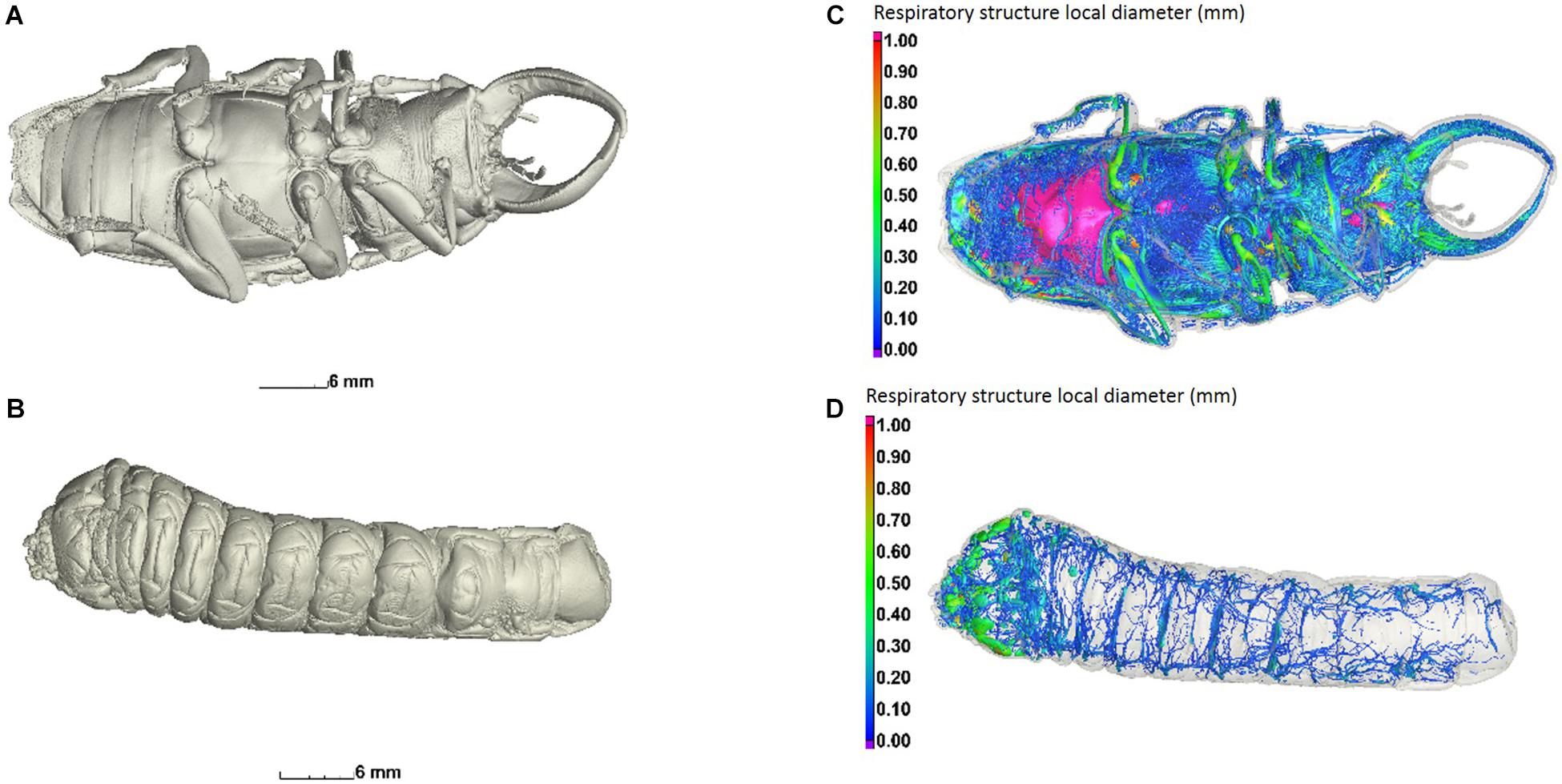
Figure 3. Central view of an adult male (A) and a larva (B) of C. newmannii. Ventral view of the tracheal structure of adult (C) and larva (D). Air-filled structures are colored according to their local diameter, using wall thickness analysis (sphere method), and scaled as shown on the left. The wall thickness measures the size of the largest sphere that fits in every location inside the structure.
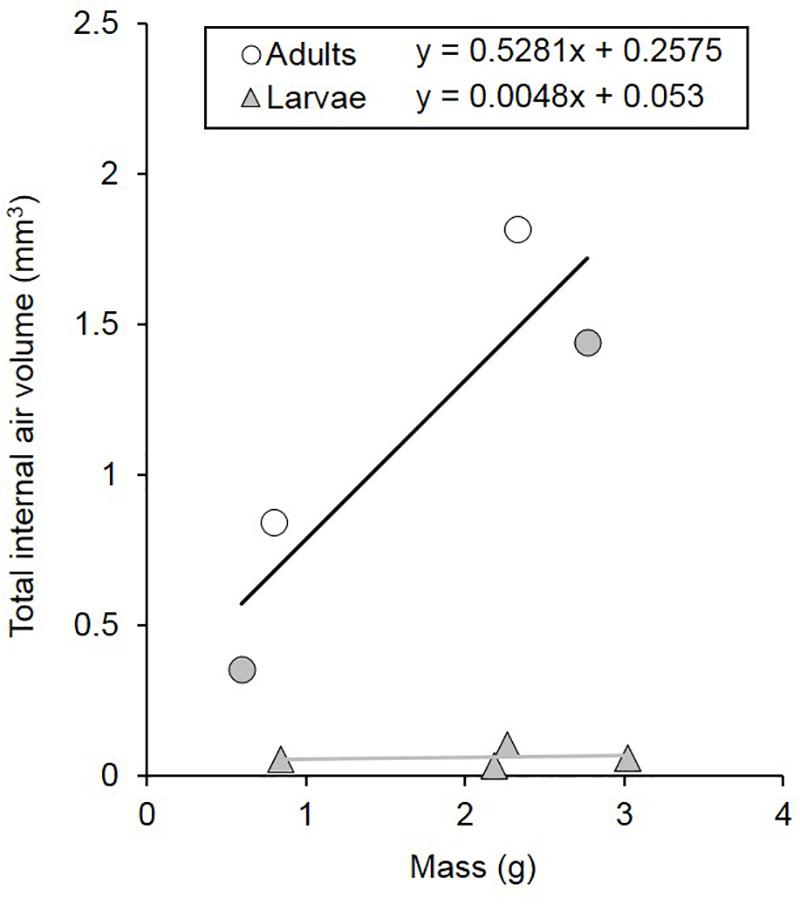
Figure 4. Tracheal volumes of C. newmannii adults (males: white circles, n = 2; females: gray circles, n = 2) and larvae (gray triangles, n = 4) of different masses. Regression equations are displayed on the figure (for adults, r2 = 0.791; for larvae, r2 = 0.021).
Discussion
In this study, we assessed the tolerance to different environmental conditions (oxygen and temperature) of a previously unreported cerambycid pest species, representing a taxonomic family which is often considered to be hypoxia-adapted over evolutionary timescales (Chappell and Rogowitz, 2000; Pincebourde and Casas, 2016). While many aspects of the specific biology of C. newmannii remain unknown (Way et al., 2017), here we found that the upper thermal tolerance (CTmax) of this species is broadly consistent with estimates of CTmax in other Coleoptera from diverse families (Klok et al., 2004; Vorhees and Bradley, 2012; García-Robledo et al., 2016; Chidawanyika et al., 2017). C. newmannii larvae, it is presumed, typically live inside wood, a microenvironment that is assumed to be hypoxic and/or hypercapnic relative to the ambient atmosphere (Hoback and Stanley, 2001; Pincebourde and Casas, 2016). Regardless, it is frequently proposed that unlike adults, cerambycid larvae routinely experience hypoxia stress, as well as thermal constraints, given that behavioral thermoregulation is limited within plant tissues (Chappell and Rogowitz, 2000; Pincebourde and Casas, 2016; Javal et al., 2018). This microenvironment may therefore have contributed to evolved variation in larvae upper thermal limits. However, CTmax was not significantly different between larvae and adults in the present study, unlike a previous comparison of life-stages in a Lepidoptera species (Boardman and Terblanche, 2015).
One mechanistic theory proposed to explain variation in heat tolerance in ectotherms under hypoxic conditions is the OCLTT hypothesis. This hypothesis argues that insufficient capacity to supply oxygen to meet the oxygen demand of tissues can cause a progressive decline in animal performance as temperatures deviate from the optimum temperature (Giomi et al., 2014). For the OCLTT hypothesis to be supported, at least two key predictions can be made (reviewed in Verberk et al., 2016b). First, there should be a reduction in thermal performance, which is linked mechanistically with variation in thermal tolerance, when oxygen supply is reduced, and second, a decrease in the aerobic scope is expected when temperature approaches the critical limit (Verberk et al., 2016b). Even though many aquatic species conform to one or several predictions of the OCLTT hypothesis (Pörtner, 2010), the results for terrestrial insects remain equivocal and controversial (Verberk et al., 2016b). In our study, however, a reduction in CTmax was observed in both adults and larvae, although to different extents between the life-stages, when ambient oxygen supply was experimentally reduced, giving some support to the OCLTT hypothesis for a terrestrial arthropod.
If adults and larvae do not differ in terms of thermal tolerance, they do differ in several notable behaviors, as typically expected of holometabolous insect life-cycles, which in turn affects the way they can modulate their metabolism depending on their environmental constraints. Indeed, adults engage routinely in energy-costly behaviors such as fighting, flying or mating and were therefore expected to show a higher aerobic scope than larvae (Niven and Scharlemann, 2005). Larvae appeared to be more constrained in their aerobic scope than adults, but they were better able to sustain their maximum metabolic rate under hypoxic conditions. This could be related to the respiratory patterns that both life-stages can show. According to the metabolic rate hypothesis (Contreras and Bradley, 2009, 2010), insects with low metabolic rate will be more likely to show DGC than cyclic respiratory patterns. Since low metabolism is generally associated with low oxygen consumption (and CO2 production), spiracles can stay closed for longer without compromising the insect’s ability to meet its cellular metabolic demands. One can therefore assume that, as O2 partial pressure decreases, spiracles must remain open for a larger proportion of the time in order to meet a constant oxygen demand. This most likely explains why larvae maintain their metabolic rate at hypoxia but show a much lower proportion of DGC compared to adults, that are more likely to reduce their maximum metabolic rate to cope with hypoxic conditions. Indeed, hypoxia reduced maximum metabolic rate in adults to a greater degree than it did in larvae, thus reducing adults’ aerobic scope. A similar pattern was observed in two Heteroptera species where juveniles and adults did not differ in type of respiration and consequently did not differ in either CTmax nor the extent to which CTmax was influenced by oxygen levels (Verberk and Bilton, 2015).
The differences between life-stages observed in the present study could be due to ontogenetic variation or changes in respiratory structure-function relationships. Major remodeling or change in these relationships, within and across taxa, should produce predictable variation in the impacts of oxygen limitation on thermal tolerance. Depending on the type of metabolic theory and its mechanistic underpinnings (see e.g., Chown et al., 2007; Snelling et al., 2011a, b; Maino and Kearney, 2014; Harrison, 2018; Harrison et al., 2018) there may be considerable variation predicted in how the respiratory structure scales with body size. This scaling, in turn, is expected to have functional consequences for respiratory and athletic performance of a species (White et al., 2008; Snelling et al., 2011a, b). Differences among life-stages in breathing patterns and modulation thereof were expected to result from structural differences in the tracheal system. Indeed, in other insect species investigated to date (mainly Lepidoptera), the larval internal air volume stays constant within an instar, with increasing larval mass (Callier and Nijhout, 2011; Harrison, 2018). This unchanging internal air volume probably leads to an increasing mismatch between their resting metabolic rate and oxygen supply as the larvae grow, before molting (see e.g., Greenlee and Harrison, 2005 for Lepidopteran larvae, but see Kivelä et al., 2016, 2019), as suggested by the negative correlations between CTmax and body mass we observed. Adults, on the other hand, appear to have a larger tracheal system that increases with body mass and CTmax. This result is unusual and perhaps noteworthy in air-breathing insects. One potential explanation for this outcome is that the respiratory system has most likely evolved for high oxygen demand activities (such as flight), and is therefore dramatically oversized for demands during resting periods (see discussions in Snelling et al., 2011a, b). Indeed, the presence of an air sac in the adults’ abdomen further supports such an assertion, even though flight muscles might also help in convection of air and the insect may still have limited capacity when it does not use these muscles (Komai, 2001). Such air sacs have been described for many other insect species (although typically in Orthoptera) in which they are intermittently compressed by contracted muscles during flight to create enhanced oxygen delivery to flight muscles (Wasserthal et al., 2018; reviewed in Chapman, 2012). In addition, since the respiratory pattern used by an insect can reflect its oxygen delivery capacity (Respiratory adequacy hypothesis; Contreras and Bradley, 2010), the enlarged oxygen delivery capacity of the adult tracheal system is a likely explanation for why adults are able to express DGC even under relatively low experimental O2 partial pressures.
In agreement with other intra-specific studies on insects (e.g., Lease et al., 2012; Harrison et al., 2014), our results imply that metabolic rate is size-independent for C. newmannii, at least in the uncontrolled, although mainly resting, state. Several biological explanations have been reviewed by Harrison et al. (2014) but none can be formally supported on the basis of our data. Beside biological hypotheses, this result can be attributed to the experimental respirometry protocol which did not seek to restrict or prevent activity of individuals; most animals were active during the equilibration period of the TLR trials. The absence of a relationship between normoxic metabolism and body mass could also be due to the relatively small sample size that was available, although this is likely offset by the large change in mass among specimens included (>fourfold difference in mass between the smallest and the largest specimens used, for both adults and larvae). However, detailed comparison of scaling relationships and the underlying mechanistic basis thereof was beyond the scope of this present study and would require far larger sample sizes before robust interpretations could be made.
To conclude, our study shows that marked divergence in terms of aerobic scope and respiratory patterns between life-stages were most likely due to both structural and functional differences, but these may be coupled with, or have co-evolved with, life-stage specific behavioral and physiological adaptations. Our data thus show that thermal limits, and any hypoxia effects thereon, are more predictable if respiratory structure-function relationships are fully characterized.
Data Availability Statement
The datasets generated for this study can be found here: Thermolimit respirometry: 10.6084/m9.figshare.8263070, Internal air volume: 10.6084/m9.figshare.7392188.
Author Contributions
JT conceived the project. DC co-ordinated the adult and larval field collection, laboratory preparation, and shipment. DC and JT secured the funding. ST, MB, and AD performed the experiments. MJ and PL ran analyses. MJ, PL, and JT co-wrote the manuscript. All authors revised and contributed to the final version of the manuscript.
Funding
This research was supported by the Centre for Invasion Biology and the South African Sugarcane Research Institute.
Conflict of Interest
The authors declare that the research was conducted in the absence of any commercial or financial relationships that could be construed as a potential conflict of interest.
Acknowledgments
We thank C. Smit for laboratory assistance and S. le Roux for assistance at the CT scanner facility. We thank the Eshowe Biosecurity team, the late M. Way and B. Gcuma for collecting the specimens, and D. Gillespie and N. Muthusamy for rearing the field collected larvae. We also thank Art Woods, Wilco Verberk, and the referees for constructive comments on an earlier version of this manuscript.
Supplementary Material
The Supplementary Material for this article can be found online at: https://www.frontiersin.org/articles/10.3389/fphys.2019.01426/full#supplementary-material
Supplementary Figure S3 can be found in FigShare at doi: 10.6084/m9.figshare.8397221
FIGURE S1 | Example of thermolimit respirometry trace of a C. newmannii adult (A,B) and a larva (C,D) with carbon dioxide production (blue), temperature (red), and activity (green), under normoxia (A,C) and hypoxia (B,D). The blue dotted arrows indicate CTmax, which correspond to loss of spiracular control.
FIGURE S2 | Mean plot of CTmax (A), and maximum metabolism (B), for C. newmannii larvae (blue) and adults (green). Asterisks show significant differences among life stages.
FIGURE S3 | Video illustrating the tracheal structures of a male adult C. newmannii. Air-filled structures are first colored according to their local volume, using wall thickness analysis (sphere method), and scaled as shown on the left, and then shown in black and white.
References
Angilletta, M. J. J. (2009). Thermal Adaptation: A Theoretical and Empirical Synthesis. Oxford: Oxford University Press.
Bale, J. S., Masters, G. J., Hodkinson, I. D., Awmack, C., Bezemer, T. M., Brown, V. K., et al. (2002). Herbivory in global climate change research: direct effects of rising temperature on insect herbivores. Glob. Change Biol. 8, 1–16. doi: 10.1046/j.1365-2486.2002.00451.x
Basson, C. H., and Terblanche, J. S. (2010). Metabolic responses of Glossina pallidipes (Diptera: Glossinidae) puparia exposed to oxygen and temperature variation: implications for population dynamics and subterranean life. J. Insect Physiol. 56, 1789–1797. doi: 10.1016/j.jinsphys.2010.07.010
Battisti, A., and Larsson, S. (2015). Climate Change and Insect Pest Distribution Range. Climate Change and Insect Pests. Wallingford: CABI, 1–15.
Bělehrádek, J. (1930). Temperature coefficients in biology. Biol. Rev. 5, 30–58. doi: 10.1111/j.1469-185x.1930.tb00892.x
Boardman, L., Mitchell, K. A., Terblanche, J. S., and Sørensen, J. G. (2018). A transcriptomics assessment of oxygen-temperature interactions reveals novel candidate genes underlying variation in thermal tolerance and survival. J. Insect Physiol. 106, 179–188. doi: 10.1016/j.jinsphys.2017.10.005
Boardman, L., Sørensen, J. G., Koštál, V., Šimek, P., and Terblanche, J. S. (2016). Cold tolerance is unaffected by oxygen availability despite changes in anaerobic metabolism. Sci. Rep. 6:32856. doi: 10.1038/srep32856
Boardman, L., Sørensen, J. G., and Terblanche, J. S. (2015). Physiological and molecular mechanisms associated with cross tolerance between hypoxia and low temperature in Thaumatotibia leucotreta. J. Insect Physiol. 82, 75–84. doi: 10.1016/j.jinsphys.2015.09.001
Boardman, L., and Terblanche, J. S. (2015). Oxygen safety margins set thermal limits in an insect model system. J. Exp. Biol. 218, 1677–1685. doi: 10.1242/jeb.120261
Bowler, K., and Terblanche, J. S. (2008). Insect thermal tolerance: what is the role of ontogeny, ageing, and senescence? Biol. Rev. 83, 339–355. doi: 10.1111/j.1469-185x.2008.00046.x
Bubliy, O. A., Kristensen, T. N., Kellermann, V., and Loeschcke, V. (2012). Humidity affects genetic architecture of heat resistance in Drosophila melanogaster. J. Evol. Biol. 25, 1180–1188. doi: 10.1111/j.1420-9101.2012.02506.x
Callier, V., and Nijhout, H. F. (2011). Control of body size by oxygen supply reveals size-dependent and size-independent mechanisms of molting and metamorphosis. Proc. Natl. Acad. Sci. U.S.A. 108, 14664–14669. doi: 10.1073/pnas.1106556108
Chapman, R. F. (2012). The Insects: Structure and Function, 5th Edn. Cambridge: Cambridge University Press.
Chappell, M., and Rogowitz, G. L. (2000). Mass, temperature and metabolic effects on discontinuous gas exchange cycles in Eucalyptus-boring beetles (Coleoptera: Cerambycidae). J. Exp. Biol. 203, 3809–3820.
Chidawanyika, F., Nyamukondiwa, C., Strathie, L., and Fischer, K. (2017). Effects of thermal regimes, starvation and age on heat tolerance of the Parthenium beetle Zygogramma bicolorata (Coleoptera: Chrysomelidae) following dynamic and static protocols. PLoS One 12:e0169371. doi: 10.1371/journal.pone.0169371
Chown, S. L., Marais, E., Terblanche, J. S., Klok, C. J., Lighton, J. R., and Blackburn, T. M. (2007). Scaling of insect metabolic rate is inconsistent with the nutrient supply network model. Funct. Ecol. 21, 282–290. doi: 10.1111/j.1365-2435.2007.01245.x
Clark, T. D., Sandblom, E., and Jutfelt, F. (2013). Response to farell and to pörtner and giomi. J. Exp. Biol. 216, 4495–4497.
Colinet, H., and Boivin, G. (2011). Insect parasitoids cold storage: a comprehensive review of factors of variability and consequences. Biol. Control 58, 83–95. doi: 10.1016/j.biocontrol.2011.04.014
Colinet, H., and Hance, T. (2009). Male reproductive potential of Aphidius colemani (Hymenoptera: Aphidiinae) exposed to constant or fluctuating thermal regimens. Environ. Entomol. 38, 242–249. doi: 10.1603/022.038.0130
Contreras, H. L., and Bradley, T. J. (2009). Metabolic rate controls respiratory pattern in insects. J. Exp. Biol. 212, 424–428. doi: 10.1242/jeb.024091
Contreras, H. L., and Bradley, T. J. (2010). Transitions in insect respiratory patterns are controlled by changes in metabolic rate. J. Insect Physiol. 56, 522–528. doi: 10.1016/j.jinsphys.2009.05.018
du Plessis, A., Broeckhoven, C., Guelpa, A., and le Roux, S. G. (2017). Laboratory x-ray micro-computed tomography: a user guideline for biological samples. Gigascience 6, 1–11. doi: 10.1093/gigascience/gix027
Fischer, K., Liniek, S., Bauer, M., Baumann, B., Richter, S., and Dierks, A. (2012). Phenotypic plasticity in temperature stress resistance is triggered by photoperiod in a fly. Evol. Ecol. 26, 1067–1083. doi: 10.1007/s10682-011-9547-x
García-Robledo, C., Kuprewicz, E. K., Staines, C. L., Erwin, T. L., and Kress, W. J. (2016). Limited tolerance by insects to high temperatures across tropical elevational gradients and the implications of global warming for extinction. Proc. Natl. Acad. Sci. U.S.A. 113, 680–685. doi: 10.1073/pnas.1507681113
Giomi, F., Fusi, M., Barausse, A., Mostert, B., Pörtner, H. O., and Cannicci, S. (2014). Improved heat tolerance in air drives the recurrent evolution of air- breathing. Proc. Biol. Sci. 281:20132927. doi: 10.1098/rspb.2013.2927
Greco, M., Bell, D., Woolnough, L., Laycock, S., Corps, N., Mortimore, D., et al. (2014). 3-D visualisation, printing, and volume determination ofthe tracheal respiratory system in the adult desert locust, Schistocerca gregaria. Entomol. Exp. Appl. 152, 42–51. doi: 10.1111/eea.12199
Greenlee, K. J., and Harrison, J. F. (2005). Respiratory changes throughout ontogeny in the tobacco hornworm caterpillar, Manduca sexta. J. Exp. Biol. 208, 1385–1392. doi: 10.1242/jeb.01521
Greenlee, K. J., Montooth, K. L., and Helm, B. R. (2014). Predicting performance and plasticity in the development of respiratory structures and metabolic systems. Integr. Comp. Biol. 54, 307–322. doi: 10.1093/icb/icu018
Harrison, J. F. (2018). Approaches for testing hypotheses for the hypometric scaling of aerobic metabolic rate in animals. Am. J. Physiol. Reg. I. 315, 879–894. doi: 10.1152/ajpregu.00165.2018
Harrison, J. F., Greenlee, K., and Verberk, W. C. E. P. (2018). Functional hypoxia in insects: definition, assessment, and consequences for physiology, ecology and evolution. Annu. Rev. Entomol. 63, 303–325. doi: 10.1146/annurev-ento-020117-043145
Harrison, J. F., Klok, C. J., and Waters, J. S. (2014). Critical PO2 is size-independent in insects: implications for the metabolic theory of ecology. Curr. Opin. Insect Sci. 4, 54–59. doi: 10.1016/j.cois.2014.08.012
Helm, B. R., and Davidowitz, G. (2013). Mass and volume growth of an insect tracheal system within a single instar. J. Exp. Biol. 216, 4703–4711. doi: 10.1242/jeb.080648
Hetz, S. K., and Bradley, T. J. (2005). Insects breathe discontinuously to avoid oxygen toxicity. Nature 433, 516–519. doi: 10.1038/nature03106
Hoback, W. W., and Stanley, D. W. (2001). Insects in hypoxia. J. Insect Physiol. 47, 533–542. doi: 10.1016/s0022-1910(00)00153-0
Hoffmann, A. A., Shirriffs, J., and Scott, M. (2005). Relative importance of plastic vs genetic factors in adaptive differentiation: geographical variation for stress resistance in Drosophila melanogaster from eastern Australia. Funct. Ecol. 19, 222–227. doi: 10.1111/j.1365-2435.2005.00959.x
Huey, R. B., Kearney, M. R., Krockenberger, A., Holtum, J. A. M., Jess, M., and Williams, S. E. (2012). Predicting organismal vulnerability to climate warming: roles of behaviour, physiology and adaptation. Philos. Trans. R. Soc. Lond B Biol. Sci. 367, 1665–1679. doi: 10.1098/rstb.2012.0005
Iwan, D., Kamiński, M. J., and Raś, M. (2015). The last breath: μCT-based method for investigating the tracheal system in Hexapoda. Arthropod. Struct. Dev. 44, 218–227. doi: 10.1016/j.asd.2015.02.002
Javal, M., Renault, D., and Colinet, H. (2016). Impact of fluctuating thermal regime on Drosophila melanogaster survival to cold stress. Anim. Biol. 66, 427–444. doi: 10.1163/15707563-00002510
Javal, M., Roques, A., Roux, G., and Laparie, M. (2018). Respiration-based monitoring of metabolic rate following cold exposure in two invasive Anoplophora species depending on acclimation regime. Comp. Biochem. Phys. A 216, 20–27. doi: 10.1016/j.cbpa.2017.10.031
Jutfelt, F., Gräns, A., Jönsson, E., Wiklander, K., Seth, H., Olsson, C., et al. (2014). Response to ‘How and how not to investigate the oxygen and capacity limitation of thermal tolerance (OCLTT) and aerobic scope – remarks on the article by Gräns et al. J. Exp. Biol. 217, 4433–4435. doi: 10.1242/jeb.115410
Jutfelt, F., Norin, T., Ern, R., Overgaard, J., Wang, T., McKenzie, D. J., et al. (2018). Oxygen- and capacity-limited thermal tolerance: blurring ecology and physiology. J. Exp. Biol. 221:jeb169615.
Kelty, J. D., and Lee, R. E. (1999). Induction of rapid cold hardening by cooling at ecologically relevant rates in Drosophila melanogaster. J. Insect Physiol. 45, 719–726. doi: 10.1016/s0022-1910(99)00040-2
Kim, Y., and Song, W. (2000). Effect of thermoperiod and photoperiod on cold tolerance of Spodoptera exigua (Lepidoptera: Noctuidae). Environ. Entomol. 29, 868–873. doi: 10.1603/0046-225x-29.5.868
Kivelä, S., Gotthard, K., and Lehmann, P. (2019). Developmental plasticity in metabolism but not in energy reserve accumulation in a seasonally polyphenic butterfly. J. Exp. Biol. 222(Pt 13):jeb202150.
Kivelä, S., Lehmann, P., and Gotthard, K. (2016). Does oxygen supply constrain metabolism of larval insects before moulting: an empirical test at the individual level. J. Exp. Biol. 219, 3061–3071. doi: 10.1242/jeb.140442
Klok, C. J., Sinclair, B. J., and Chown, S. L. (2004). Upper thermal tolerance and oxygen limitation in terrestrial arthropods. J. Exp. Biol. 207, 2361–2370. doi: 10.1242/jeb.01023
Komai, Y. (2001). Direct measurement of oxygen partial pressure in a flying bumblebee. J. Exp. Biol. 204, 2999–3007.
Koštál, V., Korbelová, J., Rozsypal, J., Zahradníčková, H., Cimlová, J., Tomčala, A., et al. (2011). Long-term cold acclimation extends survival time at 0°C and modifies the metabolomic profiles of the larvae of the fruit fly Drosophila melanogaster. PLoS One 6:e25025. doi: 10.1371/journal.pone.0025025
Kovacevic, A., Latombe, G., and Chown, S. L. (2019). Rate dynamics of ectotherm responses to thermal stress. Proc. Biol. Sci. 286:20190174. doi: 10.1098/rspb.2019.0174
Kozłowski, J., Konarzewski, M., and Gawelczyk, A. T. (2003). Cell size as a link between noncoding DNA and metabolic rate scaling. Proc. Natl. Acad. Sci. U.S.A. 100, 14080–14085. doi: 10.1073/pnas.2334605100
LaBarbera, M. (1989). Analyzing body size as a factor in ecology and evolution. Annu. Rev. Ecol. Syst. 20, 97–117. doi: 10.1002/ece3.3899
Lease, H. M., Klok, C. J., Kaiser, A., and Harrison, J. F. (2012). Body size is not critical for critical P-O2 in scarabaeid and tenebrionid beetles. J. Exp. Biol. 215, 2524–2533. doi: 10.1242/jeb.057141
Lehmann, P., Ammunét, T., Barton, M., Battisti, B., Eigenbrode, S. D., Jepsen, J. U., et al. (2019a). Complex responses of global insect pests to climate change. Front. Ecol. Environ. [Preprint]. doi: 10.1101/425488
Lehmann, P., Javal, M., and Terblanche, J. S. (2019b). Oxygen limitation is not the cause of death during lethal high thermal exposure in an insect. Biol. Lett. 15:20180701. doi: 10.1098/rsbl.2018.0701
Lighton, J. R. B., and Turner, R. J. (2004). Thermolimit respirometry: an objective assessment of critical thermal maxima in two sympatric desert harvester ants, Pogonomyrmex rugosus and P. californicus. J. Exp. Biol. 207, 1903–1913. doi: 10.1242/jeb.00970
Maino, J., and Kearney, M. R. (2014). Ontogenetic and interspecific metabolic scaling in insects. Am. Nat. 184, 695–701. doi: 10.1086/678401
Marshall, K. E., and Sinclair, B. J. (2010). Repeated stress exposure results in a survival-reproduction trade-off in Drosophila melanogaster. Proc. Biol. Sci. 277, 963–969. doi: 10.1098/rspb.2009.1807
Mitchell, K. A., Boardman, L., Clusella-Trullas, S., and Terblanche, J. S. (2017). Effects of nutrient and water restriction on thermal tolerance: a test of mechanisms and hypotheses. Comp. Biochem. Phys. A 212, 15–23. doi: 10.1016/j.cbpa.2017.06.019
Niven, J. E., and Scharlemann, J. P. W. (2005). Do insect metabolic rates at rest and during flight scale with body mass? Biol. Lett. 1, 346–349. doi: 10.1098/rsbl.2005.0311
Nyamukondiwa, C., and Terblanche, J. S. (2009). Thermal tolerance in adult Mediterranean and Natal fruit flies (Ceratitis capitata and Ceratitis rosa): effects of age, gender and feeding status. J. Therm. Biol. 34, 406–414. doi: 10.1016/j.jtherbio.2009.09.002
Owings, A. A., Yocum, G. D., Rinehart, J. P., Kemp, W. P., and Greenlee, K. J. (2014). Changes in respiratory structure and function during post-diapause development in the alfalfa leafcutting bee, Megachile rotundata. J. Insect Physiol. 66, 20–27. doi: 10.1016/j.jinsphys.2014.05.003
Pincebourde, S., and Casas, J. (2016). Hypoxia and hypercarbia in endophagous insects: larval position in the plant gas exchange network is key. J. Insect Physiol. 84, 137–153. doi: 10.1016/j.jinsphys.2015.07.006
Pörtner, H. O. (2010). Oxygen-and capacity-limitation of thermal tolerance: a matrix for integrating climate-related stressor effects in marine ecosystems. J. Exp. Biol. 213, 881–893. doi: 10.1242/jeb.037523
Pörtner, H. O. (2014). How and how not to investigate the oxygen and capacity limitation of thermal tolerance (OCLTT) and aerobic scope – remarks on the article by Gräns, et al. J. Exp. Biol. 217, 4432–4433. doi: 10.1242/jeb.114181
Rosenzweig, C., Karoly, D., Vicarelli, M., Neofotis, P., Wu, Q., Casassa, G., et al. (2008). Attributing physical and biological impacts to anthropogenic climate change. Nature 453, 353–358. doi: 10.1038/nature06937
Sejerkilde, M., Sørensen, J. G., and Loeschcke, V. (2003). Effects of cold- and heat hardening on thermal resistance in Drosophila melanogaster. J. Insect Physiol. 49, 719–726. doi: 10.1016/s0022-1910(03)00095-7
Snelling, E., Seymour, R., Matthews, P. G. D., Runciman, S., and White, C. R. (2011a). Scaling of resting and maximum hopping metabolic rate throughout the life cycle of the locust Locusta migratoria. J. Exp. Biol. 214, 3218–3224. doi: 10.1242/jeb.058420
Snelling, E., Seymour, R., Runciman, S., Matthews, P. G. D., and White, C. R. (2011b). Symmorphosis and the insect respiratory system: allometric variation. J. Exp. Biol. 214, 3225–3237. doi: 10.1242/jeb.058438
Stevens, M. M., Jackson, S., Bester, S. A., Terblanche, J. S., and Chown, S. L. (2010). Oxygen limitation and thermal tolerance in two terrestrial arthropod species. J. Exp. Biol. 213, 2209–2218. doi: 10.1242/jeb.040170
Terblanche, J. S., Hoffmann, A. A., Mitchell, K. A., Rako, L., le Roux, P. C., and Chown, S. L. (2011). Ecologically relevant measures of tolerance to potentially lethal temperatures. J. Exp. Biol. 214, 3713–3725. doi: 10.1242/jeb.061283
Terblanche, J. S., and Woods, H. A. (2018). Why do models of insect respiratory patterns fail? J. Exp. Biol. 221:jeb130039. doi: 10.1242/jeb.130039
Van Voorhies, W. A. (2009). Metabolic function in Drosophila melanogaster in response to hypoxia and pure oxygen. J. Exp. Biol. 212, 3132–3141. doi: 10.1242/jeb.031179
Verberk, W. C. E. P., and Bilton, D. T. (2015). Oxygen limited thermal tolerance is seen in a plastron breathing insect and can be induced in a bimodal gas exchanger. J. Exp. Biol. 218, 2083–2088. doi: 10.1242/jeb.119560
Verberk, W. C. E. P., Bartolini, F., Marshall, D. J., Pörtner, H.-O., Terblanche, J. S., White, C. R., et al. (2016a). Can respiratory physiology predict thermal niches? Ann. N.Y. Acad. Sci. 1365, 73–88. doi: 10.1111/nyas.12876
Verberk, W. C. E. P., Overgaard, J., Ern, R., Bayley, M., Wang, T., Boardman, L., et al. (2016b). Does oxygen limit thermal tolerance in arthropods? A critical review of current evidence. Comp. Biochem. Physiol. A 192, 64–78. doi: 10.1016/j.cbpa.2015.10.020
Vogt, J. R., and Dillon, M. E. (2013). Allometric scaling of tracheal morphology among bumblebee sisters (Apidae: Bombus): compensation for oxygen limitation at large body sizes? Physiol. Biochem. Zool. 86, 576–587. doi: 10.1086/672211
Vorhees, A. S., and Bradley, T. J. (2012). Differences in critical thermal maxima and mortality across life stages of the mealworm beetle Tenebrio molitor. J. Exp. Biol. 215, 2319–2326. doi: 10.1242/jeb.070342
Wasserthal, L. T., Cloetens, P., Fink, R. H., and Wasserthal, L. K. (2018). X-ray computed tomography study of the flight-adapted tracheal system in the blowfly Calliphora vicina, analysing the ventilation mechanism and flow-directing valves. J. Exp. Biol. 221:jeb176024. doi: 10.1242/jeb.176024
Way, M. J., Conlong, D. E., Rutherford, R. S., Sweby, D. L., Gillespie, D. Y., Stranack, R. A., et al. (2017). Cacosceles (Zelogenes) newmannii (Thomson) (Cerambycidae: Prioninae), a new pest in the sugarcane industry. Proc. S. Afr. Sug. Technol. Ass. 90, 62–65.
West, G. B., Brown, J. H., and Enquist, B. J. (1997). A general model for the origin of allometric scaling laws in biology. Science 276, 122–126. doi: 10.1126/science.276.5309.122
West, G. B., Brown, J. H., and Enquist, B. J. (1999). The fourth dimension of life: fractal geometry and allometric scaling of organisms. Science 284, 1677–1679. doi: 10.1126/science.284.5420.1677
White, C. R., Terblanche, J. S., Kabat, A. P., Blackburn, T. M., Chown, S. L., and Butler, P. J. (2008). Allometric scaling of maximum metabolic rate: the influence of temperature. Funct. Ecol. 22, 616–623. doi: 10.1111/j.1365-2435.2008.01399.x
Woods, H. A., and Hill, R. I. (2004). Temperature-dependent oxygen limitation in insect eggs. J. Exp. Biol. 207, 2267–2276. doi: 10.1242/jeb.00991
Keywords: Cacosceles newmannii, thermolimit respirometry, critical temperature, tracheal system, hypoxia
Citation: Javal M, Thomas S, Lehmann P, Barton MG, Conlong DE, Du Plessis A and Terblanche JS (2019) The Effect of Oxygen Limitation on a Xylophagous Insect’s Heat Tolerance Is Influenced by Life-Stage Through Variation in Aerobic Scope and Respiratory Anatomy. Front. Physiol. 10:1426. doi: 10.3389/fphys.2019.01426
Received: 01 July 2019; Accepted: 04 November 2019;
Published: 20 November 2019.
Edited by:
Fernando Ariel Genta, Oswaldo Cruz Foundation (FIOCRUZ), BrazilReviewed by:
Jake Socha, Virginia Tech, United StatesJohn M. VandenBrooks, Arizona State University, United States
Copyright © 2019 Javal, Thomas, Lehmann, Barton, Conlong, Du Plessis and Terblanche. This is an open-access article distributed under the terms of the Creative Commons Attribution License (CC BY). The use, distribution or reproduction in other forums is permitted, provided the original author(s) and the copyright owner(s) are credited and that the original publication in this journal is cited, in accordance with accepted academic practice. No use, distribution or reproduction is permitted which does not comply with these terms.
*Correspondence: Marion Javal, bWphdmFsQHN1bi5hYy56YQ==