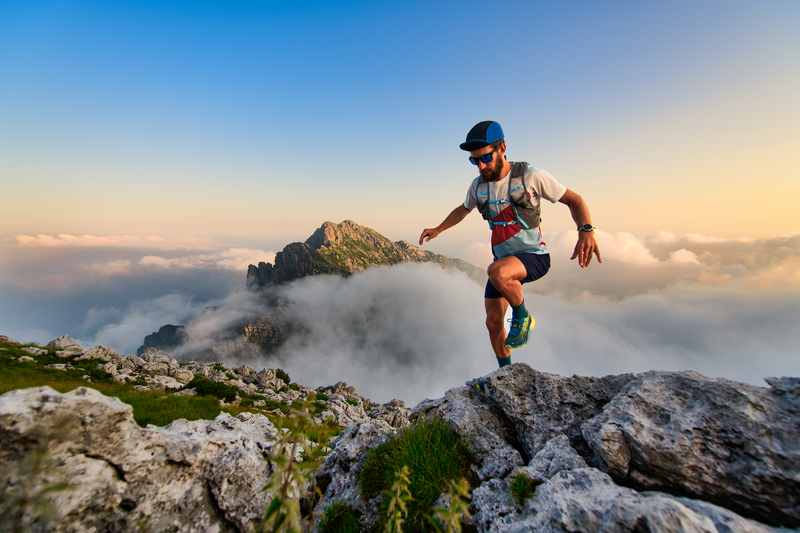
95% of researchers rate our articles as excellent or good
Learn more about the work of our research integrity team to safeguard the quality of each article we publish.
Find out more
MINI REVIEW article
Front. Physiol. , 27 September 2019
Sec. Redox Physiology
Volume 10 - 2019 | https://doi.org/10.3389/fphys.2019.01244
Biological methane formation is associated with anoxic environments and the activity of anaerobic prokaryotes (Archaea). However, recent studies have confirmed methane release from eukaryotes, including plants, fungi, and animals, even in the absence of microbes and in the presence of oxygen. Furthermore, it was found that aerobic methane emission in plants is stimulated by a variety of environmental stress factors, leading to reactive oxygen species (ROS) generation. Further research presented evidence that molecules with sulfur and nitrogen bonded methyl groups such as methionine or choline are carbon precursors of aerobic methane formation. Once generated, methane is widely considered to be physiologically inert in eukaryotes, but several studies have found association between mammalian methanogenesis and gastrointestinal (GI) motility changes. In addition, a number of recent reports demonstrated anti-inflammatory potential for exogenous methane-based approaches in model anoxia-reoxygenation experiments. It has also been convincingly demonstrated that methane can influence the downstream effectors of transiently increased ROS levels, including mitochondria-related pro-apoptotic pathways during ischemia-reperfusion (IR) conditions. Besides, exogenous methane can modify the outcome of gasotransmitter-mediated events in plants, and it appears that similar mechanism might be active in mammals as well. This review summarizes the relevant literature on methane-producing processes in eukaryotes, and the available results that underscore its bioactivity. The current evidences suggest that methane liberation and biological effectiveness are both linked to cellular redox regulation. The data collectively imply that exogenous methane influences the regulatory mechanisms and signaling pathways involved in oxidative and nitrosative stress responses, which suggests a modulator role for methane in hypoxia-linked pathologies.
Methane (CH4) is a ubiquitous, intrinsically non-toxic gas. It is a simple asphyxiant, which means that CH4 will displace oxygen to approx. 18% in air when present at about 14% (or 140000 parts per million by volume, ppmv) in a restricted area, but in this case hypoxia and the evolving cellular dysfunction will be due to the increasing concentration of CH4 and the decreased O2 content in the internal milieu and not to the chemical specificity of the gas (Boros et al., 2015).
In the Earth’s atmosphere, which contains approx. 1.8 ppmv CH4, a substantial part stems from the anaerobic degradation of biomass. Large amounts are formed in the gastrointestinal (GI) system of mammals as well, especially in ruminants, by methanogenic Archaea (Conrad, 2009; Kirschke et al., 2013). In these strictly anaerobic prokaryotes the terminal electron acceptor is carbon (mainly carbon dioxide and acetate but also other small organic compounds), and CH4 is formed from methyl-coenzyme M by methyl coenzyme M reductase (McBride and Wolfe, 1971; Ellermann et al., 1988). The intraluminally generated CH4 enters the splanchnic circulation, and then released into the breath if the partial pressure is higher than that in the atmosphere. In humans, the endogenous CH4 can be detected in the exhaled breath of 30–60% of adults with traditional analytic methods, when production is defined as a >1 ppmv increase above the ambient air level (Bond et al., 1971; de Lacy Costello et al., 2013). Here it should be noted that the intra- and inter-subject variability is usually very large (Pitt et al., 1980; Peled et al., 1985; Minocha and Rashid, 1997; Levitt et al., 2006; Roccarina et al., 2010; Sahakian et al., 2010), partly because the pulmonary route is not exclusive and the production is manifested not only in the exhaled air but also through other body surfaces (Nose et al., 2005). Besides, the production of CH4 is dependent from the age, the health condition and the physical activity of the subjects (Polag et al., 2014; Szabó et al., 2015; Tuboly et al., 2017; Polag and Keppler, 2018), and the breath output is influenced by splanchnic microcirculatory factors as well (Szücs et al., 2019). In accordance with the above findings the exhaled CH4 level in humans is always above the inhaled CH4 concentration (Keppler et al., 2016).
Apart from the above, several studies have confirmed direct, endogenous CH4 release in eukaryotes, including plants, fungi, algae, and animals, even in the absence of microbes and in the presence of O2 (Keppler et al., 2006; Wang et al., 2011; Lenhart et al., 2012; Althoff et al., 2014). In plants, “aerobic” or “non-archaeal” CH4 formation may be stimulated by reactive oxygen species (ROS) formation, UV radiation or inhibition of cytochrome c oxidase by sodium azide (NaN3) (Messenger et al., 2009; Qaderi and Reid, 2009; Wishkerman et al., 2011), and it appears that similar mechanisms might be active in animals also (Ghyczy et al., 2008; Tuboly et al., 2013; Boros et al., 2015). Based on these data, it was suggested that next to microbial origin there might be other, as yet unidentified sources for endogenous CH4 production (Keppler et al., 2009). In this sense, most of excreted CH4 in the breath of mammals may come from intestinal archaeal production, but a variable amount is possibly linked to non-archaeal processes.
Evidences were presented that molecules with sulfur and nitrogen bonded methyl groups such as methionine, methionine sulfoxide, S-adenosyl methionine, dimethyl sulfoxide or lecithin, choline, and betaine, respectively, might be carbon precursors of CH4 formation (Ghyczy and Boros, 2001; Ghyczy et al., 2003; Keppler et al., 2009; Althoff et al., 2010) and potentially serve as methyl donors for endogenous CH4 formation in eukaryotes. In this context it has been demonstrated that CH4 is readily formed from methionine in a model system containing iron(II/III), H2O2 and ascorbate under ambient (∼1.000 mbar and 22°C) and aerobic (21% O2) conditions (Althoff et al., 2014). Further mechanistic studies in non-heme oxo-iron(IV) models with tetra- or pentadentate ligands have demonstrated the formation of CH4, methanol (CH3OH), and formaldehyde (CH2O) from methionine and other thioethers (Benzing et al., 2017). In the course of the reaction, the thioether is oxidized by the oxo-iron(IV) species to a sulfoxide, with a bifurcation in the next oxidation step, either producing a sulfone or methyl radicals and sulfinic acid derivatives. In the presence of O2, the methyl radicals form predominantly CH3OH and CH2O, while in an O2-depleted environment they produce CH4 (Figure 1). In the latter case the required hydrogen radicals might be provided by hydrogen abstraction from carbohydrates or homolytic cleavage of hydrogen.
Figure 1. Simplified mechanism for oxo-iron(IV)-based formation of methane and methanol from methionine sulfoxide. The scheme is based on the results by Althoff et al. (2014) and Benzing et al. (2017).
The role of methyl thioethers in forming CH4 in biological systems is supported by further results, where the organisms were supplemented with positionally isotope-labeled methionine (Lenhart et al., 2015). These experiments provided direct evidence that the thio-CH3 group of methionine is a parent compound of CH4 and the highest CH4 formation rates are expected when the availability of O2 is limited. This conclusion is in broad agreement with previous results which showed enhanced CH4 formation in animal cells under reduced O2 content (Ghyczy et al., 2008).
Several studies demonstrated that CH4 might directly modulate the signaling mechanisms of the enteric nervous system and influences the peristaltic activity in the GI tract. The orocecal transit and total colonic transit times are prolonged in CH4-producer individuals, while diarrheal conditions are negatively associated with CH4 production (Pimentel et al., 2003; Lee et al., 2013; Triantafyllou et al., 2014; Gottlieb et al., 2016). These findings were consistent with the results of a series of in vivo and in vitro studies which demonstrated that exogenous CH4 slows the velocity of peristaltic contractions, augments the contractile force of ileal segments, and promotes the evolution of non-propagating contractions (Pimentel et al., 2006; Jahng et al., 2012). Other results provided evidence that CH4 infusion at a rate that corresponded to an increase of 50 ppmv in exhaled air induces a 59% slowing down of the intestinal transit. Furthermore, the addition of CH4 significantly increased the density of voltage-dependent potassium channels in isolated colonic smooth muscle cells (Liu et al., 2013).
Ischemia-reperfusion (IR) conditions are usually inducing antigen-independent inflammation, and inflammatory states are frequently accompanied by tissue hypoxia. An anti-inflammatory potential for CH4 was first reported in intestinal IR experiments (Boros et al., 2012). In this study the level of tissue ROS generation was reduced after CH4 administration, the vascular resistance changes were only moderate, and the local polymorphonuclear (PMN) leukocyte infiltration tended to normalize after reperfusion. The in vitro results substantiated the in vivo findings, and established that CH4 exposure specifically decreases the ROS production of activated PMN leukocytes (Boros et al., 2012). In another study normoxic ventilation with 2.5% CH4 maintained the superficial mucosal structure, the reperfusion-induced epithelial hyperpermeability was significantly alleviated and the microcirculatory flow reduction was prevented (Mészáros et al., 2017a).
Further in vitro and in vivo experimental data established that CH4 exposure can influence the activity of xanthine oxidoreductase (XOR) as well (Boros et al., 2012; Poles et al., 2018). XOR is a major enzymatic source of reperfusion-induced superoxide formation, and catalyze the reduction of nitrite to nitric oxide (NO) under hypoxic conditions in a pH-, nitrite-, and O2-dependent manner. In this line, an increase in CH4 input significantly decreased the elevated intestinal XOR activity in a rat model of intestinal IR, and in parallel, nitrotyrosine formation was suppressed. Interestingly, the reduced XOR activity was associated with a higher nNOS-immunopositive neuron ratio in several sections of the GI tract. Furthermore, normoxic CH4 administration significantly decreased tissue NO levels in the hypoxic duodenal tissue already during the ischemic phase, which suggests that CH4 may directly modulate XOR and XOR-linked nitrate reductase activities in the intestines (Poles et al., 2018).
Another important aspect is that methane-enriched saline (MRS) decreased the expression levels of activated apoptosis signal-regulating kinase 1 (ASK-1), c-Jun NH2-terminal kinase (JNK) and the pro-apoptotic protein Bcl-2 associated X protein (Bax), and increased the expression of the anti-apoptotic proto-oncogene protein B cell leukemia/lymphoma-2 (Bcl-2) proteins in a rat model of abdominal-island skin-flap IR (Song et al., 2015). Besides, MRS significantly prolonged the survival time of rats with myocardial ischemia induced by ligation of the left anterior descendent coronary artery (Chen et al., 2016). In this IR model, CH4 exerted a dose-dependent myocardial protection, characterized by a reduced infarct area and serum levels of myocardial necroenzymes. The pro-inflammatory activation [evidenced by TNF-α, IL-1β, myeloperoxidase (MPO) activity, and oxidative DNA damage] was reduced and a satisfactory cardiac function was maintained 4 weeks post-infarction with, among others, improved left ventricular ejection fraction, diastolic volume and contractility compared to non-CH4-treated animals. Again, MRS treatment reduced the protein expression of Bax, decreased cytoplasmic cytochrome c content and cleaved caspase-3, and caspase-9 levels, but markedly increased the levels of Bcl-2 and mitochondrial cytochrome c, indicating an anti-apoptotic effect here as well.
Similar efficiency and mechanisms were demonstrated in liver IR models; MRS or inhaled CH4 reduced hepatocyte apoptosis (Ye et al., 2015; Strifler et al., 2016). In addition to its anti-apoptotic properties, MRS treatment prevented the gene expression and production of early inflammatory cytokines TNF-α, IL-1β, and IL-6 and reduced infiltration of inflammatory CD68 positive cells in the liver tissue. In a partial hepatic IR model, the inhalation of normoxic CH4 preserved the respiratory capacity of mitochondria (complex II-coupled state III respiration) as compared to controls in the first 30 min of reperfusion (Strifler et al., 2016).
Secondary degeneration is a common event in traumatic nerve injuries, which involves neuronal apoptosis and mitochondrial dysfunction and among the various retinal neurons, retinal ganglion cells (RGCs) are thought to be the most vulnerable to IR injuries. MRS administration significantly attenuated RGCs loss and retinal thinning 1 week after the IR challenge. The visual function was also preserved, as demonstrated by the measurement of visual evoked potentials (Liu et al., 2016).
Analogous effects were demonstrated after optic nerve crush (ONC) as well (Wang R. et al., 2017). CH4 treatment significantly improved the signs of neurodegeneration, including RGC loss and visual dysfunction, inhibited the retinal neural apoptosis in the ganglion cell layer, accompanied by the up-regulations of anti-apoptotic factors (pGSK-3β, pBAD, Bcl-xL). The peroxisome proliferator-activated receptor gamma co-activator alpha (PGC-1α) is the master regulator of mitochondrial biogenesis, contributing to mitochondrial gene expression and mtDNA maintenance. Interestingly, CH4 administration after ONC improved the reduction of functional mitochondria markers, including citrate synthase activity and ATP content.
The nuclear factor-erythroid2 p45-related factor 2 (Nrf2)/Kelch-like ECH-associated protein 1 (Keap1) pathway is one of the major cellular defense mechanisms that operates during acute stress conditions. In another rat study with spinal cord ischemia and systemic hypotension, CH4 supplementation attenuated both motor and sensory deficits and increased the expression and transcriptional activity of Nrf2 in neurons, microglia and astrocytes in the ventral, intermediate and dorsal gray matter of lumbar segments (Wang L. et al., 2017). The CH4-induced time-dependent nuclear translocation of Nrf2 protein was accompanied by the downregulation of the Nrf2 inhibitor Keap 1 in the cytoplasmic fraction. Along these lines, hemoxygenase-1 (HO-1), SOD, catalase, and glutathione peroxidase were upregulated and oxidative stress markers glutathione disulfide, superoxide, hydrogen peroxide, malondialdehyde (MDA), 8-hydroxy-2-deoxyguanosine, and 3-nitrotyrosine were reduced (Wang L. et al., 2017).
In a similar rodent study with spinal cord injury at the T9-10 level, MRS decreased the infarct area and inflammatory cytokine production (TNF-α, IL-1β, and IL-6 content), suppressed microglial activation and improved hind limb neurological function 72 h following the insult (Wang W. et al., 2017). The protective effect of CH4 administration was demonstrated in cerebral IR as well (Zhang et al., 2017). Inhaled CH4 reduced MDA and TNF-α levels in the rat brain, significantly increased Akt phosphorylation and protected against neurological dysfunction. These effects were linked again to HO-1 activity (Zhang et al., 2017). In this line, in a recent rat study with complete Freund’s adjuvant (CFA)-induced chronic peripheral inflammation MRS treatment reduced the number of infiltrated peripheral T cells, the enhanced expression of IFN-γ and MMP-2 in the ipsilateral superficial spinal dorsal horn 10 days after CFA treatment, and allodynia was significantly alleviated as well (Zhou et al., 2018).
The generation of cytokines is one of the main consequences of lipopolysaccharide (LPS)-linked cellular reactions in various TLR4-expressing cell types. It has been shown that CH4 dose-dependently inhibited the LPS-induced NF-κB/mammalian mitogen-activated protein kinase (MAPK) signals and the expression of TNF-α and IL-6 proteins in macrophages (Zhang et al., 2016). In this study, CH4 treatment attenuated the phosphorylation of NF-κb, c-Jun NH2-terminal kinase (JNK), extracellular signal-regulated kinase (ERK) and P38MAPK in an IL-10-dependent manner via the enhanced activation of PI3K/AKT signaling (Zhang et al., 2016). Interestingly, a post-treatment regime was also effective, and the IL-6 mRNA levels were reduced by approximately 95% 6 h after LPS stimulation. Consistent with the in vitro findings, the serum levels of TNF-α and IL-6 of CH4-treated mice were significantly reduced during E. coli bacteremia, while the PI3K/AKT/GSK-3β-mediated IL-10 expression was enhanced.
In another rat model of LPS-induced acute lung injury, CH4 treatment improved the survival rate, reduced the number of infiltrated inflammatory cells (PMN leukocytes and lymphocytes), improved the lung function (the PaO2/FIO2 ratio), pulmonary permeability and the structural damage as well (Sun et al., 2017). Furthermore, MRS improved the 5-day survival and organ functions in mice with cecum ligation and puncture (CLP), and alleviated the signs of CLP-induced endoplasmic reticulum stress-related apoptosis (GRP78/ATF4/CHOP/caspase-12) in tubular endothelial cells in rats (Jia et al., 2018; Li et al., 2019).
Whereas the data establish a bioactive role for CH4 the mechanism of action is still incompletely defined, and at least four direct and indirect mechanistic ways can be considered to explain the results.
Firstly, the effects of increased CH4 concentrations on NO-, CO-, and H2S-linked reactions should be taken into account when explaining the versatile in vivo effects of exogenous CH4. It has been shown in plants that methane-enriched water (MRW) increases root organogenesis through the HO-1 pathway and CO generation (Cui et al., 2015). Further, it has also been shown that H2S and NO can also be downstream signaling molecules involved in CH4-induced adventitious root formation (Qi et al., 2017; Kou et al., 2018). Similar results were demonstrated in several stress conditions coupled to redox imbalance which confirmed that CO, NO, and H2S signaling mechanisms are involved in the molecular basis of CH4-induced stress tolerance in plant tissues (Han et al., 2017; Samma et al., 2017; Zhang et al., 2018). These data clearly demonstrate the connection between the generation of recognized gasotransmitters and the presence of CH4 in a complex living system (Song et al., 2008; Wang et al., 2013; Han et al., 2017; Khan et al., 2017; Samma et al., 2017; Kou et al., 2018; Zhang et al., 2018; Figure 2).
Figure 2. Interaction of biologically active gases, nitric oxide (NO), carbon monoxide (CO), and hydrogen sulfide (H2S) with methane (CH4) in plants. Relevant literature references (Song et al., 2008; Wang et al., 2013; Cui et al., 2015; Han et al., 2017; Khan et al., 2017; Qi et al., 2017; Samma et al., 2017; Kou et al., 2018; Zhang et al., 2018) are provided in the text. Similar mechanisms may operate in mammals.
The same datasets are not yet available in mammals, but there are many possibilities for gas interactions in the GI tract. Methanogen archaea in the intestinal lumen are compelled to compete with other microorganisms, such as sulfate-reducing bacteria for the common substrates, hence the amount of CH4 is always variable (Levitt and Bond, 1970). In this milieu the concentration of CH4 is always dependent on the concentration of O2 and the presence of other gaseous products, such as molecular hydrogen (H2) to produce CH4 from CO2 (Gibson et al., 1988). Thereafter the conversion of H2 to CH4 is associated with the reduction of five moles of gas into one mole of gas, thus the reaction decreases the intraluminal gas volume (Levitt and Bond, 1970). In contrast, the breathing of nitrous oxide (N2O) causes the expansion of CH4-containing intestinal segments (Steffey et al., 1979), while subsequent O2 breathing reduces the volume of the CH4-containing segment toward control volumes (Steffey et al., 1979). H2 can also act as an electron donor for dissimilatory sulfate reduction. In this case hydrogen sulfide (H2S) might be the primary, terminal reaction product (Gibson et al., 1990; Christl et al., 1992). Carbon monoxide (CO) may also induce an elevation in H2S production, while NO can interact with H2S (Magierowski et al., 2016).
These data suggest that the final biological effect of a gasotransmitter can be determined by multiple and multicomponent gaseous interactions. Here it should be added that there is a conceptual difference between the baseline level of a bioactive gas (i.e., NO, CO, or H2S), and its de novo release by inducer factors, as the evolving responses will be dependent on the number of molecules and/or their reactivity in the microenvironment.
Several further lines of evidence indicate that CH4 ameliorates the function of the tissue barriers, including the blood-retinal barrier, the blood-spinal cord barrier and the mucosal barrier under oxido-reductive stress conditions (Wu et al., 2015; Shen et al., 2016; Mészáros et al., 2017a). Besides, exogenous CH4 improved erythrocyte deformability at low-to-moderate shear stress rates (Mészáros et al., 2017a). These data suggest a direct effect on membrane-cytoskeleton junctions and/or on cell-cell junction proteins. As compared to NO, CH4 may reach higher concentrations when dissolved in water or colloid solutions, and ROS generation can lead to a higher level of CH4 degradation in the lipid environment of membranes. The apolar CH4 may enter and dissolve in the hydrophobic non-polar lipid tails of the phospholipid biomembranes, theoretically influencing its physicochemical condition, which is essential for the normal functioning of embedded proteins and ion channels. Membrane rigidity relates to the degree of lipid peroxidation, and CH4 dissolved in biological membranes may affect this process, thereby influencing the stereo figure of membrane proteins that determines their accessibility and morphology.
As discussed before, higher concentrations of CH4 can lead to anti-inflammatory responses via master switches such as Nrf2/Keap1 or NF-κB (Wang L. et al., 2017). Recent studies demonstrated the activation of caspase-9 and caspase-3 and significantly increased cytochrome c release into the cytoplasm from the mitochondria after spinal cord IR (Wang L. et al., 2017; Wang W. et al., 2017). Increased mRNA and content of TNF-α, IL-1β, CXCL1, and ICAM-1 were also observed in IR; however, the increases and the apoptotic effects were blocked by CH4 administration. Nrf2 has also been shown to have a key role in signaling the antioxidant response element (ARE)-mediated regulation of gene expression. As it happens, CH4 induces the time-dependent nuclear translocation of Nrf2 protein and, in addition, the increased nuclear Nrf2 was accompanied by the down-regulation of the Nrf2 inhibitor, Keap 1, in the cytoplasmic fraction. This occurred in association with the phosphorylation and nuclear translocation of the NF-κB p65 subunit. The nucleoplasmic ratio of phospho-NF-κB p65 was increased at 72 h post injury relative to sham-operated rats, but this increase was inhibited by CH4 treatment. Furthermore, after Nrf2 knockdown by intrathecal siRNA pretreatment, the nuclear accumulation of phospho-NF-κB p65 was induced as compared to CH4-treated rats. To sum up, lots of data point to a direct anti-cytokine effect of CH4 through influencing NF-κB and Nrf2 activation.
Lastly, it seems that mitochondria may have a fundamental role to connect the individual effects of distinct interventions, providing an explanation of why CH4 supplementation may interfere with the consequences of diverse conditions associated with hypoxia and inflammation (Mészáros et al., 2017b). It is well established that the antigen-independent IR stimulus can initiate mitochondria-related intrinsic signaling pathways of apoptosis. MRS and CH4-containing air preserved the oxidative phosphorylation and improved the basal mitochondrial respiration state after the onset of reperfusion in liver IR, and cytochrome c oxidase activity together with ROS production and hepatocyte apoptosis were also reduced (Ye et al., 2015; Strifler et al., 2016). These findings are consistently present in other tissues as well, such as the skin, retina, heart, and spinal cord with IR injury and CH4 treatments (Song et al., 2015; Chen et al., 2016; Liu et al., 2016; Zhang et al., 2016, 2017; Wang L. et al., 2017; Wang R. et al., 2017; Wang W. et al., 2017; Zhou et al., 2018). Based on the totality of data, it seems plausible that exogenous CH4 confers cellular protection by the restoration of mitochondrial function, and probably membrane integrity through the expression of Bcl-2 family of anti-apoptotic proteins, decreasing the release of cytochrome c and deactivating the caspase signaling cascade.
Signaling roles were demonstrated for NO, CO, and H2S, and it has become clear that gaseous mediators are forming complex intracellular pathways and regulate numerous physiological processes in cooperative ways. Whether methane itself or a reaction product acts as the effector is an intriguing possibility. To answer this question much more detailed studies are necessary and should be conducted in the future. If we discuss the available literature on the generation and biological effects of CH4 from such aspects, the current evidences support the notion that the bioactivity of CH4 is linked to other gasotransmitter-mediated events. Although the results indicate a bioactive role for higher concentrations of exogenous CH4 it should be noted that this is not obvious for endogenous sources; and there is still no clear-cut evidence that CH4 in the endogenously produced concentration range (1–30 ppmv) has a role in cellular physiology. Nevertheless, evidences are available that exogenous CH4 is able to influence cytoprotective pathways. Besides, sufficient evidence was accumulated to justify the exploration of CH4 as a therapeutic agent in inflammatory disorders or inflammation-linked pathologies. In this framework the available data support a controller role for CH4 to reduce the inflammatory signals toward resting conditions.
MB and FK contributed with the conception and literature review and analysis, drafted, and edited the final version of the manuscript.
This work was supported by Hungarian Research Foundation (NKFI K120232 and 20391-3/2018/FEKUSTRAT) and German Science Foundation (DFG; KE 884/8-1 and KE 884/8-2).
The authors declare that the research was conducted in the absence of any commercial or financial relationships that could be construed as a potential conflict of interest.
The reviewer, ET, declared a past co-authorship, with one of the authors, MB, to the handling Editor.
We thank Bianca Pokrandt for preparing Figure 1.
Althoff, F., Benzing, K., Comba, P., McRoberts, C., Boyd, D. R., Greiner, S., et al. (2014). Abiotic methanogenesis from organosulphur compounds under ambient conditions. Nat. Commun. 5:4205. doi: 10.1038/ncomms5205
Althoff, F., Jugold, A., and Keppler, F. (2010). Methane formation by oxidation of ascorbic acid using iron minerals and hydrogen peroxide. Chemosphere 80, 286–292. doi: 10.1016/j.chemosphere.2010.04.004
Benzing, K., Comba, P., Martin, B., Pokrandt, B., and Keppler, F. (2017). Nonheme iron-oxo-catalyzed methane formation from methyl thioethers: scope, mechanism, and relevance for natural systems. Chemistry 23, 10465–10472. doi: 10.1002/chem.201701986
Bond, J. H. Jr., Engel, R. R., and Levitt, M. D. (1971). Factors influencing pulmonary methane excretion in man. An indirect method of studying the in situ metabolism of the methane-producing colonic bacteria. J. Exp. Med. 133, 572–588. doi: 10.1084/jem.133.3.572
Boros, M., Ghyczy, M., Érces, D., Varga, G., Tőkés, T., Kupai, K., et al. (2012). The anti-inflammatory effects of methane. Crit. Care Med. 40, 1269–1278. doi: 10.1097/CCM.0b013e31823dae05
Boros, M., Tuboly, E., Mészáros, A., and Amann, A. (2015). The role of methane in mammalian physiology-is it a gasotransmitter? J. Breath Res. 9:014001. doi: 10.1088/1752-7155/9/1/014001
Chen, O., Ye, Z., Cao, Z., Manaenko, A., Ning, K., Zhai, X., et al. (2016). Methane attenuates myocardial ischemia injury in rats through anti-oxidative, anti-apoptotic and anti-inflammatory actions. Free Radic. Biol. Med. 90, 1–11. doi: 10.1016/j.freeradbiomed.2015.11.017
Christl, S. U., Murgatroyd, P. R., Gibson, G. R., and Cummings, J. H. (1992). Production, metabolism, and excretion of hydrogen in the large intestine. Gastroenterology 102, 1269–1277. doi: 10.1016/0016-5085(92)70022-4
Conrad, R. (2009). The global methane cycle: recent advances in understanding the microbial processes involved. Environ. Microbiol. Rep. 1, 285–292. doi: 10.1111/j.1758-2229.2009.00038.x
Cui, W., Qi, F., Zhang, Y., Cao, H., Zhang, J., Wang, R., et al. (2015). Methane-rich water induces cucumber adventitious rooting through heme oxygenase1/carbon monoxide and Ca(2+) pathways. Plant Cell Rep. 34, 435–445. doi: 10.1007/s00299-014-1723-3
de Lacy Costello, B. P., Ledochowski, M., and Ratcliffe, N. M. (2013). The importance of methane breath testing: a review. J. Breath Res. 7:024001. doi: 10.1088/1752-7155/7/2/024001
Ellermann, J., Hedderich, R., Böcher, R., and Thauer, R. K. (1988). The final step in methane formation. Investigations with highly purified methyl-CoM reductase (component C) from Methanobacterium thermoautotrophicum (strain marburg). Eur. J. Biochem. 172, 669–677. doi: 10.1111/j.1432-1033.1988.tb13941.x
Ghyczy, M., and Boros, M. (2001). Electrophilic methyl groups present in the diet ameliorate pathological states induced by reductive and oxidative stress: a hypothesis. Br. J. Nutr. 85, 409–414. doi: 10.1079/bjn2000274
Ghyczy, M., Torday, C., and Boros, M. (2003). Simultaneous generation of methane, carbon dioxide, and carbon monoxide from choline and ascorbic acid: a defensive mechanism against reductive stress? FASEB J. 17, 1124–1126. doi: 10.1096/fj.02-0918fje
Ghyczy, M., Torday, C., Kaszaki, J., Szabó, A., Czóbel, M., and Boros, M. (2008). Hypoxia-induced generation of methane in mitochondria and eukaryotic cells: an alternative approach to methanogenesis. Cell Physiol. Biochem. 21, 251–258. doi: 10.1159/000113766
Gibson, G. R., Cummings, J. H., and Macfarlane, G. T. (1988). Competition for hydrogen between sulphate-reducing bacteria and methanogenic bacteria from the human large intestine. J. Appl. Bacteriol. 65, 241–247. doi: 10.1111/j.1365-2672.1988.tb01891.x
Gibson, G. R., Cummings, J. H., Macfarlane, G. T., Allison, C., Segal, I., Vorster, H. H., et al. (1990). Alternative pathways for hydrogen disposal during fermentation in the human colon. Gut 31, 679–683. doi: 10.1136/gut.31.6.679
Gottlieb, K., Wacher, V., Sliman, J., and Pimentel, M. (2016). Review article: inhibition of methanogenic archaea by statins as a targeted management strategy for constipation and related disorders. Aliment. Pharmacol. Ther. 43, 197–212. doi: 10.1111/apt.13469
Han, B., Duan, X., Wang, Y., Zhu, K., Zhang, J., Wang, R., et al. (2017). Methane protects against polyethylene glycol-induced osmotic stress in maize by improving sugar and ascorbic acid metabolism. Sci. Rep. 7:46185. doi: 10.1038/srep46185
Jahng, J., Jung, I. S., Choi, E. J., Conklin, J. L., and Park, H. (2012). The effects of methane and hydrogen gases produced by enteric bacteria on ileal motility and colonic transit time. Neurogastroenterol. Motil. 24, 185–190. doi: 10.1111/j.1365-2982.2011.01819.x
Jia, Y., Li, Z., Feng, Y., Cui, R., Dong, Y., Zhang, X., et al. (2018). Methane-rich saline ameliorates sepsis-induced acute kidney injury through anti-inflammation, antioxidative, and antiapoptosis effects by regulating endoplasmic reticulum stress. Oxid. Med. Cell Longev. 2018:4756846. doi: 10.1155/2018/4756846
Keppler, F., Boros, M., Frankenberg, C., Lelieveld, J., McLeod, A., Pirttilä, A. M., et al. (2009). Methane formation in aerobic environments. Environ. Chem. 6, 459–465. doi: 10.1071/EN09137
Keppler, F., Hamilton, J. T., Brass, M., and Röckmann, T. (2006). Methane emissions from terrestrial plants under aerobic conditions. Nature 439, 187–191. doi: 10.1038/nature04420
Keppler, F., Schiller, A., Ehehalt, R., Greule, M., Hartmann, J., and Polag, D. (2016). Stable isotope and high precision concentration measurements confirm that all humans produce and exhale methane. J. Breath Res. 10:016003. doi: 10.1088/1752-7155/10/1/016003
Khan, M. N., Mobin, M., Abbas, Z. K., and Siddiqui, M. H. (2017). Nitric oxide-induced synthesis of hydrogen sulfide alleviates osmotic stress in wheat seedlings through sustaining antioxidant enzymes, osmolyte accumulation and cysteine homeostasis. Nitric Oxide 68, 91–102. doi: 10.1016/j.niox.2017.01.001
Kirschke, S., Bousquet, P., Ciais, P., Saunois, M., Canadell, J. G., Dlugokencky, E. J., et al. (2013). Three decades of global methane sources and sinks. Nat. Geosci. 6, 813–823. doi: 10.1038/ngeo1955
Kou, N., Xiang, Z., Cui, W., Li, L., and Shen, W. (2018). Hydrogen sulfide acts downstream of methane to induce cucumber adventitious root development. J. Plant Physiol. 228, 113–120. doi: 10.1016/j.jplph.2018.05.010
Lee, K. M., Paik, C. N., Chung, W. C., Yang, J. M., and Choi, M. G. (2013). Breath methane positivity is more common and higher in patients with objectively proven delayed transit constipation. Eur. J. Gastroenterol. Hepatol. 25, 726–732. doi: 10.1097/MEG.0b013e32835eb916
Lenhart, K., Althoff, F., Greule, M., and Keppler, F. (2015). Technical note: methionine, a precursor of methane in living plants. Biogeosciences 12, 1907–1914. doi: 10.5194/bg-12-1907-2015
Lenhart, K., Bunge, M., Ratering, S., Neu, T. R., Schüttmann, I., Greule, M., et al. (2012). Evidence for methane production by saprotrophic fungi. Nat. Commun. 3:1046. doi: 10.1038/ncomms2049
Levitt, M. D., and Bond, J. H. Jr. (1970). Volume, composition, and source of intestinal gas. Gastroenterology 59, 921–929. doi: 10.1016/s0016-5085(19)33654-6
Levitt, M. D., Furne, J. K., and Kuskowski, M. (2006). Stability of human methanogenic flora over 35 years and a review of insights obtained from breath methane measurements. Clin. Gastroenterol. Hepatol. 4, 123–129. doi: 10.1016/j.cgh.2005.11.006
Li, Z., Jia, Y., Feng, Y., Cui, R., Miao, R., Zhang, X., et al. (2019). Methane alleviates sepsis-induced injury by inhibiting pyroptosis and apoptosis in vivo and in vitro experiments. Aging 11, 1226–1239. doi: 10.18632/aging.101831
Liu, L., Sun, Q., Wang, R., Chen, Z., Wu, J., Xia, F., et al. (2016). Methane attenuates retinal ischemia/reperfusion injury via anti-oxidative and anti-apoptotic pathways. Brain Res. 1646, 327–333. doi: 10.1016/j.brainres.2016.05.037
Liu, Y., Luo, H. S., Liang, C. B., Tan, W., Xia, H., and Xu, W. J. (2013). Effects of methane on proximal colon motility of rats and ion channel mechanisms. Zhonghua Yi Xue Za Zhi 93, 459–463.
Magierowski, M., Magierowska, K., Hubalewska-Mazgaj, M., Adamski, J., Bakalarz, D., Sliwowski, Z., et al. (2016). Interaction between endogenous carbon monoxide and hydrogen sulfide in the mechanism of gastroprotection against acute aspirin-induced gastric damage. Pharmacol. Res. 114, 235–250. doi: 10.1016/j.phrs.2016.11.001
McBride, B. C., and Wolfe, R. S. (1971). A new coenzyme of methyl transfer, coenzyme M. Biochemistry 10, 2317–2324. doi: 10.1021/bi00788a022
Messenger, D. J., McLeod, A. R., and Fry, S. C. (2009). The role of ultraviolet radiation, photosensitizers, reactive oxygen species and ester groups in mechanisms of methane formation from pectin. Plant Cell Environ. 32, 1–9. doi: 10.1111/j.1365-3040.2008.01892.x
Mészáros, A. T., Büki, T., Fazekas, B., Tuboly, E., Horváth, K., Poles, M. Z., et al. (2017a). Inhalation of methane preserves the epithelial barrier during ischemia and reperfusion in the rat small intestine. Surgery 161, 1696–1709. doi: 10.1016/j.surg.2016.12.040
Mészáros, A. T., Szilágyi, ÁL., Juhász, L., Tuboly, E., Érces, D., Varga, G., et al. (2017b). Mitochondria as sources and targets of methane. Front. Med. 4:195. doi: 10.3389/fmed.2017.00195
Minocha, A., and Rashid, S. (1997). Reliability and reproducibility of breath hydrogen and methane in male diabetic subjects. Dig. Dis. Sci. 42, 672–676.
Nose, K., Nunome, Y., Kondo, T., Araki, S., and Tsuda, T. (2005). Identification of gas emanated from human skin: methane, ethylene, and ethane. Anal. Sci. 21, 625–628. doi: 10.2116/analsci.21.625
Peled, Y., Gilat, T., Liberman, E., and Bujanover, Y. (1985). The development of methane production in childhood and adolescence. J. Pediatr. Gastroenterol. Nutr. 4, 575–579. doi: 10.1097/00005176-198508000-00013
Pimentel, M., Lin, H. C., Enayati, P., van den Burg, B., Lee, H. R., Chen, J. H., et al. (2006). Methane, a gas produced by enteric bacteria, slows intestinal transit and augments small intestinal contractile activity. Am. J. Physiol. Gastrointest. Liver Physiol. 290, G1089–G1095. doi: 10.1152/ajpgi.00574.2004
Pimentel, M., Mayer, A. G., Park, S., Chow, E. J., Hasan, A., and Kong, Y. (2003). Methane production during lactulose breath test is associated with gastrointestinal disease presentation. Dig. Dis. Sci. 48, 86–92.
Pitt, P., de Bruijn, K. M., Beeching, M. F., Goldberg, E., and Blendis, L. M. (1980). Studies on breath methane: the effect of ethnic origins and lactulose. Gut 21, 951–954. doi: 10.1136/gut.21.11.951
Polag, D., and Keppler, F. (2018). Long-term monitoring of breath methane. Sci. Total Environ. 624, 69–77. doi: 10.1016/j.scitotenv.2017.12.097
Polag, D., Leiß, O., and Keppler, F. (2014). Age dependent breath methane in the German population. Sci. Total Environ. 481, 582–587. doi: 10.1016/j.scitotenv.2014.02.086
Poles, M. Z., Bódi, N., Bagyánszki, M., Fekete, É, Mészáros, A. T., Varga, G., et al. (2018). Reduction of nitrosative stress by methane: neuroprotection through xanthine oxidoreductase inhibition in a rat model of mesenteric ischemia-reperfusion. Free Radic. Biol. Med. 120, 160–169. doi: 10.1016/j.freeradbiomed.2018.03.024
Qaderi, M. M., and Reid, D. M. (2009). Methane emissions from six crop species exposed to three components of global climate change: temperature, ultraviolet-B radiation and water stress. Physiol. Plant 137, 139–147. doi: 10.1111/j.1399-3054.2009.01268.x
Qi, F., Xiang, Z., Kou, N., Cui, W., Xu, D., Wang, R., et al. (2017). Nitric oxide is involved in methane-induced adventitious root formation in cucumber. Physiol. Plant 159, 366–377. doi: 10.1111/ppl.12531
Roccarina, D., Lauritano, E. C., Gabrielli, M., Franceschi, F., Ojetti, V., and Gasbarrini, A. (2010). The role of methane in intestinal diseases. Am. J. Gastroenterol. 105, 1250–1256. doi: 10.1038/ajg.2009.744
Sahakian, A. B., Jee, S. R., and Pimentel, M. (2010). Methane and the gastrointestinal tract. Dig. Dis. Sci. 55, 2135–2143. doi: 10.1007/s10620-009-1012-0
Samma, M. K., Zhou, H., Cui, W., Zhu, K., Zhang, J., and Shen, W. (2017). Methane alleviates copper-induced seed germination inhibition and oxidative stress in medicago sativa. Biometals 30, 97–111. doi: 10.1007/s10534-017-9989-x
Shen, M., Fan, D., Zang, Y., Chen, Y., Zhu, K., Cai, Z., et al. (2016). Neuroprotective effects of methane-rich saline on experimental acute carbon monoxide toxicity. J. Neurol. Sci. 369, 361–367. doi: 10.1016/j.jns.2016.08.055
Song, K., Zhang, M., Hu, J., Liu, Y., Liu, Y., Wang, Y., et al. (2015). Methane-rich saline attenuates ischemia/reperfusion injury of abdominal skin flaps in rats via regulating apoptosis level. BMC Surg. 15:92. doi: 10.1186/s12893-015-0075-4
Song, X. G., She, X. P., and Zhang, B. (2008). Carbon monoxide-induced stomatal closure in Vicia faba is dependent on nitric oxide synthesis. Physiol. Plant 132, 514–525. doi: 10.1111/j.1399-3054.2007.01026.x
Steffey, E. P., Johnson, B. H., Eger, E. I. II, and Howland, D. Jr. (1979). Nitrous oxide: effect on accumulation rate and uptake of bowel gases. Anesth. Analg. 58, 405–408.
Strifler, G., Tuboly, E., Szél, E., Kaszonyi, E., Cao, C., Kaszaki, J., et al. (2016). Inhaled methane limits the mitochondrial electron transport chain dysfunction during experimental liver ischemia-reperfusion injury. PLoS One 11:e0146363. doi: 10.1371/journal.pone.0146363
Sun, A., Wang, W., Ye, X., Wang, Y., Yang, X., Ye, Z., et al. (2017). Protective effects of methane-rich saline on rats with lipopolysaccharide-induced acute lung injury. Oxid. Med. Cell Longev. 2017:7430193. doi: 10.1155/2017/7430193
Szabó, A., Ruzsanyi, V., Unterkofler, K., Mohácsi, Á, Tuboly, E., Boros, M., et al. (2015). Exhaled methane concentration profiles during exercise on an ergometer. J. Breath Res. 9:016009. doi: 10.1088/1752-7155/9/1/016009
Szücs, S., Bari, G., Ugocsai, M., Lashkarivand, R. A., Lajkó, N., and Mohácsi, Á, et al. (2019). Detection of intestinal tissue perfusion changes by real-time breath methane analysis in rat and pig models of mesenteric circulatory distress. Crit. Care Med. 47, e403–e411. doi: 10.1097/CCM.0000000000003659
Triantafyllou, K., Chang, C., and Pimentel, M. (2014). Methanogens, methane and gastrointestinal motility. J. Neurogastroenterol. Motil. 20, 31–40. doi: 10.5056/jnm.2014.20.1.31
Tuboly, E., Molnár, R., Tökés, T., Turányi, R. N., Hartmann, P., Mészáros, A. T., et al. (2017). Excessive alcohol consumption induces methane production in humans and rats. Sci. Rep. 7:7329. doi: 10.1038/s41598-017-07637-3
Tuboly, E., Szabó, A., Garab, D., Bartha, G., Janovszky, Á, Erõs, G., et al. (2013). Methane biogenesis during sodium azide-induced chemical hypoxia in rats. Am. J. Physiol. Cell Physiol. 304, C207–C214. doi: 10.1152/ajpcell.00300.2012
Wang, B., Hou, L., Liu, W., and Wang, Z. (2013). Non-microbial methane emissions from soils. Atmos. Environ. 80, 290–298. doi: 10.1016/j.atmosenv.2013.08.010
Wang, L., Yao, Y., He, R., Meng, Y., Li, N., Zhang, D., et al. (2017). Methane ameliorates spinal cord ischemia-reperfusion injury in rats: antioxidant, anti-inflammatory and anti-apoptotic activity mediated by Nrf2 activation. Free Radic. Biol. Med. 103, 69–86. doi: 10.1016/j.freeradbiomed.2016.12.014
Wang, R., Sun, Q., Xia, F., Chen, Z., Wu, J., Zhang, Y., et al. (2017). Methane rescues retinal ganglion cells and limits retinal mitochondrial dysfunction following optic nerve crush. Exp. Eye Res. 159, 49–57. doi: 10.1016/j.exer.2017.03.008
Wang, W., Huang, X., Li, J., Sun, A., Yu, J., Xie, N., et al. (2017). Methane suppresses microglial activation related to oxidative, inflammatory, and apoptotic injury during spinal cord injury in rats. Oxid. Med. Cell Longev. 2017:2190897. doi: 10.1155/2017/2190897
Wang, Z. P., Xie, Z. Q., Zhang, B. C., Hou, L. Y., Zhou, Y. H., Li, L. H., et al. (2011). Aerobic and anaerobic nonmicrobial methane emissions from plant material. Environ. Sci. Technol. 45, 9531–9537. doi: 10.1021/es2020132
Wishkerman, A., Greiner, S., Ghyczy, M., Boros, M., Rausch, T., Lenhart, K., et al. (2011). Enhanced formation of methane in plant cell cultures by inhibition of cytochrome c oxidase. Plant Cell Environ. 34, 457–464. doi: 10.1111/j.1365-3040.2010.02255.x
Wu, J., Wang, R., Ye, Z., Sun, X., Chen, Z., Xia, F., et al. (2015). Protective effects of methane-rich saline on diabetic retinopathy via anti-inflammation in a streptozotocin-induced diabetic rat model. Biochem. Biophys. Res. Commun. 466, 155–161. doi: 10.1016/j.bbrc.2015.08.121
Ye, Z., Chen, O., Zhang, R., Nakao, A., Fan, D., Zhang, T., et al. (2015). Methane attenuates hepatic ischemia/reperfusion injury in rats through antiapoptotic, anti-inflammatory and antioxidative actions. Shock 44, 181–187. doi: 10.1097/SHK.0000000000000385
Zhang, B., Gao, M., Shen, J., and He, D. (2017). Inhaled methane protects rats against neurological dysfunction induced by cerebral ischemia and reperfusion injury: PI3K/Akt/HO-1 pathway involved. Arch. Med. Res. 48, 520–525. doi: 10.1016/j.arcmed.2018.01.001
Zhang, X., Li, N., Shao, H., Meng, Y., Wang, L., Wu, Q., et al. (2016). Methane limit LPS-induced NF-κB/MAPKs signal in macrophages and suppress immune response in mice by enhancing PI3K/AKT/GSK-3β-mediated IL-10 expression. Sci. Rep. 6:29359. doi: 10.1038/srep29359
Zhang, Y., Su, J., Cheng, D., Wang, R., Mei, Y., Hu, H., et al. (2018). Nitric oxide contributes to methane-induced osmotic stress tolerance in mung bean. BMC Plant Biol. 18:207. doi: 10.1186/s12870-018-1426-y
Keywords: bioactive gases, methanogenesis, nitroxidative stress, ischemia-reperfusion, mitochondria
Citation: Boros M and Keppler F (2019) Methane Production and Bioactivity-A Link to Oxido-Reductive Stress. Front. Physiol. 10:1244. doi: 10.3389/fphys.2019.01244
Received: 20 June 2019; Accepted: 10 September 2019;
Published: 27 September 2019.
Edited by:
Simona Martinotti, Università del Piemonte Orientale, ItalyReviewed by:
Eszter Tuboly, University College Cork, IrelandCopyright © 2019 Boros and Keppler. This is an open-access article distributed under the terms of the Creative Commons Attribution License (CC BY). The use, distribution or reproduction in other forums is permitted, provided the original author(s) and the copyright owner(s) are credited and that the original publication in this journal is cited, in accordance with accepted academic practice. No use, distribution or reproduction is permitted which does not comply with these terms.
*Correspondence: Mihály Boros, Ym9yb3MubWloYWx5QG1lZC51LXN6ZWdlZC5odQ==; b2ZmaWNlLmV4cHN1ckBtZWQudS1zemVnZWQuaHU=
†These authors have contributed equally to this work
Disclaimer: All claims expressed in this article are solely those of the authors and do not necessarily represent those of their affiliated organizations, or those of the publisher, the editors and the reviewers. Any product that may be evaluated in this article or claim that may be made by its manufacturer is not guaranteed or endorsed by the publisher.
Research integrity at Frontiers
Learn more about the work of our research integrity team to safeguard the quality of each article we publish.