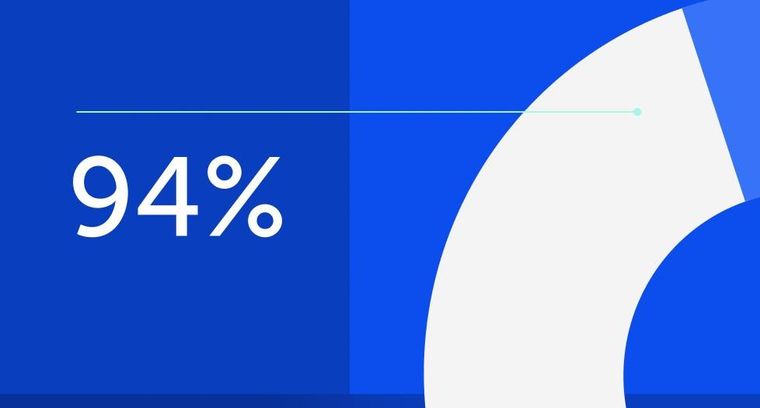
94% of researchers rate our articles as excellent or good
Learn more about the work of our research integrity team to safeguard the quality of each article we publish.
Find out more
MINI REVIEW article
Front. Physiol., 30 July 2019
Sec. Exercise Physiology
Volume 10 - 2019 | https://doi.org/10.3389/fphys.2019.00979
This article is part of the Research TopicChildren's Exercise PhysiologyView all 29 articles
Peak oxygen uptake () is internationally recognized as the criterion measure of youth aerobic fitness, but flawed laboratory assessments and fallacious interpretations of peak in ratio with body mass have confused our understanding of the development of aerobic fitness. Moreover, the recent emergence of specious predictions of peak from performance tests and the promotion of spurious “clinical red flags” and cardiometabolic cut-points have confused our understanding of the relationship between youth aerobic fitness and health. Recent longitudinal studies of 10–18-year-olds using multilevel allometric modeling have empirically demonstrated that peak increases in accord with sex-specific, concurrent changes in age- and maturity status-driven morphological covariates with the timing and tempo of changes specific to individuals. During both cycle ergometry and treadmill running age- and maturity status- driven changes in fat free mass have been revealed as the most powerful morphological influences on the development of youth aerobic fitness. To bring some clarity to current confusion, this paper argues that future studies must be founded on rigorous assessment and interpretation of peak and ensure that they address the development of youth aerobic fitness and its relationship with present and future health in relation to appropriate sex-specific morphological covariates governed by individual biological clocks.
Aerobic fitness defines the ability to deliver oxygen from the atmosphere to the skeletal muscles and to use it to generate energy to support muscle activity during exercise. Peak oxygen uptake (), the highest rate of oxygen consumed during an incremental exercise test to exhaustion, limits the capacity to perform aerobic exercise and is internationally recognized as the best single measure of youth aerobic fitness. Peak is the most researched physiological variable in pediatric exercise physiology but understanding of the development of youth aerobic fitness is embedded in confusion with shoddy assessments and fallacious interpretations of peak during growth and maturation. Moreover, youth aerobic fitness and its relationship with current and future health is shrouded in confusion through a resurgence of specious predictions of peak from performance tests and the promotion of spurious “cardiometabolic cut-points” and “clinical red flags.” To bring some clarity to current confusion, this mini-review critically reviews the evidence relating peak to changes in age, maturity status, body size, and body composition with reference to health.
Antoine-Laurent Lavoisier was the first to experiment with the measurement of during exercise in the 1770s, but it is Hill and Lupton (1923), who introduced the concept of a near-linear relationship between and running speed until, despite an increase in running speed, a plateau in emerges at the point of maximal . In the first laboratory-based study of boys Robinson (1938) ran 6–17-year-olds on a treadmill at a speed of 7 miles⋅h–1 up an 8.6% gradient until they were “exhausted” and reported their as “maximal.” In his seminal study of boys and girls, Åstrand (1952) criticized Robinson’s (1938) methodology and adopted a more rigorous discontinuous, incremental exercise protocol over several days. He noted that the plateau proposed by Hill and Lupton (1923) was found in only 50% of schoolchildren. This phenomenon was subsequently confirmed in large studies with both pre-pubertal (Armstrong et al., 1995) and pubertal (Armstrong et al., 1991) youth but generally ignored for decades. When it was addressed and the term peak introduced (Armstrong and Davies, 1981) scientific journals confused understanding of youth aerobic fitness for several years by often rejecting papers reporting peak on the basis that maximal values were not attained.
Peak is now recognized as the “gold standard” measure of youth aerobic fitness but researchers continue to wrestle with factors related to its rigorous determination. In cardiopulmonary exercise tests children and adolescents normally exercise to voluntary exhaustion but there is no way to confirm, in the single tests typical of most studies, whether an individual has delivered a maximal effort. The experience of the testing team, supported by subjective criteria of intense effort (e.g., facial flushing, sweating, hyperpnoea, and unsteady gait), is critical in deciding whether a maximal value has been attained. However, to verify efforts as maximal, secondary criteria such as pre-set (and often submaximal) values of heart rate (HR), respiratory exchange ratio, and blood lactate accumulation at the termination of exercise are widely used. However, all secondary criteria exhibit large individual variations and are exercise protocol and ergometer dependent (Armstrong and McManus, 2017). The growing tendency to rely on what are clearly submaximal criteria such as HR ≥ 85% of predicted maximum to confirm maximal values has confused understanding of both the development of aerobic fitness and its purported relationship with other health-related variables. Barker et al. (2011) reported that terminating a test with secondary criteria can underestimate a child’s “true” peak by ∼10–22%.
Both treadmills and cycle ergometers are used routinely in pediatric exercise laboratories. Due to the greater muscle mass, enhanced venous return, higher stroke volume (SV), and reduced peripheral resistance during running, mean treadmill-determined values are ∼11–14% higher than those determined on a cycle ergometer. Ergometer-dependent differences in peak at specific ages vary with sex, age, maturity status, and morphological covariates (Armstrong and Welsman, 2019a) but some reviewers have confused understanding of the development of aerobic fitness by combining treadmill- and cycle ergometer-determined peak values (e.g., Bar-Or and Rowland, 2004). Other authors have added to the confusion by “correcting” for ergometer differences by multiplying cycle ergometer values by fixed percentages regardless of sex, age, or maturity-status (e.g., Stavnsbo et al., 2018) or assuming that increasing cycle ergometer values “by ∼2–3 mL⋅kg–1⋅min–1 would make them equivalent to values obtained by a treadmill protocol” (Aadland et al., 2019, p. 248).
Robinson (1938) initially reported boys’ “maximal” in L⋅min–1 before “referring them to body weight” (p. 280), analyzing his data in ratio with body mass (i.e., in mL⋅kg–1⋅min–1), and initiating an approach for “controlling” for growth that has confused pediatric exercise physiology for over 80 years.
Tanner (1949) unequivocally established that expressing peak in ratio with body mass was fallacious. Subsequent reviews have explained the statistical assumptions underlying ratio scaling of peak with body mass and demonstrated that they are seldom (if ever) met (Welsman and Armstrong, 2008, 2019). Yet the vast majority of pediatric exercise studies still interpret peak in ratio with body mass and reports of spurious correlations with indicators of cardiovascular health are common (Mintjens et al., 2018). Purported relationships between ratio-scaled peak and other health-related variables have confused the association of youth aerobic fitness with current and future health. For example, any relationship between cardiovascular risk factors in overweight/obese youth with ratio-scaled peak is more likely to reflect overweight/obese status than aerobic fitness (Loftin et al., 2016).
In practice, ratio-scaled peak favors lighter (e.g., clinically underweight or delayed maturing) youth and penalizes heavier (e.g., overweight or advanced maturing) youth. Literature reviews (e.g., Krahenbuhl et al., 1985) and textbooks (e.g., Bar-Or and Rowland, 2004) reporting ratio-scaled peak have confused understanding of developmental exercise physiology for decades. For example, peak data ratio-scaled with body mass indicates that boys’ aerobic fitness decreases slightly or remains unchanged from 10 to 18 years, whilst in girls a progressive decline is apparent over the same time scale. However, when body mass is appropriately controlled using log-linear regression boys’ peak increases with age and girls’ peak increases at least until 13 or 14 years and then levels-off (Welsman et al., 1996). Similarly, ratio-scaled data indicate that once body mass is controlled for maturity status has no effect on peak (e.g., Fahey et al., 1979) whereas allometric (log-linear regression) scaling has demonstrated positive effects of maturity status in addition to those of age and body mass in both boys and girls (Armstrong et al., 1998).
A recent resurgence of interest in estimating/predicting peak from 20-m shuttle run test (20mSRT) performance, has confused understanding of youth aerobic fitness (Armstrong and Welsman, 2018; Welsman, 2019). The 20mSRT is not a measure of aerobic fitness but a function of willingness to run between two lines 20 m apart whilst keeping pace with audio signals which require the running speed to increase each minute until participants are unwilling or unable to maintain the pace. The number of shuttles/stages completed is converted into an estimate of peak in ratio with body mass through one of at least 17 published prediction equations (Tomkinson et al., 2017). A recent meta-analysis reported that over half of published correlation coefficients between 20mSRT scores and “true” peak explain less than 50% of the total variance in peak and concluded, “testers must be aware that the performance score of the 20MSR test is simply estimation and not a direct measure of cardiorespiratory fitness” (Mayorga-Vega et al., 2015, p. 545). The capacity for confusion created by uncritical application of 20mSRT data to relationships with indicators of health is revealed by the 95% range for a “true” peak value estimated from 20mSRT performance being ∼10 mL⋅kg–1⋅min–1 or ∼24% (Tomkinson et al., 2019a).
Further confusion has arisen over the introduction and promotion of “cardiometabolic cut-points” “to define children with poor cardiometabolic health” (Aadland et al., 2019, p. 240) and “clinical red flags” to identify “children and adolescents who may benefit from primary and secondary cardiovascular prevention programming” (Ruiz et al., 2016, p. 1451). The validity of these “cut points” and “clinical red flags,” both based on ratio-scaled peak , is also challenged through them being derived from combined cycle ergometer- and treadmill-determined peak values with a fixed 5% added to cycle ergometer values (Stavnsbo et al., 2018); an amalgam of data from treadmill- and cycle ergometer (+5%)-determined peak and peak predicted from 20mSRTs (Aadland et al., 2019); and solely from 20mSRT predictions of peak (Ruiz et al., 2016). None of the proposed “cut points” consider maturity status. “Clinical red flags” also take no account of age with the indefensible assumption that a pre-pubertal 8-year-old is comparable to a post-pubertal 18-year-old with the same peak ratio-scaled with body mass.
The laboratory measurement of peak has been progressively developed and refined as new technology has replaced the classic Douglas bag method initially with mixing chambers and more recently with breath-by-breath analyses. The importance of appropriate exercise test protocols, ergometers, breathing interfaces, size of components of respiratory gas collection systems, and sampling intervals during growth and maturation cannot be overemphasized and all methodology, apparatus, and calibration techniques should be carefully reported (see McManus and Armstrong, 2017; Falk and Dotan, 2019 for comprehensive reviews).
The typical error of youth peak rigorously determined in three tests each a week apart is in our hands ∼4% (Welsman et al., 2005). To increase confidence in obtaining a “true” peak in a single session an initial exercise test can be confirmed with a validation test. For example, a cycle ergometer ramp test to exhaustion followed ∼15 min later by a validation test consisting of a 2 min warm-up before a step change to 105% of the peak power elicited at the end of the initial test. On the few occasions (in our hands <5%) that the peak is higher than in the initial test the validation test can be repeated at 110% of peak power following full recovery (Barker et al., 2011). This protocol is facilitated by children’s ability to recover from heavy exercise faster than adults (Ratel et al., 2006; Armstrong, 2019).
Youth peak has been comprehensively documented but flawed experimental designs, statistical analyses, and data interpretation have limited insights into the development of aerobic fitness. Use of equipment and exercise protocols designed for adults, small sample sizes, combining of data from boys and girls, only reporting peak ratio-scaled with body mass, and serious concerns over whether true maximal values have been attained make peak data from young children difficult to interpret (Armstrong and Welsman, 1994). The focus herein will therefore be on the more secure database of 10–18-year-olds.
The snapshot moments in time reflected by cross-sectional studies provide few insights and rigorous examination of the development of aerobic fitness requires longitudinal studies (Armstrong and McManus, 2017). Longitudinal data based on 1057 treadmill determinations of peak have revealed that aerobic fitness is significantly correlated with age (r = 0.78, boys and 0.64, girls), body mass (r = 0.89, boys and 0.83, girls), and fat-free mass (FFM) (r = 0.94, boys and 0.87, girls) (Armstrong and Welsman, 2019b). As can be seen in Figure 1, there is a near-linear increase in boys’ peak from 10 to 18 years. In girls, a near-linear increase in peak until ∼13–14 years of age is followed by a leveling-off from ∼14 to 18 years. Boys’ peak almost doubles from 10 to 18 years while girls’ values increase by ∼50% over the same time period. Pre-pubertal boys’ peak values are, on average, ∼12% higher than those of similar aged pre-pubertal girls and the mean sex difference in peak increases as young people progress through adolescence reaching ∼50% in post-pubertal 18-year-olds. Figure 1 illustrates the relationships of body mass and FFM with peak with sex differences evident throughout the age range. Girls’ peak in relation with body mass tends to taper-off from ∼60 kg. Body mass includes both fat mass which is metabolically inert (Goran et al., 2000) and FFM which reflects the active muscle. The relationship of peak with FFM (estimated from the equations of Slaughter et al., 1988) is remarkably linear from 10 to 18 years in both sexes (Armstrong and Welsman, 2019b).
Figure 1. Peak oxygen uptake by age, body mass, and fat free mass in 10–18-year-old girls and boys: Figure founded on 1057 determinations of peak oxygen uptake, age, body mass, and fat free mass; girls (n = 501), boys (n = 556). Data from Armstrong and Welsman (2019b).
In a parallel longitudinal study 72 boys and 64 girls also had their peak determined on a cycle ergometer. The pattern of peak relationships with age, body mass, and FFM were similar to those on a treadmill although the magnitude of the covariates varied. Mean treadmill values of peak were significantly (p < 0.05) higher in boys on each testing occasion but the percentage difference varied with age, peaking at 13 years. Moreover, some individuals in both sexes demonstrated higher values on a cycle ergometer than a treadmill on at least one test occasion further illustrating the folly of predicting treadmill-determined peak by adding a fixed percentage to cycle ergometer values regardless of age, sex, and maturity status (Armstrong and Welsman, 2019a).
Collectively these longitudinal data also unequivocally show the fallacy of ratio scaling peak with body mass, regardless of whether it is determined during mass-supporting or mass- supported exercise. Statistical assumptions underlying ratio scaling include a perfect correlation (i.e., r = 1.0) between peak and body mass which was not met and an allometric exponent of 1.0 for body mass, a value which fell outside the 95% confidence limits. Moreover, significant negative correlations between ratio-scaled peak and body mass demonstrated that body mass was not controlled for by ratio scaling (Armstrong and Welsman, 2019a,b).
The application of multilevel regression modeling to trained (Nevill et al., 1998) and untrained youth (Armstrong et al., 1999) and the technique’s on-going refinement (Rasbash et al., 2018) has enabled multiple, individual growth trajectories to be examined in relation to the development of aerobic fitness. The effects of sex, age, maturity status, body size, and body composition on peak can be partitioned concurrently within an allometric framework to provide a sensitive interpretation of youth aerobic fitness. Armstrong and Welsman (2019a, b) adopted this approach and explored the development of peak through a series of models which are shown in Table 1. In contrast to traditional ratio-scaled interpretations, the initial models 1.1 (girls) and 2.1 (boys) show that with body mass controlled for, peak increases with age in both sexes. The negative age2 term indicates that the size of the age effect reduces as the rate of growth decreases. Stature was not a significant (p > 0.05) covariate in any of the models. In conflict with ratio-scaled data, the addition of maturity status, in the form of the stages of pubic hair described by Tanner (1962), showed each stage to have a positive effect on peak independent of those from body mass and age (models 1.2 and 2.2). The introduction of sum of triceps and subscapular skinfolds to act with body mass as a surrogate of FFM (Roemmich et al., 1997) resulted in the effects of maturity status being negated but age and age2 remained significant covariates. These models (1.3 and 2.3) were the best statistical fit (p < 0.05) of the data and demonstrate the powerful effects of FFM on the development of aerobic fitness.
Boys’ FFM increases, on average, by ∼90% from 11 to 16 years. It is, however, maturation which drives changes in FFM. This is evidenced by percentage changes in FFM being at their zenith around the time of peak height velocity (PHV) when FFM increases by ∼83% over the period 2 years pre-PHV to 2 years post-PHV. Girls’ FFM increases by ∼40% over the same age range with the greatest increase (∼31%) occurring over a 2 year period centered on PHV, before it levels-off in accord with the development of peak (Armstrong, 2019; Baxter-Jones et al., 2003).
For ethical and technological reasons the physiological mechanisms underpinning the development of youth aerobic fitness remain to be fully elucidated (Warburton and Bredin, 2017). HR at peak is independent of sex, age, maturity status, body size, and body composition at least until the late teens (Rowland, 2017). Developmental changes in peak are therefore a function of increases in SV and/or arterio-venous oxygen difference. Both oxygen delivery and oxygen utilization are facilitated by increases in FFM (Armstrong and McManus, 2017). Data on the development of arterio-venous oxygen difference are not available but the positive effects of FFM on the longitudinal development of SV has been demonstrated with multilevel allometric modeling (Armstrong and Welsman, 2002). Moreover, it has been reported that sex differences in SV disappear when it is expressed relative to allometrically scaled FFM (Vinet et al., 2003).
The evidence base outlined herein shows that is simplistic to describe aerobic fitness in a cross-sectional snapshot in relation to a single morphological covariate. Analyses of the development of aerobic fitness must take account not only of age but also of sex-specific, maturation-driven changes in FFM which are governed by individual biological clocks. 20mSRT predictions of peak have confused our understanding of youth aerobic fitness with proposals to establish international age-related norms (Tomkinson et al., 2019b), to provide “reference standards” for children as young as 2 years (Cadenas-Sanchez et al., 2019), to initiate fitness surveillance programs (Lang et al., 2018), and to promote inter-country comparisons of “who are the fittest?” (Lang et al., 2016). Moreover, youth who raise a “clinical red flag” or cross an age-related “cardiometabolic cut point” are more likely to be suffering from what Tanner (1949) identified as, “no more formidable a disease than statistical artifact” (p. 3) than warranting medical attention.
Peak increases in accord with sex-specific, concurrent changes in age- and maturity status-driven morphological covariates with the timing and tempo of changes specific to individuals. For clarity future studies should ensure that they address youth aerobic fitness and its relationship with present and future health with this firmly in mind.
Both authors conceived the review, drafted the manuscript, and reviewed and approved the final version of the manuscript.
JW was funded by the Darlington Foundation Trust.
The authors declare that the research was conducted in the absence of any commercial or financial relationships that could be construed as a potential conflict of interest.
Aadland, E., Anderssen, S. A., Andersen, L. B., Resaland, G. K., Kolle, E., and Steene-Johannessen, J. (2019). Aerobic thresholds to define poor metabolic health in children. Scand. J. Med. Sci. Sports 29, 240–250. doi: 10.1111/sms.13330
Armstrong, N., and Davies, B. (1981). An ergometric analysis of age-group swimmers. Br. J. Sports Med. 15, 20–26. doi: 10.1136/bjsm.15.1.20
Armstrong, N., Kirby, B. J., McManus, A. M., and Welsman, J. R. (1995). Aerobic fitness of prepubescent children. Ann. Hum. Biol. 22, 427–441. doi: 10.1080/03014469500004102
Armstrong, N., and McManus, A. M. (2017). “‘Aerobic fitness’,” in Oxford Textbook of Children’s Sport and Exercise Medicine, eds N. Armstrong and W. van Mechelen (Oxford: Oxford University Press), 161–180.
Armstrong, N., and Welsman, J. (2002). Cardiovascular responses to submaximal treadmill running in 11 to 13 year olds. Acta Paediatr. 91, 125–131. doi: 10.1080/080352502317285081
Armstrong, N., and Welsman, J. (2018). Twenty-metre shuttle run: (mis)representation, (mis)interpretation and (mis)use. Br. J. Sports Med. doi: 10.1136/bjsports-2018-100082 [Epub ahead of print].
Armstrong, N., and Welsman, J. (2019a). Development of peak oxygen uptake from 11-16 years determined using both treadmill and cycle ergometry. Eur. J. Appl. Physiol. 119, 801–812. doi: 10.1007/s00421-019-04071-3
Armstrong, N., and Welsman, J. (2019b). Sex-specific longitudinal modeling of youth peak oxygen uptake. Pediatr. Exerc. Sci. 31, 204–212. doi: 10.1123/pes.2018-0175
Armstrong, N., and Welsman, J. R. (1994). Assessment and interpretation of aerobic fitness in children and adolescents. Exerc. Sport Sci. Rev. 22, 435–476. doi: 10.1249/00003677-199401000-00016
Armstrong, N., Welsman, J. R., and Kirby, B. J. (1998). Peak oxygen uptake and maturation in 12-year-olds. Med. Sci. Sports Exerc. 30, 165–169. doi: 10.1097/00005768-199801000-00023
Armstrong, N., Welsman, J. R., Nevill, A. M., and Kirby, B. J. (1999). Modeling growth and maturation changes in peak oxygen uptake in 11-13 yr olds. J. Appl. Physiol. 87, 2230–2236. doi: 10.1152/jappl.1999.87.6.2230
Armstrong, N., Williams, J., Balding, J., Gentle, P., and Kirby, B. (1991). Peak oxygen uptake of British children with reference to age, sex and sexual maturity. Eur. J. Appl. Physiol. 62, 369–375. doi: 10.1007/bf00634975
Åstrand, P. O. (1952). Experimental Studies of Physical Working Capacity in Relation to Sex and Age. Copenhagen: Munksgaard.
Barker, A. R., Williams, C. A., Jones, A. M., and Armstrong, N. (2011). Establishing maximal oxygen uptake in young people during a ramp test to exhaustion. Br. J. Sports Med. 45, 498–503. doi: 10.1136/bjsm.2009.063180
Bar-Or, O., and Rowland, T. W. (2004). Pediatric Exercise Medicine. Champaign, IL: Human Kinetics, 4–7.
Baxter-Jones, A. D. G., Mirwald, R. L., McKay, H. A., and Bailey, D. A. (2003). A longitudinal analysis of sex differences in bone mineral accrual in healthy 8-19-year-old boys and girls. Ann. Hum. Biol. 30, 160–175. doi: 10.1080/0301446021000034642
Cadenas-Sanchez, C., Intemann, T., Labayen, I., Peinado, A. B., Vidal-Conti, J., Sanchis-Moysie, J., et al. (2019). Physical fitness reference standards for preschool children: the PREFIT project. J. Sci. Med. Sport 22, 430–437. doi: 10.1016/j.jsams.2018.09.227
Fahey, T. D., Del Valle-Zuris, A., Oehlsen, G., Trieb, M., and Seymour, J. (1979). Pubertal stage differences in hormonal and hematological responses to maximal exercise in males. J. Appl. Physiol. 46, 823–827. doi: 10.1152/jappl.1979.46.4.823
Falk, B., and Dotan, R. (2019). Measurement and interpretation of maximal aerobic power in children. Pediatr. Exerc. Sci. 31, 144–151. doi: 10.1123/pes.2018-0191
Goran, M., Fields, D. A., Hunter, G. R., Herd, S. L., and Weinster, R. L. (2000). Total body fat does not influence maximal aerobic capacity. Int. J. Obes. 24, 841–848. doi: 10.1038/sj.ijo.0801241
Hill, A. V., and Lupton, H. (1923). Muscular exercise, lactic acid and the supply and utilization of oxygen. Q. J. Med. 16, 135–171. doi: 10.1093/qjmed/os-16.62.135
Krahenbuhl, G. S., Skinner, J. S., and Kohrt, W. M. (1985). Developmental aspects of maximal aerobic power in children. Exerc. Sports Sci. Rev. 13, 503–538.
Lang, J. J., Tomkinson, G. R., Janssen, I., Ruiz, J. R., Ortega, F. B., Léger, L., et al. (2018). Making a case for cardiorespiratory fitness surveillance among children and youth. Exerc. Sport Sci. Rev. 46, 66–75.
Lang, J. J., Tremblay, M. S., Leger, L., Olds, T., and Tomkinson, G. R. (2016). International variability in 20m shuttle run performance in children and youth: who are the fittest from a 50-country comparison? A systematic literature review with pooling of aggregate results. Br. J. Sports Med. 52:276. doi: 10.1136/bjsports-2016-096224
Loftin, M., Sothern, M., Abe, T., and Bonis, M. (2016). Expression of peak in children and youth with special reference to allometric scaling. Sports Med. 46, 1451–1460 doi: 10.1007/s40279-016-0536-7
Mayorga-Vega, D., Aguiler-Soto, P., and Viciana, J. (2015). Criterion-related validity of the 20-m shuttle run test for estimating cardiorespiratory fitness: a meta-analysis. J. Sports Sci. Med. 14, 536–547.
McManus, A. M., and Armstrong, N. (2017). “‘Maximal oxygen uptake’,” in Cardiopulmonary Exercise Testing in Children and Adolescents, ed. T. W. Rowland (Champaign, IL: Human Kinetics), 79–93.
Mintjens, S., Menting, M. D., Daams, J. G., van Poppel, M. N. M., Roseboom, T. J., and Gemke, R. J. B. J. (2018). Cardiorespiratory fitness in childhood and adolescence affects future cardiovascular risk factors: a systematic review of longitudinal studies. Sports Med. 48, 2577–2605. doi: 10.1007/s40279-018-0974-5
Nevill, A. M., Holder, R. L., Baxter-Jones, A., Round, J. M., and Jones, D. A. (1998). Modelling developmental changes in strength and aerobic power in children. J. Appl. Physiol. 84, 963–970. doi: 10.1152/jappl.1998.84.3.963
Rasbash, J., Steele, F., Browne, W. J., and Goldstein, H. (2018). A User’s Guide to MLwiN Version 3.02. Bristol: University of Bristol Centre for Multilevel Modelling, 2018.
Ratel, S., Duche, P., and Williams, C. A. (2006). Muscle fatigue during high-intensity exercise in children. Sports Med. 36, 1031–1065. doi: 10.2165/00007256-200636120-00004
Robinson, S. (1938). Experimental studies of physical fitness in relation to age. Arbeitsphysiologie 10, 251–323. doi: 10.1007/bf02011412
Roemmich, J. N., Clark, P. A., Weltman, A., and Rogol, A. D. (1997). Alterations in growth and body composition during puberty. I. comparing multicompartment body composition models. J. Appl. Physiol. 83, 927–935. doi: 10.1152/jappl.1997.83.3.927
Rowland, T. W. (2017). “‘Cardiovascular function’,” in Oxford Textbook of Children’s Sport and Exercise Medicine, eds N. Armstrong and W. van Mechelen (Oxford: Oxford University Press), 147–159.
Ruiz, J. R., Cavero-Redondo, I., Ortega, F. B., Welk, G. J., Andersen, L. B., and Martinez-Vizcaino, V. (2016). Cardiorespiratory fitness cut points to avoid cardiovascular disease risk in children and adolescents: what level of fitness should raise a red flag? A systematic review and meta-analysis. Br. J. Sports Med. 50, 1451–1458. doi: 10.1136/bjsports-2015-095903
Slaughter, M. H., Lohman, T. G., Boileau, R. A., Horswill, C. A., Stillman, R. J., Van Loan, M. D., et al. (1988). Skinfold equations for estimation of body fatness in children and youth. Hum. Biol. 60, 709–723.
Stavnsbo, M., Resaland, G. K., Anderssen, S. A., Steene-Johannessen, J., Domazet, S. L., Skrede, T., et al. (2018). Reference values for cardiometabolic risk scores in children and adolescents: suggesting a common standard. Atherosclerosis 278, 299–306. doi: 10.1016/j.atherosclerosis.2018.10.003
Tanner, J. M. (1949). Fallacy of per-weight and per-surface area standards and their relation to spurious correlation. J. Appl. Physiol. 2, 1–15. doi: 10.1152/jappl.1949.2.1.1
Tomkinson, G. R., Lang, J. J., Blanchard, J., Leger, L., and Tremblay, M. S. (2019a). The 20-m shuttle run: assessment and interpretation of data in relation to youth aerobic fitness and health. Pediatr. Exerc. Sci. 31, 152–163. doi: 10.1123/pes.2018-0179
Tomkinson, G. R., Lang, J. J., and Tremblay, M. (2019b). Temporal trends in the cardiorespiratory fitness of children and adolescents representing 19 high-income and upper middle-income countries between 1981 and 2014. Br. J. Sports Med. 53, 478–486. doi: 10.1136/bjsports-2017-097982
Tomkinson, G. R., Lang, J. J., Tremblay, M., Dale, M., LeBlanc, A. G., Belanger, K., et al. (2017). International normative 20 m shuttle run values from 1 142 026 children and youth representing 50 countries. Br. J. Sports Med. 51, 1545–1554. doi: 10.1136/bjsports-2016-095987
Vinet, A., Mandigout, S., Nottin, S., Nguyen, L., Lecoq, A.-M., Courteix, D., et al. (2003). Influence of body composition, hemoglobin concentration, and cardiac size and function on gender differences in maximal oxygen uptake in prepubertal children. Chest 124, 1494–1499. doi: 10.1378/chest.124.4.1494
Warburton, E. R., and Bredin, S. R. (2017). “‘Cardiac output measurement techniques’,” in Cardiopulmonary Exercise Testing in Children and Adolescents, ed. T. W. Rowland (Champaign, IL: Human Kinetics), 107–118.
Welsman, J. (2019). Reflecting on field performance tests of pediatric aerobic fitness: after 30 years it really is time to move on. Pediatr. Exerc. Sci. 31, 191–193. doi: 10.1123/pes.2019-0034
Welsman, J., and Armstrong, N. (2019). Interpreting aerobic fitness in youth: the fallacy of ratio scaling. Pediatr. Exerc. Sci. 32, 184–190. doi: 10.1123/pes.2018-0141
Welsman, J. R., and Armstrong, N. (2008). “Scaling for size: relevance to understanding the effects of growth on performance’,” in The Young Athlete, eds H. Hebestreit and O. Bar-Or (Oxford: Blackwell), 50–62. doi: 10.1002/9780470696255.ch5
Welsman, J. R., Armstrong, N., Kirby, B. J., Nevill, A. M., and Winter, E. M. (1996). Scaling peak for differences in body size. Med. Sci. Sports Exerc. 28, 259–265. doi: 10.1097/00005768-199602000-00016
Keywords: aerobic fitness, assessment, clinical red flags, fat free mass, multilevel allometric modeling, peak oxygen uptake, scaling, youth
Citation: Armstrong N and Welsman J (2019) Clarity and Confusion in the Development of Youth Aerobic Fitness. Front. Physiol. 10:979. doi: 10.3389/fphys.2019.00979
Received: 30 May 2019; Accepted: 11 July 2019;
Published: 30 July 2019.
Edited by:
Filipe Manuel Clemente, Polytechnic Institute of Viana do Castelo, PortugalReviewed by:
João Paulo Brito, Polytechnic Institute of Santarém, PortugalCopyright © 2019 Armstrong and Welsman. This is an open-access article distributed under the terms of the Creative Commons Attribution License (CC BY). The use, distribution or reproduction in other forums is permitted, provided the original author(s) and the copyright owner(s) are credited and that the original publication in this journal is cited, in accordance with accepted academic practice. No use, distribution or reproduction is permitted which does not comply with these terms.
*Correspondence: Neil Armstrong, Ti5Bcm1zdHJvbmdAZXhldGVyLmFjLnVr
Disclaimer: All claims expressed in this article are solely those of the authors and do not necessarily represent those of their affiliated organizations, or those of the publisher, the editors and the reviewers. Any product that may be evaluated in this article or claim that may be made by its manufacturer is not guaranteed or endorsed by the publisher.
Research integrity at Frontiers
Learn more about the work of our research integrity team to safeguard the quality of each article we publish.