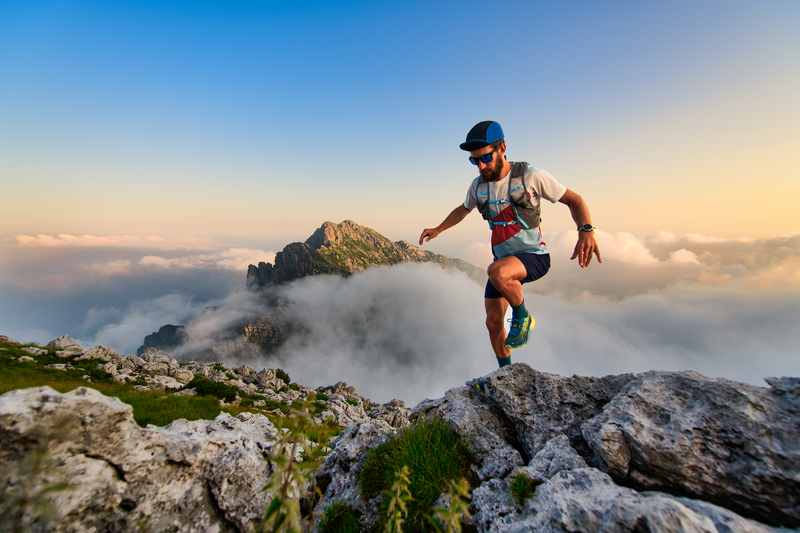
95% of researchers rate our articles as excellent or good
Learn more about the work of our research integrity team to safeguard the quality of each article we publish.
Find out more
SYSTEMATIC REVIEW article
Front. Physiol. , 22 March 2019
Sec. Exercise Physiology
Volume 10 - 2019 | https://doi.org/10.3389/fphys.2019.00262
Endurance is not only a key factor in many sports but endurance-related variables are also associated with good health and low mortality. Twin and family studies suggest that several endurance-associated traits are ≈50% inherited. However, we still poorly understand what DNA sequence variants contribute to endurance heritability. To address this issue, we conducted a systematic review to identify genes whose experimental loss or gain-of-function increases endurance capacity in mice. We found 31 genes including two isoforms of Ppargc1a whose manipulation increases running or swimming endurance performance by up to 1800%. Genes whose gain-of-function increases endurance are Adcy5, Adcy8, Hk2, Il15, Mef2c, Nr4a3, Pck1 (Pepck), Ppard, Ppargc1a (both the a and b isoforms of the protein Pgc-1α), Ppargc1b, Ppp3ca (calcineurin), Scd1, Slc5a7, Tfe3, Tfeb, Trib3 & Trpv1. Genes whose loss-of-function increases endurance in mice are Actn3, Adrb2, Bdkrb2, Cd47, Crym, Hif1a, Myoz1, Pappa, Pknox1, Pten, Sirt4, Thbs1, Thra, and Tnfsf12. Of these genes, human DNA sequence variants of ACTN3, ADCY5, ADRB2, BDKRB2, HIF1A, PPARD, PPARGC1A, PPARGC1B, and PPP3CA are also associated with endurance capacity and/or VO2max trainability suggesting evolutionary conservation between mice and humans. Bioinformatical analyses show that there are numerous amino acid or copy number-changing DNA variants of endurance genes in humans, suggesting that genetic variation of endurance genes contributes to the variation of human endurance capacity, too. Moreover, several of these genes/proteins change their expression or phosphorylation in skeletal muscle or the heart after endurance exercise, suggesting a role in the adaptation to endurance exercise.
Endurance is a key trait in many sports such as marathon running and triathlon. Endurance is also associated with health as a high endurance capacity is associated with fewer cardiovascular events and reduced all-cause mortality (Kodama et al., 2009). In rats, selection for low endurance capacity is associated with more cardiovascular risk factors than selection for high endurance capacity suggesting a direct link between endurance capacity and disease risk (Wisloff et al., 2005).
Endurance capacity is a multi-factorial trait that depends on several sub-traits and organ systems:
(1) Aerobic capacity (VO2max) is influenced by the maximal cardiac output and by the oxygen transport capacity of the blood, and blood volume (Bergh et al., 2000; Lundby et al., 2017).
(2) Skeletal muscle endurance has been linked to type I and II subtype muscle fiber proportions (Costill et al., 1976), muscle capillary density (Brodal et al., 1977), mitochondrial and other metabolic enzyme activities (Gollnick and Saltin, 1983) as well as the glycogen concentration of the exercising muscles (Bergstrom et al., 1967).
(3) Mechanical efficiency describes how much chemical energy is converted into mechanical power (Bassett and Howley, 2000). Efficiency depends on many factors including body weight and height (Maldonado et al., 2002).
(4) Mental endurance depends on the nervous system and is defined as fatigue resistance during prolonged periods of demanding cognitive activity (Van Cutsem et al., 2017).
In relation to human endurance, two important questions are: How much is endurance inherited? What DNA sequence variants affect endurance capacity? Classical genetic studies suggest that maximal aerobic performance variables (i.e., VO2max, physical working capacity or threshold values) are between 38 and 94% inherited (Peeters et al., 2009). In the Heritage Study, Bouchard et al. estimated that the VO2max was 50% inherited (Bouchard et al., 1998), and that the VO2max trainability was 47% inherited (Bouchard et al., 1999). Similarly, the muscle fiber distribution was estimated to be ≈45% inherited (Simoneau and Bouchard, 1995). Collectively, especially the Heritage study data suggest that the variation of major human endurance-related traits depends probably to ≈50% on DNA sequence variation [i.e., genetics, Simoneau and Bouchard, 1995; Bouchard et al., 1998, 1999)] implying that ≈50% is dependent on environmental factors such as endurance training and nutrition.
We still incompletely understand the genetics of human endurance and some researchers are even skeptical about the importance of genetics especially in relation to the VO2max. A recent review for endurance-related DNA variants in humans highlighted 93 endurance-associated DNA variants (Ahmetov et al., 2016). Furthermore, a systematic search identified 97 DNA variants that are associated with VO2max/peak trainability (Williams et al., 2017). Whilst the effect size of many endurance-associated polymorphisms is small, the effect of a rare EPOR DNA variant on the haematocrit and presumably VO2max is large, given that one carrier was an Olympic gold medalist in cross country skiing (de la Chapelle et al., 1993). More recently, genome-wide association studies (GWAS) have added to our knowledge of the genetics of human endurance. Here, Rankinen et al. (2016) found in 1520 elite endurance athletes and 2760 controls, no common single nucleotide polymorphism (SNP) profile that distinguishes elite endurance athletes from ethnicity-matched controls (only one SNP near the GALNTL6 locus was significant across all studies) (Rankinen et al., 2016). Another study in ≈40,000 individuals, and replication in ≈27,000, identified 30 loci that associated with heart rate change at the onset and recovery after exercise. Many of the loci included genes linked to the autonomic nervous system, a known regulator of heart rate (Ramírez et al., 2018). Finally, a study of ≈91,000 individuals identified 14 loci that associated with device-measured physical activity and sleep duration of which several are linked to the central nervous system (Doherty et al., 2018). Given that few physiologically plausible genetic associations have been discovered for endurance, Lundby et al. (2017) question whether “DNA variants in the key physiological pathways for VO2max […] will be identified for both average individuals and also elite endurance athletes.” Finally, in his bestselling science book Malcolm Gladwell concludes that 10,000 h is all it takes to achieve expert performance in various fields including sports, leaving little room for genetics or talent (Gladwell, 2008). Thus whilst humans seem to have genetically evolved as an endurance running species (Bramble and Lieberman, 2004), the genetic contribution to the large variation of endurance traits in current human populations is still unclear.
Insights into the genetics of endurance come from studies with good statistical power into the genetics of body height. Body height is not only a ≈80% inherited trait (Silventoinen et al., 2003) but also influences endurance performance, e.g., in rowing, which is an endurance sport (Maldonado et al., 2002). GWAS involving hundreds of thousands of individuals have revealed that the variation of body height is influenced by thousands of SNPs. For example, ≈9,500 SNPs are estimated to explain 29% of human body height variation (Wood et al., 2014). Human body height is additionally influenced by rare, large-effect size mutations of genes such as mutations of AIP that cause gigantism (Chahal et al., 2011) or mutations of genes such as FGFR3 that can cause dwarfism (Foldynova-Trantirkova et al., 2012). Because body height is only one of many endurance performance-limiting factors and already influenced by thousands of DNA variants, it seems likely that even more common DNA sequence variants with small effect sizes and some rarer DNA variants with larger effect sizes combine to explain the effect of DNA sequence variation on human endurance capacity.
The current challenge for molecular exercise physiologists is to start to draw an overall picture of the genetics of human endurance capacity. This picture should both give an idea of the likely number of endurance capacity-influencing DNA variants and identify especially those DNA variants that have a major effect on endurance capacity. A special challenge is to explain the genetics of highly talented elite endurance athletes such as East African runners. Do these athletes share otherwise rare DNA variants with a high effect size as is the case in populations that live at high altitude (Bigham, 2016)? Or do they carry thousands of endurance-promoting DNA variants with small effect sizes? Do the DNA variants mainly affect exons or do they cause variation of regulatory DNA elements?
To identify genes and alleles that can have a major effect on endurance it is especially useful to review the data of transgenic mouse studies (Garton et al., 2016). In transgenic mouse studies, genes are manipulated to produce a gain or loss-of-function of a gene. If this results in a measurable increase of endurance, then the gene is a candidate gene for human endurance capacity, too. The aim of this study was therefore to systematically search the literature for published studies where a gain or loss-of-function mutation of a gene increases endurance capacity in mice. A second aim of the study was to study the identified endurance genes further through bioinformatical analyses.
We conducted a systematic review using the PRISMA guidelines (Moher et al., 2009) and included all studies according to the participants, interventions, comparators and outcomes (PICO) process (Schardt et al., 2007). Its main aim was to identify genes whose gain or loss-of-function significantly increases endurance capacity in mice. We first searched the six English-language databases (Google Scholar, Bio Med, Scopus, PubMed, Science Direct, and Web of Science) using our systematic search strategy and used the following combination of search terms: (“mouse” OR “murine” OR “mouse model” OR “mice” OR “mice transgenic”) AND (“overexpression” OR “knock out” OR “knock in” OR “gene transfer techniques” OR “mutagenesis” OR “gene deletion” OR “gene manipulation”) AND [“endurance exercise” OR “swimming” OR “wheel running” OR “endurance capacity” OR “mPXT” (speed progress until exhaustion test in mice) OR “mGXT” (graded maximal exercise in mice)].
After eliminating duplicates, we examined the published studies in two stages: First, we reviewed results by title and abstract and then by full-text. At each step, we deleted studies that did not match with the review’s inclusion and exclusion criteria. We included studies in this review if they met the following criteria:
(1) The study needs to be published in a paper or in online peer-reviewed journal;
(2) Publication language must be English;
(3) Each study must show original empirical, primary data/evidence;
(4) Mice must be healthy, and gene manipulation is the only intervention;
(5) Increased endurance capacity must be reported as the distance or time achieved during an endurance exercise test (treadmill running, swimming, wheel-running).
(6) In case an endurance capacity-influencing gene was mentioned more than once, we only analyzed the paper where it was mentioned first.
We excluded studies based on the following criteria:
(1) Rat or in vitro study;
(2) No transgenesis, double mutation or long non-coding RNAs manipulation;
(3) Major pathological abnormalities result from the gene manipulation;
(4) Mice are older than 24 months;
(5) No statistically significant effect or no outcome measures;
(6) No wild type mice as controls;
(7) Use of an additional drug treatment or dietary supplement (we included studies where a transgene was induced, e.g., through doxycycline injection).
We illustrate our literature search in Supplementary Data S1.
We extracted the following information from each relevant study: author(s), gene name, protein name, method of gene manipulation, acclimated, exercise testing protocols, output measure, endurance capacity for control and transgenic mice, difference between control and transgenic mice as a percentage, age of the mice, mouse strain, additional measurements, and remarks (Supplementary Table S2). Sometimes, the output measure values were presented only in a bar graph and not as a number. In these cases, we manually measured and estimated the relative difference of mean values between controls and transgenic mice by using the bar height. Moreover, we adopted official gene names from the Universal Protein Resource (UniProt, NCBI) and the official gene names may differ from the gene or protein names that are used in the original papers.
To obtain more information about the identified endurance genes and the proteins that they encode, we asked several research questions and performed bioinformatical analyses to answer these questions. This information is summarized in Supplementary Data S3–S11.
Initially, we identified 2315 manuscripts with publication dates until January 2018. Based on the title or abstract we excluded 2171 studies. After that, 263 articles remained which we read fully for eligibility. We identified another 43 articles by reviewing the reference lists of the full-text articles or other sources. Finally, we read 144 full-text articles and analyzed 32 articles quantitatively. A PRISMA flowchart of our search and reading strategy is in the Supplementary Data S1. We used the information of this systematic literature search and several bioinformatical analyses to answer several research questions which are stated as headers below.
Our analysis revealed 31 genes/isoforms including two isoforms of Ppargc1a whose gain or loss-of-function increased endurance performance in mice. Specifically, we identified 19 genes [Adcy5, Adcy8, Hk2, Il15, Mef2c, Nr4a3, Pck1 (Pepck), Ppard, Ppargc1a (both the a and b isoforms of the mitochondrial biogenesis regulator Pgc-1α), Ppargc1b, Ppp3ca (calcineurin), Scd1, Slc5a7, Tfe3, Tfeb, Trib3, and Trpv1; Figure 1] whose gain-of-function increased endurance capacity in mice. We also found 14 genes (Actn3, Adrb2, Bdkrb2, Cd47, Crym, Hif1a, Myoz1, Pappa, Pknox1, Pten, Sirt4, Thbs1, Thra, Tnfsf12; Figure 2) whose loss-of-function increases endurance capacity. Collectively, we will refer to these genes as endurance genes for simplicity. The relative increase ranged from 12% for Pten to 1800% for Pck1 (Figures 1,2). To explain, if the mean value for the wildtype mouse was e.g., 100 units in an endurance test, then the transgenic mice achieved on average between 112 units (i.e., an increase of 12 units or %) or 1900 units (i.e., an increase by 1800 units or %), respectively. The detailed experimental design and endurance performance data for each transgenic and wildtype mouse pair are summarized in Table 1 and in full detail in Supplementary Data S2.
Figure 1. Genes whose gain-of-function increases running or swimming endurance in mice. Percentage increase in % were calculated by direct comparison the control animals. Finally, genes are plotted from high to low effect size.
Figure 2. Genes whose loss-of-function increases running or swimming endurance in mice. Percentage increase in % were calculated by direct comparison the control animals. Finally, genes are plotted from high to low effect size.
To study whether endurance genes also play a role in the variation of human endurance, we overlapped our set of endurance genes with a list of human genes where DNA variants associate with endurance (Ahmetov et al., 2016) and genes where DNA variants associate with VO2max trainability (Williams et al., 2017). Moreover, we searched for phenotypes for endurance genes reported in human GWAS by searching the human GWAS catalog. The first overlap analysis we screened the human homologs of the mouse genes. Here, we revealed that human endurance gene variants of ACTN3, ADRB2, BDKRB2, HIF1A, PPARD, PPARGC1A, PPARGC1B, and PPP3CA are also associated with human endurance (Ahmetov et al., 2016). Moreover, DNA variants linked to ADCY5, PPARD, and HIF1A are associated with VO2max trainability in humans (Williams et al., 2017). In a second step, we investigated whether the identified endurance genes were linked in GWAS to phenotypes that are potentially relevant for endurance performance. To do so, we performed a GWAS catalog search for each gene. This search revealed several associations between endurance genes and a several physiological and pathological human phenotypes (Supplementary Data S3). Associations of potential relevance for endurance performance include an association of PCK1 with the hemoglobin concentration (Astle et al., 2016), an association of PPARGC1A with the resting heart rate (Eppinga et al., 2016), an association of SCD with metabolic traits (Suhre et al., 2011) specifically blood levels of myristate (14:0)/myristoleate (14:1n5) (Shin et al., 2014), and an association of TFEB with left ventricular wall thickness (Wild et al., 2017). Collectively, these analyses demonstrate that some endurance genes are assocuated with human endurance-associated traits, too.
Next we used the ExAC browser to explore the extent to which the DNA sequence of human endurance gene exoms varies (Lek et al., 2016). This analysis revealed extensive genetic variation of endurance genes in humans. On average, each human homologue of an endurance gene had 174 missense DNA variants, 5 loss-of-function DNA variants and 11 copy number DNA variants. Additionally, for ACTN3, CRYM, TFE3, and THRA homozygous loss-of-function DNA variants were reported. For ACTN3 it is already known that a loss-of-function can be tolerated as ≈20% of the population are homozygous for a ACTN3 R577X variant (Yang et al., 2003). This and the results of GWAS s suggest a large amount of common or rare, functionally relevant DNA sequence variation in the human homologues of mouse endurance genes.
We have already mentioned that endurance is a trait that is determined by the function and interplay of several organ systems. These include skeletal muscle as the key force-generating and energy converting organ, the liver for glycogen storage and gluconeogenesis, the oxygen-delivering organs lung, heart, vasculature and blood as well as the brain due to its role in mental fatigue. To study the expression in resting human organs, we retrieved gene expression data from the GTEx Portal database (GTEx Consortium, 2015) and plotted this as a heat map (Figure 3 and Supplementary Data S5). This reveals that Myoz1 and Actn3 are selectively expressed in skeletal muscle whereas Pck1 is selectively expressed in the liver, at least at rest. In addition, several other genes, such as Sirt4, Ppargc1a, Il15Adcy8Bdkrb2, Pappa, and Slc5a7, are not expressed in these selected organs.
Figure 3. Heatmap illustrating the expression levels of endurance genes in transcripts per million (TPM) in some endurance exercise organs. If no expression value was given in GTEx Portal, we recorded the expression as “0” which signifies either no expression or no data.
Several genes may be expressed at low levels in resting human organs but may increase their expression in response to endurance exercise. One example is the gene Ppargc1a which encodes various isoforms of the mitochondrial biogenesis regulator Pgc-1α. The expression of Ppargc1a increases in response to endurance exercise both in mouse (Baar et al., 2002) and human skeletal muscle (Pilegaard et al., 2003). Moreover, proteins encoded by endurance genes may become phosphorylated after a bout of endurance exercise as can be demonstrated by phosphoproteomics (Hoffman et al., 2015). To test whether endurance genes/proteins change their expression or phosphorylation after a bout of endurance exercise, we re-analyzed published datasets (Vissing and Schjerling, 2014; Hoffman et al., 2015). These analyses reveal that PPARGC1A (encoding PGC-1α), NR4A3 which encodes a nuclear hormone receptor and THSB1, which encodes thrombospondin 1, are examples for genes that increase their expression 2.5 and 5 h in the vastus lateralis after cycling for 120 min at 60% of the VO2peak (Figure 4 and Supplementary Data S6; Vissing and Schjerling, 2014). In contrast, ACTN3 decreases its expression after human endurance exercise (Figure 4). Whilst the direction of the expression changes of PPARGC1A, NR4A3, and ACTN3 is consistent with the respective mouse phenotypes, THSB1 expression increases after endurance exercise but a global deletion of Thsp1 increases capillarity and exercise capacity in mice (Malek and Olfert, 2009). Thus, THSB1 expression after endurance exercise suggests that it promotes a reduced adaptation to endurance exercise.
Figure 4. Effect of human endurance exercise on the expression of PPARGC1A (A), NR4A3 (B), THSB1 (C), and ACTN3 (D) in the vastus lateralis 2.5 and 5 h after exercise (Vissing and Schjerling, 2014).
In addition, proteins encoded by ACTN3, ADRB2, MEF2C and TFEB were detected as phosphorylated proteins in vastus lateralis samples after a single bout of high-intensity cycle exercise at ≈90% of the maximal power output (Wmax) for ≈10 min (Supplementary Data S7). Of these proteins, TFEB phosphorylation significantly decreased from pre to post exercise (Hoffman et al., 2015). Collectively these expression and phosphoproteomics data show that some endurance genes change their expression or phosphorylation in human skeletal muscle after exercise and are therefore potential regulators of skeletal muscle endurance adaptations.
As we have mentioned, skeletal muscle is not the only organ whose function limits endurance performance. The heart is a particularly important endurance organ. Endurance training induces the athlete’s heart or physiological cardiac hypertrophy. Such a heart has a hypertrophied left ventricle, which increases stroke volume, cardiac output and the VO2max (Ekblom, 1968; Ellison et al., 2012). To test whether endurance genes change their expression in a mouse model of physiological cardiac hypertrophy induced through 8 weeks of swimming versus a pathologically hypertrophied heart achieved through isoproterenol treatment versus sedentary controls, we re-analyzed the dataset of Galindo et al. (2009). This analysis revealed that depending on the measured probe, Ppargc1a increased by 4.27 and 6.96-fold, whereas Thra decreased -2.50-fold, Ppard by -2.86-fold and Tnfsf12 by -1.80 fold, in the physiologically hypertrophied heart specifically when compared to sedentary control, respectively (Supplementary Data S8). Some endurance genes alter their expression in response to both physiological and pathological hypertrophy of the heart. Hif1a increases by 1.99-fold, Mef2c by 1.99 – 2.90-fold and Pten by 2.05-fold in during physiological hypertrophy, while Hif1a decreases -1.87-fold, Mef2c-2.16-fold, and Pten by -1.74 -1.84-fold during pathological hypertrophy. Ppargc1b and Hk2 specifically decrease their expression in pathological hypertrophy by -2.80-fold, and -1.95-fold, respectively, and not during physiological hypertrophy. These data demonstrate that endurance exercise can induce or repress the expression of some endurance genes in the heart of mice.
Next, we investigated whether endurance genes are functionally linked and whether these genes are enriched among specific classes of genes such as genes that share a common domain or molecular function. First, we performed a STRING analysis that predicts direct physical and other associations for a group of proteins (Supplementary Data S9; Szklarczyk et al., 2017). Figure 5 illustrates the results of this analysis. Clusters in this figure are linked to the mitochondrial biogenesis regulator Pgc1a (encoded by Ppargc1a), the calcium/calmodulin-stimulated phosphatase calcineurin encoded by PPP3CA and the β2-adrenoceptor encoded by Adrb2. This suggests some functional interaction between endurance genes.
Figure 5. String analysis for endurance genes. Lines between proteins indicate evidence of association.
We then performed a functional enrichment analysis to see whether the endurance performance-increasing genes share common features. It has been pointed out that such enrichment analyses may be erroneous if the enrichment is not adjusted to the transcriptome of the tissue of interest (Timmons et al., 2015). However, the endurance effect of our gene list could be due to expression in several tissues and so we are unable to use one tissue-specific background list. Instead, we have conducted a general meta-enrichment analysis using ToppGene (Chen et al., 2009). This analysis has revealed potentially relevant enrichments that include the mouse phenotype “abnormal heart rate” (MP:0001629) for 8 of the genes and “abnormal metabolism” (MP:0005266) for 13 of the endurance genes. All significant enrichments are listed in Supplementary Data S10.
Finally, ≈3000 proteins are predicted to be secreted from cells. To find out how many endurance genes are predicted to encode secreted proteins, we retrieved the list of secreted genes/proteins from Protein Atlas1 and compared these genes with the list of endurance genes. We identified three endurance genes, Pappa (Pappalysin 1), Thbs1 (Thrombospondin 1) and Tnfsf12 (TNF superfamily member 12) that encode proteins that are predicted to be secreted (Supplementary Data S11). Together, these secreted proteins could play possible roles in inter-organ signaling in relation to acute exercise or adaptation to chronic exercise.
The main finding of this systematic review is the identification of 31 genes whose gain or loss-of-function increases endurance performance in mice by up to 1800% when compared to wildtype control mice. Further bioinformatical analyses reveal the DNA sequence variability of these genes in humans, their organ-specific expression pattern, functional links in-between these genes and the proteins they encode, and a role for some endurance genes during adaptation to endurance exercise. This endurance gene list also provides an up-to-date candidate list for more targeted human genetic analyses for endurance performance or trainability.
A key question is whether the mouse endurance genes are relevant for the genetics of human endurance. Our analyses suggest that this is probably the case. First, human variants of ACTN3, ADRB2, BDKRB2, HIF1A, PPARD, PPARGC1A, PPARGC1B, and PPP3CA are associated with human endurance (Ahmetov et al., 2016) and human variants of ADCY5, PPARD and HIF1A are associated with VO2max trainability (Williams et al., 2017). Second, GWAS studies identified SNPs linked to the human homologues of endurance genes to the hemoglobin concentration (Astle et al., 2016), resting heart rate (Eppinga et al., 2016), metabolic traits (Suhre et al., 2011; Shin et al., 2014), and left ventricular wall thickness (Wild et al., 2017). Finally, we found that the exon DNA sequence of human endurance genes varies considerably. On average, each endurance gene has 174 missense DNA variants, 5 loss-of-function DNA variants and 11 copy number DNA variants in 60,706 individuals (Lek et al., 2016; note that this data refers to the number of alleles and that the number of carriers is much higher). However, associations or DNA variants of endurance genes does not mean that they actually increase endurance in humans (Houweling et al., 2018). Associations need to be replicated and supported by functional analysis, such as in mouse models, to find mechanisms responsible for endurance phenotypes (Eynon et al., 2017). So far, only for ACTN3 there is consistent data showing that a common DNA variant in humans influences muscle performance, which is similar in the ACTN3 mouse model (Houweling et al., 2018). Collectively, this suggests that variants of the human homologues of mouse endurance genes could contribute to the variation of endurance-related traits seen in the human population. This has to be replicated in future studies.
The majority of the endurance genes identified in our study are linked to skeletal muscle metabolism and mitochondrial biogenesis. Here, the transcriptional co-factor Pgc-1α plays a key role (Lin et al., 2002). The authors of the original Ppargc1a (which encodes Pgc-1α) overexpression study did not test the endurance capacity of the transgenic mice. However, subsequent studies demonstrated the effect of the overexpression of Ppargc1a (Calvo et al., 2008), of the b-isoform of Ppargc1a (Tadaishi et al., 2011) and of Ppargc1b (Arany et al., 2007) on endurance capacity, skeletal muscle mitochondrial biogenesis and muscle fiber-related gene expression. A related factor is Ppard whose overexpression has similar effects on mitochondrial biogenesis, muscle fiber-related gene expression and endurance capacity (Wang et al., 2004). Many of the other endurance genes regulate the expression of Ppargc1a isoforms or of Ppard or the activity of the proteins that these genes encode which explains their effect on endurance capacity. Ppargc1a expression also increases after endurance exercise in mouse (Baar et al., 2002) and human skeletal muscle (Pilegaard et al., 2003; Figure 4) as well as the heart during swimming-induced cardiac hypertrophy (Supplementary Data S8) suggesting that it is a mediator of muscle and heart adaptations to endurance exercise (Holloszy, 1967).
Two of the endurance genes haven been linked to thyroid hormone signaling. They are Crym, which encodes a thyroid hormone-binding crystallin (Seko et al., 2016) and Thra which encodes a nuclear thyroid hormone receptor (Pessemesse et al., 2012). The mechanisms are probably linked to the effect of thyroid hormones on mitochondrial biogenesis via Pgc-1α and related factors (Weitzel and Alexander Iwen, 2011). Interestingly, some endurance athletes have been reported to take thyroid medication as a treatment (Hart, 2017) which is a concern as the real purpose might be to enhance endurance capacity through thyroid hormone treatment.
Pgc-1α and related factors are, however, not the only regulators of mitochondrial biogenesis, muscle metabolism and fiber type-specific gene expression. A different group includes Ppp3ca which encodes a subunit the Ca2+-activated phosphatase calcineurin (Jiang et al., 2010), the calcineurin regulator calsarcin-2 encoded by Myoz1 (Frey et al., 2008) and the calcineurin-regulated transcription factor Tfeb which promotes mitochondrial biogenesis and other metabolic adaptations (Mansueto et al., 2017). A genome-wide association study has also linked TFEB to left ventricular wall thickness and TFEB phosphorylation decreased significantly from pre to post exercise (Hoffman et al., 2015), suggesting that TFEB may regulate skeletal muscle and heart adaptations to endurance exercise.
In humans, a key determinant of a high endurance capacity is the VO2max, which depends on the maximal oxygen transport capacity. This in turn depends on the maximal cardiac output, which is increased in the athlete’s heart, and on the oxygen transport capacity of the blood (Bergh et al., 2000; Lundby et al., 2017). Earlier studies reported that cardiac-specific expression of the kinase Mek1 increased cardiac function (Bueno et al., 2000) and that the expression of a dominant negative form of Pi3k in the heart prevented physiological cardiac hypertrophy (i.e., the development of an athlete’s heart) after swimming in mice (McMullen et al., 2003). Unfortunately, whether the heart-specific overexpression of these two genes increased exercise capacity in mice was not tested. In another study, researchers overexpressed the catecholamine-related, adenylyl cyclase-encoding genes Adcy5 and Adcy8 in the heart. They found that this overexpression increased cardiac contractility and endurance capacity in the transgenic mice when compared to wildtype controls (Esposito et al., 2008). Also, ADCY5 gene variants are associated with VO2max trainability in humans (Williams et al., 2017) and for ADCY5 and ADCY8 together 659 different missense DNA variants, 17 loss-of-function DNA variants and 13 DNA copy number variants have been reported for 60,706 humans in the Exac study (Lek et al., 2016). Collectively, this suggests that numerous DNA variants of ADCY5 and ADCY8 might contribute to the variation of VO2max trainability and perhaps VO2max seen in humans. In a different model, the knockout of Thbs1 (encoding thrombospondin-1) increased skeletal muscle and cardiac capillary density, left ventricular size and endurance capacity (Malek and Olfert, 2009). Together this demonstrates changed activity of some endurance genes may contribute to the development of an Athlete’s heart.
Other endurance genes change their expression in the heart in endurance-exercising mice (Figure 3; especially Ppargc1a appears to increase during physiological cardiac hypertrophy) or are associated with cardiac phenotypes in GWAS studies (Supplementary Data S3). Here, the SNP near PCK1 was associated with the hemoglobin concentration (Astle et al., 2016). However, it is unclear whether the overexpression of Pck1 (encoding Pepck) in skeletal muscle can explain an increased hemoglobin concentration (Hakimi et al., 2007).
Mental fatigue has recently been highlighted as an endurance-influencing factor (Van Cutsem et al., 2017) but we know little about the molecular mechanisms that influence mental fatigue, neural function and behavior in relation to endurance. Two of the endurance genes are linked to the nervous system. Acetylcholine is synthesized from acetyl-CoA and choline and released from motor endplates to cause muscle fibers to contract. Interestingly, the overexpression of the sodium-choline channel gene Slc5a7 increased choline transport, endurance capacity but not strength and physical activity in mice (Holmstrand et al., 2014). The overexpression of Pck1 in skeletal muscle not only increased the endurance capacity most (Figure 1) but made these mice hyperactive in their home cages (Hakimi et al., 2007). How the high expression of a gluconeogenetic enzyme in skeletal muscle can increase spontaneous activity in mice is unclear.
Like in our earlier review on hypertrophy-causing genes (Verbrugge et al., 2018), a limitation of this review is that the manipulated genes are subjectively chosen by the researchers of each study. Moreover, many researchers do not test whether a manipulated gene changes endurance exercise capacity. The consequence is that the list of manipulated genes and the mice that are tested in an endurance test is subjective, resulting in a biased list of endurance genes. Currently, the International Mouse Phenotyping Consortium (IMPC) generates and phenotypically analyses 20,000 mouse lines2 but measurement of endurance capacity is not included in the first-line analysis (Brown and Moore, 2012). Here a triage system might be useful, so that mice that have increased cardiac function, a higher haematocrit or other endurance-associated phenotypes are then also tested in an endurance test.
Another limitation of the study is that the variation of endurance performance also depends on the endurance tests used in the individual studies. For example, if the endurance performance of the same wildtype and transgenic animals is measured in a graded exercise test versus a time trial test then the results will differ. For example, in the Pck1 mouse study the researchers found that wildtype mice ran ≈200 m at a speed of 20 m/min whereas Pck1-overexpressing mice ran in-between 2000 and 6000 m, which is on average ≈1800% more than the wildtype mice. In contrast, when the same mice were compared in a graded exercise test with an increase of 1 m/min every minute then the Pck1-overexpressing mice achieved a maximum speed of ≈50 m/min whereas the wildtype controls ran up to a speed of ≈20 m/min. This is a much smaller increase of 150% (Hakimi et al., 2007). Together this demonstrates that the type of endurance performance test can greatly affect the outcome and differences in percent between wildtype and transgenic mouse strains. Generally, researchers should aim for standardized protocols for running tests to exhaustion or for graded exercise tests. Booth et al. give some specific recommendations for such exercise tests in mice (Booth et al., 2010).
A final limitation of this study is that we do not include mouse models where the gain or loss-of-function of a gene reduces endurance performance. Such mouse models can provide important insights into genes that influence endurance performance (Garton et al., 2016). However, the major problem with such mouse models is that it is difficult to judge whether the decrease in performance is because of the reduction of a true endurance-increasing variable or whether endurance is decreased because of a disease. For example, we would expect that almost all tumor-bearing mice have a reduced endurance capacity even though the effect of the genetic manipulation causes tumor and does not directly affect endurance-influencing variables.
A high endurance capacity is important for many sports, is associated with good health, low mortality and many endurance-associated traits are ≈50% inherited. However, how DNA variants contribute to the variation of human endurance and especially of VO2max and VO2max trainability is still incompletely understood and some aspects are controversial (Lundby et al., 2017). The contribution of this study to our understanding of endurance genetics is a list of 31 genes whose gain or loss-of-function increases endurance performance by up to 1800% in mice. Many of the identified endurance genes are linked to biological pathways that are relevant for endurance, especially mitochondrial biogenesis and muscle metabolism. Moreover, exome sequencing data for 60,706 individuals contain a large number of amino acid sequence and/or function-changing DNA variants for these genes (Lek et al., 2016), suggesting that human variants of these genes partially explain the variation of endurance capacity. In contrast, few endurance genes are linked to the oxygen-transporting systems that limit the VO2max. Here the best VO2max-influencing candidate genes are the ADCY5 and ADCY8 adenylyl cyclase genes that increase cardiac contractility (Esposito et al., 2008). ADCY5 gene variants are also associated with VO2max trainability in humans (Williams et al., 2017) and more than 600 types of function-altering DNA variants have been reported for ADCY5 and ADCY8 in 60,706 humans (Lek et al., 2016). Still, this leaves much of the known genetic variability of the VO2max and VO2max trainability unexplained. Practically, this endurance gene list and our earlier list of hypertrophy-promoting list may be useful for more targeted and in depth genetic analyses of elite endurance athletes such as East African runners.
FYN and SV conducted the systematic paper analysis. FYN, SV, MS, and HW did the bioinformatical analyses. LB and MH revised the manuscript. HW drafted the manuscript and all authors contributed to writing the manuscript.
This work was supported by the German Research Foundation (DFG) and the Technical University of Munich within the Open Access Publishing Funding Program and the German Federal Ministry of Education and Research (Infrafrontier grant 01KX1012).
The authors declare that the research was conducted in the absence of any commercial or financial relationships that could be construed as a potential conflict of interest.
We thank Dr. Kenneth Dyar for commenting on the manuscript.
The Supplementary Material for this article can be found online at: https://www.frontiersin.org/articles/10.3389/fphys.2019.00262/full#supplementary-material
Ahmetov, I. I., Egorova, E. S., Gabdrakhmanova, L. J., and Fedotovskaya, O. N. (2016). Genes and athletic performance: an update. Med. Sport Sci. 61, 41–54. doi: 10.1159/000445240
An, D., Lessard, S. J., Toyoda, T., Lee, M.-Y., Koh, H.-J., Qi, L., et al. (2014). Overexpression of TRB3 in muscle alters muscle fiber type and improves exercise capacity in mice. Am. J. Physiol. Regul. Integr. Comp. Physiol. 306, R925–R933. doi: 10.1152/ajpregu.00027.2014
Arany, Z., Lebrasseur, N., Morris, C., Smith, E., Yang, W., Ma, Y., et al. (2007). The transcriptional coactivator PGC-1beta drives the formation of oxidative type IIX fibers in skeletal muscle. Cell Metab. 5, 35–46. doi: 10.1016/j.cmet.2006.12.003
Astle, W. J., Elding, H., Jiang, T., Allen, D., Ruklisa, D., Mann, A. L., et al. (2016). The allelic landscape of human blood cell trait variation and links to common complex disease. Cell 167, 1415–1429.e19. doi: 10.1016/j.cell.2016.10.042
Baar, K., Wende, A. R., Jones, T. E., Marison, M., Nolte, L. A., Chen, M., et al. (2002). Adaptations of skeletal muscle to exercise: rapid increase in the transcriptional coactivator PGC-1. FASEB J. 16, 1879–1886. doi: 10.1096/fj.02-0367com
Bassett, D. R. Jr., and Howley, E. T. (2000). Limiting factors for maximum oxygen uptake and determinants of endurance performance. Med. Sci. Sports Exerc. 32, 70–84. doi: 10.1097/00005768-200001000-00012
Bergh, U., Ekblom, B., and Astrand, P. O. (2000). Maximal oxygen uptake “classical” versus “contemporary” viewpoints. Med. Sci. Sports Exerc. 32, 85–88. doi: 10.1097/00005768-200001000-00013
Bergstrom, J., Hermansen, L., Hultman, E., and Saltin, B. (1967). Diet, muscle glycogen and physical performance. Acta Physiol. Scand. 71, 140–150. doi: 10.1111/j.1748-1716.1967.tb03720.x
Bigham, A. W. (2016). Genetics of human origin and evolution: high-altitude adaptations. Curr. Opin. Genet. Dev. 41, 8–13. doi: 10.1016/j.gde.2016.06.018
Booth, F. W., Laye, M. J., and Spangenburg, E. E. (2010). Gold standards for scientists who are conducting animal-based exercise studies. J. Appl. Physiol. 108, 219–221. doi: 10.1152/japplphysiol.00125.2009
Bouchard, C., An, P., Rice, T., Skinner, J. S., Wilmore, J. H., Gagnon, J., et al. (1999). Familial aggregation of VO(2max) response to exercise training: results from the HERITAGE Family Study. J. Appl. Physiol. 87, 1003–1008. doi: 10.1152/jappl.1999.87.3.1003
Bouchard, C., Daw, E. W., Rice, T., Perusse, L., Gagnon, J., Province, M. A., et al. (1998). Familial resemblance for VO2max in the sedentary state: the HERITAGE family study. Med. Sci. Sports Exerc. 30, 252–258. doi: 10.1097/00005768-199802000-00013
Bramble, D. M., and Lieberman, D. E. (2004). Endurance running and the evolution of homo. Nature 432, 345–352. doi: 10.1038/nature03052
Brodal, P., Ingjer, F., and Hermansen, L. (1977). Capillary supply of skeletal muscle fibers in untrained and endurance-trained men. Am. J. Physiol. 232, H705–H712. doi: 10.1152/ajpheart.1977.232.6.H705
Brown, S. D., and Moore, M. W. (2012). The international mouse phenotyping consortium: past and future perspectives on mouse phenotyping. Mamm. Genome 23, 632–640. doi: 10.1007/s00335-012-9427-x
Bueno, O. F., De Windt, L. J., Tymitz, K. M., Witt, S. A., Kimball, T. R., Klevitsky, R., et al. (2000). The MEK1-ERK1/2 signaling pathway promotes compensated cardiac hypertrophy in transgenic mice. EMBO J. 19, 6341–6350. doi: 10.1093/emboj/19.23.6341
Calvo, J. A., Daniels, T. G., Wang, X., Paul, A., Lin, J., Spiegelman, B., et al. (2008). Muscle-specific expression of PPARγ coactivator-1α improves exercise performance and increases peak oxygen uptake. J. Appl. Physiol. 104,1304–1312. doi: 10.1152/japplphysiol.01231.2007
Chahal, H. S., Stals, K., Unterlander, M., Balding, D. J., Thomas, M. G., Kumar, A. V., et al. (2011). AIP mutation in pituitary adenomas in the 18th century and today. N. Engl. J. Med. 364, 43–50. doi: 10.1056/NEJMoa1008020
Chen, J., Bardes, E. E., Aronow, B. J., and Jegga, A. G. (2009). ToppGene Suite for gene list enrichment analysis and candidate gene prioritization. Nucleic Acids Res. 37, W305–W311. doi: 10.1093/nar/gkp427
Conover, C. A., Bale, L. K., and Nair, K. S. (2016). Comparative gene expression and phenotype analyses of skeletal muscle from aged wild-type and PAPP-A-deficient mice. Exp. Gerontol. 80, 36–42. doi: 10.1016/j.exger.2016.04.005
Costill, D. L., Daniels, J., Evans, W., Fink, W., Krahenbuhl, G., and Saltin, B. (1976). Skeletal muscle enzymes and fiber composition in male and female track athletes. J. Appl. Physiol. 40, 149–154. doi: 10.1152/jappl.1976.40.2.149
de la Chapelle, A., Traskelin, A. L., and Juvonen, E. (1993). Truncated erythropoietin receptor causes dominantly inherited benign human erythrocytosis. Proc. Natl. Acad. Sci. U.S.A. 90, 4495–4499. doi: 10.1073/pnas.90.10.4495
Doherty, A., Smith-Byrne, K., Ferreira, T., Holmes, M. V., Holmes, C., Pulit, S. L., et al. (2018). GWAS identifies 14 loci for device-measured physical activity and sleep duration. Nat. Commun. 9:5257. doi: 10.1038/s41467-018-07743-4
Ekblom, B. (1968). Effect of physical training on oxygen transport system in man. Acta Physiol. Scand. Suppl. 328, 1–45.
Ellison, G. M., Waring, C. D., Vicinanza, C., and Torella, D. (2012). Physiological cardiac remodelling in response to endurance exercise training: cellular and molecular mechanisms. Heart 98, 5–10. doi: 10.1136/heartjnl-2011-300639
Eppinga, R. N., Hagemeijer, Y., Burgess, S., Hinds, D. A., Stefansson, K., Gudbjartsson, D. F., et al. (2016). Identification of genomic loci associated with resting heart rate and shared genetic predictors with all-cause mortality. Nat. Genet. 48, 1557–1563. doi: 10.1038/ng.3708
Esposito, G., Perrino, C., Ozaki, T., Takaoka, H., Defer, N., Petretta, M. P., et al. (2008). Increased myocardial contractility and enhanced exercise function in transgenic mice overexpressing either adenylyl cyclase 5 or 8. Basic Res. Cardiol. 103, 22–30. doi: 10.1007/s00395-007-0688-6
Eynon, N., Voisin, S., Lucia, A., Wang, G., and Pitsiladis, Y. (2017). Preface: genomics and biology of exercise is undergoing a paradigm shift. BMC Genomics 18:825. doi: 10.1186/s12864-017-4184-6
Foldynova-Trantirkova, S., Wilcox, W. R., and Krejci, P. (2012). Sixteen years and counting: the current understanding of fibroblast growth factor receptor 3 (FGFR3) signaling in skeletal dysplasias. Hum. Mutat. 33, 29–41. doi: 10.1002/humu.21636
Frazier, E. P., Isenberg, J. S., Shiva, S., Zhao, L., Schlesinger, P., Dimitry, J., et al. (2011). Age-dependent regulation of skeletal muscle mitochondria by the thrombospondin-1 receptor CD47. Matrix Biol. 30, 154–161. doi: 10.1016/j.matbio.2010.12.004
Frey, N., Frank, D., Lippl, S., Kuhn, C., Kogler, H., Barrientos, T., et al. (2008). Calsarcin-2 deficiency increases exercise capacity in mice through calcineurin/NFAT activation. J. Clin. Invest. 118, 3598–3608. doi: 10.1172/JCI36277
Fueger, P. T., Shearer, J., Krueger, T. M., Posey, K. A., Bracy, D. P., Heikkinen, S., et al. (2005). Hexokinase II protein content is a determinant of exercise endurance capacity in the mouse. J. Physiol. 566, 533–541. doi: 10.1113/jphysiol.2005.085043
Galindo, C. L., Skinner, M. A., Errami, M., Olson, L. D., Watson, D. A., Li, J., et al. (2009). Transcriptional profile of isoproterenol-induced cardiomyopathy and comparison to exercise-induced cardiac hypertrophy and human cardiac failure. BMC Physiol. 9:23. doi: 10.1186/1472-6793-9-23
Garton, F. C., North, K. N., Koch, L. G., Britton, S. L., Nogales-Gadea, G., and Lucia, A. (2016). Rodent models for resolving extremes of exercise and health. Physiol. Genomics 48, 82–92. doi: 10.1152/physiolgenomics.00077.2015
Gollnick, P. D., and Saltin, B. (1983). “Skeletal muscle adaptability: significance for metabolism and performance,” in Handbook of Physiology. Skeletal Muscle, eds L. D. Peachey, R. H. Adrian, and S. R. Geiger (Baltimore: Williams & Wilkins).
Hakimi, P., Yang, J., Casadesus, G., Massillon, D., Tolentino-Silva, F., Nye, C. K., et al. (2007). Overexpression of the cytosolic form of phosphoenolpyruvate carboxykinase (GTP) in skeletal muscle repatterns energy metabolism in the mouse. J. Biol. Chem. 282, 32844–32855. doi: 10.1074/jbc.M706127200
Hart, M. (2017). This Doesn’t Sound Legal’: Inside Nike’s Oregon Project. New York, NY: The New York Times.
Hoffman, N. J., Parker, B. L., Chaudhuri, R., Fisher-Wellman, K. H., Kleinert, M., Humphrey, S. J., et al. (2015). Global phosphoproteomic analysis of human skeletal muscle reveals a network of exercise-regulated kinases and AMPK substrates. Cell Metab. 22, 922–935. doi: 10.1016/j.cmet.2015.09.001
Holloszy, J. O. (1967). Biochemical adaptations in muscle. Effects of exercise on mitochondrial oxygen uptake and respiratory enzyme activity in skeletal muscle. J. Biol. Chem. 242, 2278–2282.
Holmstrand, E. C., Lund, D., Cherian, A. K., Wright, J., Martin, R. F., Ennis, E. A., et al. (2014). Transgenic overexpression of the presynaptic choline transporter elevates acetylcholine levels and augments motor endurance. Neurochem. Int. 73, 217–228. doi: 10.1016/j.neuint.2013.11.008
Houweling, P. J., Papadimitriou, I. D., Seto, J. T., Pérez, L. M., Coso, J. D., North, K. N., et al. (2018). Is evolutionary loss our gain? The role of ACTN3 p.Arg577Ter (R577X) genotype in athletic performance, ageing, and disease. Hum. Mutat. 39, 1774–1787. doi: 10.1002/humu.23663
Iwasaki, H., Naka, A., Iida, K. T., Nakagawa, Y., Matsuzaka, T., Ishii, K., et al. (2012). TFE3 regulates muscle metabolic gene expression, increases glycogen stores, and enhances insulin sensitivity in mice. Am. J. Physiol. Endocrinol. Metab. 302, E896–E902. doi: 10.1152/ajpendo.00204.2011
Jiang, L. Q., Garcia-Roves, P. M., de Castro Barbosa, T., and Zierath, J. R. (2010). Constitutively active calcineurin in skeletal muscle increases endurance performance and mitochondrial respiratory capacity. Am. J. Physiol. Endocrinol. Metab. 298, E8–E16. doi: 10.1152/ajpendo.00403.2009
Kanzleiter, T., Rath, M., Penkov, D., Puchkov, D., Schulz, N., Blasi, F., et al. (2014). Pknox1/Prep1 regulates mitochondrial oxidative phosphorylation components in skeletal muscle. Mol. Cell. Biol. 34, 290–298. doi: 10.1128/MCB.01232-13
Kodama, S., Saito, K., Tanaka, S., Maki, M., Yachi, Y., Asumi, M., et al. (2009). Cardiorespiratory fitness as a quantitative predictor of all-cause mortality and cardiovascular events in healthy men and women: a meta-analysis. Jama 301, 2024–2035. doi: 10.1001/jama.2009.681
Laurent, G., German, N. J., Saha, A. K., de Boer, V. C. J., Davies, M., Koves, T. R., et al. (2013). SIRT4 coordinates the balance between lipid synthesis and catabolism by repressing malonyl CoA decarboxylase. Mol. Cell. 50, 686–698. doi: 10.1016/j.molcel.2013.05.012
Lek, M., Karczewski, K. J., Minikel, E. V., Samocha, K. E., Banks, E., Fennell, T., et al. (2016). Analysis of protein-coding genetic variation in 60,706 humans. Nature 536, 285–291. doi: 10.1038/nature19057
Lin, J., Wu, H., Tarr, P. T., Zhang, C. Y., Wu, Z., Boss, O., et al. (2002). Transcriptional co-activator PGC-1 alpha drives the formation of slow-twitch muscle fibres. Nature 418, 797–801. doi: 10.1038/nature00904
Lundby, C., Montero, D., and Joyner, M. (2017). Biology of VO2 max: looking under the physiology lamp. Acta Physiol. 220, 218–228. doi: 10.1111/apha.12827
Luo, Z., Ma, L., Zhao, Z., He, H., Yang, D., Feng, X., et al. (2012). TRPV1 activation improves exercise endurance and energy metabolism through PGC-1α upregulation in mice. Cell. Res. 22, 551–564. doi: 10.1038/cr.2011.205
MacArthur, D. G., Seto, J. T., Raftery, J. M., Quinlan, K. G., Huttley, G. A., Hook, J. W., et al. (2007). Loss of ACTN3 gene function alters mouse muscle metabolism and shows evidence of positive selection in humans. Nat. Genet. 39, 1261–1265. doi: 10.1038/ng2122
Maldonado, S., Mujika, I., and Padilla, S. (2002). Influence of body mass and height on the energy cost of running in highly trained middle- and long-distance runners. Int. J. Sports Med. 23, 268–272. doi: 10.1055/s-2002-29083
Malek, M. H., and Olfert, I. M. (2009). Global deletion of thrombospondin-1 increases cardiac and skeletal muscle capillarity and exercise capacity in mice. Exp. Physiol. 94, 749–760. doi: 10.1113/expphysiol.2008.045989
Mansueto, G., Armani, A., Viscomi, C., D’Orsi, L., De Cegli, R., Polishchuk, E. V., et al. (2017). Transcription factor EB controls metabolic flexibility during exercise. Cell Metab. 25, 182–196. doi: 10.1016/j.cmet.2016.11.003
Mason, S. D., Howlett, R. A., Kim, M. J., Olfert, I. M., Hogan, M. C., McNulty, W., et al. (2004). Loss of skeletal muscle HIF-1alpha results in altered exercise endurance. PLoS Biol. 2:e288. doi: 10.1371/journal.pbio.0020288
McMullen, J. R., Shioi, T., Zhang, L., Tarnavski, O., Sherwood, M. C., Kang, P. M., et al. (2003). Phosphoinositide 3-kinase(p110alpha) plays a critical role for the induction of physiological, but not pathological, cardiac hypertrophy. Proc. Natl. Acad. Sci. U.S.A. 100, 12355–12360. doi: 10.1073/pnas.1934654100
Moher, D., Liberati, A., Tetzlaff, J., and Altman, D. G. (2009). Preferred reporting items for systematic reviews and meta-analyses: the PRISMA statement. PLoS Med. 6:e1000097. doi: 10.1371/journal.pmed.1000097
Pearen, M. A., Eriksson, N. A., Fitzsimmons, R. L., Goode, J. M., Martel, N., Andrikopoulos, S., et al. (2012). The nuclear receptor, Nor-1, markedly increases type II oxidative muscle fibers and resistance to fatigue. Mol. Endocrinol. 26, 372–384. doi: 10.1210/me.2011-1274
Peeters, M. W., Thomis, M. A., Beunen, G. P., and Malina, R. M. (2009). Genetics and sports: an overview of the pre-molecular biology era. Med. Sport Sci. 54, 28–42. doi: 10.1159/000235695
Pessemesse, L., Schlernitzauer, A., Sar, C., Levin, J., Grandemange, S., Seyer, P., et al. (2012). Depletion of the p43 mitochondrial T3 receptor in mice affects skeletal muscle development and activity. FASEB J. 26, 748–756. doi: 10.1096/fj.11-195933
Pilegaard, H., Saltin, B., and Neufer, P. D. (2003). Exercise induces transient transcriptional activation of the PGC-1alpha gene in human skeletal muscle. J. Physiol. 546(Pt 3), 851–858.
Potthoff, M. J., Wu, H., Arnold, M. A., Shelton, J. M., Backs, J., McAnally, J., et al. (2007). Histone deacetylase degradation and MEF2 activation promote the formation of slow-twitch myofibers. J. Clin. Invest. 117, 2459–2467. doi: 10.1172/JCI31960
Quinn, L. S., Anderson, B. G., Conner, J. D., and Wolden-Hanson, T. (2013). IL-15 overexpression promotes endurance, oxidative energy metabolism, and muscle PPARδ, SIRT1, PGC-1α, and PGC-1β expression in male mice. Endocrinology 154, 232–245. doi: 10.1210/en.2012-1773
Ramírez, J., Duijvenboden, S. V., Ntalla, I., Mifsud, B., Warren, H. R., Tzanis, E., et al. (2018). Thirty loci identified for heart rate response to exercise and recovery implicate autonomic nervous system. Nat. Commun. 9:1947. doi: 10.1038/s41467-018-04148-1
Rankinen, T., Fuku, N., Wolfarth, B., Wang, G., Sarzynski, M. A., Alexeev, D. G., et al. (2016). No evidence of a common DNA variant profile specific to world class endurance athletes. PLoS One 11:e0147330. doi: 10.1371/journal.pone.0147330
Reis, F. C. G., Haro, A. S., Bacurau, A. V. N., Hirabara, S. M., Wasinski, F., Ormanji, M. S., et al. (2015). Deletion of Kinin B2 receptor alters muscle metabolism and exercise performance. PLoS One 10:e0134844. doi: 10.1371/journal.pone.0134844
Rogowski, M. P., Flowers, M. T., Stamatikos, A. D., Ntambi, J. M., and Paton, C. M. (2013). SCD1 activity in muscle increases triglyceride PUFA content, exercise capacity, and PPARδ expression in mice. J. Lipid. Res. 54, 2636–2646. doi: 10.1194/jlr.M035865
Sato, S., Ogura, Y., Mishra, V., Shin, J., Bhatnagar, S., Hill, B. G., et al. (2013). TWEAK promotes exercise intolerance by decreasing skeletal muscle oxidative phosphorylation capacity. Skelet. Muscle 3, 18. doi: 10.1186/2044-5040-3-18
Schardt, C., Adams, M. B., Owens, T., Keitz, S., and Fontelo, P. (2007). Utilization of the PICO framework to improve searching PubMed for clinical questions. BMC Med. Inform. Decis. Mak. 7:16. doi: 10.1186/1472-6947-7-16
Seko, D., Ogawa, S., Li, T.-S., Taimura, A., and Ono, Y. (2016). mu-Crystallin controls muscle function through thyroid hormone action. FASEB J. 30,1733–1740. doi: 10.1096/fj.15-280933
Shin, S. Y., Fauman, E. B., Petersen, A. K., Krumsiek, J., Santos, R., Huang, J., et al. (2014). An atlas of genetic influences on human blood metabolites. Nat. Genet. 46, 543–550. doi: 10.1038/ng.2982
Silventoinen, K., Sammalisto, S., Perola, M., Boomsma, D. I., Cornes, B. K., Davis, C., et al. (2003). Heritability of adult body height: a comparative study of twin cohorts in eight countries. Twin Res. 6, 399–408. doi: 10.1375/136905203770326402
Simoneau, J. A., and Bouchard, C. (1995). Genetic determinism of fiber type proportion in human skeletal muscle. FASEB J. 9, 1091–1095. doi: 10.1096/fasebj.9.11.7649409
Suhre, K., Shin, S. Y., Petersen, A. K., Mohney, R. P., Meredith, D., Wagele, B., et al. (2011). Human metabolic individuality in biomedical and pharmaceutical research. Nature 477, 54–60. doi: 10.1038/nature10354
Szklarczyk, D., Morris, J. H., Cook, H., Kuhn, M., Wyder, S., Simonovic, M., et al. (2017). The STRING database in 2017: quality-controlled protein-protein association networks, made broadly accessible. Nucleic Acids Res. 45,D362–D368. doi: 10.1093/nar/gkw937
Tadaishi, M., Miura, S., Kai, Y., Kano, Y., Oishi, Y., and Ezaki, O. (2011). Skeletal muscle-specific expression of PGC-1alpha-b, an exercise-responsive isoform, increases exercise capacity and peak oxygen uptake. PLoS One 6:e28290. doi: 10.1371/journal.pone.0028290
Timmons, J. A., Szkop, K. J., and Gallagher, I. J. (2015). Multiple sources of bias confound functional enrichment analysis of global -omics data. Genome Biol. 16:186. doi: 10.1186/s13059-015-0761-7
Van Cutsem, J., Marcora, S., De Pauw, K., Bailey, S., Meeusen, R., and Roelands, B. (2017). The effects of mental fatigue on physical performance: a systematic review. Sports Med. 47, 1569–1588. doi: 10.1007/s40279-016-0672-0
Verbrugge, S. A. J., Schonfelder, M., Becker, L., Yaghoob Nezhad, F., Hrabe de Angelis, M., and Wackerhage, H. (2018). Genes whose gain or loss-of-function increases skeletal muscle mass in mice: a systematic literature review. Front. Physiol. 9:553. doi: 10.3389/fphys.2018.00553
Vissing, K., and Schjerling, P. (2014). Simplified data access on human skeletal muscle transcriptome responses to differentiated exercise. Sci. Data 1:140041. doi: 10.1038/sdata.2014.41
Voltarelli, V. A., Bacurau, A. V. N., Bechara, L. R. G., Bueno, C. R., Bozi, L. H. M., Mattos, K. C., et al. (2012). Lack of β2-AR improves exercise capacity and skeletal muscle oxidative phenotype in mice. Scand. J. Med. Sci. Sports 22, e125–e132. doi: 10.1111/j.1600-0838.2012.01519.x
Wang, Y.-X., Zhang, C.-L., Yu, R. T., Cho, H. K., Nelson, M. C., Bayuga-Ocampo, C. R., et al. (2004). Regulation of muscle fiber type and running endurance by PPARdelta. PLoS Biol. 2:e294. doi: 10.1371/journal.pbio.0020294
Weitzel, J. M., and Alexander Iwen, K. (2011). Coordination of mitochondrial biogenesis by thyroid hormone. Mol. Cell. Endocrinol. 342, 1–7. doi: 10.1016/j.mce.2011.05.009
Wild, P. S., Felix, J. F., Schillert, A., Teumer, A., Chen, M.-H., Leening, M. J. G., et al. (2017). Large-scale genome-wide analysis identifies genetic variants associated with cardiac structure and function. J. Clin. Invest. 127, 1798–1812. doi: 10.1172/JCI84840
Williams, C. J., Williams, M. G., Eynon, N., Ashton, K. J., Little, J. P., Wisloff, U., et al. (2017). Genes to predict VO2max trainability: a systematic review. BMC Genomics 18(Suppl. 8):831. doi: 10.1186/s12864-017-4192-6
Wisloff, U., Najjar, S. M., Ellingsen, O., Haram, P. M., Swoap, S., Al Share, Q., et al. (2005). Cardiovascular risk factors emerge after artificial selection for low aerobic capacity. Science 307, 418–420. doi: 10.1126/science.1108177
Wood, A. R., Esko, T., Yang, J., Vedantam, S., Pers, T. H., Gustafsson, S., et al. (2014). Defining the role of common variation in the genomic and biological architecture of adult human height. Nat. Genet. 46, 1173–1186. doi: 10.1038/ng.3097
Yang, N., MacArthur, D. G., Gulbin, J. P., Hahn, A. G., Beggs, A. H., Easteal, S., et al. (2003). ACTN3 genotype is associated with human elite athletic performance. Am. J. Hum. Genet. 73, 627–631. doi: 10.1086/377590
Keywords: endurance, running, transgenic mice, genetics, mitochondrial biogenesis, GWAS, oxygen uptake, metabolism
Citation: Yaghoob Nezhad F, Verbrugge SAJ, Schönfelder M, Becker L, Hrabě de Angelis M and Wackerhage H (2019) Genes Whose Gain or Loss-of-Function Increases Endurance Performance in Mice: A Systematic Literature Review. Front. Physiol. 10:262. doi: 10.3389/fphys.2019.00262
Received: 03 December 2018; Accepted: 28 February 2019;
Published: 22 March 2019.
Edited by:
Vincent Pialoux, Université Claude Bernard Lyon 1, FranceReviewed by:
Maha Sellami, Qatar University, QatarCopyright © 2019 Yaghoob Nezhad, Verbrugge, Schönfelder, Becker, Hrabě de Angelis and Wackerhage. This is an open-access article distributed under the terms of the Creative Commons Attribution License (CC BY). The use, distribution or reproduction in other forums is permitted, provided the original author(s) and the copyright owner(s) are credited and that the original publication in this journal is cited, in accordance with accepted academic practice. No use, distribution or reproduction is permitted which does not comply with these terms.
*Correspondence: Henning Wackerhage, aGVubmluZy53YWNrZXJoYWdlQHR1bS5kZQ==
†Joint first authors
Disclaimer: All claims expressed in this article are solely those of the authors and do not necessarily represent those of their affiliated organizations, or those of the publisher, the editors and the reviewers. Any product that may be evaluated in this article or claim that may be made by its manufacturer is not guaranteed or endorsed by the publisher.
Research integrity at Frontiers
Learn more about the work of our research integrity team to safeguard the quality of each article we publish.