- 1Institute of Nephrology, Zhong Da Hospital, Southeast University School of Medicine, Nanjing, China
- 2Department of Medicine and Therapeutics, and Li Ka Shing Institute of Health Sciences, The Chinese University of Hong Kong, Hong Kong, Hong Kong
Extracellular vesicles (EVs) are lipid-based membrane-bound particles secreted by virtually all types of cells under both physiological and pathological conditions. Given their unique biological and pharmacological properties, EVs have spurred a renewed interest in their utility for therapeutics. Herein, efforts are made to give a comprehensive overview on the recent advances of EV-based therapy in renal diseases. The fact that EVs are implicated in various renal diseases provides us with new therapeutic modalities by eliminating these pathogenic entities. Strategies that target EVs to inhibit their production, release, and uptake will be discussed. Further, EVs-derived predominantly from stem cells can stimulate tissue repair and ameliorate renal injury via transferring proteins and nucleic acids to injured cells. Such EVs can be exploited as agents in renal regenerative medicine. Finally, we will focus on the specific application of EVs as a novel drug delivery system and highlight the challenges of EVs-based therapies for renal diseases.
Introduction
Extracellular vesicles (EVs) are small membrane vesicles secreted by various types of cells and are found in most body fluids. Depending on their size and biogenesis, EVs are classified into three major categories: exosomes, microvesicles, and apoptotic bodies (van der Pol et al., 2012; Raposo and Stoorvogel, 2013). Here, we focus on the first two classes of EVs. Exosomes, ranging from 30 to 150 nm in diameter, are formed by the fusion of intracellular multivesicular bodies with the plasma membrane (Colombo et al., 2014), whereas microvesicles, 50–1,000 nm in size, are shed directly from the plasma membrane (Morel et al., 2011; Figure 1).
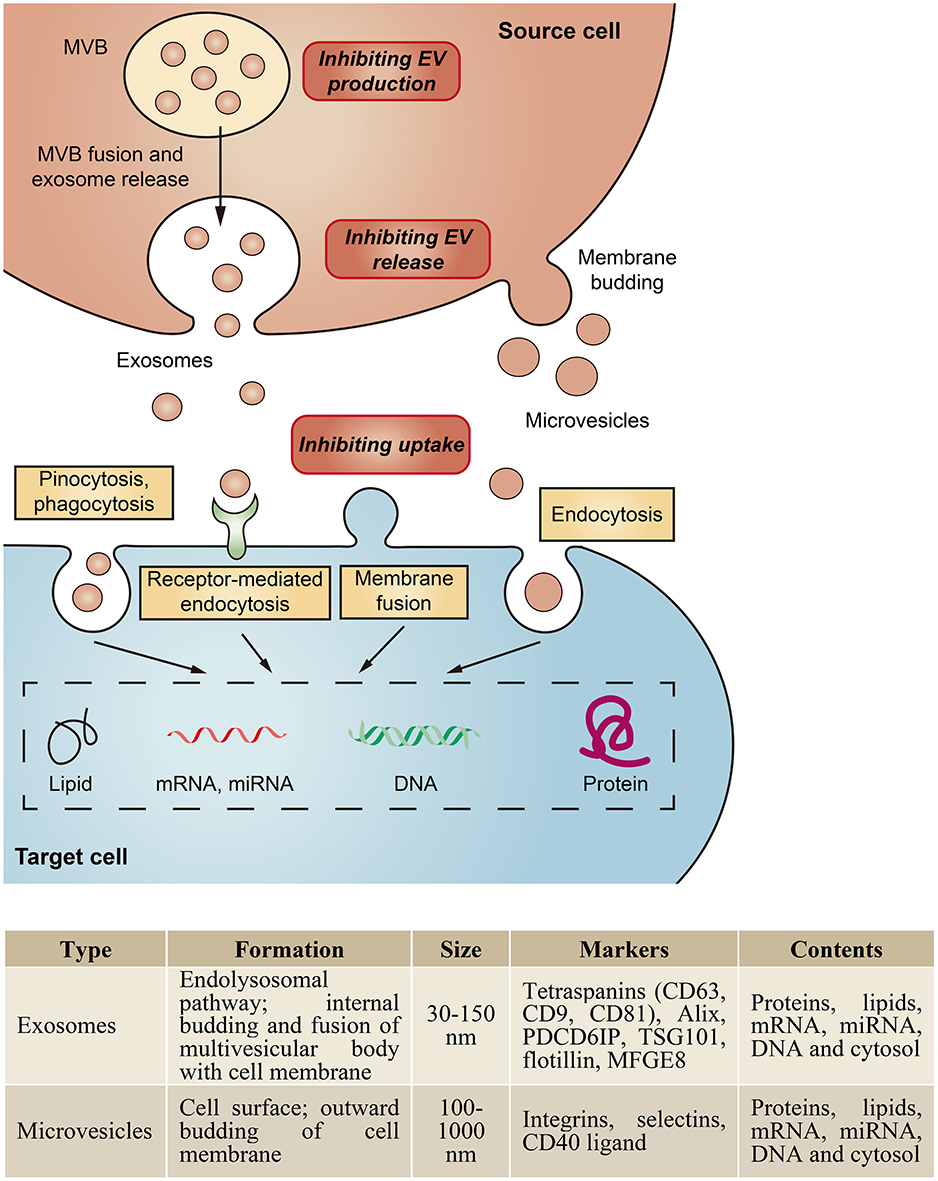
Figure 1. Biogenesis and characteristics of major classes of EVs. EVs can be classed as exosomes, microvesicles, and apoptotic bodies based on their biogenesis and size. Exosomes are formed by the fusion of intracellular multivesicular bodies (MVB) with the plasma membrane, whereas microvesicles are shed directly from the plasma membrane. EVs are taken up by cells by endocytosis, phagocytosis, pinocytosis, or membrane fusion, and subsequently transfer cell membrane receptors or deliver effectors including mRNA, miRNA, DNA, lipid or protein into recipient cells. In addition, EVs could serve as a therapeutic target by inhibition of their production, release or cellular uptake.
EVs were initially regarded as cell dust with no biological significance (Wolf, 1967), however, there is growing evidence for their important role not only in the regulation of normal physiological processes, but also in the pathology underlying several diseases (Camussi et al., 2010; Erdbrügger and Le, 2016; Morrison et al., 2016; Zhang W. et al., 2016; Karpman et al., 2017). In kidneys, EVs have been tightly linked to inflammation, fibrosis, thrombosis, adhesion, immune suppression, and growth and regeneration (Zhang W. et al., 2016; Karpman et al., 2017; Li et al., 2019). Therefore, targeting EVs to inhibit their effects should be an emerging strategy for therapy, such as inhibiting EV assembly and release, modifying harmful compositions, and blocking their dissemination and uptake. In addition, as stem cell-derived EVs contain growth factors, proteins, bioactive lipids, and genetic material that can promote tissue repair, they could be utilized directly as therapeutic agents in renal regenerative medicine. For example, EVs from mesenchymal stem cells protected against acute tubular injury and attenuated kidney inflammation (Bruno et al., 2009, 2012; Rani et al., 2015; Eirin et al., 2017).
Finally, given the natural role in transporting bioactive entities of EVs, they also have potential as drug carrier like a “Trojan horse” (van Dommelen et al., 2012; Fuhrmann et al., 2015). Recent studies indicate that EVs can function as efficient carriers of chemotherapeutic drugs (Tang et al., 2012; Yang et al., 2015), RNA drugs (Alvarez-Erviti et al., 2011; Kamerkar et al., 2017) and anti-inflammatory drugs (Sun et al., 2010; Zhuang et al., 2011). In this review, we will focus on recent developments in EV-based therapy as potential targets and as novel therapeutic agents, especially in the use of EVs as smart drug carriers.
Inhibition of the Release and Uptake of Extracellular Vesicles for Disease Therapy
Within the kidney, EVs can originate from blood cells, endothelial cells, podocytes or tubular epithelial cells (TECs), which have been strongly implicated in the pathogenesis of both acute kidney injury (AKI) and chronic kidney disease (CKD). Our group demonstrated that in the setting of proteinuric kidney disease, albumin triggered TECs to release exosomes packaged with CCL2 mRNA, which was delivered to macrophages and led to interstitial inflammation (Lv et al., 2018a). Borges et al. identified that injured TECs released exosomes containing TGF-β mRNA to activate fibroblasts, contributing to the development of renal fibrosis in post-AKI kidneys (Borges et al., 2013). Moreover, microvesicle-mediated delivery of miR-21 among TECs could also drive the progressive renal fibrosis (Zhou et al., 2013a). Recent data found that transglutaminase-2, a matrix crosslinking enzyme for fibrotic remodeling, was secreted from TECs via exosomes (Furini et al., 2018). Thus, specifically inhibiting the biogenesis or uptake of these pathogenic EVs could be a potential therapeutic approach to alleviate disease progression (Figure 1).
Various cellular components are known to be crucial for the biogenesis and release of EVs, and a number of possible therapeutic targets have been identified. For exosomes, ceramide is an important component in endosomal sorting and exosome biogenesis and its inhibition by GW4869 (neutral sphingomyelinase inhibitor) or amiloride (an antihypertensive agent) decreases exosome production (Trajkovic et al., 2008; Chalmin et al., 2010). GTPases Rab27b can regulate exosome release in some tumor cells, and this was demonstrated to be a therapeutic target (using RNAi) for reducing tumor progression (Ostrowski et al., 2010; Bobrie et al., 2012; Peinado et al., 2012). For microvesicles, the calpain inhibitor calpeptin or calpastain can reduce the shedding of microvesicles (Yano et al., 1993; Zafrani et al., 2012), as well as blocking P2X receptors (Arvidsson et al., 2015). Furthermore, C1 inhibitor lessens the release of endothelial microvesicles, alleviating inflammatory diseases such as vasculitis (Mossberg et al., 2017). However, there are a great many of limitations to target EV biogenesis and release because the precise mechanism remains elusive and is likely to vary among different cells.
In addition to reducing the level of EVs, inhibition of their uptake into cells is also possible by certain substances and antibodies (Mulcahy et al., 2014). Blocking surface phosphatidylserine (which is important for cell adhesion) using diannexin decreases the uptake of EVs derived from tumor cells (Al-Nedawi et al., 2009; Lima et al., 2009). Besides, an antibody to DEL1, annexin V, abciximab, chlorqromazine, cytochalasin D, or cytochalasin B also have been demonstrated to block the uptake of EVs (Barrès et al., 2010; Dasgupta et al., 2012; Faille et al., 2012; Mulcahy et al., 2014), but it is difficult to translate these into therapeutic intervention due to the lack of specific mechanism regarding the key steps in EV trafficking and target definition.
Intrinsic Therapeutic Potential of Extracellular Vesicles
An increasing number of studies have demonstrated EVs, especially those derived from stem cells, have innate therapeutic potential by virtue of their intrinsic cargoes, such as growth factors, soluble proteins, and nucleic acids (EL Andaloussi et al., 2013). In kidneys, mesenchymal stem cell-derived EVs from different origin exhibit encouraging renoprotective efficacy, as shown in models of AKI, diabetic nephropathy, CKD, and fibrosis. The application of these EVs in kidney diseases has been summarized in Table 1. For instance, Wang et al. showed that exosomes derived from bone marrow MSCs were able to transfer miR-let7c to damaged kidney cells and attenuate renal fibrosis in UUO mice (Wang et al., 2016). Kholia et al. reported that EVs derived from liver stem cells exhibited a regenerative, anti-inflammatory, and anti-fibrotic role in aristolochic acid-induced kidney fibrosis (Kholia et al., 2018). In addition, EVs obtained from umbilical cord MSCs (Zhou et al., 2013b; Ju et al., 2015), Warthon's Jelly MSCs (Zou et al., 2014; Gu et al., 2016; Zhang G. et al., 2016), adipose derived MSCs (Lin et al., 2016; Eirin et al., 2017), kidney MSCs (Choi et al., 2014, 2015; Ranghino et al., 2017), as well as urine derived MSCs (Jiang et al., 2016) also showed potential therapeutic benefits on kidney diseases.
Mechanistically, the protective effect of MSC-EVs on kidney diseases depends on their transfer of genetic materials including mRNA and miRNA (He et al., 2015; Rani et al., 2015; Grange et al., 2017; Nargesi et al., 2017). This was confirmed in many studies when degradation of the RNAs in MSC-EVs using RNase could abolish aforementioned therapeutic benefits (Bruno et al., 2009; Gatti et al., 2011; Choi et al., 2015; Zou et al., 2016), suggesting RNA-dependent biological effects. Also, EVs derived from the Drosha-knockdown MSCs that had miRNA-processing defect showed global downregulation of miRNAs, resulting in ineffective renal repair of glycerol-induced AKI (Collino et al., 2015). Gene ontology analysis further showed that those genes shuttled by MSC-EVs were involved in healing pathways associated with renal regeneration (Collino et al., 2015). Moreover, EVs can also deliver proteins from MSCs to injured kidney cells. Proteins related to cell proliferation, adhesion, migration and morphogenesis have been identified in the vesicles by extensive proteomic analysis (Kim et al., 2012; Jiang et al., 2016; Shen et al., 2016; Eirin et al., 2017). In this regard, an elegant study showed that adipose-derived MSC-EVs attenuated renal inflammation by their cargo of IL-10 in a porcine model of coexisting metabolic syndrome and renal artery stenosis (Eirin et al., 2017).
In addition to MSC-EVs, other sources of cell-derived EVs, such as endothelial colony-forming cells (ECFC), endothelial progenitor cells (EPC), and hypoxic TECs, have shown significant beneficial effects as well (Table 1). In models of ischemic AKI, both ECFC-derived exosomes and EPC-derived EVs ameliorated renal injury via transfer of miRNAs (Cantaluppi et al., 2012; Burger et al., 2015; Viñas et al., 2016). In anti-Thy1.1-induced model of glomerulonephritis, EPC-derived EVs alleviated mesangial cell activation, leukocyte infiltration and apoptosis, which was related to its content of mRNAs coding for anti-apoptotic factors and the complement inhibitors (Cantaluppi et al., 2014). Interestingly, Dominguez et al. found that EVs derived from hypoxic TECs significantly improved renal tubular damage, fibrosis, and microvascular pruning in established renal IRI (Dominguez et al., 2017). However, paradoxically, EVs from injured TECs also contribute to the progression of interstitial inflammation and fibrosis (Borges et al., 2013; Zhou et al., 2013a; Furini et al., 2018; Liu et al., 2018; Lv et al., 2018a; Li et al., 2019), the dual role of TEC-derived EVs need to be further clarified.
Extracellular Vesicles as Smart Drug Carriers
Currently, the most preferred drug delivery systems are nanoparticle platforms based on liposomes, albumin, polymeric micelles, and nanosized polymer-drug conjugates, which effectively improve the pharmacokinetics and biodistribution of drugs (Kamaly et al., 2016). However, their immunogenicity, stability and toxicity still remain a concern. In this case, EV-based drug delivery—with many of advantages, such as high permeability, less immunogenicity and non-cytotoxicity—appears to be a potential better tolerated and more efficacious alternative, overcoming the limitations observed with nanoparticles (Ha et al., 2016; Lv et al., 2018b; Yang et al., 2018). So far, EVs have been elegantly demonstrated to be therapeutic nanocarriers for delivering a variety of cargos, including siRNAs, miRNAs, proteins, and drugs (van Dommelen et al., 2012; Fuhrmann et al., 2015). But the application of EVs in kidney diseases has just begun.
Cargo-Loading Techniques
In order to employ EV-based drug delivery, it is essential to consider the methods of cargo loading and their suitability under different circumstances. Each loading strategy has its advantages and limitations depending on the type of therapeutic cargo and site of the disease, and thus further understanding is needed to select the optimal approach for mass production. In brief, cargo encapsulation can be performed exogenously or endogenously (van Dommelen et al., 2012; Batrakova and Kim, 2015; Fuhrmann et al., 2015; Figure 2).
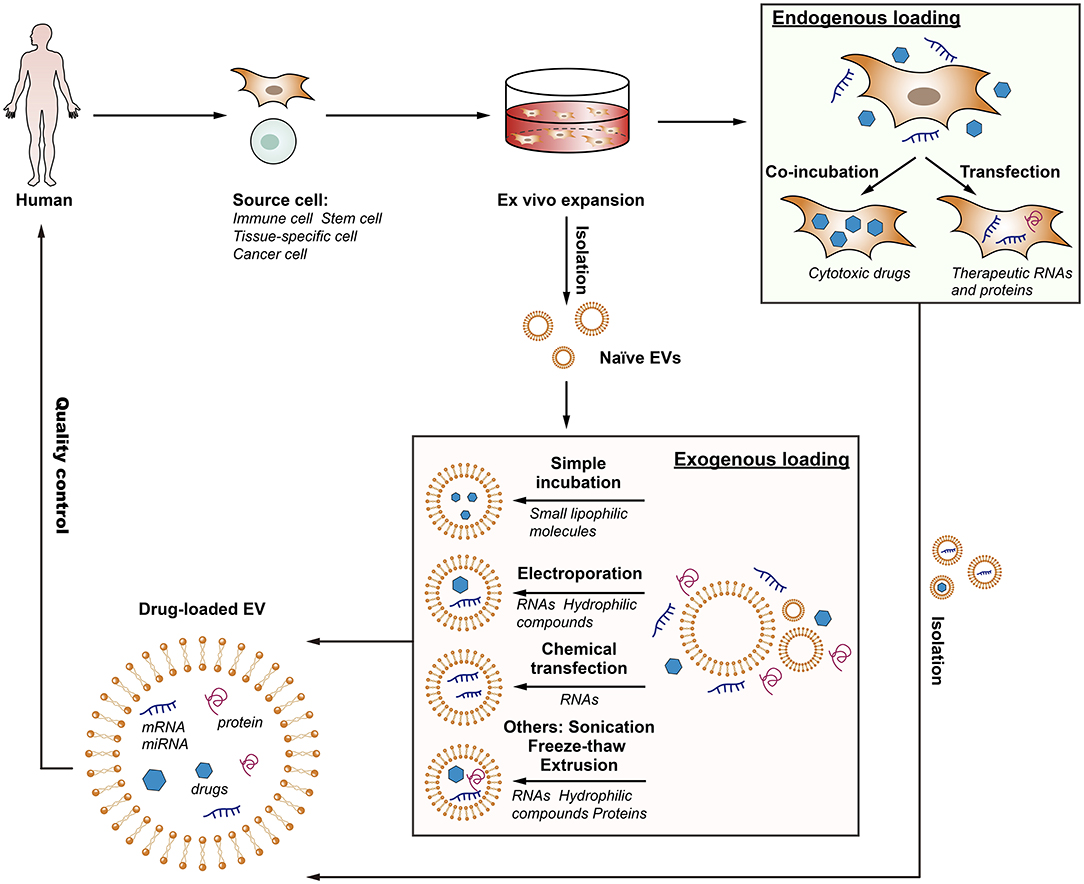
Figure 2. The flow of the production of drug-loaded EVs. EV-based drug delivery requires the correct choice of source cell type for the specific application, and should ideally be patient-derived to avoid triggering immune response. The therapeutic cargo can include different types of siRNA, miRNA, proteins or small molecule compounds such as curcumin or chemotherapeutics. Drug loading can be carried out either endogenously or exogenously. Endogenous loading is achieved by loading source cell with a therapeutic agent or transfecting source cell with drug-encoding gene which is then released in EVs upon collection. Exogenous loading allows the isolation of EVs before their loading with therapeutic cargo with the help of electroporation, simple incubation, chemical transfection, or other approaches. Importantly, the generation process should meet the quality requirements.
Endogenously Loading
For endogenously loading, the drug-loaded EVs are isolated from the modified parent cells through genetic engineering or medication with cytotoxic drugs. This way of loading is convenient and requires very few manipulation steps.
Transfection
Transfection of donor cells to make them overexpress therapeutic molecules is one of the important ways to load therapeutic RNAs or proteins into EVs (Zeelenberg et al., 2008; Lee et al., 2015; Yim et al., 2016). This method has been used for the loading of miRNAs or proteins in few studies. For example, Ohno et al. transfected HEK293 cells to express GE11 and miR-let7a in order to produce GE11-positive miR-let7a-containing exosomes for targeted treatment of EGFR-expressing tumors (Ohno et al., 2013). Although modification of the parent cells is a feasible and well-developed approach, the productivity of RNA is unstable and the factors influencing the RNA level and RNA loading are not completely clear (Batagov et al., 2011). In addition, peptides fused to N terminus of exosome-associated transmembrane protein may degraded by endosomal proteases, resulting in no detection of the peptide on both cells and exosomes (Hung and Leonard, 2015).
Co-incubation
Co-incubation donor cells with exogenous compounds is another approach to load drugs into EVs before they were secreted. It is reported that MSCs-derived exosomes were loaded with paclitaxel by incubating the parent cells with the drug (Pascucci et al., 2014). Similar results were reported for cancer cells that were incubated with different anticancer medicines: doxorubicin, etoposide, carboplatin, irinotecan, epirubicin, and mitoxantrone (Lv et al., 2012; Tang et al., 2012; Yang et al., 2015). However, such technique is of low yield and low entrapment efficacy, and the durg loading is uncontrollable.
Exogenously Loading
For exogenously loading, the cargos were packaged into pre-assembled EVs ex vitro. A number of methods, including simple incubation, chemical transfection, electroporation and sonication are valid strategies for drug incorporation in this regard (Syn et al., 2017).
Simple incubation
Simple incubation is a versatile and feasible approach employed in many cases, through which several small lipophilic molecules, such as curcumin (Sun et al., 2010; Zhuang et al., 2011), doxorubicin (Tian et al., 2014; Rani et al., 2015) and paclitaxel (Yang et al., 2015), are passively loaded into exosomes, but such loading capacity is low and is only work for hydrophobic entities.
Chemical transfection
Chemical transfection methods have been reported to load siRNA in EVs by simply incubating exosomes with siRNA-lipofectamine complexes (siRNA embedded in lipid micelles), but the loading efficiency was low compared to electroporation (Shtam et al., 2013). Additionally, the excess of micelles (siRNA embedded in lipid micelles) and the transfection agents (Lipofectamine 2000) were difficult to separate from the exosomes, which may cause immunogenicity and toxicity, limiting its application.
Electroporation
Electroporation is an elegant approach that create pores on the EV membrane to allow the penetration of the therapeutic molecules into the EVs, mostly RNAs and hydrophilic compounds (Alvarez-Erviti et al., 2011; Tian et al., 2014; Liao et al., 2018). Alvarez-Erviti et al. loaded siRNA into exosomes successful by electroporation at 400 V and 125 μF, achieving the knockdown of a target protease in Alzheimer's disease (Alvarez-Erviti et al., 2011). Nevertheless, Kooijmans et al. (2013) reported later that electroporation caused extensive siRNA aggregate formation, which could co-precipitate with exosomes by centrifugation and obscured the loading efficacy. Moreover, the pulses may affect the zeta potential and stability of EVs and increase EV aggregation (Syn et al., 2017).
Others
Additionally, there are several other methods used for drug loading in EVs, such as sonication, extrusion, and freeze-thaw cycles (Syn et al., 2017; Liao et al., 2018). Sonication is an effective method with high loading efficiency, but is often restricted to the loading of smaller non-biologic molecules. Besides, EV integrity and the loss of intrinsic contents and biological properties after the loading process also deserve further attention.
Nucleic Acid Delivery
It is known that EVs naturally carry nucleic acids, making them stable in the circulation and protecting from degradation. Given this, EVs may offer unique advantages for genetic therapy, and key studies using EVs as carriers for genetic materials are highlighted below. The first report on EV-mediated transfer of exogenous nucleic acids was published in 2010, when it was shown that THP-1 cells, which were transfected with a miR-150 mimic, secreted miR-150-enriched EVs and that could be functionally delivered to recipient cells (Zhang et al., 2010). A subsequent study conducted by Akao et al. found that THP-1 monocytes transfected with miR-143 mimic ex vivo secreted miR-143-containing EVs in nude mice after intravenous injection (Akao et al., 2011). Furthermore, when injected intravenously into UUO mice, engineered MSCs that overexpressed miR-let7c attenuated renal fibrosis via secreting miR-let7c-loaded exosomes (Wang et al., 2016). All these studies have elegantly corroborated the effectiveness of miRNA transfer by EVs.
Small interference RNA (siRNA) is used to inhibit mRNA translation and has great potential for the treatment of a range of diseases. Several studies have been conducted to test the feasibility of using EVs as delivery vehicle for siRNA, and the first study conducted by Alvarez-Erviti et al. found that by expressing a neuron-targeting protein on the surface of exosomes, they could specifically deliver siRNA to the brain resulting in a specific gene knockdown (Alvarez-Erviti et al., 2011). Importantly, the treatment displayed minimal toxicity and immune stimulation, even following repeated administration, suggesting EVs are suitable delivery vectors in RNA interference therapy. This notion has been further confirmed by Wahlgren et al. that the gene MAPK1 was selectively silenced in monocytes and lymphocytes by using siRNA-loaded exosomes derived from human plasma (Wahlgren et al., 2012). More recently, an elegant study employed fibroblast-like mesenchymal cell-derived exosomes to deliver siRNA or short hairpin RNA specific to oncogenic KRAS, achieving enhanced therapeutic efficacy in suppressing tumor growth and improving the overall survival (Kamerkar et al., 2017). Notably, the therapeutic effects of engineered exosomes were greater than siRNA-loaded liposomes (Kamerkar et al., 2017). Beyond miRNA and siRNA delivery, EVs were also exploited to encapsulate adeno-associated viruses (AAVs), which were substantially more efficient than free AAVs for the delivery of genetic cargo into recipient cells (Maguire et al., 2012). Collectively, these studies emphasize the potential of using EVs for the therapeutic delivery of nucleic acids.
Protein Delivery
In addition to delivering nucleic acids, EVs are also used to deliver large molecules such as proteins. Haney and colleagues found that exosomes loaded with the antioxidant protein catalase (a high molecular weight enzyme, 240 kDa) was successfully delivered across the blood brain barrier (BBB) and provided significant neuroprotective effects in a model of Parkinson's disease (Haney et al., 2015). In this study catalase was incorporated into pre-assembled exosomes ex vivo using different methods, and identified sonication and extrusion approaches achieved better loading efficiency, sustained release, and protein preservation (Haney et al., 2015). Similar results were reported by Yuan et al., showing that macrophage-derived exosomes efficiently crossed the BBB and delivered a cargo protein to the brain, further indicating the potency of EVs as nanocarriers for brain delivery of therapeutic proteins (Yuan et al., 2017). The cargo protein in the study was loaded in an exogenous way by mixing with exosomes, in addition, the therapeutic protein can be packaged into EVs by transfecting parental cells as well. For example, HEK-293T cells transfected with suicide gene secreted EVs enriched in suicide mRNA and protein, which were subsequently used to treat Schwannoma tumor in an orthotopic mouse model, leading to reduced tumor growth (Mizrak et al., 2013). Overall, these studies suggest that EVs can serve as novel nanocarriers to effectively deliver therapeutic proteins.
Drug Delivery
EVs have been utilized as delivery vehicles for therapeutic drugs in extensive research (Sun et al., 2010; Zhuang et al., 2011; Tang et al., 2012; Yang et al., 2015). Early studies demonstrated an anti-inflammatory small molecule compound curcumin could be incorporated into exosomes by mixing curcumin with murine tumor cell line (EL-4) or microglia cell (JSI124)-derived exosomes, and found that exosomal curcumin exhibited enhanced anti-inflammatory activity in LPS-induced septic shock mouse model (Sun et al., 2010; Zhuang et al., 2011). Interestingly, exosomal packaging lead to an increase in the solubility, stability and bioavailability of curcumin (Sun et al., 2010), suggesting EVs are capable to modify the bioavailability of the native drug. For another natural phytochemical compound celastrol, exosome-mediated delivery also improved drug biodistribution and subsequently enhanced its anti-tumor efficacy (Aqil et al., 2016). This study further highlighted the benefits of EVs in enhancing the functionality of drugs, such as solubility, stability and bioavailability.
In addition, the deployment of EVs encapsulating chemotherapeutics such as paclitaxel and doxorubicin has yielded promising results, representing encouraging anti-cancer efficacy with minimal cytotoxicity toward non-cancerous cells (Tang et al., 2012; Jang et al., 2013; Pascucci et al., 2014; Tian et al., 2014; Saari et al., 2015; Toffoli et al., 2015; Yang et al., 2015; Martins-Marques et al., 2016; Srivastava et al., 2016; Syn et al., 2017). For example, anti-cancer drug-loaded exosomes or exosome-like vesicles were shown to traffic to tumor tissue and reduce tumor growth in mice without overt adverse effects (Jang et al., 2013; Tian et al., 2014). Importantly, exosomes had superior therapeutic effects when compared to liposomes (Jang et al., 2013). Moreover, the administration of doxorubicin loaded in exosomes resulted in significantly less drug accumulation in non-target organs and prevented the onset of off-target cardiotoxicity compared with mice treated with unmodified doxorubicin (Saari et al., 2015; Toffoli et al., 2015; Martins-Marques et al., 2016; Srivastava et al., 2016). Thus, the advantages of exosomes packaging may improve the safety profile of cytotoxic agents and present further opportunities to address cancer therapy.
Benefits and Challenges of Extracellular Vesicle Therapy
Undoubtedly, the field of EV-based therapeutics holds significant promise to enable targeted drug delivery with superior efficiency (Table 2). Compared with existing liposomes or polymeric nanoparticles, the outstanding advantage of EV-based therapy is their naturally lipid and surface protein composition, which enable them to evade phagocytosis, extend blood half-life, and reduce long-term safety issues. For example, CD47 on exosomes can limit their clearance by circulating monocytes (Kamerkar et al., 2017). EVs derived from inflammatory cells expressing integrins or adhesion molecules elicit homing affinities to inflamed tissues (Yuan et al., 2017). The intrinsic contents and biological properties of EVs should be considered and utilized when developing EV-based therapeutics for kidney diseases. Moreover, the small size of EVs facilitates their extravasation, translocation through physical barriers, and passage through extracellular matrix (van den Boorn et al., 2011; van Dommelen et al., 2012). Several studies have demonstrated that EVs successfully cross the BBB and deliver cargos into the brain, but whether EVs are able to pass through the glomerular filtration barrier in healthy states remains unclear. In addition, EVs encapsulation also makes the new drug candidates such as proteins and nucleic acids more stable and targetable to treatment site (Zhu et al., 2012; Bruno et al., 2013).
However, before EV-based therapy can be translated to the clinic, several hurdles need to be overcome (Table 2). First, many properties and mechanisms about EV biology such as the biochemical composition of EV currently remain elusive, and the production or uptake mechanism yet poorly described. Even though from the same cell types, EVs may have contradictory effects as a consequence of differences in cell culture conditions, differences in the purification protocols used or due to a lack of robust extracellular vesicle characterization (Zhu et al., 2012; Bruno et al., 2013; EL Andaloussi et al., 2013). In addition, a major bottleneck in the translation of EV-based therapy into clinic is the lack of good manufacturing practice (GMP) standards. To develop clinical-grade EVs, sterile generation, high scale and efficient production of sufficient amounts of EVs with therapeutic payloads for clinical testing are required. Very recently, Mendt and colleagues have illustrated the process and feasibility of generating GMP-grade exosomes (Mendt et al., 2018). Finally, regarding to the particularity of kidney, the glomerular filtration barrier is the primary obstacle that excludes EVs from accessing podocytes or tubular cells (Kamaly et al., 2016). Under normal conditions, only water and small solutes with a molecular weight less than that of albumin (68 kDa) and a hydrodynamic diameter <5–7 nm are allowed to cross the barrier. In disease, the breakdown of the barrier, especially enlarged endothelial gaps, can aid the accumulation of EVs in various kidney cells and components (Kamaly et al., 2016). However, the level of EVs in the kidney is highly restricted based on the degree of injury to the glomerulus. Fortunately, strategies adapted from the nanomedicines, including engineering of the size, shape, and surface charge of EVs, are valid approaches, but how to apply them in EV-based therapy needs to be further investigated.
Conclusion
The field of EV-based therapy has expanded greatly over the last few years, and application of this strategy to renal disease therapy should have profound translational potential. In this review, we concentrate on emerging therapies including targeting EVs to inhibit their pathogenic effects, exploiting their innate potential for renal regenerative medicine, or using them for robust drug delivery. Although EVs provide an enormous promise and a fresh therapeutic area for nephrotherapy, several key scientific and technical issues need to be urgently addressed, ranging from their preparation, functionalization, and characterization, to their individualized application (Figure 3). Fortunately, numerous studies originally intended for cancer therapy can help us to kick-start the era of EV-based therapy for renal diseases.
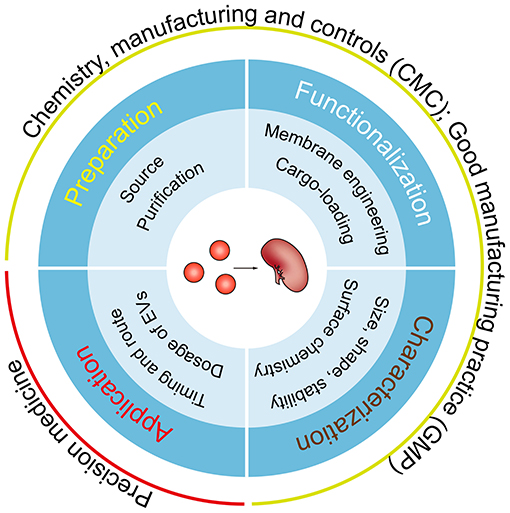
Figure 3. Key considerations for EV-based clinical applications. A number of key steps require optimization before clinical translation, including the source and the purification method of EVs, feasible approaches for membrane engineering and cargo-loading, more comprehensive and precision characterization methods, and the dose, route and timing of EV administration. Of note, all the steps must meet CMC and GMP requirements and the needs of individualized treatment.
Author Contributions
All authors listed have made a substantial, direct and intellectual contribution to the work, and approved it for publication.
Funding
This study was supported by grants from the National Key R&D Program of China (2018YFC1314000), National Natural Science Foundation of China (No.81720108007, 81670696, 81470922 and 31671194), the Clinic Research Center of Jiangsu Province (No. BL2014080), and the Postgraduate Research & Practice Innovation Program of Jiangsu Province (No. KYCX18_0171).
Conflict of Interest Statement
The authors declare that the research was conducted in the absence of any commercial or financial relationships that could be construed as a potential conflict of interest.
References
Akao, Y., Iio, A., Itoh, T., Noguchi, S., Itoh, Y., Ohtsuki, Y., et al. (2011). Microvesicle-mediated RNA molecule delivery system using monocytes/macrophages. Mol. Ther. 19, 395–399. doi: 10.1038/mt.2010.254
Al-Nedawi, K., Meehan, B., Kerbel, R. S., Allison, A. C., and Rak, J. (2009). Endothelial expression of autocrine VEGF upon the uptake of tumor-derived microvesicles containing oncogenic EGFR. Proc. Natl. Acad. Sci. U.S.A. 106, 3794–3799. doi: 10.1073/pnas.0804543106
Alvarez-Erviti, L., Seow, Y., Yin, H., Betts, C., Lakhal, S., and Wood, M. J. (2011). Delivery of siRNA to the mouse brain by systemic injection of targeted exosomes. Nat. Biotechnol. 29, 341–345. doi: 10.1038/nbt.1807
Aqil, F., Kausar, H., Agrawal, A. K., Jeyabalan, J., Kyakulaga, A. H., Munagala, R., et al. (2016). Exosomal formulation enhances therapeutic response of celastrol against lung cancer. Exp. Mol. Pathol. 101, 12–21. doi: 10.1016/j.yexmp.2016.05.013
Arvidsson, I., Ståhl, A. L., Hedström, M. M., Kristoffersson, A. C., Rylander, C., Westman, J. S., et al. (2015). Shiga toxin-induced complement-mediated hemolysis and release of complement-coated red blood cell-derived microvesicles in hemolytic uremic syndrome. J. Immunol. 194, 2309–2318. doi: 10.4049/jimmunol.1402470
Barrès, C., Blanc, L., Bette-Bobillo, P., André, S., Mamoun, R., Gabius, H. J., et al. (2010). Galectin-5 is bound onto the surface of rat reticulocyte exosomes and modulates vesicle uptake by macrophages. Blood 115, 696–705. doi: 10.1182/blood-2009-07-231449
Batagov, A. O., Kuznetsov, V. A., and Kurochkin, I. V. (2011). Identification of nucleotide patterns enriched in secreted RNAs as putative cis-acting elements targeting them to exosome nano-vesicles. BMC Genomics 12 (Suppl. 3):S18. doi: 10.1186/1471-2164-12-S3-S18
Batrakova, E. V., and Kim, M. S. (2015). Using exosomes, naturally-equipped nanocarriers, for drug delivery. J. Control. Release 219, 396–405. doi: 10.1016/j.jconrel.2015.07.030
Bobrie, A., Krumeich, S., Reyal, F., Recchi, C., Moita, L. F., Seabra, M. C., et al. (2012). Rab27a supports exosome-dependent and -independent mechanisms that modify the tumor microenvironment and can promote tumor progression. Cancer Res. 72, 4920–4930. doi: 10.1158/0008-5472.CAN-12-0925
Borges, F. T., Melo, S. A., Özdemir, B. C., Kato, N., Revuelta, I., Miller, C. A., et al. (2013). TGF-β1-containing exosomes from injured epithelial cells activate fibroblasts to initiate tissue regenerative responses and fibrosis. J. Am. Soc. Nephrol. 24, 385–392. doi: 10.1681/ASN.2012101031
Bruno, S., Collino, F., Deregibus, M. C., Grange, C., Tetta, C., and Camussi, G. (2013). Microvesicles derived from human bone marrow mesenchymal stem cells inhibit tumor growth. Stem Cells Dev. 22, 758–771. doi: 10.1089/scd.2012.0304
Bruno, S., Grange, C., Collino, F., Deregibus, M. C., Cantaluppi, V., Biancone, L., et al. (2012). Microvesicles derived from mesenchymal stem cells enhance survival in a lethal model of acute kidney injury. PLoS ONE 7:e33115. doi: 10.1371/journal.pone.0033115
Bruno, S., Grange, C., Deregibus, M. C., Calogero, R. A., Saviozzi, S., Collino, F., et al. (2009). Mesenchymal stem cell-derived microvesicles protect against acute tubular injury. J. Am. Soc. Nephrol. 20, 1053–1067. doi: 10.1681/ASN.2008070798
Burger, D., Viñas, J. L., Akbari, S., Dehak, H., Knoll, W., Gutsol, A., et al. (2015). Human endothelial colony-forming cells protect against acute kidney injury: role of exosomes. Am. J. Pathol. 185, 2309–2323. doi: 10.1016/j.ajpath.2015.04.010
Camussi, G., Deregibus, M. C., Bruno, S., Cantaluppi, V., and Biancone, L. (2010). Exosomes/microvesicles as a mechanism of cell-to-cell communication. Kidney Int. 78, 838–848. doi: 10.1038/ki.2010.278
Cantaluppi, V., Gatti, S., Medica, D., Figliolini, F., Bruno, S., Deregibus, M. C., et al. (2012). Microvesicles derived from endothelial progenitor cells protect the kidney from ischemia-reperfusion injury by microRNA-dependent reprogramming of resident renal cells. Kidney Int. 82, 412–427. doi: 10.1038/ki.2012.105
Cantaluppi, V., Medica, D., Mannari, C., Stiaccini, G., Figliolini, F., Dellepiane, S., et al. (2014). Endothelial progenitor cell-derived extracellular vesicles protect from complement-mediated mesangial injury in experimental anti-Thy1.1 glomerulonephritis. Nephrol. Dial. Transplant. 30, 410–422. doi: 10.1093/ndt/gfu364
Chalmin, F., Ladoire, S., Mignot, G., Vincent, J., Bruchard, M., Remy-Martin, J. P., et al. (2010). Membrane-associated Hsp72 from tumor-derived exosomes mediates STAT3-dependent immunosuppressive function of mouse and human myeloid-derived suppressor cells. J. Clin. Invest. 120, 457–471. doi: 10.1172/JCI40483
Choi, H. Y., Lee, H. G., Kim, B. S., Ahn, S. H., Jung, A., Lee, M., et al. (2015). Mesenchymal stem cell-derived microparticles ameliorate peritubular capillary rarefaction via inhibition of endothelial-mesenchymal transition and decrease tubulointerstitial fibrosis in unilateral ureteral obstruction. Stem Cell Res. Ther. 6:18. doi: 10.1186/s13287-015-0012-6
Choi, H. Y., Moon, S. J., Ratliff, B. B., Ahn, S. H., Jung, A., Lee, M., et al. (2014). Microparticles from kidney-derived mesenchymal stem cells act as carriers of proangiogenic signals and contribute to recovery from acute kidney injury. PLoS ONE. 9:e87853. doi: 10.1371/journal.pone.0087853
Collino, F., Bruno, S., Incarnato, D., Dettori, D., Neri, F., Provero, P., et al. (2015). AKI recovery induced by mesenchymal stromal cell-derived extracellular vesicles carrying microRNAs. J. Am. Soc. Nephrol. 26, 2349–2360. doi: 10.1681/ASN.2014070710
Colombo, M., Raposo, G., and Théry, C. (2014). Biogenesis, secretion, and intercellular interactions of exosomes and other extracellular vesicles. Annu. Rev. Cell Dev. Biol. 30, 255–289. doi: 10.1146/annurev-cellbio-101512-122326
Dasgupta, S. K., Le, A., Chavakis, T., Rumbaut, R. E., and Thiagarajan, P. (2012). Developmental endothelial locus-1 (Del-1) mediates clearance of platelet microparticles by the endothelium. Circulation 125, 1664–1672. doi: 10.1161/CIRCULATIONAHA.111.068833
Dominguez, J. H., Liu, Y., Gao, H., Dominguez, J. M. II., Xie, D., et al. (2017). Renal tubular cell-derived extracellular vesicles accelerate the recovery of established renal ischemia reperfusion injury. J. Am. Soc. Nephrol. 28, 3533–3544. doi: 10.1681/ASN.2016121278
Eirin, A., Zhu, X. Y., Puranik, A. S., Tang, H., McGurren, K. A., van Wijnen, A. J., et al. (2017). Mesenchymal stem cell-derived extracellular vesicles attenuate kidney inflammation. Kidney Int. 92, 114–124. doi: 10.1016/j.kint.2016.12.023
EL Andaloussi, S., Mäger, I., Breakefield, X. O., and Wood, M. J. (2013). Extracellular vesicles: biology and emerging therapeutic opportunities. Nat. Rev. Drug Discov. 12, 347–357. doi: 10.1038/nrd3978
Erdbrügger, U., and Le, T. H. (2016). Extracellular vesicles in renal diseases: more than novel biomarkers? J. Am. Soc. Nephrol. 27, 12–26. doi: 10.1681/ASN.2015010074
Faille, D., El-Assaad, F., Mitchell, A. J., Alessi, M. C., Chimini, G., Fusai, T., et al. (2012). Endocytosis and intracellular processing of platelet microparticles by brain endothelial cells. J. Cell. Mol. Med. 16, 1731–1738. doi: 10.1111/j.1582-4934.2011.01434.x
Fuhrmann, G., Herrmann, I. K., and Stevens, M. M. (2015). Cell-derived vesicles for drug therapy and diagnostics: opportunities and challenges. Nano Today 10, 397–409. doi: 10.1016/j.nantod.2015.04.004
Furini, G., Schroeder, N., Huang, L., Boocock, D., Scarpellini, A., Coveney, C., et al. (2018). Proteomic profiling reveals the transglutaminase-2 externalization pathway in kidneys after unilateral ureteric obstruction. J. Am. Soc. Nephrol. 29, 880–905. doi: 10.1681/ASN.2017050479
Gatti, S., Bruno, S., Deregibus, M. C., Sordi, A., Cantaluppi, V., Tetta, C., et al. (2011). Microvesicles derived from human adult mesenchymal stem cells protect against ischaemia-reperfusion-induced acute and chronic kidney injury. Nephrol. Dial. Transplant. 26, 1474–1483. doi: 10.1093/ndt/gfr015
Grange, C., Iampietro, C., and Bussolati, B. (2017). Stem cell extracellular vesicles and kidney injury. Stem Cell Investig. 4:90. doi: 10.21037/sci.2017.11.02
Gu, D., Zou, X., Ju, G., Zhang, G., Bao, E., and Zhu, Y. (2016). Mesenchymal stromal cells derived extracellular vesicles ameliorate acute renal ischemia reperfusion injury by inhibition of mitochondrial fission through miR-30. Stem Cells Int. 2016:2093940. doi: 10.1155/2016/2093940
Ha, D., Yang, N., and Nadithe, V. (2016). Exosomes as therapeutic drug carriers and delivery vehicles across biological membranes: current perspectives and future challenges. Acta Pharm. Sin. B 6, 287–296. doi: 10.1016/j.apsb.2016.02.001
Haney, M. J., Klyachko, N. L., Zhao, Y., Gupta, R., Plotnikova, E. G., He, Z., et al. (2015). Exosomes as drug delivery vehicles for Parkinson's disease therapy. J. Control. Release 207, 18–30. doi: 10.1016/j.jconrel.2015.03.033
He, J., Wang, Y., Lu, X., Zhu, B., Pei, X., Wu, J., et al. (2015). Microvesicles derived from bone marrow stem cells protect the kidney both in vivo and in vitro by microRNA-dependent repairing. Nephrology (Carlton) 20, 591–600. doi: 10.1111/nep.12490
Herrera Sanchez, M. B., Bruno, S., Grange, C., Tapparo, M., Cantaluppi, V., Tetta, C., et al. (2014). Human liver stem cells and derived extracellular vesicles improve recovery in a murine model of acute kidney injury. Stem Cell Res. Ther. 5:124. doi: 10.1186/scrt514
Hung, M. E., and Leonard, J. N. (2015). Stabilization of exosome-targeting peptides via engineered glycosylation. J. Biol. Chem. 290, 8166–8172. doi: 10.1074/jbc.M114.621383
Jang, S. C., Kim, O. Y., Yoon, C. M., Choi, D. S., Roh, T. Y., Park, J., et al. (2013). Bioinspired exosome-mimetic nanovesicles for targeted delivery of chemotherapeutics to malignant tumors. ACS Nano 7, 7698–7710. doi: 10.1021/nn402232g
Jiang, Z. Z., Liu, Y. M., Niu, X., Yin, J. Y., Hu, B., Guo, S. C., et al. (2016). Exosomes secreted by human urine-derived stem cells could prevent kidney complications from type I diabetes in rats. Stem Cell Res. Ther. 7:24. doi: 10.1186/s13287-016-0287-2
Ju, G. Q., Cheng, J., Zhong, L., Wu, S., Zou, X. Y., Zhang, G. Y., et al. (2015). Microvesicles derived from human umbilical cord mesenchymal stem cells facilitate tubular epithelial cell dedifferentiation and growth via hepatocyte growth factor induction. PLoS ONE 10:e0121534. doi: 10.1371/journal.pone.0121534
Kamaly, N., He, J. C., Ausiello, D. A., and Farokhzad, O. C. (2016). Nanomedicines for renal disease: current status and future applications. Nat. Rev. Nephrol. 12, 738–753. doi: 10.1038/nrneph.2016.156
Kamerkar, S., LeBleu, V. S., Sugimoto, H., Yang, S., Ruivo, C. F., Melo, S. A., et al. (2017). Exosomes facilitate therapeutic targeting of oncogenic KRAS in pancreatic cancer. Nature 546, 498–503. doi: 10.1038/nature22341
Karpman, D., Ståhl, A. L., and Arvidsson, I. (2017). Extracellular vesicles in renal disease. Nat. Rev. Nephrol. 13, 545–562. doi: 10.1038/nrneph.2017.98
Kholia, S., Herrera Sanchez, M. B., Cedrino, M., Papadimitriou, E., Tapparo, M., Deregibus, M. C., et al. (2018). Human liver stem cell-derived extracellular vesicles prevent aristolochic acid-induced kidney fibrosis. Front. Immunol. 9:1639. doi: 10.3389/fimmu.2018.01639
Kim, H. S., Choi, D. Y., Yun, S. J., Choi, S. M., Kang, J. W., Jung, J. W., et al. (2012). Proteomic analysis of microvesicles derived from human mesenchymal stem cells. J. Proteome Res. 11, 839–849. doi: 10.1021/pr200682z
Kooijmans, S. A. A., Stremersch, S., Braeckmans, K., de Smedt, S. C., Hendrix, A., Wood, M. J. A., et al. (2013). Electroporation-induced siRNA precipitation obscures the efficiency of siRNA loading into extracellular vesicles. J. Control Release 172, 229–238. doi: 10.1016/j.jconrel.2013.08.014
Lee, J., Kim, J., Jeong, M., Lee, H., Goh, U., Kim, H., et al. (2015). Liposome-based engineering of cells to package hydrophobic compounds in membrane vesicles for tumor penetration. Nano Lett. 15, 2938–2944. doi: 10.1021/nl5047494
Li, Z. L., Lv, L. L., Tang, T. T., Wang, B., Feng, Y., Zhou, L. T., et al. (2019). HIF-1α inducing exosomal microRNA-23a expression mediates the cross-talk between tubular epithelial cells and macrophages in tubulointerstitial inflammation. Kidney Int. 95, 388–404. doi: 10.1016/j.kint.2018.09.013
Liao, W., Du, Y., Zhang, C., Pan, F., Yao, Y., Zhang, T., et al. (2018). Exosomes: the next generation of endogenous nanomaterials for advanced drug delivery and therapy. Acta Biomater. 86, 1–14. doi: 10.1016/j.actbio.2018.12.045
Lima, L. G., Chammas, R., Monteiro, R. Q., Moreira, M. E., and Barcinski, M. A. (2009). Tumor-derived microvesicles modulate the establishment of metastatic melanoma in a phosphatidylserine-dependent manner. Cancer Lett. 283, 168–175. doi: 10.1016/j.canlet.2009.03.041
Lin, K. C., Yip, H. K., Shao, P. L., Wu, S. C., Chen, K. H., Chen, Y. T., et al. (2016). Combination of adipose-derived mesenchymal stem cells (ADMSC) and ADMSC-derived exosomes for protecting kidney from acute ischemia-reperfusion injury. Int. J. Cardiol. 216, 173–185. doi: 10.1016/j.ijcard.2016.04.061
Liu, B. C., Tang, T. T., Lv, L. L., and Lan, H. Y. (2018). Renal tubule injury: a driving force toward chronic kidney disease. Kidney Int. 93, 568–579. doi: 10.1016/j.kint.2017.09.033
Lv, L. H., Wan, Y. L., Lin, Y., Zhang, W., Yang, M., Li, G. L., et al. (2012). Anticancer drugs cause release of exosomes with heat shock proteins from human hepatocellular carcinoma cells that elicit effective natural killer cell antitumor responses in vitro. J. Biol. Chem. 287, 15874–15885. doi: 10.1074/jbc.M112.340588
Lv, L. L., Feng, Y., Wen, Y., Wu, W. J., Ni, H. F., Li, Z. L., et al. (2018a). Exosomal CCL2 from tubular epithelial cells is critical for albumin-induced tubulointerstitial inflammation. J. Am. Soc. Nephrol. 29, 919–935. doi: 10.1681/ASN.2017050523
Lv, L. L., Wu, W. J., Feng, Y., Li, Z. L., Tang, T. T., and Liu, B. C. (2018b). Therapeutic application of extracellular vesicles in kidney disease: promises and challenges. J. Cell. Mol. Med. 22, 728–737. doi: 10.1111/jcmm.13407
Maguire, C. A., Balaj, L., Sivaraman, S., Crommentuijn, M. H., Ericsson, M., Mincheva-Nilsson, L., et al. (2012). Microvesicle-associated AAV vector as a novel gene delivery system. Mol. Ther. 20, 960–971. doi: 10.1038/mt.2011.303
Martins-Marques, T., Pinho, M. J., Zuzarte, M., Oliveira, C., Pereira, P., Sluijter, J. P., et al. (2016). Presence of Cx43 in extracellular vesicles reduces the cardiotoxicity of the anti-tumour therapeutic approach with doxorubicin. J. Extracell. Vesicles 5:32538. doi: 10.3402/jev.v5.32538
Mendt, M., Kamerkar, S., Sugimoto, H., McAndrews, K. M., Wu, C. C., Gagea, M., et al. (2018). Generation and testing of clinical-grade exosomes for pancreatic cancer. JCI Insight 3:99263. doi: 10.1172/jci.insight.99263
Mizrak, A., Bolukbasi, M. F., Ozdener, G. B., Brenner, G. J., Madlener, S., Erkan, E. P., et al. (2013). Genetically engineered microvesicles carrying suicide mRNA/protein inhibit schwannoma tumor growth. Mol. Ther. 21, 101–108. doi: 10.1038/mt.2012.161
Morel, O., Jesel, L., Freyssinet, J. M., and Toti, F. (2011). Cellular mechanisms underlying the formation of circulating microparticles. Arterioscler. Thromb. Vasc. Biol. 31, 15–26. doi: 10.1161/ATVBAHA.109.200956
Morrison, E. E., Bailey, M. A., and Dear, J. W. (2016). Renal extracellular vesicles: from physiology to clinical application. J. Physiol. 594, 5735–5748. doi: 10.1113/JP272182
Mossberg, M., Ståhl, A. L., Kahn, R., Kristoffersson, A. C., Tati, R., Heijl, C., et al. (2017). C1-Inhibitor decreases the release of vasculitis-like chemotactic endothelial microvesicles. J. Am. Soc. Nephrol. 28, 2472–2481. doi: 10.1681/ASN.2016060637
Mulcahy, L. A., Pink, R. C., and Carter, D. R. (2014). Routes and mechanisms of extracellular vesicle uptake. J. Extracell. Vesicles 3:24641. doi: 10.3402/jev.v3.24641
Nagaishi, K., Mizue, Y., Chikenji, T., Otani, M., Nakano, M., Konari, N., et al. (2016). Mesenchymal stem cell therapy ameliorates diabetic nephropathy via the paracrine effect of renal trophic factors including exosomes. Sci. Rep. 6:34842. doi: 10.1038/srep34842
Nargesi, A. A., Lerman, L. O., and Eirin, A. (2017). Mesenchymal stem cell-derived extracellular vesicles for renal repair. Curr. Gene Ther. 17, 29–42. doi: 10.2174/1566523217666170412110724
Ohno, S., Takanashi, M., Sudo, K., Ueda, S., Ishikawa, A., Matsuyama, N., et al. (2013). Systemically injected exosomes targeted to EGFR deliver antitumor microRNA to breast cancer cells. Mol. Ther. 21, 185–191. doi: 10.1038/mt.2012.180
Ostrowski, M., Carmo, N. B., Krumeich, S., Fanget, I., Raposo, G., Savina, A., et al. (2010). Rab27a and Rab27b control different steps of the exosome secretion pathway. Nat. Cell Biol. 12, 19–30. doi: 10.1038/ncb2000
Pascucci, L., Coccè, V., Bonomi, A., Ami, D., Ceccarelli, P., Ciusani, E., et al. (2014). Paclitaxel is incorporated by mesenchymal stromal cells and released in exosomes that inhibit in vitro tumor growth: a new approach for drug delivery. J. Control. Release 192, 262–270. doi: 10.1016/j.jconrel.2014.07.042
Peinado, H., Alečković, M., Lavotshkin, S., Matei, I., Costa-Silva, B., Moreno-Bueno, G., et al. (2012). Melanoma exosomes educate bone marrow progenitor cells toward a pro-metastatic phenotype through MET. Nat. Med. 18, 883–891. doi: 10.1038/nm.2753
Ranghino, A., Bruno, S., Bussolati, B., Moggio, A., Dimuccio, V., Tapparo, M., et al. (2017). The effects of glomerular and tubular renal progenitors and derived extracellular vesicles on recovery from acute kidney injury. Stem Cell Res. Ther. 8:24. doi: 10.1186/s13287-017-0478-5
Rani, S., Ryan, A. E., Griffin, M. D., and Ritter, T. (2015). Mesenchymal stem cell-derived extracellular vesicles: toward cell-free therapeutic applications. Mol. Ther. 23, 812–823. doi: 10.1038/mt.2015.44
Raposo, G., and Stoorvogel, W. (2013). Extracellular vesicles: exosomes, microvesicles, and friends. J. Cell Biol. 200, 373–383. doi: 10.1083/jcb.201211138
Saari, H., Lázaro-Ibáñez, E., Viitala, T., Vuorimaa-Laukkanen, E., Siljander, P., and Yliperttula, M. (2015). Microvesicle- and exosome-mediated drug delivery enhances the cytotoxicity of Paclitaxel in autologous prostate cancer cells. J. Control. Release 220, 727–737. doi: 10.1016/j.jconrel.2015.09.031
Shen, B., Liu, J., Zhang, F., Wang, Y., Qin, Y., Zhou, Z., et al. (2016). CCR2 positive exosome released by mesenchymal stem cells suppresses macrophage functions and alleviates ischemia/reperfusion-induced renal injury. Stem Cells Int. 2016:1240301. doi: 10.1155/2016/1240301
Shtam, T. A., Kovalev, R. A., Varfolomeeva, E. Y., Makarov, E. M., Kil, Y. V., and Filatov, M. V. (2013). Exosomes are natural carriers of exogenous siRNA to human cells in vitro. Cell Commun. Signal. 11:88. doi: 10.1186/1478-811X-11-88
Srivastava, A., Amreddy, N., Babu, A., Panneerselvam, J., Mehta, M., Muralidharan, R., et al. (2016). Nanosomes carrying doxorubicin exhibit potent anticancer activity against human lung cancer cells. Sci. Rep. 6:38541. doi: 10.1038/srep38541
Sun, D., Zhuang, X., Xiang, X., Liu, Y., Zhang, S., Liu, C., et al. (2010). A novel nanoparticle drug delivery system: the anti-inflammatory activity of curcumin is enhanced when encapsulated in exosomes. Mol. Ther. 18, 1606–1614. doi: 10.1038/mt.2010.105
Syn, N. L., Wang, L., Chow, E. K., Lim, C. T., and Goh, B. C. (2017). Exosomes in cancer nanomedicine and immunotherapy: prospects and challenges. Trends Biotechnol. 35, 665–676. doi: 10.1016/j.tibtech.2017.03.004
Tang, K., Zhang, Y., Zhang, H., Xu, P., Liu, J., Ma, J., et al. (2012). Delivery of chemotherapeutic drugs in tumour cell-derived microparticles. Nat. Commun. 3:1282. doi: 10.1038/ncomms2282
Tian, Y., Li, S., Song, J., Ji, T., Zhu, M., Anderson, G. J., et al. (2014). A doxorubicin delivery platform using engineered natural membrane vesicle exosomes for targeted tumor therapy. Biomaterials 35, 2383–2390. doi: 10.1016/j.biomaterials.2013.11.083
Toffoli, G., Hadla, M., Corona, G., Caligiuri, I., Palazzolo, S., Semeraro, S., et al. (2015). Exosomal doxorubicin reduces the cardiac toxicity of doxorubicin. Nanomedicine 10, 2963–2971. doi: 10.2217/nnm.15.118
Trajkovic, K., Hsu, C., Chiantia, S., Rajendran, L., Wenzel, D., Wieland, F., et al. (2008). Ceramide triggers budding of exosome vesicles into multivesicular endosomes. Science 319, 1244–1247. doi: 10.1126/science.1153124
van den Boorn, J. G., Schlee, M., Coch, C., and Hartmann, G. (2011). SiRNA delivery with exosome nanoparticles. Nat. Biotechnol. 29, 325–326. doi: 10.1038/nbt.1830
van der Pol, E., Böing, A. N., Harrison, P., Sturk, A., and Nieuwland, R. (2012). Classification, functions, and clinical relevance of extracellular vesicles. Pharmacol. Rev. 64, 676–705. doi: 10.1124/pr.112.005983
van Dommelen, S. M., Vader, P., Lakhal, S., Kooijmans, S. A., van Solinge, W. W., Wood, M. J., et al. (2012). Microvesicles and exosomes: opportunities for cell-derived membrane vesicles in drug delivery. J. Control. Release 161, 635–644. doi: 10.1016/j.jconrel.2011.11.021
Viñas, J. L., Burger, D., Zimpelmann, J., Haneef, R., Knoll, W., Campbell, P., et al. (2016). Transfer of microRNA-486-5p from human endothelial colony forming cell-derived exosomes reduces ischemic kidney injury. Kidney Int. 90, 1238–1250. doi: 10.1016/j.kint.2016.07.015
Wahlgren, J., De L Karlson, T., Brisslert, M., Vaziri Sani, F., Telemo, E., Sunnerhagen, P., et al. (2012). Plasma exosomes can deliver exogenous short interfering RNA to monocytes and lymphocytes. Nucleic Acids Res. 40:e130. doi: 10.1093/nar/gks463
Wang, B., Yao, K., Huuskes, B. M., Shen, H. H., Zhuang, J., Godson, C., et al. (2016). Mesenchymal stem cells deliver exogenous microRNA-let7c via exosomes to attenuate renal fibrosis. Mol. Ther. 24, 1290–1301. doi: 10.1038/mt.2016.90
Wang, Y., Lu, X., He, J., and Zhao, W. (2015). Influence of erythropoietin on microvesicles derived from mesenchymal stem cells protecting renal function of chronic kidney disease. Stem Cell Res. Ther. 6:100. doi: 10.1186/s13287-015-0095-0
Wolf, P. (1967). The nature and significance of platelet products in human plasma. Br. J. Haematol. 13, 269–288. doi: 10.1111/j.1365-2141.1967.tb08741.x
Yang, B., Chen, Y., and Shi, J. (2018). Exosome biochemistry and advanced nanotechnology for next-generation theranostic platforms. Adv. Mater. Weinheim. 20:e1802896. doi: 10.1002/adma.201802896
Yang, T., Martin, P., Fogarty, B., Brown, A., Schurman, K., Phipps, R., et al. (2015). Exosome delivered anticancer drugs across the blood-brain barrier for brain cancer therapy in Danio rerio. Pharm. Res. 32, 2003–2014. doi: 10.1007/s11095-014-1593-y
Yano, Y., Shiba, E., Kambayashi, J., Sakon, M., Kawasaki, T., Fujitani, K., et al. (1993). The effects of calpeptin (a calpain specific inhibitor) on agonist induced microparticle formation from the platelet plasma membrane. Thromb. Res. 71, 385–396. doi: 10.1016/0049-3848(93)90163-I
Yim, N., Ryu, S. W., Choi, K., Lee, K. R., Lee, S., Choi, H., et al. (2016). Exosome engineering for efficient intracellular delivery of soluble proteins using optically reversible protein-protein interaction module. Nat. Commun. 7:12277. doi: 10.1038/ncomms12277
Yuan, D., Zhao, Y., Banks, W. A., Bullock, K. M., Haney, M., Batrakova, E., et al. (2017). Macrophage exosomes as natural nanocarriers for protein delivery to inflamed brain. Biomaterials 142, 1–12. doi: 10.1016/j.biomaterials.2017.07.011
Zafrani, L., Gerotziafas, G., Byrnes, C., Hu, X., Perez, J., Lévi, C., et al. (2012). Calpastatin controls polymicrobial sepsis by limiting procoagulant microparticle release. Am. J. Respir. Crit. Care Med. 185, 744–755. doi: 10.1164/rccm.201109-1686OC
Zeelenberg, I. S., Ostrowski, M., Krumeich, S., Bobrie, A., Jancic, C., Boissonnas, A., et al. (2008). Targeting tumor antigens to secreted membrane vesicles in vivo induces efficient antitumor immune responses. Cancer Res. 68, 1228–1235. doi: 10.1158/0008-5472.CAN-07-3163
Zhang, G., Zou, X., Huang, Y., Wang, F., Miao, S., Liu, G., et al. (2016). Mesenchymal stromal cell-derived extracellular vesicles protect against acute kidney injury through anti-oxidation by enhancing Nrf2/ARE activation in rats. Kidney Blood Press. Res. 41, 119–128. doi: 10.1159/000443413
Zhang, W., Zhou, X., Zhang, H., Yao, Q., Liu, Y., and Dong, Z. (2016). Extracellular vesicles in diagnosis and therapy of kidney diseases. Am. J. Physiol. Renal Physiol. 311, F844–F851. doi: 10.1152/ajprenal.00429.2016
Zhang, Y., Liu, D., Chen, X., Li, J., Li, L., Bian, Z., et al. (2010). Secreted monocytic miR-150 enhances targeted endothelial cell migration. Mol. Cell 39, 133–144. doi: 10.1016/j.molcel.2010.06.010
Zhou, Y., Xiong, M., Fang, L., Jiang, L., Wen, P., Dai, C., et al. (2013a). miR-21-containing microvesicles from injured tubular epithelial cells promote tubular phenotype transition by targeting PTEN protein. Am. J. Pathol. 183, 1183–1196. doi: 10.1016/j.ajpath.2013.06.032
Zhou, Y., Xu, H., Xu, W., Wang, B., Wu, H., Tao, Y., et al. (2013b). Exosomes released by human umbilical cord mesenchymal stem cells protect against cisplatin-induced renal oxidative stress and apoptosis in vivo and in vitro. Stem Cell Res. Ther. 4:34. doi: 10.1186/scrt194
Zhu, W., Huang, L., Li, Y., Zhang, X., Gu, J., Yan, Y., et al. (2012). Exosomes derived from human bone marrow mesenchymal stem cells promote tumor growth in vivo. Cancer Lett. 315, 28–37. doi: 10.1016/j.canlet.2011.10.002
Zhuang, X., Xiang, X., Grizzle, W., Sun, D., Zhang, S., Axtell, R. C., et al. (2011). Treatment of brain inflammatory diseases by delivering exosome encapsulated anti-inflammatory drugs from the nasal region to the brain. Mol. Ther. 19, 1769–1779. doi: 10.1038/mt.2011.164
Zou, X., Gu, D., Xing, X., Cheng, Z., Gong, D., Zhang, G., et al. (2016). Human mesenchymal stromal cell-derived extracellular vesicles alleviate renal ischemic reperfusion injury and enhance angiogenesis in rats. Am. J. Transl. Res. 8, 4289–4299.
Zou, X., Kwon, S. H., Jiang, K., Ferguson, C. M., Puranik, A. S., Zhu, X., et al. (2018). Renal scattered tubular-like cells confer protective effects in the stenotic murine kidney mediated by release of extracellular vesicles. Sci. Rep. 8:1263. doi: 10.1038/s41598-018-19750-y
Keywords: extracellular vesicles, renal inflammation, renal fibrosis, treatment, drug delivery
Citation: Tang T-T, Lv L-L, Lan H-Y and Liu B-C (2019) Extracellular Vesicles: Opportunities and Challenges for the Treatment of Renal Diseases. Front. Physiol. 10:226. doi: 10.3389/fphys.2019.00226
Received: 08 December 2018; Accepted: 21 February 2019;
Published: 19 March 2019.
Edited by:
Bellamkonda K. Kishore, University of Utah Health Care, United StatesReviewed by:
Frank Park, University of Tennessee Health Science Center (UTHSC), United StatesCarolyn Mary Ecelbarger, Georgetown University, United States
Copyright © 2019 Tang, Lv, Lan and Liu. This is an open-access article distributed under the terms of the Creative Commons Attribution License (CC BY). The use, distribution or reproduction in other forums is permitted, provided the original author(s) and the copyright owner(s) are credited and that the original publication in this journal is cited, in accordance with accepted academic practice. No use, distribution or reproduction is permitted which does not comply with these terms.
*Correspondence: Lin-Li Lv, bHZsaW5saUBzZXUuZWR1LmNu
Bi-Cheng Liu, bGl1YmM2NEAxNjMuY29t