- 1Department of Life Sciences, University of Modena and Reggio Emilia, Modena, Italy
- 2Padiglione Besta, University of Modena and Reggio Emilia, Reggio Emilia, Italy
Trehalases (treh) have been found in different organisms, such as bacteria, fungi, yeast, nematodes, insects, vertebrates, and plants. Their biochemical properties are extremely variable and not yet fully understood. Gene expression patterns have shown differences among insect species suggesting a potential functional diversification of trehalase enzymes during their evolution. A second gene family encoding for enzymes with hypothetical trehalase activity has been repeatedly annotated in insect genome as acid trehalases/acid trehalase-like (ath), but its functional role is still not clear. The currently available large amount of genomic data from many insect species may enable a better understanding of the evolutionary history, phylogenetic relationships and possible roles of trehalase encoding genes in this taxon. The aim of the present study is to infer the evolutionary history of trehalases and acid trehalase genes in insects and analyze the trehalase functional divergence during their evolution, combining phylogenetic and genomic synteny/colinearity analyses.
Introduction
Trehalases, commonly found in different organisms (such as bacteria, fungi, yeast, nematodes, insects, vertebrates, and plants) (Jorge et al., 1997), catalyze the hydrolysis of trehalose into two glucose molecules (Becker et al., 1996). In insects, trehalases have been shown to exist as a soluble form (tre-1) and as a membrane bound form (tre-2) and they play a pivotal role in energy metabolism, chitin biosynthesis, flight and in many other physiological processes, including development and reproduction (Candy, 1974; Shukla et al., 2014). Combining the different role to the variable biochemical properties of trehalases it has been suggested that a functional diversification of trehalase enzymes occurred during insect evolution (Kalf and Rieder, 1958; Duve, 1972; Terra et al., 1978; Huang et al., 2006; Tang et al., 2008, 2012; Chen et al., 2010; Santos et al., 2012.
At present, the availability of a large set of genomic data from many insect species (Yin et al., 2015) makes possible genomic analyses suggesting that: (i) treh genes frequently experienced gene duplication during insect evolution; (ii) duplicated copies (paralogs) have been retained in several species bringing to treh gene family (Kalf and Rieder, 1958; Duve, 1972; Huang et al., 2006; Tang et al., 2008, 2012; Chen et al., 2010; Santos et al., 2012; Adhav et al., 2018).
Furthermore, the presence of genes coding for acid trehalases/acid trehalase-like (ath) has been evidenced in different insects, such as Bombyx mori (Lepidoptera: Bombycidae) (Mita, 2004) and Plutella xylostella (Lepidoptera: Plutellidae) (You et al., 2013). In other genomes, ath/ath-like sequences are present as well, but not annotated, as in aphid species Acyrthosiphon pisum (Hemiptera: Aphididae; Genome Sequence of the pea aphid A. pisum, 2010), Diuraphis noxia (Hemiptera: Aphididae) (Nicholson et al., 2015) and Myzus persicae (Hemiptera: Aphididae) (Ramsey et al., 2007). Acid trehalases are mainly described in bacteria and fungi (Destruelle et al., 1995; D’Enfert and Fontaine, 1997; Inagaki et al., 2001; Murata et al., 2001) and the origin of such genes in insect genomes is still unexplained.
In view of the consideration that progresses in understanding the molecular characterization of trehalase could favor the use of these proteins as a novel target for insecticides, in the present study we analyzed the evolutionary history of the treh/treh-like and ath/ath-like genes in insects with particular emphasis on phytophagous species, combining genomic analyses (related to the sequence presence/conservation and to synteny and/or co-linearity) to the phylogenetic distribution of the observed gene duplications.
Materials and Methods
The study of treh/treh-like and ath/ath-like gene families has been performed looking at 40 insect species representatives of five different orders. For each species, genes coding for trehalase, trehalase-like, acid trehalase and acid trehalase-like genes have been identified by retrieving the available coding sequences (even if it was not previously annotated) from NCBI online databases1. When trehalase genes were not annotated, transcript sequence from the phylogenetically nearest species has been used to find trehalase genes in the genome of the target species, i.e., Drosophila pseudoobscura (Diptera, Drosophilidae) using annotated D. melanogaster treh gene. M. persicae sequences were retrieved from Aphidbase databases2.
Nucleotide sequences of exons from NCBI predictions have been used to build treh and ath gene family phylogenies. For the construction of the trehalase phylogenetic tree, treh/treh-like genes sequences from different ecdysozoans other than insects were also analyzed, including the nematode Caenorhabiditis elegans (Nematoda: Rhabditidae), the tardigrad Ramazzottius varieornatus (Tardigrada, Hypsibiidae) and the crustacean Artemia franciscana (Crustacea: Branchiopoda).
Exons sequences were analyzed with Virtual Ribosome (Wernersson, 2006) and the predicted coding sequences were aligned with Muscle in MEGA5 (Tamura et al., 2007). The alignment was trimmed to contain only the region between the first and the last conserved domains: VIVPGGR, QWDYPNAWPP, DSKTFVDM, RSQPPL, PRPESYREDY, and ELKAA and glycine rich domain GGGEYE (Barraza and Sánchez, 2013; Xie et al., 2013). The Maximum Likelihood phylogenetic tree was constructed with raxmlGUI (Silvestro and Michalak, 2011) (ML + throught bootstrap, 10 runs, 1000 reps, jModeltest and GTRGAMMAI, outgroup Escherichia coli treh EU893513.1). The phylogenetic tree was visualized and edited in iTOL (Letunic and Bork, 2006). For each conserved domain in each sequence, the p-distance with the protein consensus sequence was computed and visualized on the tree to show the level of conservation of the different trehalase genes.
Since trehalase enzymes have been described in insects in two distinct forms (soluble and membrane bound) (i.e., Gu et al., 2009), the amino-acid sequences have been analyzed with Signalp 4.1 (Petersen et al., 2011) to predict the presence of signal peptides and TMHMM Server v. 2.0 to predict transmembrane domains (Sonnhammer et al., 1998).
Acid trehalases isoforms have been compared considering the distribution of six consensus discrete motifs, besides the catalytic domain: a transmembrane span (LFFFFFFFLCFSFTTSML), a cAMP-dependent phosphorylation site (RRXS), an EF-like Ca2+-binding motif (DTXGDXQITIXD), two trehalase signature motifs 1 and 2, (PGGRFXEXYXWDXY) and (QWDXPX[G/A]W[P/A/S]P), respectively, and the glycosyl phosphatidyl inositol (GPI) membrane anchor motif (CRTNYGYSAA) (Barraza and Sánchez, 2013).
Genomic scaffolds containing treh and ath genes were compared looking for synteny and co-linearity among insect species by analysis of the neighboring genes located in the same scaffold/contigs hosting treh and ath genes.
Results
Identification of treh and ath Genes Currently Available in DNA Databases
The search of Genbank databases allowed us to identify 160 treh/treh-like genes among 40 insect species and 31 ath/ath-like genes in 14 species (Table 1). Except for Dipterans, the other insect taxa have experienced specific treh gene duplications and maintained multiple treh gene copies in their genomes. Hemipteran genome showed the highest number of treh gene duplications (54 treh copies in 7 species). The pea aphid A. pisum possessed the highest number of treh gene copies with 13 treh genes, followed by Aethina tumida (Coleoptera: Nitidulidae) with 11 treh genes and the two aphid species D. noxia and M. persicae with 8 treh copies, respectively (Table 1 and Supplementary Table S1). A. pisum genome possesses a treh pseudogene with a high similarity to plant trehalases, but with a partial coding sequence due to a large deletion in the gene. P. xylostella possessed two treh genes (LOC105397091 and LOC105395616) with high level of sequence similarity with a treh gene encoded by Enterobacter cloacae (Bacteria: Enterobacteriacea; scaffold CP015227).
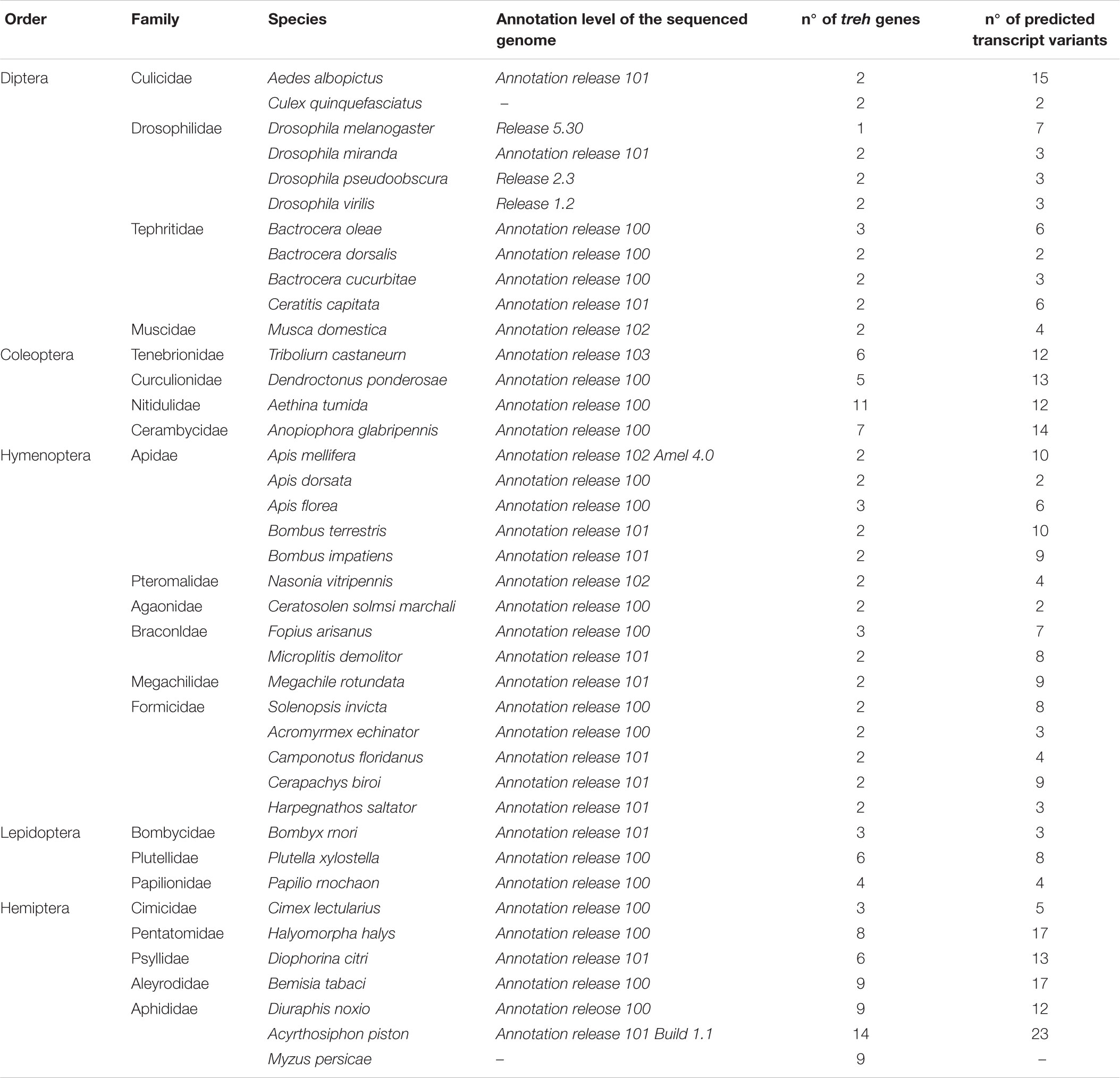
Table 1. List of species and genomes considered in the present study for the search of treh genes and transcripts.
Diptera, Lepidoptera, Hymenoptera and some Hemipteran species mostly possess a unique gene coding for acid trehalase, that resulted not phylogenetically related to bacterial or fungal acid trehalase ath genes. Differently, multiple genes coding for acid trehalases have been identified in A. pisum, M. persicae, P. xylostella, and Bactrocera oleae (Diptera: Tephritidae) since they possessed duplicated ath genes as well (LOC100159015, LOC100167863, MYZPE13164_G006, MYZPE13164_G006, LOC105394851, LOC105386635 and LOC106624493, LOC106624487, LOC106622467) and Aedes albopictus (Diptera: Culicidae) where 11 ath genes have been identified (LOC109417656, LOC109417657, LOC109417661, LOC109417662, LOC109417664, LOC109622150, LOC109622151, LOC109622152, LOC109622154, LOC109622125, and LOC109622155). En exception to these previous statements is Apis florea (Hymenoptera: Apididae), where an ath-like gene LOC105737051 with high similarity to Lactobacillus sp. (i.e., L. acetotolerans AP014808.1 and L. gasseri CP000413.1) has been identified.
Trehalase Phylogenetic Tree
Phylogenetic analysis evidenced that at least one member of the treh-1 and treh-2 subfamily was present in each species considered, except for Diptera that didn’t possess any treh-1 gene (Figure 1 and Supplementary Figure S1).
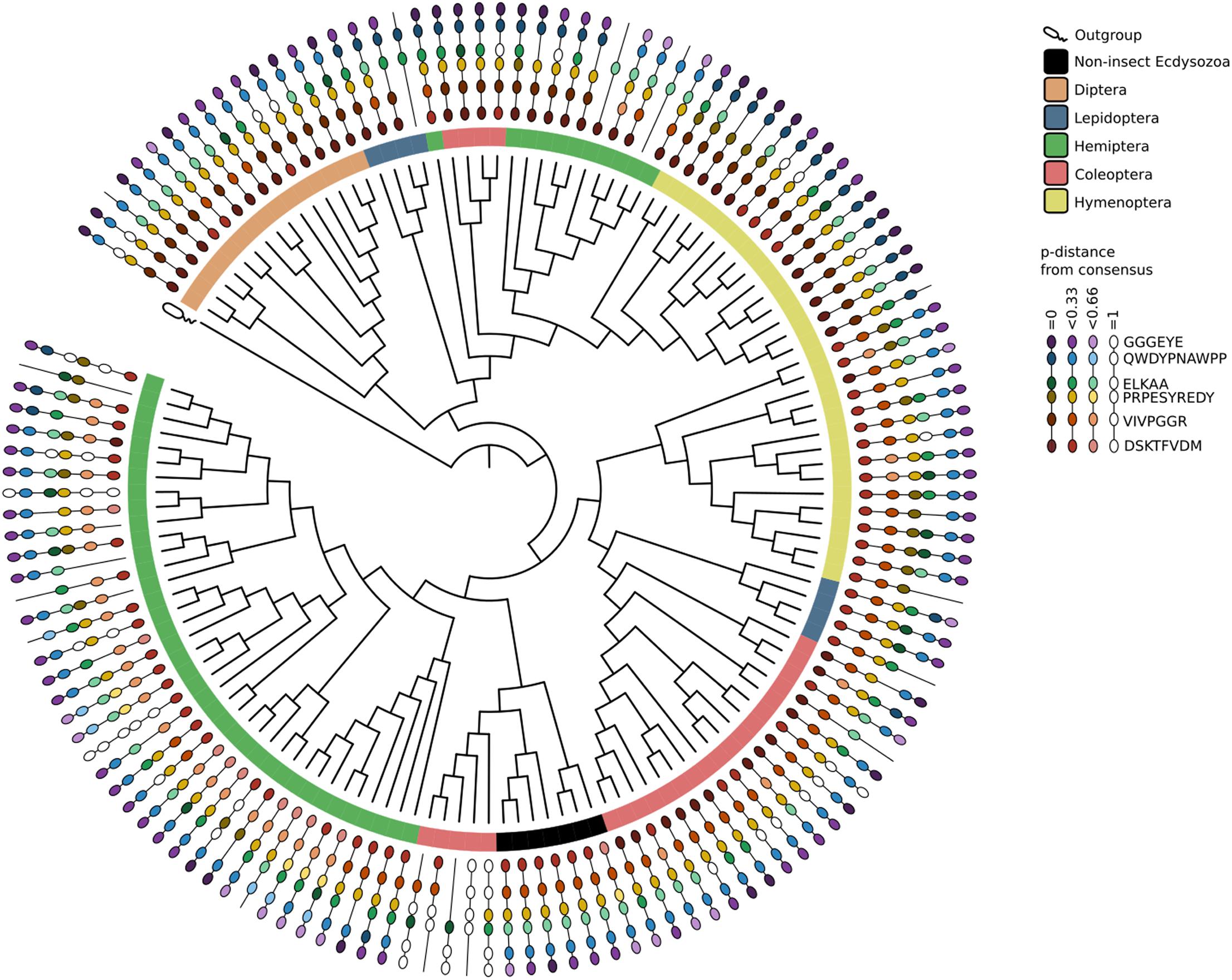
Figure 1. Phylogenetic tree of treh genes and protein isoform diversity in insects and other taxa. For each paralog only one transcript variant has been considered while protein diversity represents all the transcript variants predicted by NCBI algorithms.
Treh-1 subfamily is represented by a higher number of members (110/160 treh-1 genes/n° of treh-1 and treh-2 genes) which encode for lesser conserved trehalase isoforms, in respect to treh-2 subfamily. Coleopteran and hemipteran treh genes, for instance, had species-specific duplication events involving treh-1. Conversely, Hymenoptera and Lepidoptera didn’t show such significant differences between treh-1 and treh-2. A. tumida represented an interesting exception possessing unique treh paralogs that results more similar to trehalase genes of the outgroup species than to insects ones.
Gene Synteny and Co-linearity
The comparison of the genomic regions containing the treh and ath genes evidenced high levels of synteny and co-linearity among species belonging to the same insect order, but not among them (Figure 7).
The highest level of genomic synteny was found in Hymenoptera with 3 treh neighboring genes shared by 15 species on 30 genomic scaffolds (Figure 2), Coleoptera (Figure 3), and Lepidoptera (Figure 3). Hemipterans, on the opposite, show very low synteny with no treh neighboring genes shared by all species studied (Figure 4). However, within the super-family Aphidoidea, 13 shared treh neighboring genes have been found between A. pisum and D. noxia, considering 44 genomic scaffolds and 54 treh genes (Figure 4).
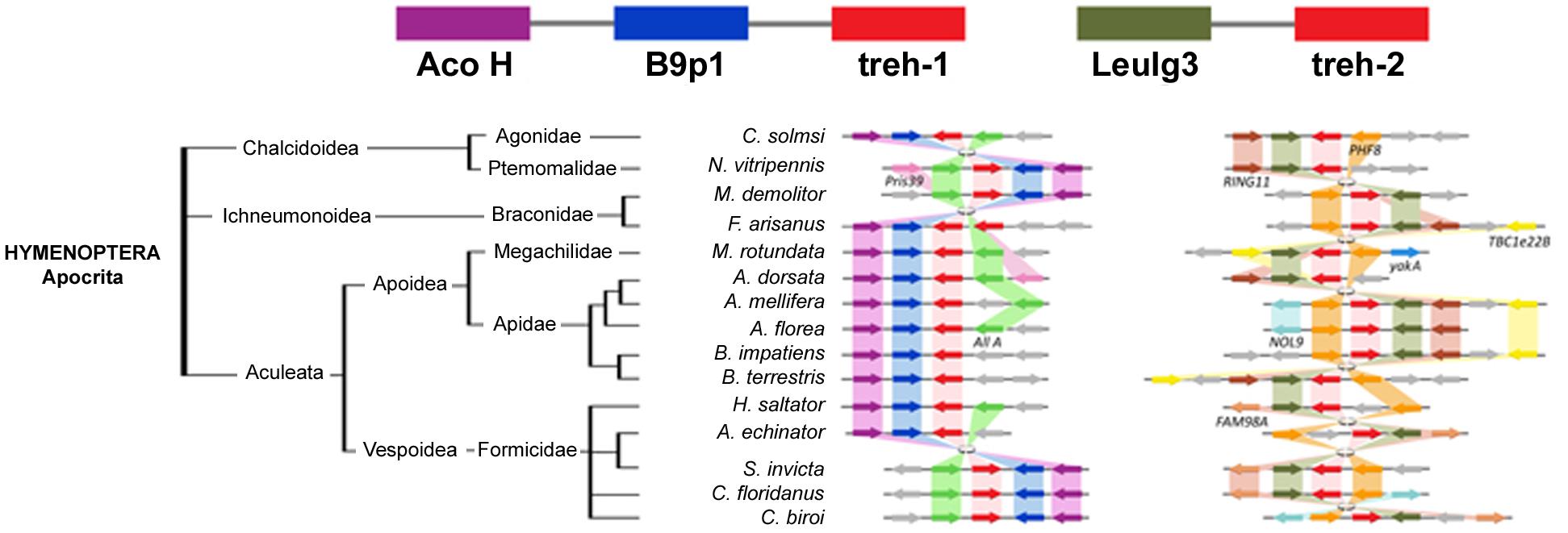
Figure 2. Gene synteny and co-linearity of trehalase genes in Diptera. Boxes represent syntenic genes. Arrows show reading frame of each gene. nrnp1, heterogeneous nuclear ribonucleoprotein L; ZnCCHCp24, Zinc finger CCHC domain containing protein 24-like; uncharact, uncharacterized protein; U4/U6.U5, U4/U6.U5 tri-snRNP associated protein 1; LTV1, LTV1 ribosome biogenesis factor; Raba, Rabaptin GTPase binding effector protein 1; C3/PZPa2p8, C3 and PZP like alpha 2 macroglobulin domain containing protein 8; dystrob. B, dystrobrevin beta; PpAsx, Polycomb protein Asx; cof/act, cofilin/actin de-polymerizing factor homoloug; Chi-9, chitinase 9; RIP3, ras interacting protein RIP3. Phylogenetic tree modified from tol (www.tol.org).
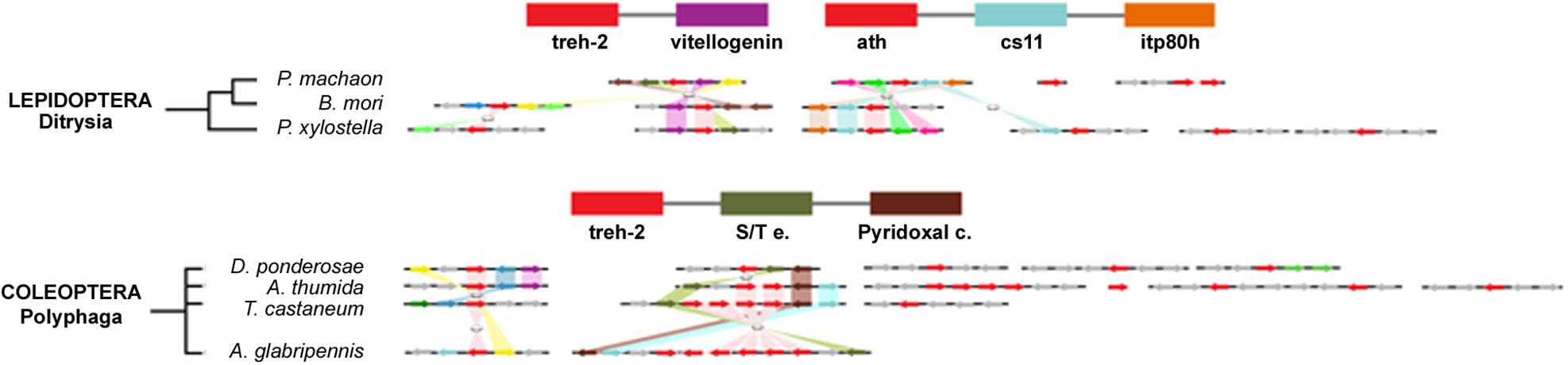
Figure 3. Gene synteny and co-linearity of the trehalase genes in Hymenoptera. Boxes represent syntenic genes. Arrows show the reading frame of each gene. Aco H, Aconitate hydratase mythocondrial like; B9p1, B9 domain containing protein 1 like; LeuIg3, Leucine-rich repeats and immunoglobulin like domains protein 3; Pris39, Prisilkin 39; All A, Allatostatin receptor like; PHF8, Histone-lysine demethylase PHF8 like; RING11, RING finger protein 11; TBC1e22B, TBC1 domain family member 22B; yakA, probable Serine/Threonine protein kinase yakA; NOL9, Polynucleotide 5′-hydroxyl kinase NOL9; FAM98A, FAM98A protein. Phylogenetic tree modified from tol (www.tol.org).

Figure 4. Gene synteny and co-linearity of the trehalase genes in Lepidoptera and Coleoptera. Boxes represent syntenic genes. Arrows show the reading frame of each gene. Lepidoptera; cs11, carbohydrate sulfotransferase 11; itp80, intraflagellar transport protein 80 homoloug; DNAHQ1, ADP dependent DNA Helicase Q1-like; B9P1, B9 domain containg P1; tf Adf1, transcription factor Adf1-like; Tret-1, facilitated trehalose transporter Tret-1 like; CatL1, Cathepsine L1-like; G3PDH, glycerol 3 phosphate dehydrogenase mitochondrial; tf Sp3, transcription factor Sp3 or 4-like. Coleoptera; S/T e., Ser/Threo extracellular; Pyridoxal c., pyridoxal carboxylase; Ser pst, Serine proteinase stubble; Rho190, Rho GTPase active protein 190; Tsk2, Trans-skeletase 2; PlexA, Plexine A; Ub c.H, Ubiquitin carboxyl hydrolase. Phylogenetic tree modified from tol (www.tol.org).
Aedes albopictus and Culex quinquefasciatus (Diptera: Cucilidae) duplicated the entire region harboring treh genes and lack of synteny with Diptera Brachicera scaffolds (Figure 5).
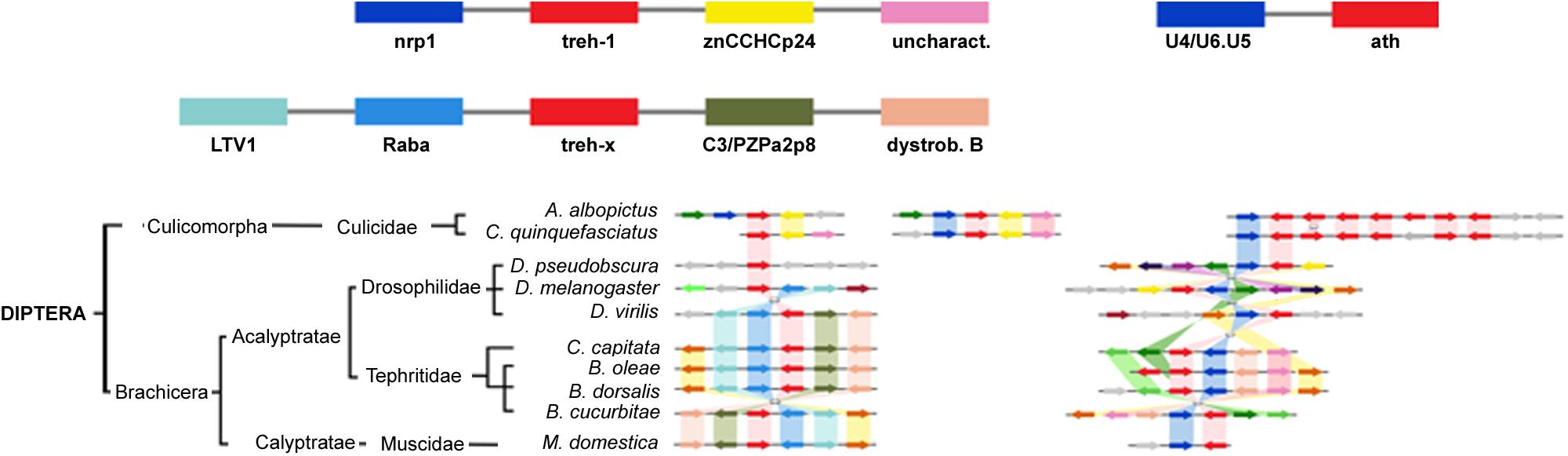
Figure 5. Gene synteny and co-linearity of the trehalase genes in Hemiptera. Boxes represent syntenic genes. Arrows show reading direction of each gene. mlip3, mucolipin 3; dyn1, dynamin 1 like protein; glyp4, glypican 4 like; nudEh1, nuclear distribution protein nudE homolog 1; UbcH43, Ubiquitin carboxyl-terminal hydrolase 43; grhead, protein grainyhead; eiger, protein eiger; unch., uncharacterized; hgf, hepatocyte growth factor-regulated tyrosine kinase substrate; Rab8, ras related protein Rab 8A; ag2, alpha-glucosidase 2; ATPsF1, ATP synthase mitochondrial F1 complex assembly factor 1; NHERF1, Na (+) / H (+) exchange regulatory cofactor NHE-RF1. Phylogenetic tree modified from tol (www.tol.org).
Paralogs With Protein Functional Specialization
To evaluate the solubility of trehalase and acid trehalase enzymes, the predicted protein sequences were tested with Signal p 4.1 to identify potential signal peptides and TMHMM Server v. 2.0 to predict transmembrane domains (Figure 6).
In order to test if treh gene duplications were accompanied by functional divergence, the number of genes, transcript variants and protein isoforms has been compared among insect species (Figure 7). Isoform diversity has been analyzed firstly considering complete protein amino acid sequences and their conservation at the whole sequence level (isoforms sensu stricto) and secondly focusing only on the conservation of the amino acidic sequence of the functional domains occurring in these proteins (isoforms sensu lato).
The number of protein isoforms varied widely and reached the highest rates in Coleoptera (2,687 isoforms sensu stricto/n° of species; 1,6875 isoforms sensu lato/n° of species) and Hemiptera (2,020 isoforms sensu stricto/n° of species; 1,405 isoforms sensu lato/n° of species). The lowest diversity can be found in Hymenoptera with 0,444 isoforms sensu stricto/n° of genes and 0,227 isoforms “sensu lato”/n° genes (Table 2). A. thumida possess high isoform diversity representing an interesting exception in Coleoptera (Table 2).
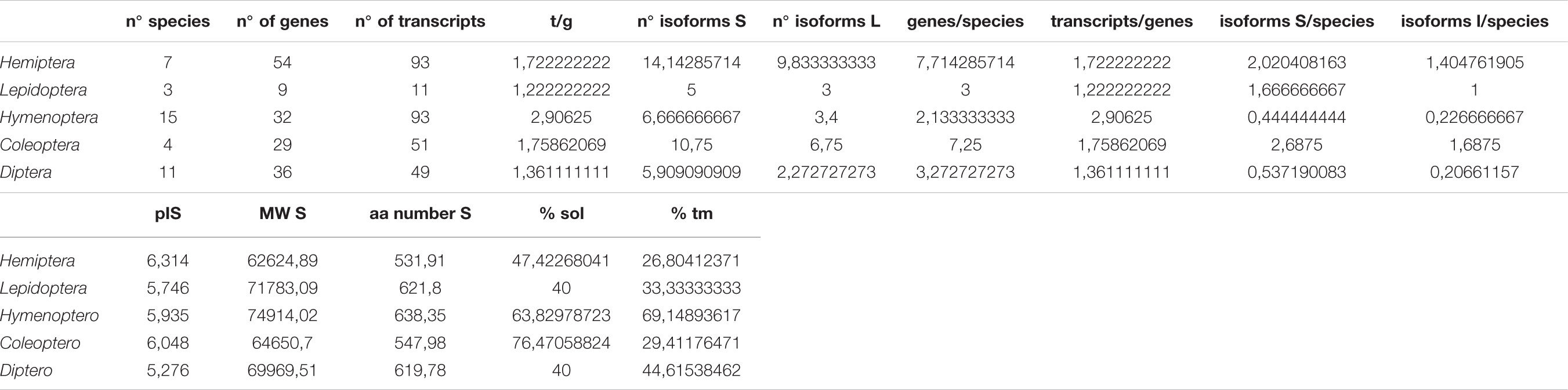
Table 2. Distribution of trehalase genes in insects, evaluating for each Order the number of genes (g), transcripts (t), isoforms, isoelectric point, molecular weight (MW), size (expressed as number of amino acids, aa), solubility and presence of transmembrane domains (tm).
Hemiptera trehalase isoforms showed a higher average isoelectric point (6,314), a lower average molecular weight (62624,89 g/mol) and amino acid number (531,91) than other insects, but no significant differences have be noticed evaluating soluble isoforms percentage (47,42% of isoforms possess a signal peptide in aphids) or the presence of transmembrane domains (26,80% of isoforms has at least one transmembrane domain) (Figures 6, 7 and Table 2).
All acid trehalases genes, on the contrary, encoded for only one isoform, apparently lacking exons and introns. Amino acidic sequences of predicted ATH isoforms were conserved only within insect orders and never possessed typical bacterial or fungal ath domains (Table 2).
Discussion
Treh Genes Duplication and Trehalase Sub-Functionalization in Insects
Trehalose is commonly present in the haemolymph of most insects and it has been suggested a role of this sugar in osmoregulation in some insects (Iturriaga et al., 2009).
The analysis of the evolutionary history of the treh/treh-like and ath/ath-like genes and their functional divergence during the insect evolution evidenced that at least two treh paralogs are present in most of studied species, except for Dipterans, that probably never duplicated the treh gene. Many insect species have experienced specific treh gene duplications and maintained the multiple treh genes as functional copies (paralogs) in their genomes (Avonce et al., 2006; Tang et al., 2018).
This is interesting since, according to literature (Zhang, 2003; Zhang et al., 1998), a loss of function generally occurs for most of the paralogs. The treh gene family in insects represents therefore an interesting exception for studying the adaptive effect of duplications. Gene duplication is a major evolutionary mechanism that can confer adaptive advantages to organisms through the occurrence of mutations in paralogs resulting in new genetic variants (Duda and Palumbi, 1999; Lynch and Conery, 2003; Conant and Wolfe, 2008; Warren et al., 2014). Indeed, paralogous genes may have a decreased purifying selective pressure resulting in the fixation of mutations. In some rare cases, no deleterious mutations occur so that paralogs are maintained functionally active and may undergo processes of sub-functionalization (with paralogous and orthologous genes cooperating to the same function) or a neo-functionalization, based on the gaining of new functions of paralogous genes in respect to orthologous ones (Hughes, 1994; Zhang et al., 1998; Force et al., 1999; Kellogg, 2003; Zhang, 2003; Innan and Kondrashov, 2010).
The presence of low sequence conservation at C and N-termini of the amino acidic sequences of trehalase isoforms in Hemiptera and Coleoptera, in comparison to other insects, suggests that a functional divergence occurred in treh family during the evolution of these taxa. Interestingly, most of the Coleopteran treh gene duplications involved the treh-1 gene only and paralogs are clustered in the same scaffold. The presence of multiple copies of the treh genes in Coleoptera could be explained as an adaptation to a trehalose rich diet in insectivorous, detritivorous, and mycophagous species since this sugar is present at high concentration in fungi (Thevelein, 1984). A. thumida, on the contrary, represents an exception since, despite its ecological adaptation as a beehive parasite, it possesses a higher number of treh copies encoding for a high number of trehalase isoforms. In P. xylostella, the finding of trehalase similar to E. cloacae treh probably derives from a bacterial DNA contamination of genomic database considering that E. cloacae is widely adopted in agriculture as a bio-control agent against pathogens (Duponnois et al., 1999; Watanabe et al., 2001).
Trehalase genes have been duplicated more frequently in Hemipterans than in other insects, but numerous rearrangements (including inversion and conspicuous genomic insertions or deletions) seem to have occurred in their genomes so that the multiple treh genes were not clustered in the same scaffold. This result is not surprising considering the holocentric nature of their chromosomes that can confer the ability to retain chromosomal rearrangements, such as intrachromosomal translocations and/or chromosomal fission/fusions (Manicardi et al., 2015). Furthermore, hemipteran trehalases have the longest glycine-rich domain and the higher rate of fixed mutations. Both these aspects seem to be functionally relevant since the additional amino acids enriched in the glycine rich regions are likely to influence the interactions of these regions with other proteins or RNA and may facilitate homo- and hetero-meric interactions (Wang et al., 1997; Gsponer et al., 2008). The high diversification of treh gene family in Hemipterans is particularly interesting, since it suggests that the presence of multiple copies of these enzymes is not the result of an adaptation to a sugar-rich diet (that should favor the presence of multiple copies of highly similar genes), but could be due to the occurrence of different roles of trehalase in these species. Indeed, according to literature data, defective or inhibited trehalases may be associated in insects to altered sugar metabolism (Wegener et al., 2003) or to morphological abnormalities (Zhang et al., 2012) suggesting that treh gene duplication could result in a sub-functionalization of trehalases in Hemiptera.
The role of acid trehalases in insects is still unknown and horizontal gene transfer events from bacteria or fungi to insects could be involved. Horizontal gene transfer has been indeed already suggested, for instance, to explain the presence of carotenoids genes in aphids (Moran and Jarvik, 2010; Mandrioli et al., 2016) and the occurrence of seven highly expressed trehalase genes with strong similarity for bacterial trehalases in the rotifer Adineta vaga (Hespeels et al., 2015). However, insect acid trehalases were not phylogenetically related to bacterial and fungal ath and they don’t possess the functional domains typically observed in the ATH proteins so that a different origin of these genes should be evaluated. Differently, the presence of bacterial ath-like genes in A. florea seems to result from a bacterial DNA contamination of genomic database since Lactobacillus sp. is reported as a typical component of the A. florea microbiota (Saraithong et al., 2014).
Trehalase Diversification in Response to Plant Inhibitors: Salivary Proteins as Key Molecules in the Co-evolution of Aphids and Their Host Plants
Trehalase enzymes play important roles in the insect metabolism so that they are related to the insect survival (Iturriaga et al., 2009). For this reason, in phloem sap sucking insects, trehalase enzymes represent molecules that host plants can target to establish efficient defensive strategies. For instance, plants produce trehalose as a signal molecule in response to aphid infestation (Smith and Boyko, 2007; Louis et al., 2012) and the presence of trehalase in aphid saliva may be relevant to modulate the trehalose-based defensive plant pathways (Cooper et al., 2010, 2011; Cui et al., 2012; Vandermoten et al., 2013; Chaudhary et al., 2015). In particular, trehalases in aphid saliva could act as PAD4 suppressor blocking the local accumulation of trehalose in the wounded plant tissue (Singh and Shah, 2012; Bansal et al., 2013).
At the same time, however, plants evolved in their turn trehalase inhibitors (Tatun et al., 2014) in a true arms race against phytophagous insects resulting in a strong selective pressure on the treh gene family that resulted in the maintenance of duplicated treh copies and in their divergence in order to allow aphids and other sap sucking insects to escape the plant defensive strategies.
The presence of co-evolution between plant trehalase inhibition and duplication/fixation of mutations in the treh genes could be particularly relevant in aphids in view of their peculiar reproductive mode. Indeed, the reproduction of aphids is mainly based (with the exception of a unique generation in autumn) on apomictic parthenogenesis consisting in several thelytokous parthenogenetic generations, in which unfertilized eggs develop into females (Nardelli et al., 2017). In the absence of an amphygonic reproduction, aphids cannot have any recombination between female and male genomes during spring and summer causing a reduced gene flow that will delay the spread of advantageous alleles. In this case, this means that treh alleles with favorable mutations couldn’t be spread in aphid populations during the parthenogenetic phase of their life cycle. Interestingly, the occurrence of multiple copies of the treh genes within the aphid genomes could allow the presence of multiple alleles in the same genome making gene duplication and mutations a sort of alternative pathway (in respect to genome recombination) to favor the presence of advantageous alleles.
Aphids seems to be particularly unusual in term of presence of duplications since they possess four times the gene duplications observed on average in other arthropods (The International Aphid Genomics Consortium, 2010; Mathers et al., 2017), a feature that is in common, together with the reproduction based on parthenogenesis, with the water flea Daphnia pulex (Crustacea: Cladocera). In aphids and D. pulex most of identified duplications are clade-specific and it has been suggested that duplicates were involved in rapid adaptation to environment (Pennisi, 2009; Simon et al., 2011). From this view, treh gene duplications in aphid could be an effective tool in a molecular adaptive strategy evolved to adapt to host plants, in absence of sexual reproduction and allelic recombination. The treh gene family has been indeed involved in many aphid-specific duplication events and treh paralogous genes (possessing different mutations) could be retained to face the evolution of plant trehalase inhibitors in a sort of aphid-host plant arm race (Shcherbakov and Wegierek, 1991; Hong et al., 2009; Szwedo and Nel, 2011; Liu et al., 2014).
In view of the relevant role that treh genes could play in aphids, the understanding of the biochemical nature and physiological function of trehalases could be therefore useful not only from an evolutionary point of view, but also at an applicative level, since a better understanding of trehalase could be crucial to develop new insectcides (based on trehalase inhibitors) or plant cultivars more resistant to aphids and/or to other sap sucking agricultural pest insects.
Author Contributions
All the authors contributed to the data analysis and interpretation, drafting and revising the manuscript, and approved the final version of the manuscript. The original study design was made by AN and discussed with the other authors.
Conflict of Interest Statement
The authors declare that the research was conducted in the absence of any commercial or financial relationships that could be construed as a potential conflict of interest.
Supplementary Material
The Supplementary Material for this article can be found online at: https://www.frontiersin.org/articles/10.3389/fphys.2019.00062/full#supplementary-material
FIGURE S1 | Phylogenetic distribution of the identified treh genes.
TABLE S1 | Complete list of the identified treh genes and protein main features.
Footnotes
References
Adhav, A. S., Kokane, S. R., and Joshi, R. S. (2018). Functional characterization of Helicoverpa armigera trehalase and investigation of physiological effects caused due its inhibition by Validamycin a formulation. Int. J. Biol. Macromol. 112, 638–647. doi: 10.1016/j.ijbiomac.2018.01.221
Avonce, N., Mendoza-Vargas, A., Morett, E., and Iturriaga, G. (2006). Insights on the evolution of trehalose biosynthesis. BMC Evol. Biol. 6:109. doi: 10.1186/1471-2148-6-109
Bansal, R., Mian, M., Mittapalli, O., and Michel, A. (2013). Molecular characterization and expression analysis of soluble trehalase gene in Aphis glycines, a migratory pest of soybean. Bull. Entomol. Res. 103, 286–295. doi: 10.1017/S0007485312000697
Barraza, A., and Sánchez, F. (2013). Trehalases: a neglected carbon metabolism regulator. Plant Signal. Behav. 8:e24778. doi: 10.4161/psb.24778
Becker, A., Schlöder, P., Steele, J. E., and Wegener, G. (1996). The regulation of trehalose metabolism in insects. Experientia 52, 433–439. doi: 10.1007/BF01919312
Candy, D. J. (1974). The control of muscle trehalase activity during locust flight. Biochem. Soc. Trans. 2, 1107–1109. doi: 10.1042/bst0021107
Chaudhary, R., Atamian, H., Shen, Z., Briggs, S., and Kaloshian, I. (2015). Potato aphid salivary proteome: enhanced salivation using resorcinol and identification of aphid phosphoproteins. J. Proteome Res. 14, 1762–1778. doi: 10.1021/pr501128k
Chen, J., Tang, B., Chen, H., Yao, Q., Huang, X., Chen, J., et al. (2010). Different functions of the insect soluble and membrane-bound trehalase genes in chitin biosynthesis revealed by RNA interference. PLoS One 5:e10133. doi: 10.1371/journal.pone.0010133
Conant, G. C., and Wolfe, K. H. (2008). Turning a hobby into a job: how duplicated genes find new functions. Nat. Rev. Genet. 9, 938–950. doi: 10.1038/nrg2482
Cooper, W., Dillwith, J., and Puterka, G. (2010). Salivary proteins of Russian wheat aphid (Hemiptera: Aphididae). Environ. Entomol. 39, 223–231. doi: 10.1603/EN09079
Cooper, W., Dillwith, J., and Puterka, G. (2011). Comparisons of salivary proteins from five aphid (Hemiptera: Aphididae) species. Environ. Entomol. 40, 151–156. doi: 10.1603/EN10153
Cui, F., Michael Smith, C., Reese, J., Edwards, O., and Reeck, G. (2012). Polymorphisms in salivary-gland transcripts of Russian wheat aphid biotypes 1 and 2. Insect Sci. 19, 429–440. doi: 10.1111/j.1744-7917.2011.01487.x
D’Enfert, C., and Fontaine, T. (1997). Molecular characterization of the Aspergillus nidulans treA gene encoding an acid trehalase required for growth on trehalose. Mol. Microbiol. 24, 203–216. doi: 10.1046/j.1365-2958.1997.3131693.x
Destruelle, M., Holzer, H., and Klionsky, D. (1995). Isolation and characterization of a novel yeast gene, ATH1, that is required for vacuolar acid trehalase activity. Yeast 11, 1015–1025. doi: 10.1002/yea.320111103
Duda, T. F., and Palumbi, S. R. (1999). Molecular genetics of ecological diversification: duplication and rapid evolution of toxin genes of the venomous gastropod Conus. Proc. Natl. Acad. Sci. U.S.A. 96, 6820–6823. doi: 10.1073/pnas.96.12.6820
Duponnois, R., Mateille, T., and Ba, M. A. (1999). Beneficial effects of Enterobacter cloacae and Pseudomonas mendocina for biocontrol of Meloidogyne incognita with the endospore-forming bacterium Pasteuria penetrans. Nematology 1, 95–101. doi: 10.1163/156854199507901
Duve, H. (1972). Purification and properties of trehalase isolated from the blowfly Calliphora erythrocephala. Insect Biochem. 2, 444–450. doi: 10.1016/0020-1790(72)90024-8
Force, A., Lynch, M., Pickett, F. B., Amores, A., Yan, Y. L., and Postlethwait, J. (1999). Preservation of duplicate genes by complementary, degenerative mutations. Genetics 151, 1531–1545.
Gsponer, J., Futschik, M. E., Teichmann, S. A., and Babu, M. M. (2008). Tight regulation of unstructured proteins: from transcript synthesis to protein degradation. Science 322, 1365-1368. doi: 10.1126/science.1163581
Gu, J., Shao, Y., Zhang, C., Liu, Z., and Zhang, Y. (2009). Characterization of putative soluble and membrane-bound trehalases in a hemipteran insect. Nilaparvata lugens. J. Insect Physiol. 55, 997–1002. doi: 10.1016/j.jinsphys.2009.07.003
Hespeels, B., Li, X., Flot, J., Pigneur, L., Malaisse, J., Da Silva, C., et al. (2015). Against all odds: trehalose-6-phosphate synthase and trehalase genes in the bdelloid rotifer Adineta vaga were acquired by horizontal gene transfer and are upregulated during desiccation. PLoS One 10:e0131313. doi: 10.1371/journal.pone.0131313
Hong, Y., Zhang, Z., Guo, X., and Heie, O. (2009). A new species representing the oldest aphid (Hemiptera, Aphidomorpha) from the Middle Triassic of China. J. Paleontol. 83, 826–831. doi: 10.1666/07-135.1
Huang, J., Furusawa, T., Sadakane, K., and Sugimura, Y. (2006). Purification and properties of two types of soluble trehalases from embryonic larvae of the silkworm, Bombyx mori. J. Insect Biotechnol. Sericol. 75, 1–8.
Hughes, A. L. (1994). The evolution of functionally novel proteins after gene duplication. Proc. R. Soc. Lond. Ser. B Biol. Sci. 256, 119–124. doi: 10.1098/rspb.1994.0058
Inagaki, K., Ueno, N., Tamura, T., and Tanaka, H. (2001). Purification and characterization of an acid trehalase from Acidobacterium capsulatum. J. Biosci. Bioeng. 91, 141–146. doi: 10.1016/S1389-1723(01)80056-6
Innan, H., and Kondrashov, F. (2010). The evolution of gene duplications: classifying and distinguishing between models. Nat. Rev. Genet. 11, 97–108. doi: 10.1038/nrg2689
Iturriaga, G., Suárez, R., and Nova-Franco, B. (2009). Trehalose metabolism: from osmoprotection to signaling. Int. J. Mol. Sci. 10, 3793–3810. doi: 10.3390/ijms10093793
Jorge, J. A., Polizeli, M. L., Thevelein, J. M., and Terenzi, H. F. (1997). Trehalases and trehalose hydrolysis in fungi. FEMS Microbiol. Lett. 154, 165–171. doi: 10.1111/j.1574-6968.1997.tb12639.x
Kalf, G. F., and Rieder, S. V. (1958). The purification and properties of trehalase. J. Biol. Chem. 230, 691–698.
Kellogg, E. (2003). What happens to genes in duplicated genomes. Proc. Natl. Acad. Sci. U.S.A. 100, 4369–4371. doi: 10.1073/pnas.0831050100
Letunic, I., and Bork, P. (2006). Interactive tree of life (iTOL): an online tool for phylogenetic tree display and annotation. Bioinformatics 23, 127–128. doi: 10.1093/bioinformatics/btl529
Liu, Q., Chen, J., Huang, X., Jiang, L., and Qiao, G. (2014). Ancient association with Fagaceae in the aphid tribe Greenideini (Hemiptera: Aphididae: Greenideinae). Syst. Entomol. 40, 230–241. doi: 10.1111/syen.12100
Louis, J., Singh, V., and Shah, J. (2012). Arabidopsis thaliana-aphid interaction. Arabidopsis Book 10:e0159. doi: 10.1199/tab.0159
Lynch, M., and Conery, J. S. (2003). The evolutionary demography of duplicate genes. J. Struct. Funct. Genom. 3, 35–44. doi: 10.1023/A:1022696612931
Mandrioli, M., Rivi, V., Nardelli, A., and Manicardi, G. (2016). Genomic and cytogenetic localization of the carotenoid genes in the aphid genome. Cytogenet. Genome Res. 149, 207–217. doi: 10.1159/000448669
Manicardi, G., Mandrioli, M., and Blackman, R. (2015). The cytogenetic architecture of the aphid genome. Biol. Rev. 90, 112–125. doi: 10.1111/brv.12096
Mathers, T. C., Chen, Y., Kaithakottil, G., Legeai, F., Mugford, S. T., Baa-Puyoulet, P., et al. (2017). Rapid transcriptional plasticity of duplicated gene clusters enables a clonally reproducing aphid to colonise diverse plant species. Genome Biol. 18:27. doi: 10.1186/s13059-016-1145-3
Moran, N., and Jarvik, T. (2010). Lateral transfer of genes from fungi underlies carotenoid production in aphids. Science 328, 624–627. doi: 10.1126/science.1187113
Murata, M., Nagai, M., Takao, M., Suzuki, A., Sakai, T., and Terashita, T. (2001). Purification and characterization of an extracellular acid trehalase from Lentinula edodes. Mycoscience 42, 479–482. doi: 10.1007/BF02464344
Nardelli, A., Peona, V., Toschi, A., Mandrioli, M., and Manicardi, G. (2017). Afit: a bioinformatic tool for measuring aphid fitness and invasiveness. Bull. Entomol. Res. 107, 458–465. doi: 10.1017/S0007485316001061
Nicholson, S., Nickerson, M., Dean, M., Song, Y., Hoyt, P., Rhee, H., et al. (2015). The genome of Diuraphis noxia, a global aphid pest of small grains. BMC Genomics 16:429. doi: 10.1186/s12864-015-1525-1
Pennisi, E. (2009). Water flea boasts whopper gene count. Science 324, 1252–1252. doi: 10.1126/science.324_1252b
Petersen, T., Brunak, S., von Heijne, G., and Nielsen, H. (2011). SignalP 4.0: discriminating signal peptides from transmembrane regions. Nat. Methods 8, 785–786. doi: 10.1038/nmeth.1701
Ramsey, J., Wilson, A., de Vos, M., Sun, Q., Tamborindeguy, C., Winfield, A., et al. (2007). Genomic resources for Myzus persicae: EST sequencing, SNP identification, and microarray design. BMC Genomics 8:423. doi: 10.1186/1471-2164-8-423
Santos, R., Alves-Bezerra, M., Rosas-Oliveira, R., Majerowicz, D., Meyer-Fernandes, J. R., and Gondim, K. C. (2012). Gene identification and enzymatic properties of a membrane-bound trehalase from the ovary of Rhodnius prolixus. Arch. Insect Biochem. Physiol. 81, 199–213. doi: 10.1002/arch.21043
Saraithong, P., Li, Y., Saenphet, K., Chen, Z., and Chantawannakul, P. (2014). Bacterial community structure in Apis florea larvae analyzed by denaturing gradient gel electrophoresis and 16S rRNA gene sequencing. Insect Sci. 22, 606–618. doi: 10.1111/1744-7917.12155
Shcherbakov, D., and Wegierek, P. (1991). Creaphididae, a new and the oldest aphid family from the Triassic of Middle Asia. Psyche 98, 81–85. doi: 10.1155/1991/87106
Shukla, E., Thorat, L., Nath, B., and Gaikwad, S. (2014). Insect trehalase: physiological significance and potential applications. Glycobiology 25, 357–367. doi: 10.1093/glycob/cwu125
Silvestro, D., and Michalak, I. (2011). RaxmlGUI: a graphical front-end for RAxML. Org. Divers. Evol. 12, 335–337. doi: 10.1007/s13127-011-0056-0
Simon, J., Pfrender, M., Tollrian, R., Tagu, D., and Colbourne, J. (2011). Genomics of environmentally induced phenotypes in two extremely plastic arthropods. J. Hered. 102, 512–525. doi: 10.1093/jhered/esr020
Singh, V., and Shah, J. (2012). Tomato responds to green peach aphid infestation with the activation of trehalose metabolism and starch accumulation. Plant Signal. Behav. 7, 605–607. doi: 10.4161/psb.20066
Smith, C. M., and Boyko, E. V. (2007). The molecular bases of plant resistance and defense responses to aphid feeding: current status. Entomol. Exp. Appl. 122, 1–16. doi: 10.1111/j.1570-7458.2006.00503.x
Sonnhammer, E. L., von Heijne, G., and Krogh, A. (1998). A hidden markov model for predicting transmembrane helices in protein sequences. Proc. Int. Conf. Intell. Syst. Mol. Biol. 6, 175–182.
Szwedo, J., and Nel, A. (2011). The oldest aphid insect from the Middle Triassic of the Vosges, France. Acta Palaeontol. Pol. 56, 757–766. doi: 10.4202/app.2010.0034
Tamura, K., Dudley, J., Nei, M., and Kumar, S. (2007). MEGA4: molecular evolutionary genetics analysis (MEGA) software version 4.0. Mol. Biol. Evol. 24, 1596–1599. doi: 10.1093/molbev/msm092
Tang, B., Chen, X., Liu, Y., Tian, H., Liu, J., Hu, J., et al. (2008). Characterization and expression patterns of a membrane-bound trehalase from Spodoptera exigua. BMC Mol. Biol. 9:51. doi: 10.1186/1471-2199-9-51
Tang, B., Wang, S., Wang, S., Wang, H., Zhang, J., and Cui, S. (2018). Invertebrate trehalose-6-phosphate synthase gene: genetic architecture, biochemistry, physiological function, and potential applications. Front. Physiol. 9:30. doi: 10.3389/fphys.2018.00030
Tang, B., Wei, P., Chen, J., Wang, S. G., and Zhang, W. Q. (2012). Progress in gene features and functions of insect trehalases. Acta Entomol. Sin. 55, 1315–1321.
Tatun, N., Vajarasathira, B., Tungjitwitayakul, J., and Sakurai, S. (2014). Inhibitory effects of plant latex on trehalase activity and trehalase gene expression in the red flour beetle, Tribolium castaneum (Coleoptera: Tenebrionidae). Eur. J. Entomol. 111, 11–18. doi: 10.14411/eje.2014.002
Terra, W. R., Ferreira, C., and de Bianchi, A. G. (1978). Physical properties and inhibition of an insect trehalase and a thermodynamic approach to the nature of its active site. Biochim. Biophys. Acta 524, 131–141. doi: 10.1016/0005-2744(78)90111-0
The International Aphid Genomics Consortium. (2010). Genome sequence of the pea aphid Acyrthosiphon pisum. PLoS Biol. 8:e1000313. doi: 10.1371/journal.pbio.1000313
Vandermoten, S., Harmel, N., Mazzucchelli, G., De Pauw, E., Haubruge, E., and Francis, F. (2013). Comparative analyses of salivary proteins from three aphid species. Insect Mol. Biol. 23, 67–77. doi: 10.1111/imb.12061
Wang, J., Dong, Z., and Bell, L. R. (1997). Sex-lethal interactions with protein and RNA. Roles of glycine-rich and RNA binding domains. J. Biol. Chem. 272, 22227-22235. doi: 10.1074/jbc.272.35.22227
Warren, I. A., Ciborowski, K. L., Casadei, E., Hazlerigg, D. G., Martin, S., Jordan, W. C., et al. (2014). Extensive local gene duplication and functional divergence among paralogs in Atlantic salmon. Genome Biol. Evol. 6, 1790–1805. doi: 10.1093/gbe/evu131
Watanabe, K., Abe, K., and Sato, M. (2001). Biological control of an insect pest by gut-colonizing Enterobacter cloacae transformed with ice nucleation gene. J. Appl. Microbiol. 88, 90–97. doi: 10.1046/j.1365-2672.2000.00904.x
Wegener, G., Tschiedel, V., Schlöder, P., and Ando, O. (2003). The toxic and lethal effects of the trehalase inhibitor trehazolin in locusts are caused by hypoglycaemia. J. Exp. Biol. 206, 1233–1240. doi: 10.1242/jeb.00217
Wernersson, R. (2006). Virtual ribosome–a comprehensive DNA translation tool with support for integration of sequence feature annotation. Nucleic Acids Res. 34, W385–W388. doi: 10.1093/nar/gkl252
Xie, Y. F., Yang, W. J., Dou, W., and Wang, J. J. (2013). Characterization of the cDNA encoding membrane-bound trehalase, its expression and enzyme activity in Bactrocera dorsalis (Diptera: Tephritidae). Fla. Entomol. 96, 1233–1242. doi: 10.1653/024.096.0401
Yin, C., Shen, G., Guo, D., Wang, S., Ma, X., Xiao, H., et al. (2015). InsectBase: a resource for insect genomes and transcriptomes. Nucleic Acids Res. 44, D801–D807. doi: 10.1093/nar/gkv1204
You, M., Yue, Z., He, W., Yang, X., Yang, G., Xie, M., et al. (2013). A heterozygous moth genome provides insights into herbivory and detoxification. Nat. Genet. 45, 220–225. doi: 10.1038/ng.2524
Zhang, J. (2003). Evolution by gene duplication: an update. Trends Ecol. Evol. 18, 292–298. doi: 10.1016/S0169-5347(03)00033-8
Zhang, J., Rosenberg, H. F., and Nei, M. (1998). Positive Darwinian selection after gene duplication in primate ribonuclease genes. Proc. Natl. Acad. Sci. U.S.A. 95, 3708–3713. doi: 10.1073/pnas.95.7.3708
Keywords: trehalase, acid trehalase, gene family evolution, duplicated gene functionalization, insects, phytophagous
Citation: Nardelli A, Vecchi M, Mandrioli M and Manicardi GC (2019) The Evolutionary History and Functional Divergence of Trehalase (treh) Genes in Insects. Front. Physiol. 10:62. doi: 10.3389/fphys.2019.00062
Received: 17 May 2018; Accepted: 21 January 2019;
Published: 15 February 2019.
Edited by:
Arash Zibaee, University of Guilan, IranReviewed by:
Marcela Barbosa Figueiredo, Swansea University, United KingdomEmanuele Mazzoni, Università Cattolica del Sacro Cuore, Italy
Copyright © 2019 Nardelli, Vecchi, Mandrioli and Manicardi. This is an open-access article distributed under the terms of the Creative Commons Attribution License (CC BY). The use, distribution or reproduction in other forums is permitted, provided the original author(s) and the copyright owner(s) are credited and that the original publication in this journal is cited, in accordance with accepted academic practice. No use, distribution or reproduction is permitted which does not comply with these terms.
*Correspondence: Mauro Mandrioli, bWF1cm8ubWFuZHJpb2xpQHVuaW1vcmUuaXQ=