- 1Instituto de Investigaciones Biomédicas “Alberto Sols” (CSIC-UAM)/CIBER-CV, Madrid, Spain
- 2INSERM UMR-S 1180, University of Paris-Sud, University of Paris-Saclay, Châtenay-Malabry, France
- 3Cardiorenal Translational Laboratory, Institute of Research i+12, Hospital Universitario 12 de Octubre/CIBER-CV, Madrid, Spain
Cardiovascular complications are the primary death cause in type 2 diabetes, where inflammation can play a role. We, and others, have previously shown that, in diabetic cardiomyopathy, cardiac dysfunction is associated with Ca2+ mishandling. It is possible that diabetic cardiomyopathy differently affects men and women, as the latter present higher risk to develop heart failure and a higher plasmatic level of the pro-inflammatory cytokine, tumor necrosis factor alpha (TNFα), than men. However, the gender-dependent regulation of Ca2+ signaling in diabetes and its relationship with TNFα signaling are still unclear. Here, we analyzed TNFα signaling pathway and its role in Ca2+ signaling dysfunction in male and female rodent models of type 2 diabetes linked to obesity (db/db mice) using confocal microscopy in freshly isolated cardiomyocytes. TNFα increased [Ca2+]i transient amplitude and accelerated its decay without affecting SR Ca2+ load or Ca2+ spark frequency in cells from control mice. All TNFα effects on Ca2+ handling were prevented by the inhibition of the ceramidase and the phospholipase A2 (PLA2). While the plasmatic level of TNFα was similar in male and female db/db mice, only male db/db hearts over-expressed both TNFα converting enzyme (TACE) and the protective TNFα receptors 2 (TNF-R2). TNFα receptor 1 (TNF-R1) expression, involved in negative inotropic response of TNFα, was unchanged in both male and female db/db mice compared to controls. We found that male db/db mice cardiomyocytes presented a decrease in [Ca2+]i transient amplitude associated to a drop of sarcoplasmic reticulum Ca2+ load, not seen in female db/db mice. Interestingly, sustained incubation with TNFα did not restored Ca2+ signaling alteration observed in male db/db mice but still induces an increase in Ca2+ spark frequency as seen in control littermates. In cardiomyocytes from female db/db mice, TNFα had no visible effects on Ca2+ handling. In conclusion, our study shows that the alteration of Ca2+ signaling and TNFα, seen in db/db mice, is gender specific presenting an increase in TNFα cardio-protective pathway in male mice.
Introduction
Cardiovascular complications, such as coronary artery diseases, hypertension, and heart failure, are a leading cause of death in type 2 diabetes (Laakso, 1999; Bauters et al., 2003; Bell, 2007). Preclinical studies have shown that diabetic cardiac dysfunction, with depressed contraction and relaxation, results from dysregulation of metabolism, mitochondrial function, oxidative stress, and Ca2+ handling (Bugger and Abel, 2014). These knowledge result almost exclusively from male animal studies. However, in the clinical setting, the risk for developing cardiac diseases in diabetes is known to be gender specific (Galderisi et al., 1991; Rutter et al., 2003; Toedebusch et al., 2018). Indeed, the Framingham Heart Study showed that diabetic women present a 5.1-fold increased risk to develop heart failure than non-diabetic patients, whereas in diabetic men, this risk is only multiplied by 2.4 (Galderisi et al., 1991; Rutter et al., 2003). In addition, the hospital admission rate for cardiovascular diseases is higher in diabetic women compared to diabetic men. Yet, the gender differences in the alterations of cardiac cellular function in diabetes are unclear, notably regarding Ca2+ mishandling.
Ca2+ regulates contraction through the excitation-contraction coupling in cardiomyocytes. For each heartbeat, sarcolemmal L type Ca2+ channels open during the action potential, leading to Ca2+ influx that activates Ca2+ release from the ryanodine receptors (RyR) located at the sarcoplasmic reticulum (SR). This release of Ca2+ by the RyR (visualized as a [Ca2+]i transient) activates contractile myofibrils to generate cardiomyocyte contraction. After the contraction, the Ca2+ is re-uptaken into the SR by the SERCA pump and extruded outside the cardiomyocytes mainly by the Na+/Ca2+ exchanger, resulting in cardiomyocyte relaxation. We and others have shown that, in animal models of type 2 diabetes linked to obesity, contractile dysfunction is associated with a decrease in the Ca2+ transient amplitude. This lower Ca2+ transient amplitude is associated to reduced L-type Ca2+ current density combined with downregulation of RyR expression (Belke et al., 2004; Pereira et al., 2006b, 2014). We found that these alterations may be different in male and female db/db mice (Pereira et al., 2014); however, the mechanisms remain unclear.
Clinical and preclinical studies pointed out an increase in plasmatic level of TNFα, in type 2 diabetes, notably in women (Yamakawa et al., 1995; Pereira et al., 2006a; Preciado-Puga et al., 2014). TNFα is an inflammatory cytokine commonly associated to infectious and non-infectious cardiomyopathy, such as viral myocarditis, congestive heart failure, and myocardial infarction. The level of TNFα seems correlated to the development of cardiac dysfunction (Feldman et al., 2000; Blum and Miller, 2001), and its over-expression leads to cardiac hypertrophy, fibrosis, arrhythmia, and dysfunction (Kubota et al., 1997; Kadokami et al., 2000; London et al., 2003). Yet, whether TNFα is a cause or a consequence of cardiac dysfunction is still under debate. The biological response of TNFα is mediated through two receptors, the TNFα receptor 1 (TNF-R1) and TNFα receptor 2 (TNF-R2). TNF-R1 activation is responsible for a cardiac negative inotropic response, whereas TNF-R2 mediates cardiac positive inotropic response (Meldrum, 1998). At the cellular level, TNFα regulates contraction either by direct regulation of Ca2+ signaling in acute condition or via iNOS activation in sustained conditions (Fernandez-Velasco et al., 2007). Still, whether TNFα activation positively or negatively alters the Ca2+ transient is quite controversial, and studies found either a decrease, an increase, or no effect on Ca2+ transient. Those discrepancies seem to depend on the animal model, the concentration of TNFα used, and the incubation time (Yokoyama et al., 1993; Goldhaber et al., 1996; Bick et al., 1997; Sugishita et al., 1999; Li et al., 2003; Zhang et al., 2005; Duncan et al., 2010; Greensmith and Nirmalan, 2013). In addition, whether the regulation of TNFα signaling in type 2 diabetic cardiomyopathy linked to obesity is gender specific remains unknown.
Considering all these controversial findings surrounding TNFα regulation of Ca2+ handling, we first studied the effect of TNFα on Ca2+ signaling in WT mice. Then, using the db/db mice, an animal model of type 2 diabetes with insulin resistance linked to obesity, we found that both Ca2+ and TNFα signaling underwent distinct alterations in male compared to female. Here, we found that male db/db mice presented a depressed Ca2+ transient associated with a lower SR Ca2+ load, not seen in female db/db mice. More interestingly, in male db/db, cardiomyocytes seem to put in place a protective mechanism to counteract those alterations by increasing the expression of cardio-protective TNF-R2 signaling pathway.
Materials and Methods
Cell Isolation
Experiments were carried out according to the ethical principles of the French Ministry of Agriculture and the European Parliament on the protection of animals. Ventricular adult cardiomyocytes were isolated from 8 weeks old male C56Bl6 mice, male and female 15 weeks old db/db (Janvier), and their control littermates (db/+). Mice were euthanized by intraperitoneal injection of sodium pentobarbital (100 mg/kg). Cardiac ventricular myocyte isolation was performed by standard enzymatic methods (collagenase type II, Worthington) using the Langendorff perfusion as previously described (Pereira et al., 2006b, 2007, 2012; Leroy et al., 2011; Ruiz-Hurtado et al., 2015). After isolation, cells were kept in 1 mM [Ca2+] for an hour prior experiments. Only rod-shaped cells and quiescent cells when unstimulated and excitable were used for the Ca2+ experiments.
Measurements of Plasmatic TNFα
TNFα determination by ELISA Soluble TNFα concentration was determined in plasma samples from mice using commercial ELISA test (BIOTRAK, Amersham Life Science, Sweden).
Confocal Microscopy
Ca2+ handling was recorded in freshly isolated ventricular adult cardiomyocytes loaded with the fluorescent Ca2+ dye, the Fluo-3 acetoxymethyl ester (Fluo-3 AM, Molecular Probes) at 5 μM diluted in a mixture of DMSO-pluronic acid 20%. A line scan across the longitudinal axis of the myocyte was performed to measure cardiomyocyte shortening. Cardiomyocyte shortening corresponds to the difference between cardiomyocyte length at rest and cardiomyocyte length during contraction (during electrical stimulation), as previously described (Fernandez-Velasco et al., 2009). Ca2+ transient, Ca2+ sparks, and SR Ca2+ load were recorded using confocal microscopy (Meta Zeiss LSM 510, objective w.i. 63×, n.a. 1.2) in line scan mode (1.54 ms) along the longitudinal axis of the cell. Ca2+ transients were evoked by field stimulation (1 Hz) applied through two parallel platinum electrodes. Spontaneous Ca2+ sparks were recorded in quiescent cells after Ca2+ transient recording. Ca2+ transient decay time corresponds to the kinetic of the relaxation phase due to the re-uptake of Ca2+ into the SR by the SERCA pump as well as the extrusion of Ca2+ by the Na2+/Ca2+ exchanger. Ca2+ transient decay time is calculated using a mono-exponential function to fit the Ca2+ transient decline phase. SR Ca2+ load was assessed by rapid caffeine application (10 mM) after 1 min pacing to reach the steady state. Parameters were studied with or without TNFα (1 h to 1 h 30 min) supplemented or not with a ceramidase inhibitor n-oleoylethanolamine (NOE, 5 μM) and a phospholipase A2 (PLA2) inhibitor (ATK, 10 μM) (Sigma-Aldrich). Fluo-3 AM was excited with an Argon laser (λex = 488 nm), and emission was collected at wavelengths >505 nm. Image analysis was performed using homemade routines in interactive data language (IDL).
Western-Blot Analysis
Adult ventricular homogenates were quickly frozen in liquid nitrogen and then placed in Tris solution (50 mmol/L, pH = 7.4) containing proteases and phosphatase inhibitors (10 μg/ml leupeptin, 10 μg/ml trypsin inhibitor, 2 μg/ml aprotinin, and 5 μM okadaic acid). Homogenization was performed on ice using a Politron. Homogenate was centrifuged at 18,925 g for 10 min at 4°C. Proteins were resuspended in Laemmli (5%) sample buffer, boiled (90°C for 5–10 min), and separated by sodium dodecyl sulfate polyacrylamide gel electrophoresis (SDS-PAGE) using 10% polyacrylamide gels. After separation, proteins were transferred to polyvinylidene fluoride membranes (Amersham Biosciences), and non-specific binding sites were blocked overnight at 4°C in 5% dried milk and Tris-buffer saline (TBS, pH = 7.4) and 0.01% Tween 20. Membranes were incubated overnight (at 4°C) for the rabbit polyclonal anti-TACE (1:300; Proscience) and the rabbit polyclonal anti-TNFR2 (H-202) (1:250; Santa Cruz), at room temperature for 1 h 30 min for the rabbit polyclonal anti-TNFR1 (H-271) (1:500; Santa Cruz). A secondary horseradish peroxidase-conjugated goat anti-rabbit IgG (Amersham Biosciences) was used in combination with an enhanced chemiluminescence detection system (SuperSignal West Pico Chemiluminescent Substrate, Pierce) to visualize the primary antibodies. Band densities were determined with a laser-scanning densitometer (HP-3970) and Quantity One software (BioRad SA). Protein loading was controlled by probing all Western blots with anti-GADPH antibody (1:4,000) (Ambion).
Statistical Analysis
Results were expressed as mean ± SEM. Significance between two groups was determined using unpaired Student’s t test or non-parametric Mann-Whitney test. Data involving more than two groups were analyzed using either one-way ANOVA or two-way ANOVA as appropriate. We used GraphPad Prism 7 (GraphPad) for statistical comparison. Differences with values of p < 0.05 were considered significant.
Results
Sustained TNFα Exposure Increases Ca2+-Induced Ca2+ Release
TNFα-mediated Ca2+ signaling regulation is quite controversial, which is probably due to protocol differences. Therefore, we first studied, in our experimental settings, the effect of sustained activation (1–1 h 30 min) of TNFα on Ca2+ handling parameters such as Ca2+ transient, Ca2+ spark frequency, and SR Ca2+ load (Figure 1). In our hands, 10 and 50 ng/ml TNFα treatment significantly increased Ca2+ transient amplitude (F/F0 of 3.1 ± 0.3 for 10 ng/ml, 3.5 ± 0.3 for 50 ng/ml vs. 2.5 ± 0.14 for baseline, p < 0.05). Moreover, TNFα significantly accelerated the Ca2+ re-uptake into the SR as shown by the faster SR Ca2+ transient decay time (Figures 1A,B) (~29% faster for 10 ng/ml and ~25% for 50 ng/ml, p < 0.01). This acceleration of Ca2+ re-uptake did not modified SR Ca2+ load (Figure 1D) and did not affect Ca2+ spark frequency (Figures 1E,F) at any concentration studied. However, 100 ng/ml of TNFα had no effects on either Ca2+ transient amplitude, Ca2+ spark frequency, or SR Ca2+ load. However, 100 ng/ml of TNFα still accelerated the Ca2+ transient decay (Figure 1C). These results clearly show that sustained TNFα activation mediates an increase in systolic Ca2+ release. Altogether, our results lean toward the idea of a positive inotropic effect.
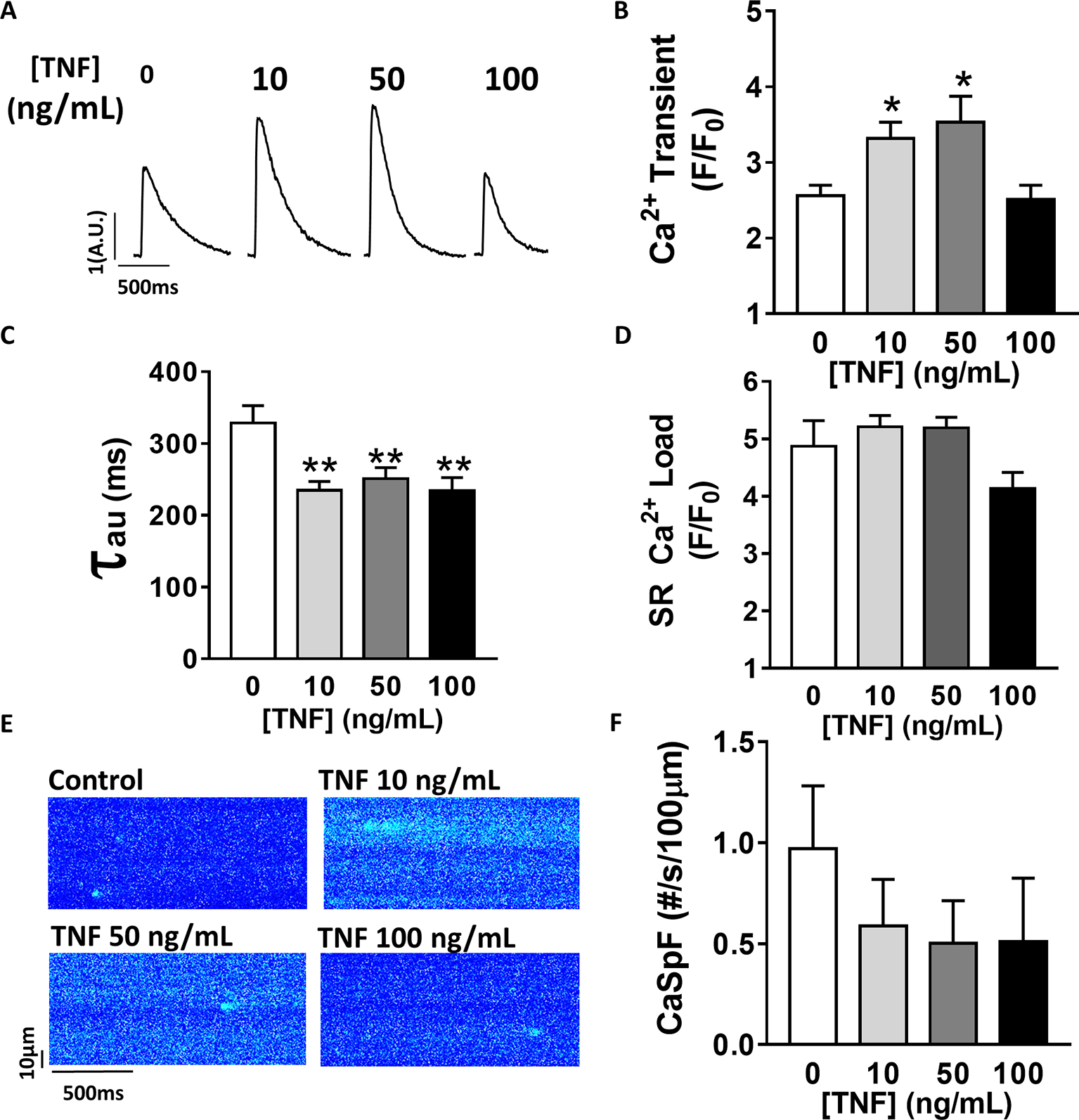
Figure 1. Positive inotropic effect of TNFα incubation. (A) Examples of Ca2+ transient recordings in freshly isolated cardiomyocytes (5 μM Fluo-3 AM) at baseline, at 10 ng/ml TNFα and 100 ng/ml TNFα. (B) Mean Ca2+ transient amplitude from cardiomyocytes at baseline (n = 22) and with incremental TNFα treatment (n = 21 for 10 ng/ml, n = 15 for 50 ng/ml, n = 10 for 100 ng/ml). (C) Ca2+ transient decay time (in ms) at baseline (n = 20) and with incremental TNFα treatments (n = 20 for 10 ng/ml, n = 15 for 50 ng/ml, n = 9 for 100 ng/ml). (D) Mean of sarcoplasmic reticulum (SR) Ca2+ load obtained by caffeine application after 1 min field stimulation in same conditions (respectively, n = 10, n = 11, n = 11, and n = 7). (E) Examples Ca2+ spark frequency (CaSpF) recording in freshly isolated cardiomyocytes at baseline, at 10 ng/ml TNFα and 100 ng/ml TNFα. (F) Mean of CaSpF (number of sparks (#) per second per 100 μm) obtained in same groups as in (A) (respectively, n = 19, n = 20, n = 15, and n = 7). *p < 0.05, **p < 0.01.
PLA2 and Ceramidase Mediate TNFα Regulation of Ca2+ Signaling
Previous work has suggested that TNFα response is mediated by the sphingosine signaling pathway (Hofmann et al., 2003). To investigate the signaling pathway involved in TNFα regulation of Ca2+ signaling, we used a ceramidase inhibitor (5 μM NOE) and a PLA2 inhibitor (10 μM ATK). NOE fully prevented the increase of Ca2+ transient amplitude (Figures 2A,B) and the faster Ca2+ transient decay time induced by 10 ng/ml of TNFα (Figure 2C). NOE had no significant effects on neither the Ca2+ spark frequency nor the SR Ca2+ load (Figures 2D–F). Similarly, the phospholipase A2 inhibitor blunted all TNFα-mediated effects on the Ca2+ transient and the Ca2+ transient decay time (Figures 2B,C). As for NOE, ATK had no effect on SR Ca2+ load (Figure 2D). However, ATK, contrarily to NOE, did significantly reduce basal Ca2+ spark frequency. Altogether, those results suggest that TNFα alters Ca2+ signaling via the activation of the ceramidase and phospholipase A2 signaling pathway.
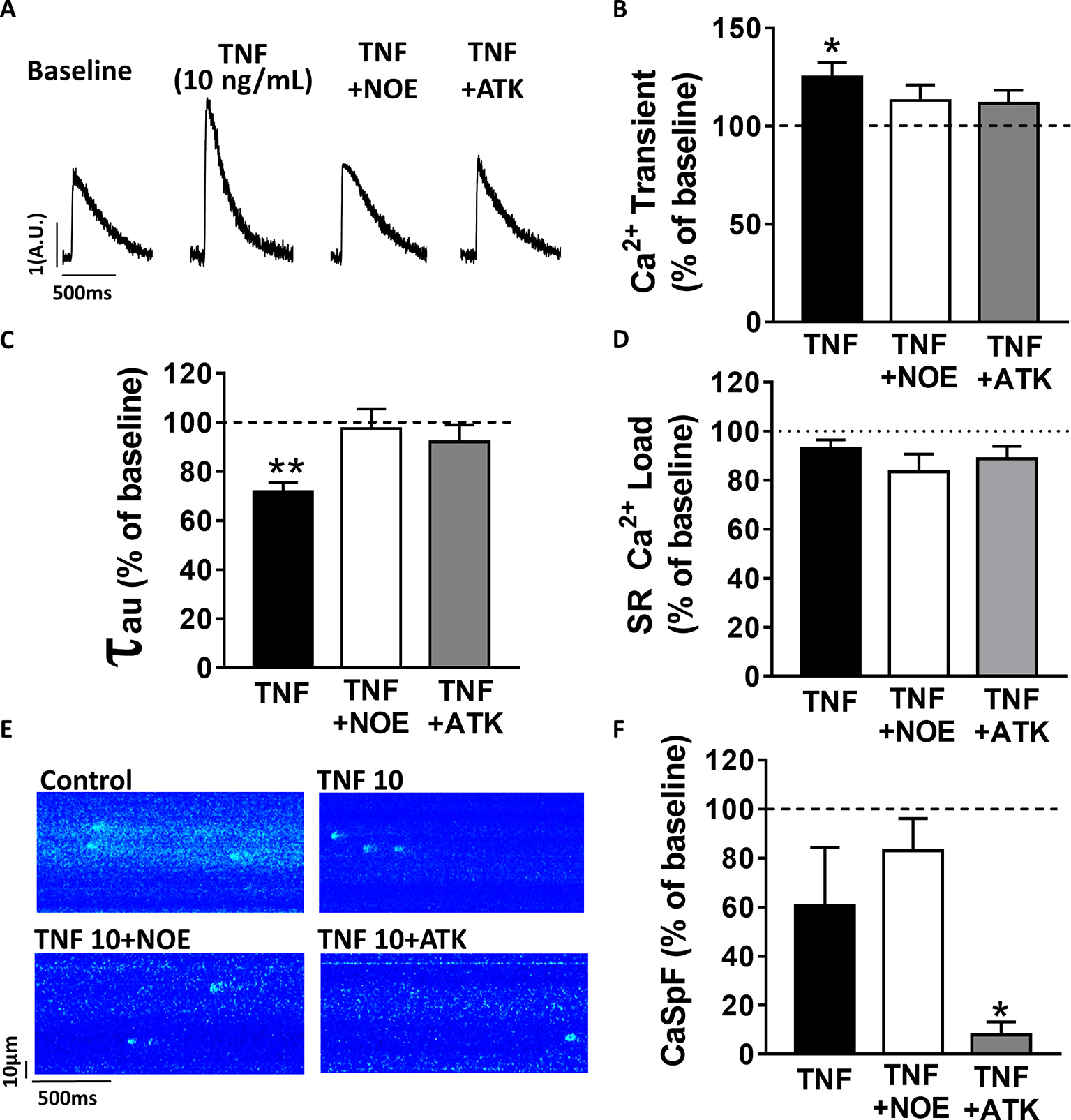
Figure 2. TNFα regulates Ca2+ signaling via the sphingosine pathway. (A) Representative Ca2+ transient examples obtained at baseline, at 10 ng/ml TNFα ± ceramide inhibitor (NOE) or phospholipase A2 inhibitor (ATK). (B) Percentage of effect on Ca2+ transient amplitude of TNFα treatment ±NOE or ATK (n = 20 for 10 ng/ml TNFα, n = 10 for TNFα+NOE, and n = 10 for TNFα+ATK). (C) Ca2+ transient decay time (in ms) in same groups (respectively, n = 19, n = 10, and n = 10). (D) Mean of SR Ca2+ load in the same groups (respectively, n = 11, n = 10, and n = 9). (E) Examples Ca2+ spark frequency recording in freshly isolated cardiomyocytes at baseline, at TNFα ± NOE or ATK. (F) Mean of CaSpF obtained in conditions (respectively, n = 20, n = 10, and n = 10). *p < 0.05, **p < 0.01.
Gender Differences in Upstream TNFα Signaling Pathway in Obesity-Linked Type 2 Diabetic Mice (db/db)
Since plasmatic TNFα level is significantly elevated in type 2 diabetic patients, we first measured the plasmatic level of TNFα in male and female db/db mice. At 15 weeks old, db/db mice develop a type 2 diabetes linked to obesity with associated cardiomyopathy (Pereira et al., 2006b). Surprisingly, neither male nor female db/db mice presented an increase in their plasmatic level of TNFα compared to control (Figure 3A). Then, we measured the expression of key proteins involved in the TNFα signaling pathway, such as type 1 and type 2 TNFα receptors and the TNFα conversion enzyme TACE in both male and female db/db mice. Interestingly, TACE expression was significantly higher in male db/db mice compared to controls, whereas no change was detectable in the female group (Figure 3B). Moreover, while TNF-R1 receptor expression was unchanged in both db/db groups (Figure 3C), TNF-R2 in the db/db male group was significantly increased (Figure 3D). These results clearly suggest that in male db/db mice hearts, the TNF-R2, known to mediate a cardio-protective pathway, is over-expressed, probably to protect the heart from diabetic-induced stress.
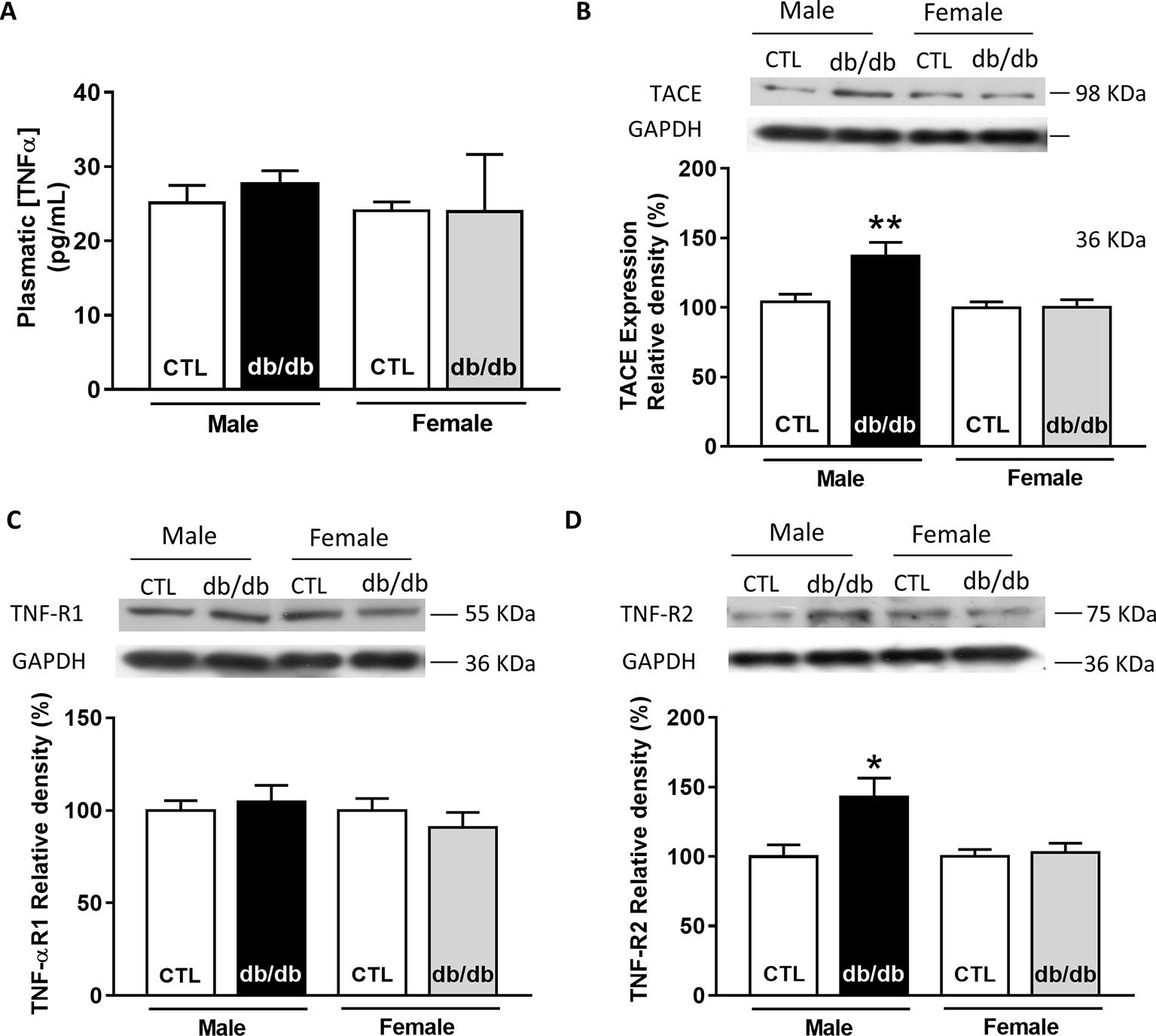
Figure 3. Gender-dependent alteration of the TNFα signaling pathway in type 2 diabetic mice (db/db). (A) Plasmatic level of TNFα obtained in male and female control and db/db heart tissue homogenates (male control: n = 5, male db/db: n = 7; female control and db/db: n = 8). (B) Representative Western-blot example of TACE (98 kDa) and the percent of relative density normalized by GAPDH signal from male and female control (n = 15, n = 17) and db/db (n = 14, n = 17) heart tissue homogenates. (C) Representative Western-blot example of TNF-R1 (55 kDa) expression and percentage of relative density normalized by GAPDH signal in male and female control (n = 16, n = 18) and db/db (n = 16, n = 18) heart tissue homogenates. (D) Representative Western-blot example of TNF-R2 (75 kDa) expression and percentage of relative density normalized by GAPDH signal in male and female control (n = 13, n = 13) and db/db (n = 13, n = 15) heart tissue homogenates. *p < 0.05.
Gender Differences in Obesity-Linked Type 2 Diabetic (db/db) Ca2+ Mishandling
In db/db mice, cardiac dysfunction has been associated with a decrease in SR Ca2+ transient amplitude and SR Ca2+ load (Belke et al., 2004; Pereira et al., 2006b, 2014). Here, we confirmed, in isolated cardiac myocytes from male db/db mice, that Ca2+ transient amplitude is significantly decreased (Figures 4A,B). This drop in Ca2+ transient amplitude (~51% lower than control, p < 0.01) is correlated with a drop in SR Ca2+ load (Figure 4D) (~51% lower than control, p < 0.01), which could explain the smaller (although not significant) cardiac cell shortening (Figure 4C). In our experimental conditions, Ca2+ spark frequency does not seem to be altered in db/db compared to control (db/+) (p = N.S.) (Figures 4E,F). In female db/db mice, the Ca2+ handling was similar in db/db compared to their control littermates (Figure 5). Indeed, all parameters such as Ca2+ transient amplitude (Figure 5A), Ca2+ spark frequency (Figure 5C), SR Ca2+ load (Figure 5D), and cell shortening (Figure 5B) were not significantly modified in freshly isolated cardiomyocytes in female db/db compared to control. In conclusion, we found a gender-specific alteration of Ca2+ handling in db/db mice, with lower SR Ca2+ release associated to a drop in SR Ca2+ load in male, not seen in female.
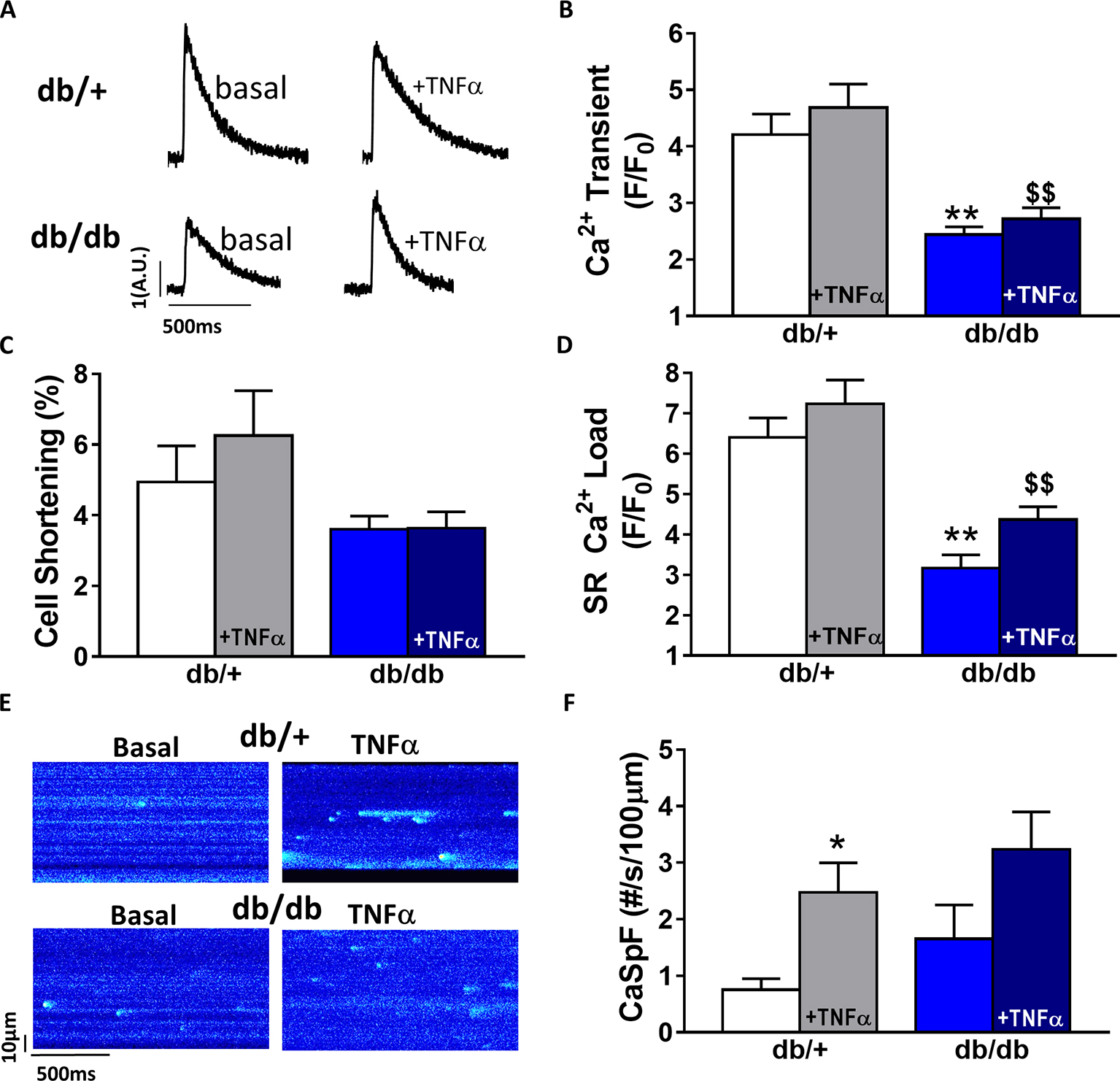
Figure 4. Ca2+ signaling is impaired in male type 2 diabetic mice. (A) Line scan images of Ca2+ transient recorded in male cardiomyocytes from db/+, db/db ± TNFα (10 ng/ml). (B) Mean of Ca2+ transient amplitude from db/db ± TNFα (n = 21 and n = 19) cardiomyocytes and their control littermates (db/+, n = 14 and n = 20). (C) Cell shortening measured in intact db/db ± TNFα (n = 13 and n = 15) and db/+ cardiomyocytes (n = 10 and n = 13) stimulated at 1 Hz. (D) Mean of SR Ca2+ load in intact db/db ± TNFα (n = 6 and n = 11) and db/+ cardiomyocytes (n = 10 and 11). (E) Examples Ca2+ spark frequency recording in freshly isolated cardiomyocytes at in db/+ and db/db with or without TNFα (F) Ca2+ spark frequency in the same groups (for db/db: n = 12 and 17, for db/+: n = 12 and n = 18) **p < 0.01 compared to db/+ and $$ p < 0.05 compared to db/db without TNFα treatment.
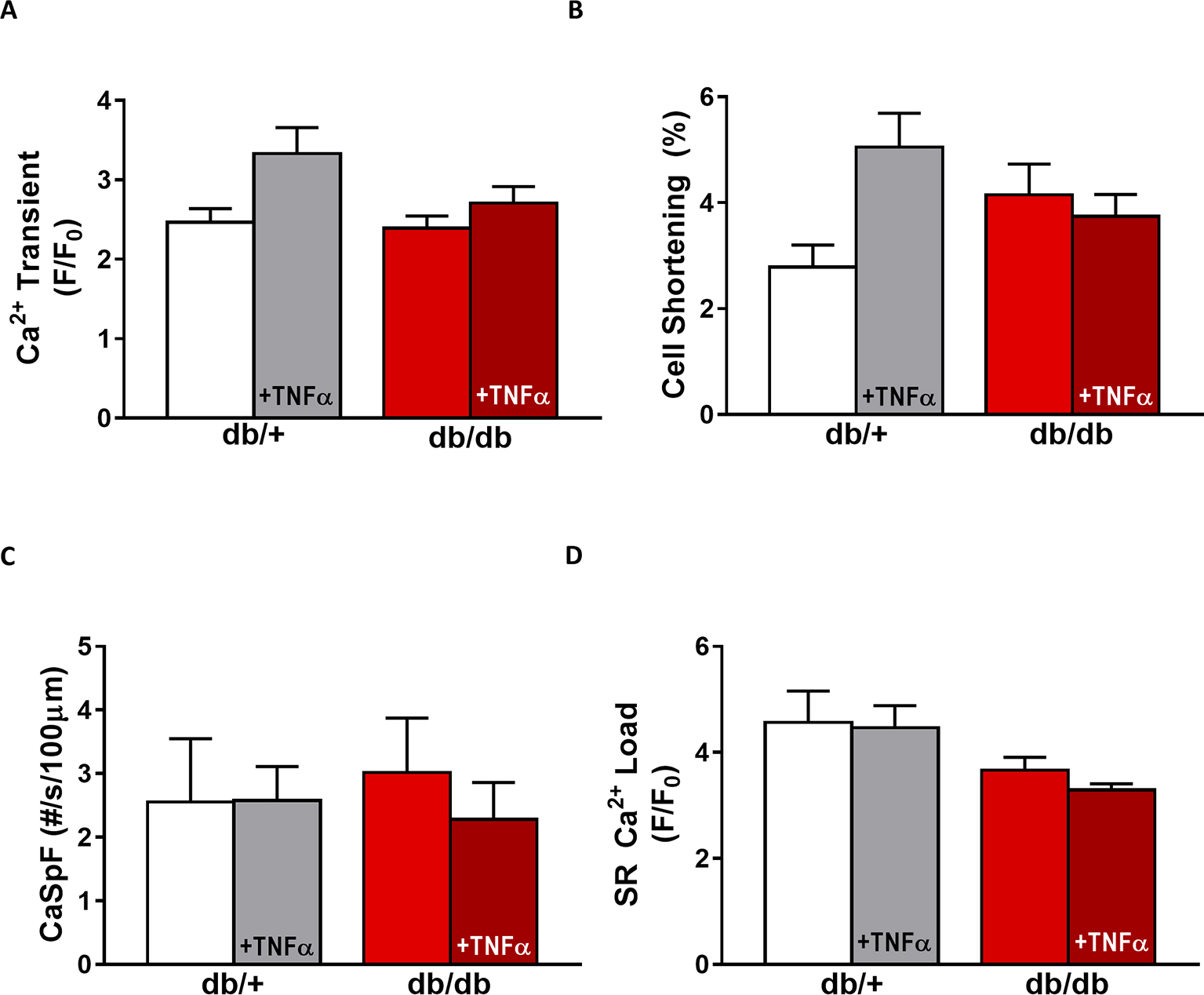
Figure 5. EC coupling is unchanged in type 2 diabetic female mice. (A) Mean Ca2+ transient amplitude from db/db ± TNFα (n = 27 and n = 23) cardiomyocytes and their control littermates (db/+, n = 11 and n = 18). (B) Cell shortening measured in intact db/db ± TNFα (n = 12 and n = 9) and db/+ cardiomyocytes (n = 8 and n = 14). (C) Ca2+ spark frequency in the same groups (for db/db: n = 9 and n = 12, for db/+: n = 10 and n = 17). (D) Mean of SR Ca2+ load in intact db/db ± TNFα (n = 17 and n = 18) and db/+ cardiomyocytes (n = 9 and n = 14). p = N.S.
Gender Differences of TNFα-Mediated Effect in Type 2 Diabetic (db/db)
Next, we compared TNFα regulation of Ca2+ signaling between male and female db/db mice. In male db/db mice, 10 ng/ml TNFα did not alter Ca2+ transient amplitude, cell shortening, nor SR Ca2+ load (Figures 4A–C,F). However, 10 ng/ml of TNFα similarly increased Ca2+ spark frequency in both control (~3.29 fold, p < 0.05) and db/db (1.5 fold, p = 0.06) (Figure 4D). In female control, the higher Ca2+ transient amplitude and cell shortening did not reach significance. Both female db/db and control had unchanged Ca2+ spark frequency. Those results suggest that, in 15 weeks old female db/db, the excitation-contraction coupling is unchanged compared to female control. Moreover, TNFα fails to show the effects found in male db/db (Figure 4D). Therefore, there are gender differences in Ca2+ mishandling and the underlying mechanisms in type 2 diabetes.
Discussion
We have previously shown that cardiac dysfunction in type 2 diabetes is associated with cardiomyocyte Ca2+ mishandling, resulting from a decrease in the Ca2+ channels involved in the Ca2+-induced Ca2+ release process (RyR and L-Type Ca2+ channels) (Belke et al., 2004; Pereira et al., 2006b). Although TNFα is elevated in diabetic patient and animal model of diabetes (Yamakawa et al., 1995; Pereira et al., 2006a; Preciado-Puga et al., 2014), little was known about its role in cellular alteration, notably regarding the Ca2+ signaling pathway and gender specificity in animal model of diabetes linked to obesity. Here, we found a gender-specific alteration of Ca2+ and TNFα signaling in db/db mice, a common model of type 2 diabetes linked to obesity. Indeed, we found that male db/db mice, not female, presented the previously described Ca2+ mishandling with lower systolic Ca2+ release and SR Ca2+ load. More interestingly, we found that male and female db/db mice expressed differently TNF-R2, with an increased expression in male db/db mice that might reflect the activation of the TNFα cardio-protective TNF-R2-dependent pathway, not seen in female db/db.
Cardiac Positive Inotropic Effect of TNFα
Discrepancies regarding the TNFα regulation of Ca2+ signaling are quite important in the literature with reported positive or negative ionotropic effect. For instance, in cat cardiomyocytes, short time exposure of TNFα reduced Ca2+ transient amplitude in response to a disruption of Ca2+ influx via L type Ca2+ channels leading to cellular shortening, supporting, then, a negative ionotropic effect of TNFα (Yokoyama et al., 1993). This negative inotropic effect of TNFα has been also described, in rabbit and guinea pigs, with TNFα-induced impaired cellular shortening cardiomyocytes mediated by NO dependent but Ca2+ independent (Goldhaber et al., 1996; Sugishita et al., 1999). However, various studies performed in rodents have shown that TNFα can lead to inotropic positive effects (Bick et al., 1997; Greensmith and Nirmalan, 2013). Here, we found that TNFα treatments (10 and 50 ng/ml) induced a time and concentration-dependent effect leading to a significant increase in Ca2+ transient amplitude between 1 h and 1 h 30 min suggesting a positive inotropic effect. Our results are in concordance with Bick et al. study (Bick et al., 1997), who have found that TNFα incubation increases Ca2+ transient and cellular contraction in neo-natal cardiomyocytes. In adult rat cardiomyocytes treated with 50 ng/ml of TNFα (Greensmith and Nirmalan, 2013), Ca2+ transient amplitude and cellular shortening were also increased (Greensmith and Nirmalan, 2013). The absence of effect observed under 100 ng/ml of TNFα might be explained by its bimodal effect, as previously described in cardiomyocytes, depending on exposure time or dose (Amadou et al., 2002; Shanmugam et al., 2016). Then, 100 ng/ml TNFα or higher doses, and with prolonged exposure, is expected to induce negative inotropic effects on Ca2+ handling.
In Mice Cardiomyocytes, TNFα Regulates Ca2+ Signaling via the Sphingosine and PLA2 Pathways
Previous studies have shown that TNFα produces myocardial effects (negative or positive inotropic effect) through different mechanisms such as PLA2 or sphingosine signaling pathway (Murray and Freeman, 1996; Oral et al., 1997; Liu and McHowat, 1998). Here, we found that exposure of TNFα (1 h to 1 h 30 min) mediates Ca2+ transient increase via the activation of both ceramidase (sphingosine precursor) and PLA2 (for arachidonic acid production). Sphingosine is commonly associated to short-term (within minutes) negative inotropic effect of TNFα (Oral et al., 1997). However, other studies have shown that ceramide enhanced SR Ca2+ release and SR Ca2+ re-uptake in adult ventricular myocytes (Liu and Kennedy, 2003). Those results are in line with our prevention of TNFα-mediated elevation of systolic Ca2+ release and Ca2+ transient decay time in cardiomyocytes treated with the ceramidase inhibitor NOE (Figures 2B,C). Moreover, inhibition of the PLA2 prevented TNFα-mediated increase in Ca2+ transient amplitude and SR Ca2+ transient decay time, suggesting that TNFα induces Ca2+ mishandling via PLA2-mediated phosphorylation of RyR. Indeed, 10 ng/ml of TNFα has been shown to increase Ca2+ transient amplitude as a result of PLA-2 mediated RyR PKA phosphorylation at serine 2,808 in wild-type mice of RASSF1A knock out (Mohamed et al., 2014). This PKA-dependent mediated effect of PLA-2/arachidonic acid on the RyR phosphorylation state perfectly explains why we observed a dramatic drop of Ca2+ spark frequency under the inhibition of the PLA-2 (Figure 2F). In addition, TNFα also accelerates SR Ca2+ re-uptake reflecting an increase in SERCA pump activity as seen under PKA phosphorylation of phospholamban supporting the TNFα/PLA-2/PKA pathway. This mechanism is confirmed by the restoration of the TNFα-mediated acceleration Ca2+ transient decay time under ATK, the PLA-2 inhibitor (Figures 2B,C).
Gender-Dependent Ca2+ Mishandling in db/db Mice, an Obesity-Linked Type 2 Diabetic Model
Type 2 diabetes is the most common form of diabetes. In western countries, 80% of type 2 diabetic patients have developed a diabetes linked to obesity resulting in severe glucose intolerance compared to lean type 2 diabetic patients (Schaffer and Mozaffari, 1996). Our study was performed in db/db mice, a model that recapitulates, in that sense, the human pathology. Indeed, the leptin receptor mutation of db/db mice impairs the satiety feeling and leads to obesity around 4–5 weeks of age, which is followed by diabetic state with hyperglycemia and insulin resistance (Coleman, 1978). In type 2 diabetes linked to obesity, cardiac dysfunction has been associated to Ca2+ mishandling and structural remodeling (Belke et al., 2004; Pereira et al., 2006b; Falcao-Pires and Leite-Moreira, 2012). Indeed, overall, animal models of type 2 diabetes present a reduced Na+/Ca2+ exchanger activity, and depressed Ca2+ transient linked to downregulation of Ca2+ channels, RyRs, and reduced SERCA activity (Netticadan et al., 2001; Zhong et al., 2001; Abe et al., 2002; Belke et al., 2004; Pereira et al., 2006b; Boudina and Abel, 2010). Here, our results show that those effects are recapitulated in male db/db mice (Figure 4), but not in female db/db mice. However, the gender-specific regulation in Ca2+ handling and/or β-adrenergic response has been previously described (Parks et al., 2014). Supporting this idea, we found that basal Ca2+ transient amplitude is lower in female control compared to male control cardiomyocytes. Although Parks et al. (2014) have shown that Ca2+ current, diastolic Ca2+, and SR Ca2+ load were similar between control male and female, basal cAMP level was lower in control female compared to control male due to higher PDE4B expression in female. These results are in line with our previous work showing that db/db female mice have reduced phosphorylation of the RyR, which reduce Ca2+ spark frequency and could explain the preserve SR Ca2+ load and Ca2+ transient seen in female db/db compared to db/db male. Our results are paradoxical compared to the higher risk to develop heart failure for type 2 diabetic women compared to diabetic men. This discrepancy could be explain as follow: the decrease in [Ca2+]i transient in male db/db mice could be protective at long term, maybe by preventing Ca2+ toxic effects such as apoptosis or preserve ATP content by limiting the ATP expense in pumping Ca2+ (Javorkova et al., 2010; Parks et al., 2014). Future studies will be needed to confirm this hypothesis.
Gender Dependent Alteration of Molecular TNFα Signaling Pathway in db/db
To our knowledge, plasmatic TNFα level parallels the degree of cardiac dysfunction in diabetic patients. In the db/db mice, we did not observe any changes in the plasmatic level of TNFα compared to control. Even though circulating TNFα is unchanged, male db/db mice present an increase in TACE expression suggesting a paracrine elevation of TNFα in the heart. Surprisingly, despite cardiomyocyte treatment with 10 ng/ml of TNFα, a concentration within the in vivo range measured under stress and injury (Bitterman et al., 1991), TNFα did not induce an increase in Ca2+ transient amplitude or decay time in db/db, as seen in C57Bl6 mice (Figures 4B,C). One explanation could be that in db/db control littermate strain background (C57BKS/J strain), TNFα is not as effective as in C57Bl6 strain. Indeed, genetic background, such as between C57BL6/J and C57BL6/N, has been shown to influence cardiac phenotype and propensity to develop cardiomyopathies (Tian et al., 2011; Simon et al., 2013). This could also explain the ineffective response of TNFα in female control and db/db mice (Figure 5). Although TNFα activation has been linked with oxidative stress, no gender-specific difference in cardiomyocytes redox state at baseline or during pathology has been observed (Ren, 2007; Bell et al., 2015). Another possibility could be that in male db/db, the dramatically reduced SR Ca2+ load would prevent the high Ca2+ systolic release induced by TNFα probably due to the phosphorylation of the RyR via the activation of PLA2. Indeed, we found in the presence of TNFα an increase in Ca2+ spark frequency in both db/+ and db/db mice reflecting an elevated diastolic RyR opening resulting from RyR phosphorylation by PKA previously described in male db/db (Pereira et al., 2014). Interestingly, in male db/db mice, the TNF-R2 was overexpressed, which is known to exert cardio-protective effects via the activation of NF-κB (Burchfield et al., 2010). Indeed, in liver, TNFα inhibits PDE3 expression elevating cAMP level and PKA activation (Ke et al., 2015). This activation of PKA could explain, in cardiomyocytes, the elevation of Ca2+ spark frequency in male db/+ cardiomyocytes treated with TNFα (Figure 4F). Moreover, TNF-R2 is known to be involved in positive cardiac inotropic effect (Defer et al., 2007). As a result, [Ca2+] overload was prevented and Ca2+ transient increased leading to an increase in inotropic response. The over-expressed TNF-R2 in a male db/db appears as an attempt to counteract the already present Ca2+ mishandling to protect from cardiac dysfunction. Indeed, prolonged activation of the TNF-R2 pathway in the db/db male cardiomyocytes could then activate phosphorylation of excitation-contraction coupling key proteins, such as phospholamban, to restore Ca2+ transient and cardiomyocytes contraction.
In conclusion, we found for the first time that both Ca2+ and TNFα signaling are altered only in male type 2 diabetic mice, whereas female does not seem to be affected. Although this study has several limitations in the interpretation such as non-comparable hormonal state between female db/db mice and diabetic women, lower effect of TNFα in db/+ than C57BL6 control, we still clearly show that male db/db mice develop Ca2+ mishandling leading to impaired contraction already at a young age, while woman seemed to be protected. Moreover, we found that male db/db mice put into place a protective mechanism to counteract those negative effects by over-expressing TNF-R2 cardio-protective signaling pathway.
Data Availability
The datasets generated for this study are available on request to the corresponding author.
Ethics Statement
The study was carried out in accordance to the ethical principles of the French Ministry of Agriculture and the European Parliament on the protection of animals. The protocol was approved by the French Ministry of Agriculture and Bioethical Committee of the CSIC following recommendation of the Spanish Animal Care and the European Parliament on the protection of animals.
Author Contributions
CD and AG conceived and designed the project, supervised the data acquisition and participated in analysis. LP and GR performed most of the experiments and analyses. LP interpreted the data and wrote the first draft of the manuscript. MS participated in the figure preparation. All authors have edited the manuscript.
Funding
This work has been funded by Acuerdos Bilaterales España-Francia (CSIC-INSERM) grant no. 2005FR0020 and partially by SAF2017-84777R from the Spanish Ministerio de Industria, Economia y Competitividad (to CD) and partially by CP15/00129 and PI17/01093 (to GR), and the ANR-11-1DEX-0003-02 to LP as members of the Laboratory of Excellence LERMIT.
Conflict of Interest Statement
The authors declare that the research was conducted in the absence of any commercial or financial relationships that could be construed as a potential conflict of interest.
Abbreviations
ATK, arachidonyl trifluoromethyl ketone; TNFα, tumor necrosis factor alpha; TNF-R1, TNFα receptor 1; TNF-R2, TNFα receptor 2; KO, knock-out; NO, nitric oxide; NOE, n-oleoylethanolamine; o.i., oil immersion; PKA, protein kinase A; PLA2, phospholipase A2; RyR, cardiac ryanodine receptor; SR, sarcoplasmic reticulum; SERCA, sarco/endoplasmic reticulum Ca2+-ATPase; TACE, TNFα converting enzyme.
References
Abe, T., Ohga, Y., Tabayashi, N., Kobayashi, S., Sakata, S., Misawa, H., et al. (2002). Left ventricular diastolic dysfunction in type 2 diabetes mellitus model rats. Am. J. Physiol. Heart Circ. Physiol. 282, H138–H148. doi: 10.1152/ajpheart.2002.282.1.H138
Amadou, A., Nawrocki, A., Best-Belpomme, M., Pavoine, C., and Pecker, F. (2002). Arachidonic acid mediates dual effect of TNF-alpha on Ca2+ transients and contraction of adult rat cardiomyocytes. Am. J. Phys. Cell Physiol. 282, C1339–C1347. doi: 10.1152/ajpcell.00471.2001
Bauters, C., Lamblin, N., Mc Fadden, E. P., Van Belle, E., Millaire, A., and de Groote, P. (2003). Influence of diabetes mellitus on heart failure risk and outcome. Cardiovasc. Diabetol. 2:1. doi: 10.1186/1475-2840-2-1
Belke, D. D., Swanson, E. A., and Dillmann, W. H. (2004). Decreased sarcoplasmic reticulum activity and contractility in diabetic db/db mouse heart. Diabetes 53, 3201–3208. doi: 10.2337/diabetes.53.12.3201
Bell, D. S. (2007). Heart failure in the diabetic patient. Cardiol. Clin. 25, 523–538; vi. doi: 10.1016/j.ccl.2007.08.003
Bell, J. R., Raaijmakers, A. J., Curl, C. L., Reichelt, M. E., Harding, T. W., Bei, A., et al. (2015). Cardiac CaMKIIdelta splice variants exhibit target signaling specificity and confer sex-selective arrhythmogenic actions in the ischemic-reperfused heart. Int. J. Cardiol. 181, 288–296. doi: 10.1016/j.ijcard.2014.11.159
Bick, R. J., Liao, J. P., King, T. W., LeMaistre, A., McMillin, J. B., and Buja, L. M. (1997). Temporal effects of cytokines on neonatal cardiac myocyte Ca2+ transients and adenylate cyclase activity. Am. J. Phys. 272, H1937–H1944. doi: 10.1152/ajpheart.1997.272.4.H1937
Bitterman, H., Kinarty, A., Lazarovich, H., and Lahat, N. (1991). Acute release of cytokines is proportional to tissue injury induced by surgical trauma and shock in rats. J. Clin. Immunol. 11, 184–192. doi: 10.1007/BF00917424
Blum, A., and Miller, H. (2001). Pathophysiological role of cytokines in congestive heart failure. Annu. Rev. Med. 52, 15–27. doi: 10.1146/annurev.med.52.1.15
Boudina, S., and Abel, E. D. (2010). Diabetic cardiomyopathy, causes and effects. Rev. Endocr. Metab. Disord. 11, 31–39. doi: 10.1007/s11154-010-9131-7
Bugger, H., and Abel, E. D. (2014). Molecular mechanisms of diabetic cardiomyopathy. Diabetologia 57, 660–671. doi: 10.1007/s00125-014-3171-6
Burchfield, J. S., Dong, J. W., Sakata, Y., Gao, F., Tzeng, H. P., Topkara, V. K., et al. (2010). The cytoprotective effects of tumor necrosis factor are conveyed through tumor necrosis factor receptor-associated factor 2 in the heart. Circ. Heart Fail. 3, 157–164. doi: 10.1161/CIRCHEARTFAILURE.109.899732
Coleman, D. L. (1978). Obese and diabetes: two mutant genes causing diabetes-obesity syndromes in mice. Diabetologia 14, 141–148. doi: 10.1007/BF00429772
Defer, N., Azroyan, A., Pecker, F., and Pavoine, C. (2007). TNFR1 and TNFR2 signaling interplay in cardiac myocytes. J. Biol. Chem. 282, 35564–35573. doi: 10.1074/jbc.M704003200
Duncan, D. J., Yang, Z., Hopkins, P. M., Steele, D. S., and Harrison, S. M. (2010). TNF-alpha and IL-1beta increase Ca2+ leak from the sarcoplasmic reticulum and susceptibility to arrhythmia in rat ventricular myocytes. Cell Calcium 47, 378–386. doi: 10.1016/j.ceca.2010.02.002
Falcao-Pires, I., and Leite-Moreira, A. F. (2012). Diabetic cardiomyopathy: understanding the molecular and cellular basis to progress in diagnosis and treatment. Heart Fail. Rev. 17, 325–344. doi: 10.1007/s10741-011-9257-z
Feldman, A. M., Combes, A., Wagner, D., Kadakomi, T., Kubota, T., Li, Y. Y., et al. (2000). The role of tumor necrosis factor in the pathophysiology of heart failure. J. Am. Coll. Cardiol. 35, 537–544.
Fernandez-Velasco, M., Rueda, A., Rizzi, N., Benitah, J. P., Colombi, B., Napolitano, C., et al. (2009). Increased Ca2+ sensitivity of the ryanodine receptor mutant RyR2R4496C underlies catecholaminergic polymorphic ventricular tachycardia. Circ. Res. 104, 201–209, 212p following 209. doi: 10.1161/CIRCRESAHA.108.177493
Fernandez-Velasco, M., Ruiz-Hurtado, G., Hurtado, O., Moro, M. A., and Delgado, C. (2007). TNF-alpha downregulates transient outward potassium current in rat ventricular myocytes through iNOS overexpression and oxidant species generation. Am. J. Physiol. Heart Circ. Physiol. 293, H238–H245. doi: 10.1152/ajpheart.01122.2006
Galderisi, M., Anderson, K. M., Wilson, P. W., and Levy, D. (1991). Echocardiographic evidence for the existence of a distinct diabetic cardiomyopathy (the Framingham Heart Study). Am. J. Cardiol. 68, 85–89. doi: 10.1016/0002-9149(91)90716-X
Goldhaber, J. I., Kim, K. H., Natterson, P. D., Lawrence, T., Yang, P., and Weiss, J. N. (1996). Effects of TNF-alpha on [Ca2+]i and contractility in isolated adult rabbit ventricular myocytes. Am. J. Phys. 271, H1449–H1455. doi: 10.1152/ajpheart.1996.271.4.H1449
Greensmith, D. J., and Nirmalan, M. (2013). The effects of tumor necrosis factor-alpha on systolic and diastolic function in rat ventricular myocytes. Phys. Rep. 1:e00093. doi: 10.1002/phy2.93
Hofmann, U., Domeier, E., Frantz, S., Laser, M., Weckler, B., Kuhlencordt, P., et al. (2003). Increased myocardial oxygen consumption by TNF-alpha is mediated by a sphingosine signaling pathway. Am. J. Physiol. Heart Circ. Physiol. 284, H2100–H2105. doi: 10.1152/ajpheart.00888.2002
Javorkova, V., Mezesova, L., Vlkovicova, J., and Vrbjar, N. (2010). Influence of sub-chronic diabetes mellitus on functional properties of renal Na(+),K(+)-ATPase in both genders of rats. Gen. Physiol. Biophys. 29, 266–274. doi: 10.4149/gpb_2010_03_266
Kadokami, T., McTiernan, C. F., Kubota, T., Frye, C. S., and Feldman, A. M. (2000). Sex-related survival differences in murine cardiomyopathy are associated with differences in TNF-receptor expression. J. Clin. Invest. 106, 589–597. doi: 10.1172/JCI9307
Ke, B., Zhao, Z., Ye, X., Gao, Z., Manganiello, V., Wu, B., et al. (2015). Inactivation of NF-kappaB p65 (RelA) in liver improves insulin sensitivity and inhibits cAMP/PKA pathway. Diabetes 64, 3355–3362. doi: 10.2337/db15-0242
Kubota, T., McTiernan, C. F., Frye, C. S., Slawson, S. E., Lemster, B. H., Koretsky, A. P., et al. (1997). Dilated cardiomyopathy in transgenic mice with cardiac-specific overexpression of tumor necrosis factor-alpha. Circ. Res. 81, 627–635. doi: 10.1161/01.RES.81.4.627
Laakso, M. (1999). Hyperglycemia and cardiovascular disease in type 2 diabetes. Diabetes 48, 937–942. doi: 10.2337/diabetes.48.5.937
Leroy, J., Richter, W., Mika, D., Castro, L. R., Abi-Gerges, A., Xie, M., et al. (2011). Phosphodiesterase 4B in the cardiac L-type Ca(2)(+) channel complex regulates Ca(2)(+) current and protects against ventricular arrhythmias in mice. J. Clin. Invest. 121, 2651–2661. doi: 10.1172/JCI44747
Li, X. Q., Zhao, M. G., Mei, Q. B., Zhang, Y. F., Guo, W., Wang, H. F., et al. (2003). Effects of tumor necrosis factor-alpha on calcium movement in rat ventricular myocytes. Acta Pharmacol. Sin. 24, 1224–1230.
Liu, S. J., and Kennedy, R. H. (2003). Positive inotropic effect of ceramide in adult ventricular myocytes: mechanisms dissociated from its reduction in Ca2+ influx. Am. J. Physiol. Heart Circ. Physiol. 285, H735–H744. doi: 10.1152/ajpheart.01098.2002
Liu, S. J., and McHowat, J. (1998). Stimulation of different phospholipase A2 isoforms by TNF-alpha and IL-1beta in adult rat ventricular myocytes. Am. J. Phys. 275, H1462–H1472.
London, B., Baker, L. C., Lee, J. S., Shusterman, V., Choi, B. R., Kubota, T., et al. (2003). Calcium-dependent arrhythmias in transgenic mice with heart failure. Am. J. Physiol. Heart Circ. Physiol. 284, H431–H441. doi: 10.1152/ajpheart.00431.2002
Mohamed, T. M., Zi, M., Prehar, S., Maqsood, A., Abou-Leisa, R., Nguyen, L., et al. (2014). The tumour suppressor Ras-association domain family protein 1A (RASSF1A) regulates TNF-alpha signalling in cardiomyocytes. Cardiovasc. Res. 103, 47–59. doi: 10.1093/cvr/cvu111
Murray, D. R., and Freeman, G. L. (1996). Tumor necrosis factor-alpha induces a biphasic effect on myocardial contractility in conscious dogs. Circ. Res. 78, 154–160. doi: 10.1161/01.RES.78.1.154
Netticadan, T., Temsah, R. M., Kent, A., Elimban, V., and Dhalla, N. S. (2001). Depressed levels of Ca2+-cycling proteins may underlie sarcoplasmic reticulum dysfunction in the diabetic heart. Diabetes 50, 2133–2138. doi: 10.2337/diabetes.50.9.2133
Oral, H., Dorn, G. W. 2nd, and Mann, D. L. (1997). Sphingosine mediates the immediate negative inotropic effects of tumor necrosis factor-alpha in the adult mammalian cardiac myocyte. J. Biol. Chem. 272, 4836–4842. doi: 10.1074/jbc.272.8.4836
Parks, R. J., Ray, G., Bienvenu, L. A., Rose, R. A., and Howlett, S. E. (2014). Sex differences in SR Ca(2+) release in murine ventricular myocytes are regulated by the cAMP/PKA pathway. J. Mol. Cell. Cardiol. 75, 162–173. doi: 10.1016/j.yjmcc.2014.07.006
Pereira, F. O., Frode, T. S., and Medeiros, Y. S. (2006a). Evaluation of tumour necrosis factor alpha, interleukin-2 soluble receptor, nitric oxide metabolites, and lipids as inflammatory markers in type 2 diabetes mellitus. Mediat. Inflamm. 2006:39062. doi: 10.1155/MI/2006/39062
Pereira, L., Matthes, J., Schuster, I., Valdivia, H. H., Herzig, S., Richard, S., et al. (2006b). Mechanisms of [Ca2+]i transient decrease in cardiomyopathy of db/db type 2 diabetic mice. Diabetes 55, 608–615. doi: 10.2337/diabetes.55.03.06.db05-1284
Pereira, L., Metrich, M., Fernandez-Velasco, M., Lucas, A., Leroy, J., Perrier, R., et al. (2007). The cAMP binding protein Epac modulates Ca2+ sparks by a Ca2+/calmodulin kinase signalling pathway in rat cardiac myocytes. J. Physiol. 583, 685–694. doi: 10.1113/jphysiol.2007.133066
Pereira, L., Ruiz-Hurtado, G., Morel, E., Laurent, A. C., Metrich, M., Dominguez-Rodriguez, A., et al. (2012). Epac enhances excitation-transcription coupling in cardiac myocytes. J. Mol. Cell. Cardiol. 52, 283–291. doi: 10.1016/j.yjmcc.2011.10.016
Pereira, L., Ruiz-Hurtado, G., Rueda, A., Mercadier, J. J., Benitah, J. P., and Gomez, A. M. (2014). Calcium signaling in diabetic cardiomyocytes. Cell Calcium 56, 372–380. doi: 10.1016/j.ceca.2014.08.004
Preciado-Puga, M. C., Malacara, J. M., Fajardo-Araujo, M. E., Wrobel, K., Wrobel, K., Kornhauser-Araujo, C., et al. (2014). Markers of the progression of complications in patients with type 2 diabetes: a one-year longitudinal study. Exp. Clin. Endocrinol. Diabetes 122, 484–490. doi: 10.1055/s-0034-1372594
Ren, J. (2007). Influence of gender on oxidative stress, lipid peroxidation, protein damage and apoptosis in hearts and brains from spontaneously hypertensive rats. Clin. Exp. Pharmacol. Physiol. 34, 432–438. doi: 10.1111/j.1440-1681.2007.04591.x
Ruiz-Hurtado, G., Li, L., Fernandez-Velasco, M., Rueda, A., Lefebvre, F., Wang, Y., et al. (2015). Reconciling depressed Ca2+ sparks occurrence with enhanced RyR2 activity in failing mice cardiomyocytes. J. Gen. Physiol. 146, 295–306. doi: 10.1085/jgp.201511366
Rutter, M. K., Parise, H., Benjamin, E. J., Levy, D., Larson, M. G., Meigs, J. B., et al. (2003). Impact of glucose intolerance and insulin resistance on cardiac structure and function: sex-related differences in the Framingham Heart Study. Circulation 107, 448–454. doi: 10.1161/01.CIR.0000045671.62860.98
Schaffer, S. W., and Mozaffari, M. (1996). Abnormal mechanical function in diabetes: relation to myocardial calcium handling. Coron. Artery Dis. 7, 109–115. doi: 10.1097/00019501-199602000-00003
Shanmugam, G., Narasimhan, M., Sakthivel, R., Kumar, R. R., Davidson, C., Palaniappan, S., et al. (2016). A biphasic effect of TNF-alpha in regulation of the Keap1/Nrf2 pathway in cardiomyocytes. Redox Biol. 9, 77–89. doi: 10.1016/j.redox.2016.06.004
Simon, M. M., Greenaway, S., White, J. K., Fuchs, H., Gailus-Durner, V., Wells, S., et al. (2013). A comparative phenotypic and genomic analysis of C57BL/6J and C57BL/6N mouse strains. Genome Biol. 14:R82. doi: 10.1186/gb-2013-14-7-r82
Sugishita, K., Kinugawa, K., Shimizu, T., Harada, K., Matsui, H., Takahashi, T., et al. (1999). Cellular basis for the acute inhibitory effects of IL-6 and TNF- alpha on excitation-contraction coupling. J. Mol. Cell. Cardiol. 31, 1457–1467. doi: 10.1006/jmcc.1999.0989
Tian, C., Shao, C. H., Moore, C. J., Kutty, S., Walseth, T., DeSouza, C., et al. (2011). Gain of function of cardiac ryanodine receptor in a rat model of type 1 diabetes. Cardiovasc. Res. 91, 300–309. doi: 10.1093/cvr/cvr076
Toedebusch, R., Belenchia, A., and Pulakat, L. (2018). Diabetic cardiomyopathy: impact of biological sex on disease development and molecular signatures. Front. Physiol. 9:453. doi: 10.3389/fphys.2018.00453
Yamakawa, T., Tanaka, S., Yamakawa, Y., Kiuchi, Y., Isoda, F., Kawamoto, S., et al. (1995). Augmented production of tumor necrosis factor-alpha in obese mice. Clin. Immunol. Immunopathol. 75, 51–56. doi: 10.1006/clin.1995.1052
Yokoyama, T., Vaca, L., Rossen, R. D., Durante, W., Hazarika, P., and Mann, D. L. (1993). Cellular basis for the negative inotropic effects of tumor necrosis factor-alpha in the adult mammalian heart. J. Clin. Invest. 92, 2303–2312. doi: 10.1172/JCI116834
Zhang, M., Xu, Y. J., Saini, H. K., Turan, B., Liu, P. P., and Dhalla, N. S. (2005). TNF-alpha as a potential mediator of cardiac dysfunction due to intracellular Ca2+-overload. Biochem. Biophys. Res. Commun. 327, 57–63. doi: 10.1016/j.bbrc.2004.11.131
Keywords: diabetic cardiomyopathy, TNFα, calcium, gender difference, db/db mice
Citation: Delgado C, Gomez A-M, Samia El Hayek M, Ruiz Hurtado G and Pereira L (2019) Gender-Dependent Alteration of Ca2+ and TNFα Signaling in db/db Mice, an Obesity-Linked Type 2 Diabetic Model. Front. Physiol. 10:40. doi: 10.3389/fphys.2019.00040
Edited by:
José Antonio Pariente, Universidad de Extremadura, SpainReviewed by:
Juan Martinez-Pinna, University of Alicante, SpainGines M. Salido, Universidad de Extremadura, Spain
Belma Turan, Ankara University, Turkey
Copyright © 2019 Delgado, Gomez, Samia El Hayek, Ruiz-Hurtado and Pereira. This is an open-access article distributed under the terms of the Creative Commons Attribution License (CC BY). The use, distribution or reproduction in other forums is permitted, provided the original author(s) and the copyright owner(s) are credited and that the original publication in this journal is cited, in accordance with accepted academic practice. No use, distribution or reproduction is permitted which does not comply with these terms.
*Correspondence: Carmen Delgado, Y2RlbGdhZG9AaWliLnVhbS5lcw==
Ana-Maria Gomez, YW5hLW1hcmlhLmdvbWV6QGluc2VybS5mcg==
†These authors have contributed equally to this work