- 1NICU, Fondazione IRCCS Ca’ Granda Ospedale Maggiore Policlinico, Milan, Italy
- 2Department of Clinical Sciences and Community Health, Università degli Studi di Milano, Milan, Italy
Oxidative stress is a frequent condition in critically ill patients, especially if exposed to extracorporeal circulation, and it is associated with worse outcomes and increased mortality. The inflammation triggered by the contact of blood with a non-endogenous surface, the use of high volumes of packed red blood cells and platelets transfusion, the risk of hyperoxia and the impairment of antioxidation systems contribute to the increase of reactive oxygen species and the imbalance of the redox system. This is responsible for the increased production of superoxide anion, hydrogen peroxide, hydroxyl radicals, and peroxynitrite resulting in increased lipid peroxidation, protein oxidation, and DNA damage. The understanding of the pathophysiologic mechanisms leading to redox imbalance would pave the way for the future development of preventive approaches. This review provides an overview of the clinical impact of the oxidative stress during neonatal extracorporeal support and concludes with a brief perspective on the current antioxidant strategies, with the aim to focus on the potential oxidative stress-mediated cell damage that has been implicated in both short and long-term outcomes.
Introduction
Extracorporeal membrane oxygenation is life support for reversible heart and/or lung failure refractory to standard treatment, and it is used as bridge-to-recovery, bridge-to-bridge, bridge-to-transplant, or bridge-to-decision (Brogan et al., 2017). This technique was developed by the CPB technology, and it is based on a partial/full bypass of the native cardiac and/or pulmonary function. Neonatal respiratory ECMO was introduced by Robert Bartlett during the 1970s for severe hypoxemic respiratory failure secondary to MAS, PPH and RDS (Bartlett et al., 1976). Despite the progress in neonatal care following the introduction of new therapies and ventilatory strategies, ECMO remains life-saving support for about 600–800 neonates each year worldwide (Thiagarajan et al., 2017). Across the world, from 1989 to 2017, about 27.238 newborns needed an ECMO procedure for respiratory failure, 7.592 for cardiac problems, 1.694 for extracorporeal cardiopulmonary resuscitation; the overall survival rate is 83, 64, and 66%, respectively (ELSO). Although the overall survival is high, there are consistent differences in the incidence rates between the diseases. Since 1979 the survival of ECMO patients with congenital diaphragmatic hernia and sepsis is stable at around 51 and 48%, respectively. In contrast, PPH, RDS, and MAS have a significantly higher survival of 73, 76, and 92%, each. Furthermore, cardiomyopathy and myocarditis have better survival (65 and 50%, respectively), compared to congenital heart disease and cardiogenic shock (44 and 47%, respectively) (ELSO). Nevertheless, the mortality of infants and children with congenital heart disease has reduced in recent decades as a result of the improvement of CPB techniques and myocardial protective strategies (Gilboa et al., 2010; Cheng et al., 2014; Erikssen et al., 2015). Nowadays, although the clinical management of ECMO patients has remarkably progressed over the years, the rate of complications (hemostatic disorders, brain damage, AKI, sepsis) still have a high impact on patients’ outcome (ELSO).
Oxidative stress plays a critical role in the genesis of tissue damage during an ECMO procedure, and newborns are particularly prone to the toxic effects of free radicals, due to the immaturity of their antioxidant systems resulting in lowered detoxification capacity (Comporti et al., 2004; Castellheim et al., 2005; Saugstad, 2005; Mishra et al., 2008; Signorini et al., 2008; Afolayan et al., 2012, 2015; Annagür et al., 2015; Asci et al., 2015; Mokra et al., 2015; Aras-López et al., 2016; Mikolka et al., 2016; Mátyás and Zaharie, 2018; Moore et al., 2018; Perrone et al., 2018). The inflammatory response following the introduction of ECC generates a high amount of ROS contemporarily reducing endogenous antioxidants (Descamps-Latscha, 2001; Goodyear-Bruch and Pierce, 2002; Karu et al., 2010). Various studies associated the exposure to extracorporeal circuits (CPB, ECMO, and CRRT) with the increased oxidative stress that may negatively impact on morbidity and mortality (McDonald et al., 2014a). Redox imbalance is mainly induced in the early stage of ECMO, especially after the CPB, while with prolonged exposure (beyond 24 h) the TAC gradually restores (Wan et al., 1997; Babior, 2000; Desborough, 2000; Clermont et al., 2002; Levy and Tanaka, 2003; Kouchoukos et al., 2012; Chen Q. et al., 2014; Zakkar et al., 2015a,b; Fudulu and Angelini, 2016; Petersen et al., 2018). The modulation of oxidative stress would seem to be of great clinical importance. Indeed, its manipulation through the use of exogenous antioxidants, cardioplegia during CPB, blood priming strategies, or miniaturization of the extracorporeal circuit could mitigate the ROS production (Fudulu and Angelini, 2016). However, data are scant, especially for neonatal ECMO, and are mainly related to pre-clinical evidence. This review focuses on the mechanisms of oxidative stress during neonatal ECMO, involved in the pathophysiology of both short and long-term disorders as MOF, hemostatic derangements up to neurodegenerative, cardiovascular and cancer disease in adults. Lastly, a brief overview of currently available antioxidant strategies is provided (Halliwell and Gutteridge, 2015).
Redox Biology and Oxidative Stress
Energy is produced by mitochondrial metabolism through glucose, amino acids and lipids combustion in the presence of O2 (Semenza, 2009; Vento, 2014). Approximately 98% of O2 is reduced to H2O by the conventional combustion process, while only 2% of remaining O2 loses its electrons with the rear O2- and ROS production (Maltepe and Saugstad, 2009; Vento, 2014).
The “old definition” of oxidative stress was referred to conditions in which the balance between ROS production and endogenous antioxidants break down resulting in tissue and cellular injury through lipid peroxidation, protein oxidation, and DNA damage (Schieber and Chandel, 2014). Currently, the “new definition” is much more complex, referring to an imbalance between oxidants and antioxidants in favor of the oxidants, that cause an arrest of redox signaling and/or molecular damage (Sies, 2018). More recently the term “reductive stress” has been introduced, but its exact definition is still unclear (Gutteridge and Halliwell, 2018). It would seem to be due to high levels of reduced GSH and NADPH, unbalancing the redox system to the opposite oxidative extreme, which has shown to play an essential role in the pathogenesis of dilated cardiomyopathy in cases of lamin-associated muscular dystrophy (Margaritelis et al., 2014; Dialynas et al., 2015; Stevens et al., 2017; Bellezza et al., 2018).
The O2-, H2O2, OH●, and ONO2- are the most common ROS, produced by aerobic metabolism (Schieber and Chandel, 2014). The intracellular O2- arises from the one-electron reduction of molecular O2 that is primarily produced by the oxidation of NADPH by NOX enzymes or by electron loss from aerobic respiration in mitochondria (Lambeth, 2004; Brand, 2010; Sahoo et al., 2016). Superoxide anion is rapidly converted by SOD into H2O2, and its storage is prevented by SODs, which are mainly located in the cytosol and mitochondria. Conversely, the increase in H2O2 triggers autophagy and cell death (Figure 1; Fridovich, 1997; Chen Y. et al., 2009).
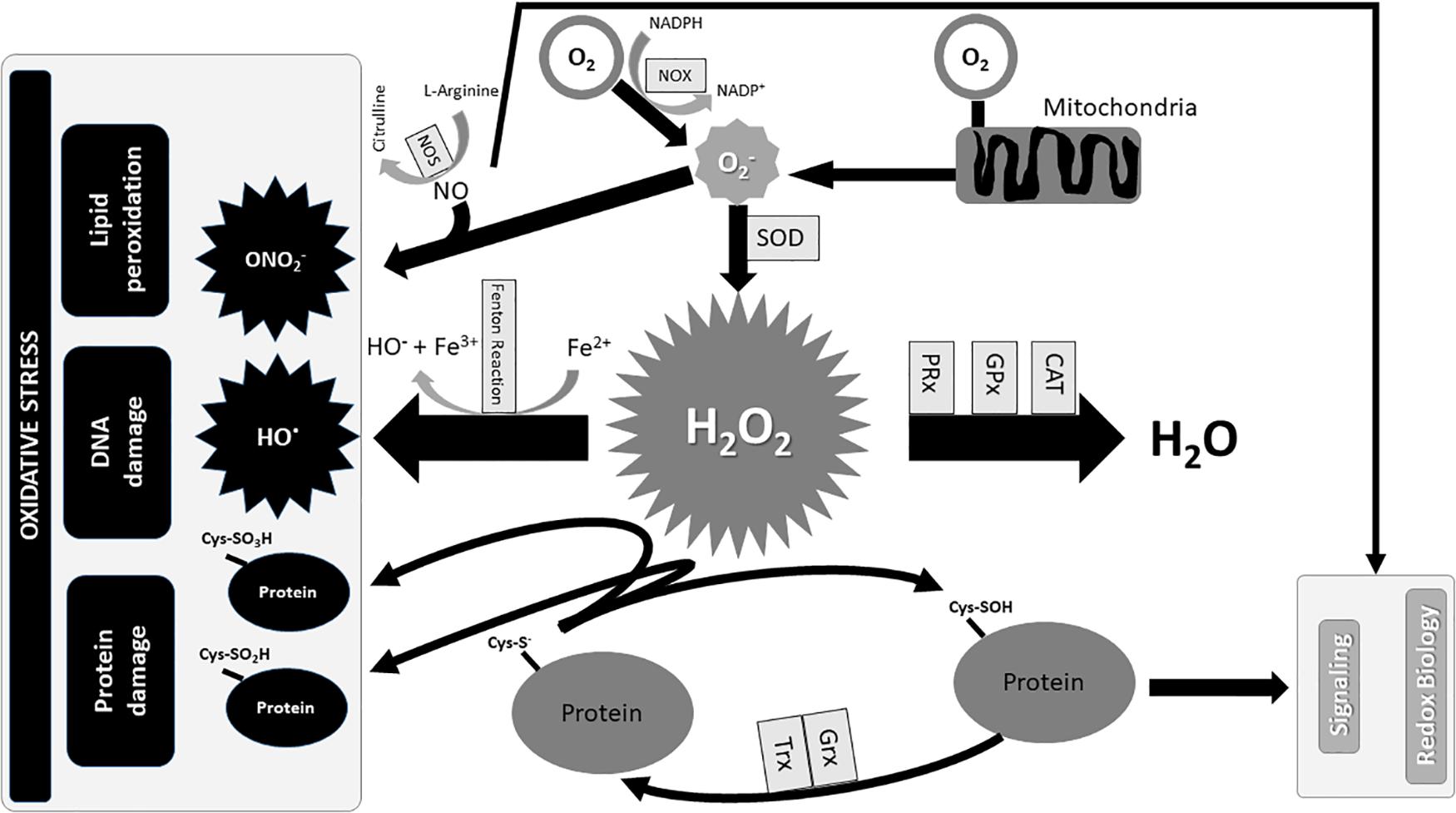
FIGURE 1. Redox Biology and Oxidative stress. The production of mainly occurs with the oxidation of NADPH into NADP+ in the presence of O2 and NOX, and partly from an electron’s loss during the combustion of carbohydrates, amino acids, and lipids for the production of energy in mitochondria. Superoxide dismutases (SOD) immediately transforms into H2O2 which is converted in OH● in the presence of iron, if not degraded by antioxidant enzymes in H2O. Furthermore, the excess of reacting with NO produces ON, which in turn participates in oxidative damage with OH●. Contrarily, the standard production of O2- contributes to the homeostasis of the redox signal. CAT, catalase; Cys–S-, cysteine thiolate anion; Cys-SO2H, cysteine sulfinic acid; Cys-SO3H, cysteine sulfonic acid; Cys–SOH, cysteine sulfenic acid; Fe2+, ferrous ions; Fe3+, ferric ions; GPx, glutathione peroxidases; Grx, glutaredoxin; H2O2, hydrogen peroxide; NADP+, nicotinamide adenine dinucleotide phosphate oxidated form; NADPH, nicotinamide adenine dinucleotide phosphate reduced form; NO, Nitric oxide; NOS, nitric oxide synthase; NOX, NADPH oxidase; O2, oxygen; O2-, superoxide anion; OH, hydroxyl radicals; ONO2-, peroxynitrite; PRx, peroxiredoxins; SOD, superoxide dismutases; Trx, thioredoxin.
Cysteine residues within proteins are oxidized by H2O2 to sulfenic form (Cys–SOH) and reduced to its basic thiolate anion (Cys–S-) form by the disulfide reductases Trx and Grx (Winterbourn and Hampton, 2008). The excess of H2O2 further oxidizes thiolate anions to sulfinic (Cys-SO2H) or sulfonic (Cys-SO3H) classes. The sulfinic and sulfonic oxidation is irreversible and could lead to permanent protein damage that is prevented by PRx, GPx and CAT (Figures 1, 2; Winterbourn and Hampton, 2008; Finkel, 2012). In contrast, the OH produced by Fenton reaction in the presence of Fe2+ has a detrimental effect on lipids, proteins, and DNA (Figure 1; Dizdaroglu and Jaruga, 2012).
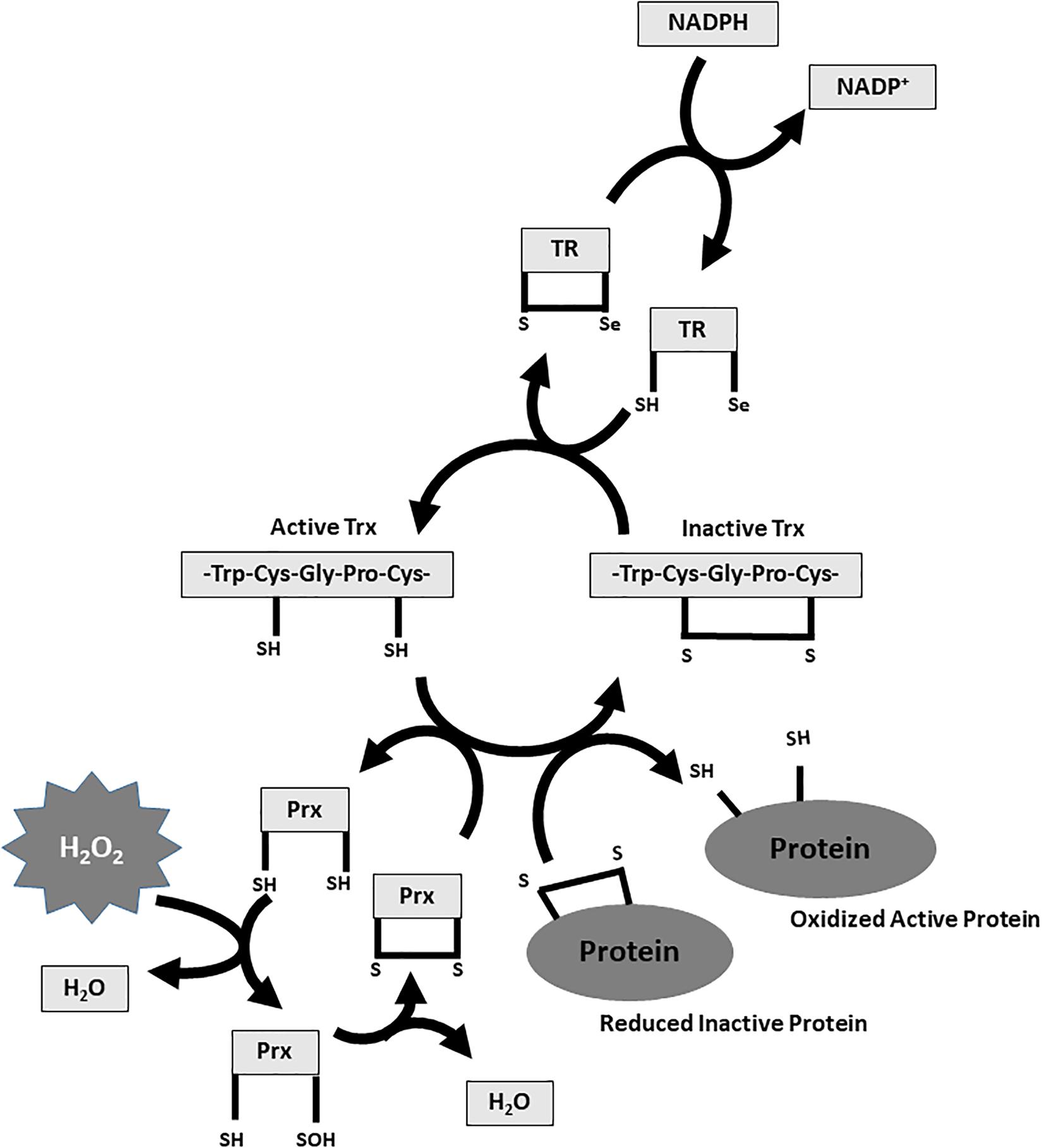
FIGURE 2. Thioredoxin and Peroxiredoxin system. Reduced Trx-SH catalyzes the reduction of disulfide (s–s) bridges within oxidized cell proteins. This process causes the oxidation of Trx-SH to Trx-disulfide which is subsequently reduced by TR at the expense of NADPH. The TR in its catalytic Trx reduction process uses Se as a cofactor. Reduced PRx-SH promotes the separation of two H2O molecules from H2O2 through a “two-step mechanism.” The peroxidic cysteine sulfhydryl group of a Prx subunit is oxidized to sulfenic acid (–SOH). Subsequently, sulfenic acid condenses with the reduced cysteine group present in the other subunit to form a disulfide bond between the units. This two-step process releases two H2O molecules from H2O2 with the oxidation of PRx-SH to PRx-SOH to PRx-disulfide. The oxidized PRx is in turn reduced by the Trx-SH. H2O2, hydrogen peroxide; NADP+, nicotinamide adenine dinucleotide phosphate oxidated form; NADPH, nicotinamide adenine dinucleotide phosphate reduced form; PRx, peroxiredoxin; TR, thioredoxin reductase; Trx, thioredoxin.
Nitric oxide is one of the most important and ubiquitous intracellular messengers that mediates several basic physiological processes including neurotransmission, vasodilatation, angiogenesis and host cell defense (MacMicking et al., 1997; Egginton, 2009; Bogdan, 2015; Philippu, 2016; Kraehling and Sessa, 2017). NO is synthesized from L-arginine by several forms of NOS, and its reaction with O2- produces ONO2- which alters mitochondrial function and causes apoptosis (Figure 1; Stuehr, 1999; Pacher et al., 2007; Radi, 2013).
Lipid Peroxidation
Lipid peroxidation, caused by increased ROS and RNS, is linked to many acute and chronic diseases of adulthood such as MOF, hemorrhagic and septic shock, ARDS, acute lung injury, cardiovascular pathology requiring surgery, aging, atherosclerosis, Parkinson, Alzheimer, diabetes mellitus, cataracts and rheumatoid arthritis, and several diseases of the neonatal period (Takeda et al., 1984; Hammond and Hess, 1985; Bertrand et al., 1989; Richard et al., 1990; Quinlan et al., 1996; Carpenter et al., 1998; Lamb et al., 1999; Motoyama et al., 2003; Comporti et al., 2004; Quinlan et al., 2004; Fläring et al., 2005; Laplace et al., 2005; Niki et al., 2005; Saugstad, 2005; Huet et al., 2007; Signorini et al., 2008; Giustarini et al., 2009; Briones and Touyz, 2010; Giacco and Brownlee, 2010; Khaper et al., 2010; Romano et al., 2010; Barrera et al., 2018; Maiorino et al., 2018; Mátyás and Zaharie, 2018; Moore et al., 2018; Orban and Singer, 2018; Perrone et al., 2018; Romano et al., 2018).
Lipid peroxidation is the oxidative decay of Puffs, which involves the abstraction of hydrogen from a carbon molecule with O2 insertion and consequent production of lipid peroxyl radicals and hydroperoxides (Tappel, 1970; Yin et al., 2011; Al-Dalaen and Al-Qtaitat, 2014). Hydroperoxides are very unstable and easily decompose into MDAs and 4-hydroxy-2-nonenal, while peroxidation of arachidonic acid produces isoprostanes (Montuschi et al., 2004; Lakshminrusimha et al., 2006; Al-Dalaen and Al-Qtaitat, 2014). The response of the cell membrane to lipid peroxidation depends on the degree of peroxidation achieved. If lipid peroxidation rate is low, the damage is repaired by anti-oxidant defense systems. On the other hand, if the lipid peroxidation rate is high, the tissue self-repairing capacity is lost, and apoptosis or programmed cell death is induced (Chaudhary et al., 2010; Ayala et al., 2014).
Oxidative Protein Damage
Protein oxidation damage does affect not only the cell membranes but also the enzyme system because it is exclusively constituted by proteins (Goodyear-Bruch and Pierce, 2002). The amino acid fragmentation, the following generation of cross-links between proteins and the oxidation of the body of the protein molecule with loss of specific (contractile, enzymatic, and transport) function is caused by ROS and RNS (Dalle-Donne et al., 2003a,b; Giustarini et al., 2009). Protein Carbonyl groups (aldehydes and ketones) are produced generally by the direct oxidation of proline, arginine, lysine, and threonine residues, or by oxidative cleavage of the protein backbone, and indirectly by lipid peroxidation or reaction of reducing sugars or their oxidation products (Dalle-Donne et al., 2003a, 2006). The damaged proteins, in turn, contribute to many disorders and diseases (Dalle-Donne et al., 2003a). Oxidized or aldehyde-modified proteins would be perceived as “foreign proteins” by the immune system, with the production of self-antibodies in rheumatoid arthritis, lupus, and scleroderma in adults (Mantle et al., 1999; Dimon-Gadal et al., 2000; Renke et al., 2000; Dalle-Donne et al., 2003a; Halliwell and Gutteridge, 2015). Moreover, always in adults, the increase of oxidized proteins has been associated with multiple diseases, like cancer, heart failure, pre-eclampsia, ARDS, Parkinson, Alzheimer, and sepsis (Floor and Wetzel, 1998; Lenz et al., 1999; Winterbourn et al., 2000; Butterfield et al., 2001; Zusterzeel et al., 2001; Abu-Zidan et al., 2002; Castegna et al., 2002). In the neonatal period, the oxidation of proteins plays a key role in some diseases such as intraventricular hemorrhage, retinopathy of prematurity, bronchopulmonary dysplasia, NEC (Gladstone and Levine, 1994; Ramsay et al., 2001; Perrone et al., 2009, 2010, 2014, 2018; Couroucli, 2017).
Oxidative DNA Damage
Oxidative DNA damage is generated by high OH● concentrations (Cooke et al., 2003). ROS can damage both purines and pyrimidines in DNA and in the free cell nucleotide pool and generates 8-hydroxy-2′-deoxyguanosine that can be used as a biomarker of the injury (Suliman et al., 2003; Bayr, 2005; Li et al., 2014). Despite the damage, the cell continues the replication process, replicating the damage and inducing the new cells to apoptosis (Roos and Kaina, 2006). Furthermore, oxidative DNA damage would be associated with other diseases such as sepsis, carcinogenesis, neurodegenerative diseases, cardiovascular diseases, and aging (Cooke et al., 2003; Niki et al., 2005; Giustarini et al., 2009; Halliwell and Gutteridge, 2015; Bahar et al., 2018; Gutteridge and Halliwell, 2018; Klaunig and Je, 2018).
Oxidative Stress and ECMO
To date, evidence of oxidative stress during ECMO are limited and are mainly related to pre-clinical studies (Möller et al., 1993; Trittenwein et al., 1999). Möller et al. (1993) observed a reduction of the antioxidant capacity of SOD and GPx on a lamb ECMO model, while increased lipid peroxidation was demonstrated on a rabbit model of ECMO (Trittenwein et al., 1999). One study on pediatric patients described two different oxidative stress patterns during ECC; in non-survivors, lipid peroxidation augmented during the first 96 h of ECMO whereas it reached a plateau after 24 h in the survivor’s group (Hirthler et al., 1992).
No data are currently available on critically ill neonates undergoing respiratory ECMO. Despite this gap of knowledge, previous evidence, mainly derived from studies on CPB and critically ill patients, suggested that oxidative stress is a relevant contributor to morbidity and mortality (Möller et al., 1993; Trittenwein et al., 1999; Brix-Christensen et al., 2001, 2002; Goodyear-Bruch and Pierce, 2002; Levy and Tanaka, 2003). ECMO shares with both CPB and critical illness common potential triggers for increased ROS generation and redox unbalance: exposure to the extracorporeal circuit and related SIRS, transfusion burden, hyperoxia, CRRT, hemolysis, and sequestration of antioxidants into the ECMO circuit (McDonald et al., 2014a). We describe hereafter the possible role of these triggers in the pathogenesis of oxidative stress-related morbidity during ECC.
ECMO and Inflammation
A SIRS-like syndrome is typically observed from the first hours after starting an ECMO procedure, as a consequence of the blood exposure to the exogenous surface of the extracorporeal device (Davies and Hagen, 1997). Despite the use of biocompatible equipment, the interaction between biological fluids and the circuit activates both the coagulation cascade and the host inflammatory response (Tayama et al., 2000; Palatianos et al., 2003; Wiesenack et al., 2004; Thiara et al., 2010). The humoral and cell-mediated immune response triggers the release of pro-inflammatory cytokines (IL-1β, TNFα, and IFNβ), leading to endothelial damage, disrupted microcirculation and multi-organ dysfunction, in case of suboptimal compensatory anti-inflammatory response (Hocker et al., 1991; Hirthler et al., 1992; Depuydt et al., 1993; Plötz et al., 1993; Fortenberry et al., 1996; Adrian et al., 1998; Graulich et al., 2002; Golej et al., 2003; Mildner et al., 2005; Adib-Conquy and Cavaillon, 2009; Mcilwain et al., 2010; He et al., 2014; Shi et al., 2014; Rungatscher et al., 2015; Wang et al., 2015). The redox biology regulates the homeostasis of innate and adaptive immune response through ROS production. Many concurrent factors (hyperoxia, blood products, sepsis, mechanical ventilation) contribute to the increased ROS generation, thus promoting an overwhelming release of pro-inflammatory cytokines (IL-1β, TNFα, and IFNβ) and eventually the SIRS-like (Wiesenack et al., 2004; Cruz et al., 2007; Chen and Nuñez, 2010; Iwasaki and Medzhitov, 2010; Bulua et al., 2011; West et al., 2011; Mittal et al., 2014).
Furthermore, the intrinsic and extrinsic coagulation cascade could be directly activated by the contact of blood with the circuit surface or lesion of endothelium, or indirectly by ROS mediation (Davie et al., 1991; Görlach, 2004, 2005; Herkert et al., 2004; Mackman et al., 2007; Oliver, 2009; Fall et al., 2015). The intrinsic coagulative pathway would be activated exclusively during the respiratory ECMO with low thrombin generation; while the release of high amount of TF and air-blood interface would stimulate both the intrinsic and extrinsic way with high thrombin generation during the CPB (Levy and Tanaka, 2003; Oliver, 2009; Kraft et al., 2015). Indeed, TF binds to Factor VII, converting it into Factor VIIa and activating the common coagulation cascade (Davie et al., 1991; Levy and Tanaka, 2003; Mackman et al., 2007; Oliver, 2009). The intrinsic pathway is activated through the conversion of Factor XII into Factor XIIa within 10 min from the beginning of the extracorporeal procedure and is associated with an increase in Bradykinin, Kallikrein, and activation of Factor IX (Wendel et al., 1999; Millar et al., 2016). Bradykinin stimulates NO, TNFα, and IL-10 production, while Kallikrein directly activates neutrophils (Rodell et al., 1995; Wachtfogel et al., 1995). Factor IXa induces the activation of Factor X which in turn causes the conversion of prothrombin into thrombin and fibrinogen in fibrin resulting in thrombus formation (Millar et al., 2016). Thrombin stimulates the endothelial production of P-selectin, E-selectin, and platelet activating factor, thus increasing the adherence and activation of neutrophils and the expression of proinflammatory cytokines (Prescott et al., 1984; Zimmerman et al., 1985; Kaplanski et al., 1997; Levy and Tanaka, 2003). Additionally, thrombin stimulates the production of ROS in endothelial and smooth muscle cells, by stimulating NOX and by up-regulating the expression of NOX sub-unit p22phox in a kinase-dependent manner (Görlach et al., 2002; Djordjevic et al., 2005).
Oxidative stress induces platelets activation and aggregation which, in turn, increases ROS production. Neutrophils participate in the amplification of the immune response; although multifocal, the activation of neutrophils occurs mainly in the oxygenator; their margination in the artificial lung, observed both in animal models and in newborns, contribute to the deterioration of the artificial membrane during ECMO (Plötz et al., 1993; Fortenberry et al., 1996; Kotani et al., 2000; Brix-Christensen et al., 2002; Mcilwain et al., 2010; Rungatscher et al., 2015).
In conclusion, the mechanism of ECMO-induced oxidative stress is a multifactorial process, in which the interaction between the immune response, the coagulation cascade, and endothelial cells amplifies ROS generation (Figure 3).
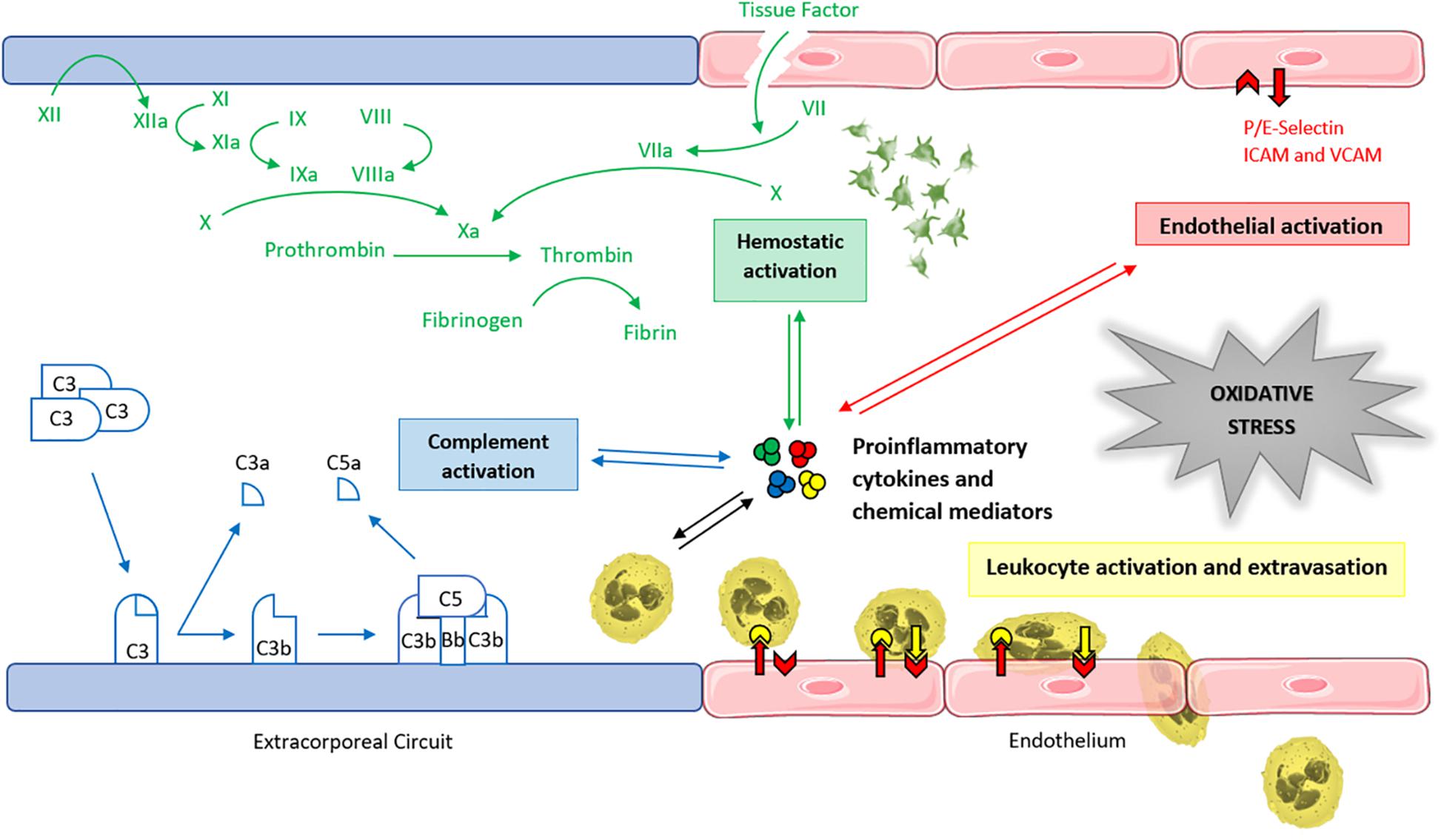
FIGURE 3. Main determinants of ECMO-induced oxidative stress. Graphical representation of the interaction between the coagulation cascade, the immune response, and the endothelium in the multifactorial amplification process of the ECMO-related redox unbalance. ICAM, intercellular adhesion molecule. VCAM, vascular cell adhesion molecule.
ECMO and Blood Transfusion
Blood products have been associated with increased morbidity and mortality in critically ill patients (Koch et al., 2008; Wang et al., 2012; Ghirardello et al., 2017). Transfusion-related oxidative stress increases with the volume, and the number of PRBC transfused (Shohat et al., 1983; Silvers et al., 1998; Dani et al., 2001; Collard et al., 2004). Rosa et al. (2011) observed that blood transfusions were associated with a significant increase in oxidative stress and a reduction of IL-6 concentration in critically ill adults. The transfusion of aged PRBC was associated with an increase of oxidative stress, as a consequence of the reduced Se content in older PRBC. Se is an essential trace element, which is incorporated into selenoproteins, some of which have antioxidants function (i.e., GPx) (McDonald et al., 2014b). In premature newborns, RBC transfusions were associated with an increase of MDA, a lipid peroxidation product, both in the bronchoalveolar lavage fluid and in urine (Wardle et al., 2002). This data suggested that RBC transfusion could increase pulmonary oxidative damage and worse prognosis (Collard et al., 2005).
Extracorporeal membrane oxygenation, as well as CPB or exchange transfusion, are procedures that expose patients to a significant increase in PRBC transfusions and therefore to oxidative stress (McCoy-Pardington et al., 1990; Luban, 1995; Butch et al., 1996; Ang et al., 2009). Newborns receive an average of 60 ml/kg/day of PRBC during an ECMO procedure (Stiller et al., 2004). This high blood requirement depends on (1) priming of the ECMO circuit with blood, to avoid acute hemodilution, (2) frequent blood samples for coagulation tests, (3) hemolysis-related anemia due to centrifugal pumps and, occasionally, (4) spontaneous bleeding from cannulation sites and surgical wounds. According to the ELSO registry, these latter events represents about 19% of all hemorrhagic complications during neonatal respiratory ECMO, impacting on survival (ELSO). Overall, the mortality rate is increased among neonates exposed to large volumes of PRBC transfusions (Smith et al., 2013; Fiser et al., 2014; Ghirardello et al., 2017). Dos Santos et al. observed a 27.8% mortality in critically ill infants exposed to transfusions during the hospital stay, with an increased relative risk of death of 1.49 in those infants who received at least one transfusion compared to controls (Dos Santos et al., 2011). In a cohort of extremely low birth weight infants, the incidence of mortality was 24% in patients transfused compared to 8.5% in the control group (Ghirardello et al., 2017). Similarly, Smith and co-authors observed a 24% increase in the odds of hospital death associated with every 10 ml/kg/day of PRBC transfusion in patients during respiratory ECMO (Smith et al., 2013).
In addition to PRBC, newborns on ECMO require approximately 25 ml/kg/day of PCs (Stiller et al., 2004). Platelet count is reduced by 40–50% during ECMO, partly due to the activation of the coagulation cascade (Nawab and Williams, 2017). Moreover, PCs are a source of ROS, especially , which modulate platelet activation and aggregation (Krötz et al., 2002; Begonja et al., 2005). The increase in ROS, whose impact on the patient’s oxidation state is still unknown, is mainly related to the storage process (Sonego et al., 2017).
ECMO and Hemolysis
ECMO-related hemolysis is a common phenomenon among pediatric patients and represents an independent predictor of mortality (Omar et al., 2015; Dalton et al., 2018). It consists of the RBCs lysis with following release of hemoglobin into the plasma (Rother et al., 2005). The shear stress is the main causative mechanism for hemolysis during mechanical support, and some risk factors have been identified, such as the negative inlet pressure, pump speed, pump and oxygenator type, cavitation and priming solution (Steinhorn et al., 1989; Lou et al., 2014; Rother et al., 2005). In addition, oxidative stress is a determinant of hemolysis (Boyle et al., 1997; Walski et al., 2018). Lipid peroxidation and protein damage of cell membranes from ROS cause enzymatic inactivation, depolarization of the cell membrane and a change in its permeability, resulting in cell lysis (Matés et al., 1999; Kirkham and Rahman, 2006). The mechanical and oxidative stress during ECMO leads to the release of the PFHb, which is cleared by hemoglobin scavengers (Dalton et al., 2018). However, in case of severe hemolysis, the hemoglobin-scavenging mechanisms become saturated, resulting in hemoglobinemia and hemoglobinuria (Rother et al., 2005). The PFHb may exert either a direct toxic effect (i.e., renal damage) or may cause endothelial dysfunction, by binding NO. The NO consumption leads to increased systemic and pulmonary resistance, platelet activation and aggregation, endothelial adhesion, fibrin deposition and thrombin formation (Rother et al., 2005).
Moreover, the shear stress induces procoagulant conformational changes of the pVWF, which interacts with circulating platelets enhancing their adhesion and aggregation properties (Cruz et al., 2018).
Furthermore, high levels of PFHb inhibit the cleavage of pVWF by ADAMTS-13, contributing to increased clotting within the mechanical devices (Da et al., 2015). Eventually, hyperbilirubinemia and iron release constitute additional drivers of the hemolysis-induced morbidity and mortality (Davis et al., 1999; Kaplan et al., 2014). Besides hemolysis, the mechanical stress may hesitate into sublethal RBCs damage, leading to increased fragility and decreased deformability of RBCs (Kameneva et al., 1999). Their consequent failure to enter small capillaries causes end-organ dysfunction, through the impairment of tissue oxygenation and microcirculation (Watanabe et al., 2007). Based on the concepts mentioned above, ECMO providers should monitor the hemolysis, as it is strictly associated with increased mortality and morbidity (kidney injury, vascular impairment, need for PRBC and PCs transfusion, thrombosis).
Moreover, efforts should focus on the technological progress, because of specific components of the circuit act as main drivers of the ECMO-induced hemolysis, such as the oxygenator (Williams et al., 2015). The use of smaller pediatric oxygenators was associated with greater shear stress and, hence, increased hemolysis, when compared to larger adult oxygenators (Williams et al., 2015). Although the relative contribution of each circuit component on the hemolysis and related oxidative stress is unknown, the use of a centrifugal pump and small size connectors should be considered as potential sites of increased hemolysis (Lou et al., 2014; Williams et al., 2015). Furthermore, the use of red-to-infrared radiation (R/NIR) to stabilize erythrocyte membranes with increased resistance to oxidative stress seems promising (Itoh et al., 2000; Komorowska et al., 2001; Chludzińska et al., 2005; Walski et al., 2015, 2018).
Technology advances should target the development of new, more bio-compatible, circuit-blood interfaces in order to reduce hemolysis and oxidative stress.
ECMO and Hyperoxia
The antioxidant capacity of tissues is overtaken with O2- production during hyperoxia. Oxygen toxicity in the perinatal period is well established, and many efforts have been made to define safety targets during neonatal resuscitation and care (Tin and Gupta, 2007; Auten and Davis, 2009; Finer and Leone, 2009; Askie et al., 2011; Saugstad et al., 2012; Vento et al., 2012; Schmidt et al., 2013; Saugstad and Aune, 2014; Manja et al., 2015).
Nevertheless, hyperoxia remains a significant driver for radical O2 species generation, especially during ECC (Cashen et al., 2018). Hyperoxia is frequent during cardiac surgery in CPB and seems to play a key role in the development of oxidative stress as a consequence of post-ischemic reperfusion (Cavarocchi et al., 1986; Börgermann et al., 2007). The clamp of the aorta induces heart and lung ischemia while declamping generates reperfusion, and either action produces an inflammatory response. On-pump cardiac surgery is associated with increased oxidative stress if compared to off-pump surgery (Matata et al., 2000; Cavalca et al., 2006). The post-ischemic reperfusion, rather than hyperoxia per se, seems to play a role; there is evidence from pediatric cardiac surgery that isolated hyperoxia does not increase oxidative stress and inflammation if compared with normoxia (Hövels-Gürich et al., 2002; Caputo et al., 2005, 2009). Although ECMO does not directly cause ischemia/reperfusion, patients requiring ECMO experience prolonged periods of pre-ECMO hypoxia causing an increase in oxidative stress (Crimi et al., 2006; Hayes et al., 2013; Chen Q. et al., 2014). ROS generation following hyperoxia is significantly exaggerated when hyperoxia is subsequent a period of hypoxia, due to the dysfunction of the post-ischemic mitochondrial electron transport chain (Neumar, 2011). Sznycer-Taub et al. (2016) identified hyperoxia within 48 h of ECMO following cardiac surgery as an independent risk factor for mortality at 30 days post-operatively, while Trittenwein et al. (1999) observed how VV-ECMO more than VA-ECMO would increase pulmonary lipid peroxidation following endotoxemia-induced hypoxia. Lipid peroxidation of the lung is a known consequence of sepsis and inflammation, but it has also been described as a consequence of tissue reperfusion (Demling et al., 1991; Horton and Walker, 1993; Christie et al., 1994; Chow et al., 2003). In the study by Trittenwein et al. (1999) hyperoxic reperfusion of the hypoxic lung following VV-ECMO, caused a significant increase of plasma MDA as an index of lipid peroxidation, higher than that observed in VA-ECMO where the hyperoxic blood supply to the lung is less.
More recently, similar results have been observed by Cashen et al. (2018) that showed higher in-hospital mortality among hyperoxic patients submitted to ECMO compared to normoxics, ascribing the high mortality to the effects of ROS on poor cardiac output rather than the SIRS-like syndrome, hemostatic activation and MOF.
ECMO and Continuous Renal Replacement Therapies
Oxidative stress is a common condition in kidney diseases (Witko-Sarsat et al., 1996; Hasselwander and Young, 1998); it is induced by renal pathology itself, reduced antioxidant intake associated with malnutrition, CRRT with the loss of antioxidant molecules during filtration and the contact of blood with CRRT circuit (Wolf et al., 1951; Hasselwander and Young, 1998; Cano, 2001; Sosa et al., 2006; Varan et al., 2010; Libetta et al., 2011; Coombes and Fassett, 2012; Ozbek, 2012). The SIRS-like syndrome, which typically occurs in the early hours of ECMO, is associated with hypotension, oligo-anuria, decreased lung compliance, pulmonary edema, increased plasma concentrations of pro-inflammatory cytokines and neutrophils’ activation (Hocker et al., 1991; Davies and Hagen, 1997). AKI and FO complicate neonatal ECMO runs in more than half of cases, with a negative impact on survival rates (Heiss et al., 1987; Weber et al., 1990; Swaniker et al., 2000; Gadepalli et al., 2011; Zwiers et al., 2013). Therefore, hemofiltration and hemodialysis have become a standard of care in the management of AKI during ECMO (Heiss et al., 1987; Meyer et al., 2001; Paden et al., 2011; Chen H. et al., 2014). During CRRT, inflammation is activated by an increase of ROS and inflammatory cytokines (TNFα, IL-1, and IL-6) (Tarakçıoğlu et al., 2003; Borazan et al., 2004) and a reduction of antioxidant proteins as hydrosoluble vitamin C and uric acids (Hegbrant and Hultkvist, 1999; Yang et al., 2006; Libetta et al., 2011). The use of biocompatible filtration systems by using vitamin E-coated filters reduces the oxidative stress linked to the contact of blood with exogenous materials (Yang et al., 2014). Selewski et al. (2012) suggested an early filtration treatment to anticipate FO, and reduce mortality rate. As reported by ELSO registry, about 33% of neonatal respiratory ECMO and 38% of cardiac ECMO require renal replacement therapy (ELSO). As a result of the combination of two ECC systems, the hemolysis-related oxidative stress would increase (Chen H. et al., 2014). The in-line use of CRRT would limit the ECMO-related inflammation by reducing the concentrations of TNFα, IL-1β, IL-6, and IL-8 (Jialiang et al., 2014).
ECMO Post-cardiopulmonary Bypass
Adult patients with cardiovascular diseases and neonatal patients with congenital cyanotic heart diseases have an increased level of oxidative stress, even before heart surgery, if compared to the healthy population (Ercan et al., 2009; Giustarini et al., 2009; Karu et al., 2010; Small et al., 2012).
Moreover, cardiac surgery is always associated with both systemic inflammation and a variable degree of oxidative stress, which might contribute to post-operative complications, failure of single and multiple organs and mortality (Kim et al., 2008; Billings et al., 2011; Antoniades et al., 2012; Plicner et al., 2014; Wu et al., 2015). Plicner et al. (2014) observed that the increase of asymmetric dimethylarginine and 8-iso-prostaglandin F2α was associated with an unfavorable outcome of patients undergoing coronary artery bypass grafting. Similarly, Antoniades et al. (2012) has shown how the increase of O2- and ONO2- concentration was closely related to atrial fibrillation, need for inotropic drugs and lengthening of hospital stay during post-cardiac surgery.
The main cause of oxidative stress during cardiac surgery is the exposure to the foreign surface of the extracorporeal circuit, hypothermia, and circulatory arrest associated with systemic inflammation, ischemia-reperfusion injury, altered hemostatic status (Prasad et al., 1992; Edmunds, 1998; Clermont et al., 2002; Levy and Tanaka, 2003; Ulus et al., 2003; Gessler et al., 2004; Karu et al., 2010). Approximately 3–5% of patients undergoing congenital cardiac surgery require post-CPB extracorporeal support, due to the weaning failure following myocardial dysfunction, low cardiac output state, cardiac or respiratory arrest, pulmonary hypertension, or shunt occlusion (Aharon et al., 2001; Salvin et al., 2008; Mascio et al., 2014). The long duration of ECMO post-CPB might impair the organ function, thus predisposing to an increased risk of complications (i.e., sepsis) and mortality (Duncan et al., 1999; Chaturvedi et al., 2004; Salvin et al., 2008; Kumar et al., 2010; Rood et al., 2011; ELSO, 2018).
Clinical Relevance of Oxidative Stress During Neonatal ECMO
The role of continuing oxidative stress has been established in the long term, in a variety of chronic diseases, such as diabetes mellitus, atherosclerosis and neurodegenerative illness (Haidara et al., 2006). Currently, the interest is moving toward the effects of the redox unbalance in the acute illness (Bar-Or et al., 2015). Here, we summarize the available evidence in relation to the role of the oxidative stress in critical conditions, which often occur during ECMO (Figure 4).
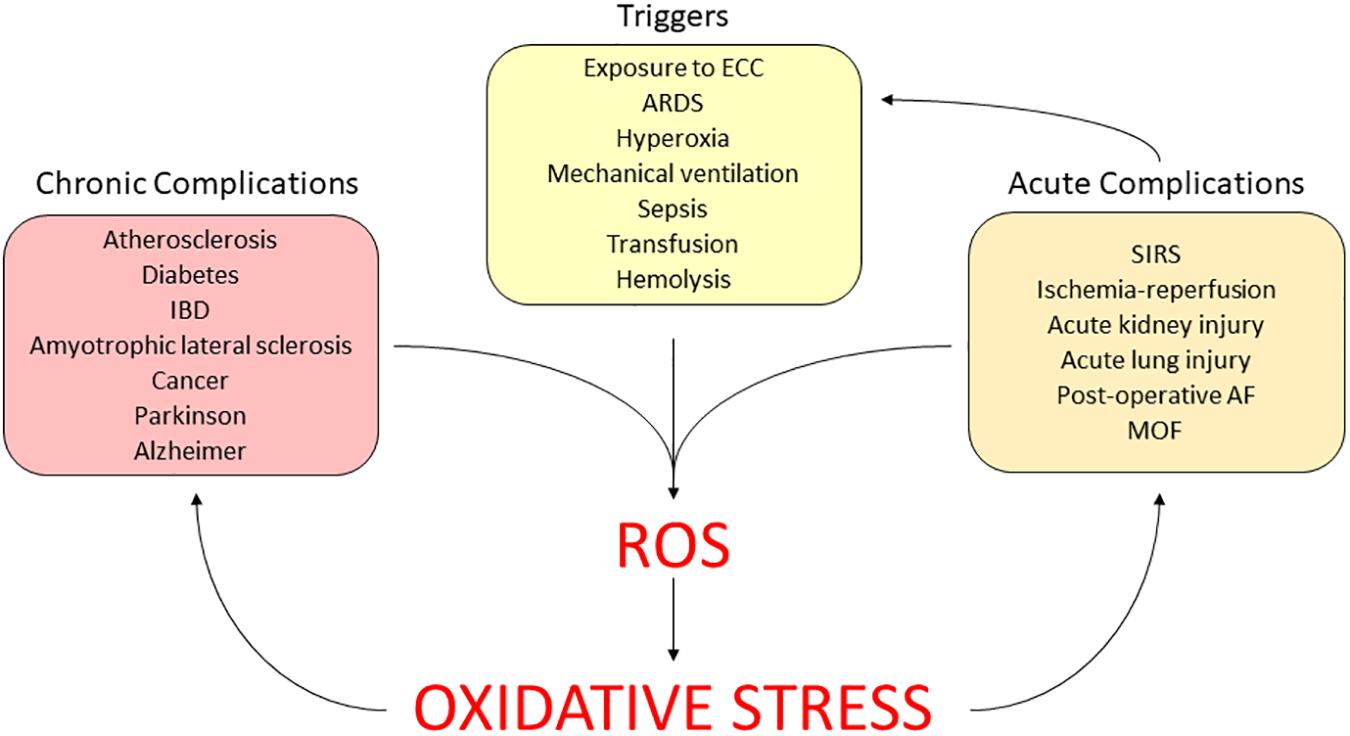
FIGURE 4. Clinical relevance of ECMO-induced oxidative stress. AF, atrial fibrillation; ARDS, acute respiratory distress syndrome; ECC, extracorporeal circuit; IBD, intestinal bowel disease; MOF, multi-organ failure; SIRS, systemic inflammatory response syndrome.
Sepsis and Multi-Organ Failure
A wealth of studies has shown that the normal redox balance is altered in septic shock and multiple organ failure, due to both an increase in derived free radicals and a decrease in the plasma antioxidant potential (Ogilvie et al., 1991; Goode et al., 1995). Adult septic patients have higher plasma CAT and SOD if compared to healthy controls (Warner et al., 1995). Moreover, non-survivors of sepsis have shown higher levels of SOD and a reduced plasmatic antioxidant status, which was associated with unfavorable outcomes (Cowley et al., 1996; Guerreiro et al., 2010). The sepsis-related MOF, which remains the major cause of death in intensive care units, is associated with oxidative stress and mitochondrial dysfunction (Brealey et al., 2002; Galley, 2011). Two antioxidants targeting the protection of mitochondria (MitoQ and MitoVitE) through the reduction of oxidative stress have been beneficial in a rat model of acute sepsis (Lowes et al., 2013). Other potential oxidative-induced mechanisms for MOF development have been proposed, as a result of the anti-oxidant impairment secondary to GSH and Se depletion, suggesting a positive effect of Se supplementation (Brealey et al., 2002; Manzanares et al., 2012; Broman et al., 2018).
Hemostatic Derangement and Anticoagulation Management
Maintaining the hemostatic homeostasis during ECMO is extremely challenging and, despite technical advances, bleeding and thrombosis remain serious complications (Murphy et al., 2015). The hemostatic perturbation during ECMO is a multifactorial process, and the redox imbalance is one of the main contributing factors. Besides the dilutional coagulopathy, which depends on priming compositions and affects both clotting factors and platelets, the shear stress has a key role as it induces platelet dysfunction, fibrinolysis activation, acquired von Willebrand syndrome and intravascular hemolysis (Görlach et al., 2002; Görlach, 2004, 2005; Fall et al., 2015). As previously mentioned, the oxidative stress is strictly associated with the shear stress, resulting in endothelial activation (see section “ECMO and Inflammation”) and enhanced hemolysis (see section “ECMO and Hemolysis”) (Görlach et al., 2002; Görlach, 2004, 2005; Herkert et al., 2004; Djordjevic et al., 2005; Millar et al., 2016).
Moreover, the exposure to the exogenous circuits triggers a SIRS-like syndrome, which is associated with the ROS generation, the release of pro-inflammatory cytokines, the activation of the intrinsic coagulation pathway with a potential procoagulant effect (Millar et al., 2016). The clot formation triggers the consumption of coagulation factors and platelets, thus increasing the risk of disseminated intravascular coagulopathy and bleeding (Millar et al., 2016). The anticoagulation management should be modulated, by taking into account the drivers of hemostatic unbalance mentioned above.
Biomarkers of the Redox State
Based on the relevant clinical implication of the redox unbalance during ECMO, the identification of diagnostic markers would help in the detection, monitoring, and follow-up of the redox potential in ECMO neonates. However, the profile of biomarkers of both oxidative stress and antioxidant defenses during neonatal ECMO has not been characterized yet. Evidence can be derived from previous pre-clinical and clinical studies, which evaluate the pro-oxidative potential during and after the exposure to an extracorporeal circuit (i.e., CRRT, CPB) (Takouli et al., 2010; McDonald et al., 2012). As most of the research has been performed on the adult population, the evaluation of the redox status in neonatal systemic diseases (i.e., septic shock) may provide further insights into the age-related antioxidant deficiency (Batra et al., 2000). Among potential pro-oxidant biomarkers, the d-ROMs have been evaluated in a dialyzer, while the MDA has been applied in a porcine ECMO model (Takouli et al., 2010; Chen Q. et al., 2014).
Additionally, as nitro-oxidative stress is involved in a large variety of pathological mechanisms, the biomarkers validated for the detection of nitrosation and nitration would be of interest (Cipak Gasparovic et al., 2017). However, their application in the extra-corporeal setting is still lacking. The anti-oxidant response may be evaluated through the detection of the TAC, SOD, and GSx activity (Takouli et al., 2010; McDonald et al., 2012). Moreover, the reduction of key trace elements, such as Se, copper, and zinc might suggest a reduced anti-oxidant activity (McDonald et al., 2012). In clinical practice, the oxidative stress may be indirectly presumed through the monitoring of inflammatory markers, such as the increased levels of IL-6 and IL-8 or by evaluating the hemolysis with the PFHb levels (Rother et al., 2005; Takouli et al., 2010; Cruz et al., 2018).
Strategies to Reduce Oxidative Stress: Update on Antioxidants
The ROS production is regulated by intra and extracellular antioxidant systems, which are impaired during the perinatal period and critical illness (Manzanares et al., 2012; McDonald et al., 2012). ECMO further complicates the issue, by adding specific sources for redox state unbalance (Manzanares et al., 2012; McDonald et al., 2012, 2014a; Jialiang et al., 2014; Millar et al., 2016; Bocca et al., 2017). The antioxidant system of the newborns is immature with both a reduction of antioxidant enzymes (SOD, CAT, and GPx) and non-enzymatic antioxidants (vitamin E, β-carotene, melatonin, ceruloplasmin, transferrin, coenzyme Q) while ascorbic acid and bilirubin are present only for a short period after birth (Gopinathan et al., 1994; Gitto et al., 2009; Bocca et al., 2017). Free iron levels are significantly higher than in adults, causing an increased Fenton reaction, which in turn stimulates the production of OH● (Saugstad, 2005). Furthermore both the SIRS-like and the sequestration into the ECMO/hemofilter circuit reduce the level of trace elements (copper, manganese, zinc, iron, Se as cofactors for SOD, GPx, and CAT function) and non-enzymatic antioxidants (albumin, uric acid, vitamins C and E) (Cano, 2001; Yamamoto et al., 2003; Yamawaki and Berk, 2006; Berndt et al., 2007; Tonelli et al., 2009; Hoffmann et al., 2011; Visser et al., 2011; Manzanares et al., 2012; Wołonciej et al., 2016; Ciapetti et al., 2018).
Based on the paucity of data regarding patients in ECMO both neonatal and adult, we will illustrate previous data regarding the use of anti-oxidants in critically ill adult patients undergoing surgery, CRRT or CPB. Moreover, taking into account the peculiarity of the perinatal period, we will mention some data on anti-oxidant strategies studied in neonatal conditions where oxidative stress is one of the pathogenic mechanisms, such as sepsis or NEC (Ozdemir et al., 2012; Poggi and Dani, 2018) (Supplementary Table S1). Among others, vitamin A, vitamin E, vitamin C, SOD, and NAC have been studied to reduce tissue damage mediated by oxidative stress with contrasting results (Tyson et al., 1999; Suresh et al., 2001; Wardle et al., 2001; Brion et al., 2003; Darlow et al., 2016).
Antioxidants have two main mechanisms of action: the prevention, inactivating the free radical present in the systems and the scavenging of the active radical, suppressing the chain initiation or breaking the chain propagation. They may exert their effect by many actions including electron donation, hydrogen donor, metal ion chelation, co-antioxidants, radical scavenger, peroxide decomposer, singlet oxygen quencher, enzyme inhibitor or by gene expression regulation (Lobo et al., 2010).
Selenoproteins contribute to the function of many enzymes among which GSx, TRs, and methionine sulfoxide reductase and seem to protect the cardiovascular system against oxidative stress (Rotruck et al., 1973; Kim and Gladyshev, 2004; Hoffmann and Berry, 2005; Berndt et al., 2007; Hoffmann et al., 2011). Based on the reduction of Se levels in critically ill patients or after cardiac surgery, Se supplementation has been investigated in this contexts with contradictory results (Manzanares et al., 2012; Stoppe et al., 2013; Broman et al., 2018). To date, there are no data to support its extended use (Allingstrup and Afshari, 2015; Manzanares et al., 2016). Although not conclusive, neonatal supplementation with oral or parenteral Se in the first weeks of life to preterm infants led to a reduction in the proportion of infants having one or more episodes of sepsis (Darlow and Austin, 2003). The administration of pre and post-operative vitamin C in cardiac surgical adults has led to a reduction of post-operative atrial fibrillation, which seems to be associated with increased atrial oxidative stress and ONO2- formation (Carnes et al., 2001).
Moreover, supplementation with ascorbic acid caused a reduction of plasma levels of oxidative stress during hemodialysis and restoration of endothelial function in critically ill patients (Eiselt et al., 2001; Wilson, 2009).
Similarly, vitamin E-coated dialysis membranes used in adult patients on hemodialysis led to a decrease in reactive oxygen metabolites and derivatives, an increase of TAC and superoxide dismutase (Takouli et al., 2010). In addition, a decrease in post-operative atrial fibrillation has been documented after the supplementation of pre and post-operative NAC in surgical adult patients (Ozaydin et al., 2008). Ozdemir et al. (2012) have shown a beneficial effect of NAC in a murine NEC model in terms of reduced levels of oxidative stress (MDA) and inflammation (TNFα), with increased antioxidant activity of the SOD. Also concerning the supplementation of glutamine, data are not conclusive. Indeed, previous studies showed that infusion of glutamine during and for 3 days after cardio-surgery in adult patients led to an increase of glutamine blood levels and GSH activity without clinical improvement (Engel et al., 2009). Enteral glutamine either alone or in combination with arginine has been tested in healthy newborn rats showing a reduction of oxidative stress. These results may suggest a potential benefit of glutamine and arginine supplementation in the prevention of NEC in premature neonates with insufficient oxidative resistance (Kul et al., 2009). The evidence is building on the neonatal use of the ROS scavenger melatonin. Its supplementation in newborns with sepsis within the first 12 h after diagnosis has led to a reduction of lipid peroxidation products (MDA) and improvement of clinical outcomes (Gitto et al., 2001). The post-operative administration of melatonin reduced the proinflammatory cytokines and NO levels in newborns undergoing surgery (Gitto et al., 2004).
Moreover, a reduction of late-onset sepsis risk ratio has been shown in newborn supplemented with lactoferrin and a reduction of the risk of antibiotic treatment failure in septic newborns exposed to zinc (Bhatnagar et al., 2012; Pammi and Suresh, 2017). Additional promising agents are the apocynin, an NOX inhibitor applied in the animal model of stroke and brain injury induced by ischemia-riperfusion, and the α-lipoic acid, a GSH-mediated antioxidant used in adults undergoing surgery during ECC (Packer et al., 1997; Kahles et al., 2007; Lambeth et al., 2008; Aly et al., 2009; Chen H. et al., 2009; Choi et al., 2010; Xia et al., 2010; Simonyi et al., 2012; Uyar et al., 2013). Novel antioxidant molecules, such as the ROS scavenger edaravone, showed promising results. In a pre-clinical model of neonatal sepsis the administration of edaravone 30 min after the injury has led to both a biochemical and clinical improvement (Kato et al., 2009). Other agents like hyperbaric oxygen and medical ozone have been suggested to reduce oxidative stress by enhancing the antioxidant system. The technique based on the use of O2/ozone mix was previously applied in CRRT with promising results, even if its mechanism of action remains still unveiled (Bocci et al., 1999, 2001; Bocci and Paolo, 2004; Di Paolo et al., 2005). Data on the use of ozone in ECMO are lacking and might be a future area of interest. Lastly, it is worth mentioning the potential role of hMSCs in ischemia/reperfusion-induced lung injury, by acting through anti-oxidant, anti-inflammatory, and anti-apoptotic defense pathways (Ortiz et al., 2003; Gupta et al., 2007; Xu et al., 2007; Lee et al., 2009; Gotts and Matthay, 2011; Liu et al., 2017).
Conclusions and Future Perspectives
The imbalance of the redox system induced by the ECC is a field of active research, but many aspects of neonatal ECMO and its potential harm remain unsolved. Key points for both caregivers and researchers of the perinatal area addressing the burden of ECMO should include the following:
• Improve our knowledge of the mechanisms of oxidative stress during neonatal ECMO, both in pre-clinical and clinical settings, with a focus on the biochemical and cellular mechanism that could contribute to multi-organ damage.
• Define biomarkers for oxidative stress that could be a potential target for pharmacological approaches.
• Support technological research to improve ECMO circuit miniaturization and biocompatibility.
• Prevent the redox state unbalance during ECMO, through the promotion of rational use of blood products, the maintenance of well-defined O2 targets, the prevention of hemolysis and AKI, the timely resort to in-line renal replacement therapy, the simplification of ECMO circuit lines.
Author Contributions
GC, GR, SG, SP, and FM conceived and designed the review. GC, GR, and SG wrote the first draft of the manuscript. All authors contributed to manuscript critical revision, read, and approved the submitted version.
Conflict of Interest Statement
The authors declare that the research was conducted in the absence of any commercial or financial relationships that could be construed as a potential conflict of interest.
Acknowledgments
The authors would like to thank all the Neonatal ECMO Team Mangiagalli of the Fondazione IRCCS Ca’ Granda Ospedale Maggiore Policlinico: nurses and neonatologists of the NICU, surgeons of the Department of Pediatric Surgery, anesthesiologists of the Pediatric Anesthesiology and Intensive Care Unit, nurses of the operating room. A special thank to Dr. Gabriele Zuanetti for the graphical support.
Supplementary Material
The Supplementary Material for this article can be found online at: https://www.frontiersin.org/articles/10.3389/fphys.2018.01739/full#supplementary-material
Abbreviations
AKI, acute kidney injury; ARDS, acute respiratory distress syndrome; ATP, adenosine triphosphate; CAT, catalase; CPB, cardiopulmonary bypass; CRRT, Continuous Renal Replacement Therapies; Cys–S-, cysteine thiolate anion; Cys-SO2H, cysteine sulfinic acid; Cys-SO3H, cysteine sulfonic acid; Cys–SOH, cysteine sulfenic acid; d-ROMs, reactive oxygen metabolism and derivatives; ECC, extracorporeal circulation; ECMO, extracorporeal membrane oxygenation; ELSO, extracorporeal life support organization; Fe2+, ferrous ions; Fe3+, ferric ions; FO, fluid overload; GPx, glutathione peroxidases; Grx, glutaredoxin; GSH, glutathione; H2O2, hydrogen peroxide; hMSCs, hypoxia-preconditioned mesenchymal stem cells; IFNβ, interferon β; IL, interleukin; MAS, meconium aspiration syndrome; MDA, malondialdehyde; MOF, multi-organ failure; NAC, N-acetylcysteine; NADP+, nicotinamide adenine dinucleotide phosphate oxidated form; NADPH, nicotinamide adenine dinucleotide phosphate reduced form; NEC, necrotizing enterocolitis; NO, Nitric oxide; NOS, nitric oxide synthase; NOX, NADPH oxidase; O2, oxygen; O2-, superoxide anion; OH, hydroxyl radicals; ONO2-, peroxynitrite; PCs, platelet concentrates; PFHb, plasma free hemoglobin; PPH, persistent pulmonary hypertension; PRBC, packed red blood cells; PRx, peroxiredoxins; PUFA, polyunsaturated fatty acid; pVWF, plasmatic Von Willebrand factor; RBCs, red blood cells; RDS, respiratory distress syndrome; RNS, reactive nitrogen species; ROS, reactive oxygen species; Se, selenium; SIRS, systemic inflammatory response syndrome; SOD, superoxide dismutases; TAC, total antioxidant capacity; TF, tissue factor; TNFα, tumor necrosis factor α; TR, thioredoxin reductase; Trx, thioredoxin; VA-ECMO, venous-arterial ECMO; VV-ECMO, venous-venous ECMO.
References
Abu-Zidan, F. M., Plank, L. D., and Windsor, J. A. (2002). Proteolysis in severe sepsis is related to oxidation of plasma protein. Eur. J. Surg. 168, 119–123. doi: 10.1080/11024150252884359
Adib-Conquy, M., and Cavaillon, J.-M. (2009). Compensatory anti-inflammatory response syndrome. Thromb. Haemost. 101, 36–47. doi: 10.1160/TH08-07-0421
Adrian, K., Mellgren, K., Skogby, M., Friberg, L. G., Mellgren, G., and Wadenvik, H. (1998). Cytokine release during long-term extracorporeal circulation in an experimental model. Artif. Organs 22, 859–863. doi: 10.1046/j.1525-1594.1998.06121.x
Afolayan, A. J., Eis, A., Alexander, M., Michalkiewicz, T., Teng, R.-J., Lakshminrusimha, S., et al. (2015). Decreased endothelial nitric oxide synthase expression and function contribute to impaired mitochondrial biogenesis and oxidative stress in fetal lambs with persistent pulmonary hypertension. Am. J. Physiol. Lung Cell. Mol. Physiol. 310, L40–L49. doi: 10.1152/ajplung.00392.2014
Afolayan, A. J., Eis, A., Teng, R.-J., Bakhutashvili, I., Kaul, S., Davis, J. M., et al. (2012). Decreases in manganese superoxide dismutase expression and activity contribute to oxidative stress in persistent pulmonary hypertension of the newborn. Am. J. Physiol. Lung Cell. Mol. Physiol. 303, L870–L879. doi: 10.1152/ajplung.00098.2012
Aharon, A. S., Drinkwater, D. C. Jr., Churchwell, K. B., Quisling, S. V., Reddy, V. S., Taylor, M., et al. (2001). Extracorporeal membrane oxygenation in children after repair of congenital cardiac lesions. Ann. Thorac. Surg. 72, 2095–2102. doi: 10.1016/S0003-4975(01)03209-X
Al-Dalaen, S., and Al-Qtaitat, A. (2014). Review article: oxidative stress versus antioxidants. Am. J. Biosci. Bioeng. 2, 60–71. doi: 10.11648/j.bio.20140205.11
Allingstrup, M., and Afshari, A. (2015). Selenium supplementation for critically ill adults. Cochrane Database Syst. Rev. 7:CD003703. doi: 10.1002/14651858.CD003703.pub3
Aly, H. A., Lightfoot, D. A., and El-Shemy, H. A. (2009). Modulatory role of lipoic acid on lipopolysaccharide-induced oxidative stress in adult rat Sertoli cells in vitro. Chem. Biol. Interact. 182, 112–118. doi: 10.1016/j.cbi.2009.08.013
Ang, A., Teo, D., Lim, C., Leou, K., Tien, S., and Koh, M. (2009). Blood transfusion requirements and independent predictors of increased transfusion requirements among adult patients on extracorporeal membrane oxygenation–a single centre experience. Vox Sang. 96, 34–43. doi: 10.1111/j.1423-0410.2008.01110.x
Annagür, A., Örs, R., Altunhan, H., Kurban, S., Ertuǧrul, S., Konak, M., et al. (2015). Total antioxidant and total oxidant states, and serum paraoxonase-1 in neonatal sepsis. Pediatr. Int. 57, 608–613. doi: 10.1111/ped.12557
Antoniades, C., Demosthenous, M., Reilly, S., Margaritis, M., Zhang, M.-H., Antonopoulos, A., et al. (2012). Myocardial redox state predicts in-hospital clinical outcome after cardiac surgery: effects of short-term pre-operative statin treatment. J. Am. Coll. Cardiol. 59, 60–70. doi: 10.1016/j.jacc.2011.08.062
Aras-López, R., Tovar, J., and Martínez, L. (2016). Possible role of increased oxidative stress in pulmonary hypertension in experimental diaphragmatic hernia. Pediatr. Surg. Int. 32, 141–145. doi: 10.1007/s00383-015-3826-5
Asci, A., Surmeli-Onay, O., Erkekoglu, P., Yigit, S., Yurdakok, M., and Kocer-Gumusel, B. (2015). Oxidant and antioxidant status in neonatal proven and clinical sepsis according to selenium status. Pediatr. Int. 57, 1131–1137. doi: 10.1111/ped.12698
Askie, L. M., Brocklehurst, P., Darlow, B. A., Finer, N., Schmidt, B., and Tarnow-Mordi, W. (2011). NeOProM: ne onatal O xygenation Pro spective M eta-analysis Collaboration study protocol. BMC Pediatr. 11:6. doi: 10.1186/1471-2431-11-6
Auten, R. L., and Davis, J. M. (2009). Oxygen toxicity and reactive oxygen species: the devil is in the details. Pediatr. Res. 66, 121–127. doi: 10.1203/PDR.0b013e3181a9eafb
Ayala, A., Muñoz, M. F., and Argüelles, S. (2014). Lipid peroxidation: production, metabolism, and signaling mechanisms of malondialdehyde and 4-hydroxy-2-nonenal. Oxid. Med. Cell. Longev. 2014:360438. doi: 10.1155/2014/360438
Babior, B. M. (2000). Phagocytes and oxidative stress. Am. J. Med. 109, 33–44. doi: 10.1016/S0002-9343(00)00481-2
Bahar, I., Elay, G., Başkol, G., Sungur, M., and Donmez-Altuntas, H. (2018). Increased DNA damage and increased apoptosis and necrosis in patients with severe sepsis and septic shock. J. Crit. Care 43, 271–275. doi: 10.1016/j.jcrc.2017.09.035
Bar-Or, D., Bar-Or, R., Rael, L. T., and Brody, E. N. (2015). Oxidative stress in severe acute illness. Redox Biol. 4, 340–345. doi: 10.1016/j.redox.2015.01.006
Barrera, G., Pizzimenti, S., Daga, M., Dianzani, C., Arcaro, A., Cetrangolo, G., et al. (2018). Lipid peroxidation-derived aldehydes, 4-hydroxynonenal and malondialdehyde in aging-related disorders. Antioxidants 7:E102. doi: 10.3390/antiox7080102
Bartlett, R. H., Gazzaniga, A. B., Jefferies, M. R., Huxtable, R. F., Haiduc, N., and Fong, S. (1976). Extracorporeal membrane oxygenation (ECMO) cardiopulmonary support in infancy. ASAIO J. 22, 80–92.
Batra, S., Kumar, R., Seema, Kapoor, A. K., and Ray, G. (2000). Alterations in antioxidant status during neonatal sepsis. Ann. Trop. Paediatr. 20, 27–33. doi: 10.1080/02724930092039
Bayr, H. (2005). Reactive oxygen species. Crit. Care Med. 33, S498–S501. doi: 10.1097/01.CCM.0000186787.64500.12
Begonja, A. J., Gambaryan, S., Geiger, J., Aktas, B., Pozgajova, M., Nieswandt, B., et al. (2005). Platelet NAD (P) H-oxidase–generated ROS production regulates αIIbβ3-integrin activation independent of the NO/cGMP pathway. Blood 106, 2757–2760. doi: 10.1182/blood-2005-03-1047
Bellezza, I., Giambanco, I., Minelli, A., and Donato, R. (2018). Nrf2-Keap1 signaling in oxidative and reductive stress. Biochim. Biophys. Acta Mol. Cell Res. 1865, 721–733. doi: 10.1016/j.bbamcr.2018.02.010
Berndt, C., Lillig, C. H., and Holmgren, A. (2007). Thiol-based mechanisms of the thioredoxin and glutaredoxin systems: implications for diseases in the cardiovascular system. Am. J. Physiol. Heart Circ. Physiol. 292, H1227–H1236. doi: 10.1152/ajpheart.01162.2006
Bertrand, Y., Pincemail, J., Hanique, G., Denis, B., Leenaerts, L., Vankeerberghen, L., et al. (1989). Differences in tocopherol-lipid ratios in ARDS and non-ARDS patients. Intensive Care Med. 15, 87–93. doi: 10.1007/BF00295983
Bhatnagar, S., Wadhwa, N., Aneja, S., Lodha, R., Kabra, S. K., Natchu, U. C. M., et al. (2012). Zinc as adjunct treatment in infants aged between 7 and 120 days with probable serious bacterial infection: a randomised, double-blind, placebo-controlled trial. Lancet 379, 2072–2078. doi: 10.1016/S0140-6736(12)60477-2
Billings, F. T., Ball, S. K., Roberts, Ii, L. J., and Pretorius, M. (2011). Postoperative acute kidney injury is associated with hemoglobinemia and an enhanced oxidative stress response. Free Radic. Biol. Med. 50, 1480–1487. doi: 10.1016/j.freeradbiomed.2011.02.011
Bocca, B., Ciccarelli, S., Agostino, R., and Alimonti, A. (2017). Trace elements, oxidative status and antioxidant capacity as biomarkers in very low birth weight infants. Environ. Res. 156, 705–713. doi: 10.1016/j.envres.2017.04.027
Bocci, V., Di, N. P., Borrelli, E., Larini, A., and Cappelletti, F. (2001). Ozonation of blood during extracorporeal circulation. II. Comparative analysis of several oxygenator-ozonators and selection of one type. Int. J. Artif. Organs 24, 890–897. doi: 10.1177/039139880102401202
Bocci, V., Di, N. P., Garosi, G., Aldinucci, C., Borrelli, E., Valacchi, G., et al. (1999). Ozonation of blood during extracorporeal circulation. I. Rationale, methodology and preliminary studies. Int. J. Artif. Organs 22, 645–651. doi: 10.1177/039139889902200910
Bocci, V., and Paolo, N. D. (2004). Oxygenation-ozonization of blood during extracorporeal circulation (EBOO). Part III: a new medical approach. Ozone Sci. Eng. 26, 195–205. doi: 10.1080/01919510490439564
Bogdan, C. (2015). Nitric oxide synthase in innate and adaptive immunity: an update. Trends Immunol. 36, 161–178. doi: 10.1016/j.it.2015.01.003
Borazan, A., Aydemir, S., Sert, M., and Yilmaz, A. (2004). The effects of hemodialysis and peritoneal dialysis on serum homocysteine and C-reactive protein levels. Mediators Inflamm. 13, 361–364. doi: 10.1080/09629350400008786
Börgermann, J., Scheubel, R., Simm, A., Silber, R., and Friedrich, I. (2007). Inflammatory response in on-versus off-pump myocardial revascularization: is ECC really the culprit? Thorac. Cardiovasc. Surg. 55, 473–480. doi: 10.1055/s-2007-965631
Boyle, E. M., Pohlman, T. H., Johnson, M. C., and Verrier, E. D. (1997). Endothelial cell injury in cardiovascular surgery: the systemic inflammatory response1. Ann. Thorac. Surg. 63, 277–284.
Brand, M. D. (2010). The sites and topology of mitochondrial superoxide production. Exp. Gerontol. 45, 466–472. doi: 10.1016/j.exger.2010.01.003
Brealey, D., Brand, M., Hargreaves, I., Heales, S., Land, J., Smolenski, R., et al. (2002). Association between mitochondrial dysfunction and severity and outcome of septic shock. Lancet 360, 219–223. doi: 10.1016/S0140-6736(02)09459-X
Brion, L. P., Bell, E. F., and Raghuveer, T. S. (2003). Vitamin E supplementation for prevention of morbidity and mortality in preterm infants. Cochrane Database Syst. Rev. 4:CD003665. doi: 10.1002/14651858.CD003665
Briones, A. M., and Touyz, R. M. (2010). Oxidative stress and hypertension: current concepts. Curr. Hypertens. Rep. 12, 135–142. doi: 10.1007/s11906-010-0100-z
Brix-Christensen, V., Petersen, T., Ravn, H. B., Hjortdal, V. E., Andersen, N. T., and Tønnesen, E. (2001). Cardiopulmonary bypass elicits a pro-and anti-inflammatory cytokine response and impaired neutrophil chemotaxis in neonatal pigs. Acta Anaesthesiol. Scand. 45, 407–413. doi: 10.1034/j.1399-6576.2001.045004407.x
Brix-Christensen, V., Tønnesen, E., Hjortdal, V. E., Chew, M., Flø, C., Marqversen, J., et al. (2002). Neutrophils and platelets accumulate in the heart, lungs, and kidneys after cardiopulmonary bypass in neonatal pigs. Crit. Care Med. 30, 670–676. doi: 10.1097/00003246-200203000-00029
Brogan, T., Laurance Lequier, M. D., Lorusso, R., Maclaren, G., and Peek, G. (eds). (2017). Extracorporeal Life Support: the ELSO Red Book, 5 th Edn. Ann Arbor, MI: ELSO.
Broman, M., Lindfors, M., Norberg,Å., Hebert, C., Rooyackers, O., Wernerman, J., et al. (2018). Low serum selenium is associated with the severity of organ failure in critically ill children. Clin. Nutr. 37, 1399–1405. doi: 10.1016/j.clnu.2017.06.014
Bulua, A. C., Simon, A., Maddipati, R., Pelletier, M., Park, H., Kim, K.-Y., et al. (2011). Mitochondrial reactive oxygen species promote production of proinflammatory cytokines and are elevated in TNFR1-associated periodic syndrome (TRAPS). J. Exp. Med. 208, 519–533. doi: 10.1084/jem.20102049
Butch, S., Knafl, P., Oberman, H., and Bartlett, R. (1996). Blood utilization in adult patients undergoing extracorporeal membrane oxygenated therapy. Transfusion 36, 61–63. doi: 10.1046/j.1537-2995.1996.36196190517.x
Butterfield, D. A., Drake, J., Pocernich, C., and Castegna, A. (2001). Evidence of oxidative damage in Alzheimer’s disease brain: central role for amyloid β-peptide. Trends Mol. Med. 7, 548–554. doi: 10.1016/S1471-4914(01)02173-6
Caputo, M., Bays, S., Rogers, C., Pawade, A., Parry, A., and Suleiman, M. (2005). The effects of normothermic and hypothermic cardiopulmonary bypass on myocardial injury, oxidative stress, and inflammatory response in paediatric open-heart surgery: a prospective randomized study. Ann. Thorac. Surg. 80, 982–988. doi: 10.1016/j.athoracsur.2005.03.062
Caputo, M., Mokhtari, A., Rogers, C. A., Panayiotou, N., Chen, Q., Ghorbel, M. T., et al. (2009). The effects of normoxic versus hyperoxic cardiopulmonary bypass on oxidative stress and inflammatory response in cyanotic pediatric patients undergoing open cardiac surgery: a randomized controlled trial. J. Thorac. Cardiovasc. Surg. 138, 206–214. doi: 10.1016/j.jtcvs.2008.12.028
Carnes, C. A., Chung, M. K., Nakayama, T., Nakayama, H., Baliga, R. S., Piao, S., et al. (2001). Ascorbate attenuates atrial pacing-induced peroxynitrite formation and electrical remodeling and decreases the incidence of postoperative atrial fibrillation. Circ. Res. 89, e32–e38. doi: 10.1161/hh1801.097644
Carpenter, C. T., Price, P. V., and Christman, B. W. (1998). Exhaled breath condensate isoprostanes are elevated in patients with acute lung injury or ARDS. Chest 114, 1653–1659. doi: 10.1378/chest.114.6.1653
Cashen, K., Reeder, R., Dalton, H. J., Berg, R. A., Shanley, T. P., Newth, C. J., et al. (2018). Hyperoxia and hypocapnia during pediatric extracorporeal membrane oxygenation: associations with complications, mortality, and functional status among survivors. Pediatr. Crit. Care Med. 19, 245–253. doi: 10.1097/PCC.0000000000001439
Castegna, A., Aksenov, M., Thongboonkerd, V., Klein, J. B., Pierce, W. M., Booze, R., et al. (2002). Proteomic identification of oxidatively modified proteins in Alzheimer’s disease brain. Part II: dihydropyrimidinase-related protein 2, α-enolase and heat shock cognate 71. J. Neurochem. 82, 1524–1532. doi: 10.1046/j.1471-4159.2002.01103.x
Castellheim, A., Pharo, A., Fung, M., Saugstad, O. D., and Mollnes, T. E. (2005). Complement C5a is a key mediator of meconium-induced neutrophil activation. Pediatr. Res. 57, 242–247. doi: 10.1203/01.PDR.0000150725.78971.30
Cavalca, V., Sisillo, E., Veglia, F., Tremoli, E., Cighetti, G., Salvi, L., et al. (2006). Isoprostanes and oxidative stress in off-pump and on-pump coronary bypass surgery. Ann. Thorac. Surg. 81, 562–567. doi: 10.1016/j.athoracsur.2005.08.019
Cavarocchi, N. C., England, M. D., Schaff, H. V., Russo, P., Orszulak, T. A., Schnell, W. Jr., et al. (1986). Oxygen free radical generation during cardiopulmonary bypass: correlation with complement activation. Circulation 74, III130–III133.
Chaturvedi, R., Macrae, D., Brown, K., Schindler, M., Smith, E., Davis, K., et al. (2004). Cardiac ECMO for biventricular hearts after paediatric open heart surgery. Heart 90, 545–551. doi: 10.1136/hrt.2002.003509
Chaudhary, P., Sharma, R., Sharma, A., Vatsyayan, R., Yadav, S., Singhal, S. S., et al. (2010). Mechanisms of 4-hydroxy-2-nonenal induced pro-and anti-apoptotic signaling. Biochemistry 49, 6263–6275. doi: 10.1021/bi100517x
Chen, G. Y., and Nuñez, G. (2010). Sterile inflammation: sensing and reacting to damage. Nat. Rev. Immunol. 10, 826–837. doi: 10.1038/nri2873
Chen, H., Song, Y. S., and Chan, P. H. (2009). Inhibition of NADPH oxidase is neuroprotective after ischemia—reperfusion. J. Cereb. Blood Flow Metab. 29, 1262–1272. doi: 10.1038/jcbfm.2009.47
Chen, Y., Azad, M., and Gibson, S. (2009). Superoxide is the major reactive oxygen species regulating autophagy. Cell Death Differ. 16, 1040–1052. doi: 10.1038/cdd.2009.49
Chen, H., Yu, R.-G., Yin, N.-N., and Zhou, J.-X. (2014). Combination of extracorporeal membrane oxygenation and continuous renal replacement therapy in critically ill patients: a systematic review. Crit. Care 18:675. doi: 10.1186/s13054-014-0675-x
Chen, Q., Yu, W., Shi, J., Shen, J., Hu, Y., Gong, J., et al. (2014). The effect of extracorporeal membrane oxygenation therapy on systemic oxidative stress injury in a porcine model. Artif. Organs 38, 426–431. doi: 10.1111/aor.12198
Cheng, H. H., Wypij, D., Laussen, P. C., Bellinger, D. C., Stopp, C. D., Soul, J. S., et al. (2014). Cerebral blood flow velocity and neurodevelopmental outcome in infants undergoing surgery for congenital heart disease. Ann. Thorac. Surg. 98, 125–132. doi: 10.1016/j.athoracsur.2014.03.035
Chludzińska, L., Ananicz, E., Jarosławska, A., and Komorowska, M. (2005). Near-infrared radiation protects the red cell membrane against oxidation. Blood Cells Mol. Dis. 35, 74–79. doi: 10.1016/j.bcmd.2005.04.003
Choi, D. K., Koppula, S., Choi, M., and Suk, K. (2010). Recent developments in the inhibitors of neuroinflammation and neurodegeneration: inflammatory oxidative enzymes as a drug target. Expert Opin. Ther. Pat. 20, 1531–1546. doi: 10.1517/13543776.2010.525220
Chow, C.-W., Herrera Abreu, M. T., Suzuki, T., and Downey, G. P. (2003). Oxidative stress and acute lung injury. Am. J. Respir. Cell Mol. Biol. 29, 427–431. doi: 10.1165/rcmb.F278
Christie, N. A., Smith, D. E., Decampos, K. N., Slutsky, A. S., Patterson, G. A., and Tanswell, A. K. (1994). Lung oxidant injury in a model of lung storage and extended reperfusion. Am. J. Respir. Crit. Care Med. 150, 1032–1037. doi: 10.1164/ajrccm.150.4.7921433
Ciapetti, M., Mancinelli, P., Cecchi, A., Borrelli, E., Bocci, V., and Peris, A. (2018). Reduction of non-enzymatic antioxidants in plasma during ECMO-treatment in ARDS by influence A H1N1. J. Crit. Care 43, 220–224. doi: 10.1016/j.jcrc.2017.08.005
Cipak Gasparovic, A., Zarkovic, N., Zarkovic, K., Semen, K., Kaminskyy, D., Yelisyeyeva, O., et al. (2017). Biomarkers of oxidative and nitro-oxidative stress: conventional and novel approaches. Br. J. Pharmacol. 174, 1771–1783. doi: 10.1111/bph.13673
Clermont, G., Vergely, C., Jazayeri, S., Lahet, J.-J., Goudeau, J.-J., Lecour, S., et al. (2002). Systemic free radical activation is a major event involved in myocardial oxidative stress related to cardiopulmonary bypass. Anesthesiology 96, 80–87. doi: 10.1097/00000542-200201000-00019
Collard, K., Godeck, S., Holley, J., and Quinn, M. (2004). Pulmonary antioxidant concentrations and oxidative damage in ventilated premature babies. Arch. Dis. Child. Fetal Neonatal Ed. 89, F412–F416. doi: 10.1136/adc.2002.016717
Collard, K. J., Godeck, S., and Holley, J. E. (2005). Blood transfusion and pulmonary lipid peroxidation in ventilated premature babies. Pediatr. Pulmonol. 39, 257–261. doi: 10.1002/ppul.20190
Comporti, M., Signorini, C., Leoncini, S., Buonocore, G., Rossi, V., and Ciccoli, L. (2004). Plasma F2-isoprostanes are elevated in newborns and inversely correlated to gestational age. Free Radic. Biol. Med. 37, 724–732. doi: 10.1016/j.freeradbiomed.2004.06.007
Cooke, M. S., Evans, M. D., Dizdaroglu, M., and Lunec, J. (2003). Oxidative DNA damage: mechanisms, mutation, and disease. FASEB J. 17, 1195–1214. doi: 10.1096/fj.02-0752rev
Coombes, J. S., and Fassett, R. G. (2012). Antioxidant therapy in hemodialysis patients: a systematic review. Kidney Int. 81, 233–246. doi: 10.1038/ki.2011.341
Couroucli, X. I. (2017). Oxidative stress in the retina: implications for Retinopathy of Prematurity. Curr. Opin. Toxicol. 7, 102–109. doi: 10.1016/j.cotox.2017.11.008
Cowley, H. C., Bacon, P. J., Goode, H. F., Webster, N. R., Jones, J. G., and Menon, D. K. (1996). Plasma antioxidant potential in severe sepsis: a comparison of survivors and nonsurvivors. Crit. Care Med. 24, 1179–1183. doi: 10.1097/00003246-199607000-00019
Crimi, E., Sica, V., Williams-Ignarro, S., Zhang, H., Slutsky, A. S., Ignarro, L. J., et al. (2006). The role of oxidative stress in adult critical care. Free Radic. Biol. Med. 40, 398–406. doi: 10.1016/j.freeradbiomed.2005.10.054
Cruz, C. M., Rinna, A., Forman, H. J., Ventura, A. L., Persechini, P. M., and Ojcius, D. M. (2007). ATP activates a reactive oxygen species-dependent oxidative stress response and secretion of proinflammatory cytokines in macrophages. J. Biol. Chem. 282, 2871–2879. doi: 10.1074/jbc.M608083200
Cruz, M. A., Yee, A., and Valladolid, C. (2018). von Willebrand factor, free hemoglobin and thrombosis in ECMO. Front. Med. 5:228. doi: 10.3389/fmed.2018.00228
Da, Q., Teruya, M., Guchhait, P., Teruya, J., Olson, J. S., and Cruz, M. A. (2015). Free hemoglobin increases von Willebrand factor-mediated platelet adhesion in vitro: implications on circulatory devices. Blood 126, 2338–2341. doi: 10.1182/blood-2015-05-648030
Dalle-Donne, I., Aldini, G., Carini, M., Colombo, R., Rossi, R., and Milzani, A. (2006). Protein carbonylation, cellular dysfunction, and disease progression. J. Cell. Mol. Med. 10, 389–406. doi: 10.1111/j.1582-4934.2006.tb00407.x
Dalle-Donne, I., Giustarini, D., Colombo, R., Rossi, R., and Milzani, A. (2003a). Protein carbonylation in human diseases. Trends Mol. Med. 9, 169–176. doi: 10.1016/S1471-4914(03)00031-5
Dalle-Donne, I., Rossi, R., Giustarini, D., Milzani, A., and Colombo, R. (2003b). Protein carbonyl groups as biomarkers of oxidative stress. Clin. Chim. Acta 329, 23–38.
Dalton, H. J., Cashen, K., Reeder, R. W., Berg, R. A., Shanley, T. P., Newth, C. J., et al. (2018). Hemolysis during pediatric extracorporeal membrane oxygenation: associations with circuitry, complications, and mortality. Pediatr. Crit. Care Med. 19, 1067–1076. doi: 10.1097/PCC.0000000000001709
Dani, C., Reali, M., Bertini, G., Martelli, E., Pezzati, M., and Rubaltelli, F. (2001). The role of blood transfusions and iron intake on retinopathy of prematurity. Early Hum. Dev. 62, 57–63. doi: 10.1016/S0378-3782(01)00115-3
Darlow, B. A., and Austin, N. (2003). Selenium supplementation to prevent short-term morbidity in preterm neonates. Cochrane Database Syst. Rev. 4:CD003312. doi: 10.1002/14651858.CD003312
Darlow, B. A., Graham, P., and Rojas-Reyes, M. X. (2016). Vitamin A supplementation to prevent mortality and short-and long-term morbidity in very low birth weight infants. Cochrane Database Syst. Rev. 8:CD000501. doi: 10.1002/14651858.CD000501.pub4
Davie, E. W., Fujikawa, K., and Kisiel, W. (1991). The coagulation cascade: initiation, maintenance, and regulation. Biochemistry 30, 10363–10370. doi: 10.1021/bi00107a001
Davies, M., and Hagen, P. O. (1997). Systemic inflammatory response syndrome. Br. J. Surg. 84, 920–935. doi: 10.1002/bjs.1800840707
Davis, C. L., Kausz, A. T., Zager, R. A., Kharasch, E. D., and Cochran, R. P. (1999). Acute renal failure after cardiopulmonary bypass is related to decreased serum ferritin levels. J. Am. Soc. Nephrol. 10, 2396–2402.
Demling, R. H., La Londe, C., Daryani, R., Zhu, D., Knox, J., and Youn, Y.-K. (1991). Relationship between the lung and systemic response to endotoxin: comparison of physiologic change and the degree of lipid peroxidation. Circ. Shock 34, 364–370.
Depuydt, L. E., Schuit, K. E., and Smith, S. D. (1993). Effect of extracorporeal membrane oxygenation on neutrophil function in neonates. Crit. Care Med. 21, 1324–1327. doi: 10.1097/00003246-199309000-00015
Desborough, J. (2000). The stress response to trauma and surgery. Br. J. Anaesth. 85, 109–117. doi: 10.1093/bja/85.1.109
Di Paolo, N., Bocci, V., Salvo, D., Palasciano, F., Biagioli, M., Meini, S., et al. (2005). Extracorporeal Blood Oxygenation and Ozonation (EBOO): a Controlled Trial in Patients with Peripheral Artery Disease. London: SAGE Publications.
Dialynas, G., Shrestha, O. K., Ponce, J. M., Zwerger, M., Thiemann, D. A., Young, G. H., et al. (2015). Myopathic lamin mutations cause reductive stress and activate the nrf2/keap-1 pathway. PLoS Genet. 11:e1005231. doi: 10.1371/journal.pgen.1005231
Dimon-Gadal, S., Gerbaud, P., Guibourdenche, J., Evain-Brion, D., Raynaud, F., Thérond, P., et al. (2000). Increased oxidative damage to fibroblasts in skin with and without lesions in psoriasis. J. Investig. Dermatol. 114, 984–989. doi: 10.1046/j.1523-1747.2000.00962.x
Dizdaroglu, M., and Jaruga, P. (2012). Mechanisms of free radical-induced damage to DNA. Free Radic. Res. 46, 382–419. doi: 10.3109/10715762.2011.653969
Djordjevic, T., Pogrebniak, A., Belaiba, R. S., Bonello, S., Wotzlaw, C., Acker, H., et al. (2005). The expression of the NADPH oxidase subunit p22phox is regulated by a redox-sensitive pathway in endothelial cells. Free Radic. Biol. Med. 38, 616–630. doi: 10.1016/j.freeradbiomed.2004.09.036
Dos Santos, A. M. N., Guinsburg, R., De Almeida, M. F. B., Procianoy, R. S., Leone, C. R., Marba, S. T. M., et al. (2011). Red blood cell transfusions are independently associated with intra-hospital mortality in very low birth weight preterm infants. J. Pediatr. 159, 371.–376.
Descamps-Latscha, B., Drüeke, T., and Witko-sarsat, V. (2001). Dialysis-induced oxidative stress: biological aspects, clinical consequences, and therapy. Semin. Dial. 14, 193–199. doi: 10.1046/j.1525-139X.2001.00052.x
Duncan, B. W., Hraska, V., Jonas, R. A., Wessel, D. L., Del Nido, P. J., Laussen, P. C., et al. (1999). Mechanical circulatory support in children with cardiac disease. J. Thorac. Cardiovasc. Surg. 117, 529–542. doi: 10.1016/S0022-5223(99)70333-8
Edmunds, L. H. Jr. (1998). Inflammatory response to cardiopulmonary bypass. Ann. Thorac. Surg. 66, S12–S16. doi: 10.1016/S0003-4975(98)00967-9
Egginton, S. (2009). Invited review: activity-induced angiogenesis. Pflügers Arch. 457, 963–977. doi: 10.1007/s00424-008-0563-9
Eiselt, J., Racek, J., Trefil, L., and Opatrný, K. (2001). Effects of a vitamin E-modified dialysis membrane and vitamin C infusion on oxidative stress in hemodialysis patients. Artif. Organs 25, 430–436. doi: 10.1046/j.1525-1594.2001.025006430.x
Engel, J., Pitz, S., Mühling, J., Menges, T., Martens, F., Kwapisz, M., et al. (2009). Role of glutamine administration on T-cell derived inflammatory response after cardiopulmonary bypass. Clin. Nutr. 28, 15–20. doi: 10.1016/j.clnu.2008.08.007
Ercan, S., Çakmak, A., Kösecik, M., and Erel, Ö (2009). The oxidative state of children with cyanotic and acyanotic congenital heart disease. Anadolu Kardiyol. Derg. 9, 486–490.
Erikssen, G., Liestøl, K., Seem, E., Birkeland, S., Saatvedt, K. J., Hoel, T. N., et al. (2015). Achievements in congenital heart defect surgery: a prospective, 40-year study of 7038 patients. Circulation 131, 337–346. doi: 10.1161/CIRCULATIONAHA.114.012033
Fall, L., New, K. J., Evans, K. A., and Bailey, D. M. (2015). Arterial hypoxaemia and its impact on coagulation: significance of altered redox homeostasis. J. Clin. Pathol. 68, 752–754. doi: 10.1136/jclinpath-2015-202952
Finer, N., and Leone, T. (2009). Oxygen saturation monitoring for the preterm infant: the evidence basis for current practice. Pediatr. Res. 65, 375–380. doi: 10.1203/PDR.0b013e318199386a
Finkel, T. (2012). From sulfenylation to sulfhydration: what a thiolate needs to tolerate. Sci. Signal. 5:e10. doi: 10.1126/scisignal.2002943
Fiser, R. T., Irby, K., Ward, R. M., Tang, X., Mckamie, W., Prodhan, P., et al. (2014). RBC transfusion in pediatric patients supported with extracorporeal membrane oxygenation: is there an impact on tissue oxygenation? Pediatr. Crit. Care Med. 15, 806–813. doi: 10.1097/PCC.0000000000000222
Fläring, U., Rooyackers, O., Hebert, C., Bratel, T., Hammarqvist, F., and Wernerman, J. (2005). Temporal changes in whole-blood and plasma glutathione in ICU patients with multiple organ failure. Intensive Care Med. 31, 1072–1078. doi: 10.1007/s00134-005-2687-0
Floor, E., and Wetzel, M. G. (1998). Increased protein oxidation in human substantia nigra pars compacta in comparison with basal ganglia and prefrontal cortex measured with an improved dinitrophenylhydrazine assay. J. Neurochem. 70, 268–275. doi: 10.1046/j.1471-4159.1998.70010268.x
Fortenberry, J. D., Bhardwaj, V., Niemer, P., Cornish, J. D., Wright, J. A., and Bland, L. (1996). Neutrophil and cytokine activation with neonatal extracorporeal membrane oxygenation. J. Pediatr. 128, 670–678. doi: 10.1016/S0022-3476(96)80133-8
Fridovich, I. (1997). Superoxide anion radical (), superoxide dismutases, and related matters. J. Biol. Chem. 272, 18515–18517. doi: 10.1074/jbc.272.30.18515
Fudulu, D., and Angelini, G. (2016). Oxidative stress after surgery on the immature heart. Oxid. Med. Cell. Longev. 2016:1971452. doi: 10.1155/2016/1971452
Gadepalli, S. K., Selewski, D. T., Drongowski, R. A., and Mychaliska, G. B. (2011). Acute kidney injury in congenital diaphragmatic hernia requiring extracorporeal life support: an insidious problem. J. Pediatr. Surg. 46, 630–635. doi: 10.1016/j.jpedsurg.2010.11.031
Galley, H. F. (2011). Oxidative stress and mitochondrial dysfunction in sepsis. Br. J. Anaesth. 107, 57–64. doi: 10.1093/bja/aer093
Gessler, P., Pretre, R., Hohl, V., Rousson, V., Fischer, J., and Dahinden, C. (2004). CXC-chemokine stimulation of neutrophils correlates with plasma levels of myeloperoxidase and lactoferrin and contributes to clinical outcome after pediatric cardiac surgery. Shock 22, 513–520. doi: 10.1097/01.shk.0000145939.54838.51
Ghirardello, S., Dusi, E., Cortinovis, I., Villa, S., Fumagalli, M., Agosti, M., et al. (2017). Effects of red blood cell transfusions on the risk of developing complications or death: an observational study of a cohort of very low birth weight infants. Am. J. Perinatol. 34, 88–95. doi: 10.1055/s-0036-1584300
Giacco, F., and Brownlee, M. (2010). Oxidative stress and diabetic complications. Circ. Res. 107, 1058–1070. doi: 10.1161/CIRCRESAHA.110.223545
Gilboa, S. M., Salemi, J. L., Nembhard, W. N., Fixler, D. E., and Correa, A. (2010). Mortality resulting from congenital heart disease among children and adults in the United States, 1999 to 2006. Circulation 122, 2254–2263. doi: 10.1161/CIRCULATIONAHA.110.947002
Gitto, E., Karbownik, M., Reiter, R. J., Tan, D. X., Cuzzocrea, S., Chiurazzi, P., et al. (2001). Effects of melatonin treatment in septic newborns. Pediatr. Res. 50, 756–760. doi: 10.1203/00006450-200112000-00021
Gitto, E., Pellegrino, S., D’arrigo, S., Barberi, I., and Reiter, R. (2009). Oxidative stress in resuscitation and in ventilation of newborns. Eur. Respir. J. 34, 1461–1469. doi: 10.1183/09031936.00032809
Gitto, E., Reiter, R. J., Cordaro, S. P., La Rosa, M., Chiurazzi, P., Trimarchi, G., et al. (2004). Oxidative and inflammatory parameters in respiratory distress syndrome of preterm newborns: beneficial effects of melatonin. Am. J. Perinatol. 21, 209–216. doi: 10.1055/s-2004-828610
Giustarini, D., Dalle-Donne, I., Tsikas, D., and Rossi, R. (2009). Oxidative stress and human diseases: origin, link, measurement, mechanisms, and biomarkers. Crit. Rev. Clin. Lab. Sci. 46, 241–281. doi: 10.3109/10408360903142326
Gladstone, I. M., and Levine, R. L. (1994). Oxidation of proteins in neonatal lungs. Pediatrics 93, 764–768.
Golej, J., Winter, P., Schöffmann, G., Kahlbacher, H., Stoll, E., Boigner, H., et al. (2003). Impact of extracorporeal membrane oxygenation modality on cytokine release during rescue from infant hypoxia. Shock 20, 110–115. doi: 10.1097/01.shk.0000075571.93053.2c
Goode, H. F., Cowley, H. C., Walker, B. E., Howdle, P. D., and Webster, N. R. (1995). Decreased antioxidant status and increased lipid peroxidation in patients with septic shock and secondary organ dysfunction. Crit. Care Med. 23, 646–651. doi: 10.1097/00003246-199504000-00011
Goodyear-Bruch, C., and Pierce, J. D. (2002). Oxidative stress in critically ill patients. Am. J. Crit. Care 11, 543–551.
Gopinathan, V., Miller, N. J., Milner, A. D., and Rice-Evans, C. A. (1994). Bilirubin and ascorbate antioxidant activity in neonatal plasma. FEBS Lett. 349, 197–200. doi: 10.1016/0014-5793(94)00666-0
Görlach, A. (2005). Redox regulation of the coagulation cascade. Antioxid. Redox Signal. 7, 1398–1404. doi: 10.1089/ars.2005.7.1398
Görlach, A., Kietzmann, T., and Hess, J. (2002). Redox signaling through NADPH oxidases: involvement in vascular proliferation and coagulation. Ann. N. Y. Acad. Sci. 973, 505–507. doi: 10.1111/j.1749-6632.2002.tb04691.x
Gotts, J. E., and Matthay, M. A. (2011). Mesenchymal stem cells and acute lung injury. Crit. Care Clin. 27, 719–733. doi: 10.1016/j.ccc.2011.04.004
Graulich, J., Sonntag, J., Marcinkowski, M., Bauer, K., Kössel, H., Bührer, C., et al. (2002). Complement activation by in vivo neonatal and in vitro extracorporeal membrane oxygenation. Mediators Inflamm. 11, 69–73. doi: 10.1080/09629350220131908
Guerreiro, M. O., Petronilho, F., Andrades, M., Constantino, L., Mina, F. G., Moreira, J. C. F., et al. (2010). Plasma superoxide dismutase activity and mortality in patients with septic. J. Trauma Acute Care Surg. 69, E102–E106. doi: 10.1097/TA.0b013e3181dbb289
Gupta, N., Su, X., Popov, B., Lee, J. W., Serikov, V., and Matthay, M. A. (2007). Intrapulmonary delivery of bone marrow-derived mesenchymal stem cells improves survival and attenuates endotoxin-induced acute lung injury in mice. J. Immunol. 179, 1855–1863. doi: 10.4049/jimmunol.179.3.1855
Gutteridge, J. M., and Halliwell, B. (2018). Mini-Review: oxidative stress, redox stress or redox success? Biochem. Biophys. Res. Commun. 502, 183–186. doi: 10.1016/j.bbrc.2018.05.045
Haidara, M. A., Yassin, H. Z., Rateb, M., Ammar, H., and Zorkani, M. A. (2006). Role of oxidative stress in development of cardiovascular complications in diabetes mellitus. Curr. Vasc. Pharmacol. 4, 215–227. doi: 10.2174/157016106777698469
Halliwell, B., and Gutteridge, J. M. (2015). Free Radicals in Biology and Medicine. New York, NY: Oxford University Press. doi: 10.1093/acprof:oso/9780198717478.001.0001
Hammond, B., and Hess, M. L. (1985). The oxygen free radical system: potential mediator of myocardial injury. J. Am. Coll. Cardiol. 6, 215–220. doi: 10.1016/S0735-1097(85)80278-3
Hasselwander, O., and Young, I. S. (1998). Oxidative stress in chronic renal failure. Free Radic. Res. 29, 1–11. doi: 10.1080/10715769800300011
Hayes, R., Shekar, K., and Fraser, J. (2013). Is hyperoxaemia helping or hurting patients during extracorporeal membrane oxygenation? Review of a complex problem. Perfusion 28, 184–193. doi: 10.1177/0267659112473172
He, C., Yang, S., Yu, W., Chen, Q., Shen, J., Hu, Y., et al. (2014). Effects of continuous renal replacement therapy on intestinal mucosal barrier function during extracorporeal membrane oxygenation in a porcine model. J. Cardiothorac. Surg. 9:72. doi: 10.1186/1749-8090-9-72
Hegbrant, J., and Hultkvist, B. U. (1999). Vitamin C and E as antioxidants in hemodialysis patients. Int. J. Artif. Organs 22, 69–73. doi: 10.1177/039139889902200203
Heiss, K. F., Pettit, B., Hirschl, R. B., Cilley, R. E., Chapman, R., and Bartlett, R. H. (1987). Renal insufficiency and volume overload in neonatal ECMO managed by continuous ultrafiltration. ASAIO J. 33, 557–560.
Herkert, O., Djordjevic, T., Belaiba, R. S., and Görlach, A. (2004). Insights into the redox control of blood coagulation: role of vascular NADPH oxidase-derived reactive oxygen species in the thrombogenic cycle. Antioxid. Redox Signal. 6, 765–776. doi: 10.1089/1523086041361695
Hirthler, M., Simoni, J., and Dickson, M. (1992). Elevated levels of endotoxin, oxygen-derived free radicals, and cytokines during extracorporeal membrane oxygenation. J. Pediatr. Surg. 27, 1199–1202. doi: 10.1016/0022-3468(92)90787-8
Hocker, J. R., Wellhausen, S. R., Ward, R. A., Simpson, P. M., and Cook, L. N. (1991). Effect of extracorporeal membrane oxygenation on leukocyte function in neonates. Artif. Organs 15, 23–28. doi: 10.1111/j.1525-1594.1991.tb00755.x
Hoffmann, F. W., Hashimoto, A. S., Lee, B. C., Rose, A. H., Shohet, R. V., and Hoffmann, P. R. (2011). Specific antioxidant selenoproteins are induced in the heart during hypertrophy. Arch. Biochem. Biophys. 512, 38–44. doi: 10.1016/j.abb.2011.05.007
Hoffmann, P. R., and Berry, M. J. (2005). Selenoprotein synthesis: a unique translational mechanism used by a diverse family of proteins. Thyroid 15, 769–775. doi: 10.1089/thy.2005.15.769
Horton, J. W., and Walker, P. B. (1993). Oxygen radicals, lipid peroxidation, and permeability changes after intestinal ischemia and reperfusion. J. Appl. Physiol. 74, 1515–1520. doi: 10.1152/jappl.1993.74.4.1515
Hövels-Gürich, H. H., Vazquez-Jimenez, J. F., Silvestri, A., Schumacher, K., Minkenberg, R., Duchateau, J., et al. (2002). Production of proinflammatory cytokines and myocardial dysfunction after arterial switch operation in neonates with transposition of the great arteries. J. Thorac. Cardiovasc. Surg. 124, 811–820. doi: 10.1067/mtc.2002.122308
Huet, O., Obata, R., Aubron, C., Spraul-Davit, A., Charpentier, J., Laplace, C., et al. (2007). Plasma-induced endothelial oxidative stress is related to the severity of septic shock. Crit. Care Med. 35, 821–826. doi: 10.1097/01.CCM.0000257464.79067.AF
Itoh, T., Murakami, H., Orihashi, K., Sueda, T., Kusumoto, Y., Kakehashi, M., et al. (2000). Low power laser protects human erythrocytes in an in vitro model of artificial heart-lung machines. Artif. Organs 24, 870–873. doi: 10.1046/j.1525-1594.2000.06624.x
Iwasaki, A., and Medzhitov, R. (2010). Regulation of adaptive immunity by the innate immune system. Science 327, 291–295. doi: 10.1126/science.1183021
Jialiang, S., Juanhong, S., Qiyi, C., Wenkui, Y., Changsheng, H., Yimin, H., et al. (2014). In-line hemofiltration minimized extracorporeal membrane oxygenation-related inflammation in a porcine model. Perfusion 29, 526–533. doi: 10.1177/0267659114529320
Kahles, T., Luedike, P., Endres, M., Galla, H.-J., Steinmetz, H., Busse, R., et al. (2007). NADPH oxidase plays a central role in blood-brain barrier damage in experimental stroke. Stroke 38, 3000–3006. doi: 10.1161/STROKEAHA.107.489765
Kameneva, M. V., Undar, A., Antaki, J. F., Watach, M. J., Calhoon, J. H., and Borovetz, H. S. (1999). Decrease in red blood cell deformability caused by hypothermia, hemodilution, and mechanical stress: factors related to cardiopulmonary bypass. ASAIO J. 45, 307–310. doi: 10.1097/00002480-199907000-00010
Kaplan, M., Bromiker, R., and Hammerman, C. (2014). Hyperbilirubinemia, hemolysis, and increased bilirubin neurotoxicity. Semin. Perinatol. 38, 429–437. doi: 10.1053/j.semperi.2014.08.006
Kaplanski, G., Fabrigoule, M., Boulay, V., Dinarello, C. A., Bongrand, P., Kaplanski, S., et al. (1997). Thrombin induces endothelial type II activation in vitro: IL-1 and TNF-alpha-independent IL-8 secretion and E-selectin expression. J. Immunol. 158, 5435–5441.
Karu, I., Taal, G., Zilmer, K., Pruunsild, C., Starkopf, J., and Zilmer, M. (2010). Inflammatory/oxidative stress during the first week after different types of cardiac surgery. Scand. Cardiovasc. J. 44, 119–124. doi: 10.3109/14017430903490981
Kato, S., Hussein, M. H., Kakita, H., Goto, T., Daoud, G. A., Kato, T., et al. (2009). Edaravone, a novel free radical scavenger, reduces high-mobility group box 1 and prolongs survival in a neonatal sepsis model. Shock 32, 586–592. doi: 10.1097/SHK.0b013e3181a2b886
Khaper, N., Bryan, S., Dhingra, S., Singal, R., Bajaj, A., Pathak, C. M., et al. (2010). Targeting the vicious inflammation–oxidative stress cycle for the management of heart failure. Antioxid. Redox Signal. 13, 1033–1049. doi: 10.1089/ars.2009.2930
Kim, H.-Y., and Gladyshev, V. N. (2004). Methionine sulfoxide reduction in mammals: characterization of methionine-R-sulfoxide reductases. Mol. Biol. Cell 15, 1055–1064. doi: 10.1091/mbc.e03-08-0629
Kim, Y. M., Kattach, H., Ratnatunga, C., Pillai, R., Channon, K. M., and Casadei, B. (2008). Association of atrial nicotinamide adenine dinucleotide phosphate oxidase activity with the development of atrial fibrillation after cardiac surgery. J. Am. Coll. Cardiol. 51, 68–74. doi: 10.1016/j.jacc.2007.07.085
Kirkham, P., and Rahman, I. (2006). Oxidative stress in asthma and COPD: antioxidants as a therapeutic strategy. Pharmacol. Ther. 111, 476–494. doi: 10.1016/j.pharmthera.2005.10.015
Klaunig, and Je, W. Z. (2018). Oxidative stress in carcinogenesis. Curr. Opin. Toxicol. 7, 116–121. doi: 10.1016/j.cotox.2017.11.014
Koch, C. G., Li, L., Sessler, D. I., Figueroa, P., Hoeltge, G. A., Mihaljevic, T., et al. (2008). Duration of red-cell storage and complications after cardiac surgery. N. Engl. J. Med. 358, 1229–1239. doi: 10.1056/NEJMoa070403
Komorowska, M., Cuissot, A., Czarnoleski, A., and Bialas, W. (2001). Erythrocyte response to near infrared radiation. Cell. Mol. Biol. Lett. 6, 212–212.
Kotani, N., Hashimoto, H., Sessler, D. I., Muraoka, M., Wang, J.-S., O’connor, M. F., et al. (2000). Neutrophil number and interleukin-8 and elastase concentrations in bronchoalveolar lavage fluid correlate with decreased arterial oxygenation after cardiopulmonary bypass. Anesth. Analg. 90, 1046–1051. doi: 10.1097/00000539-200005000-00009
Kouchoukos, N. T., Blackstone, E. H., Hanley, F. L., and Kirklin, J. K. (2012). Kirklin/Barratt-Boyes Cardiac Surgery E-Book. Amsterdam: Elsevier Health Sciences.
Kraehling, J. R., and Sessa, W. C. (2017). Contemporary approaches to modulating the nitric oxide–cgmp pathway in cardiovascular disease. Circ. Res. 120, 1174–1182. doi: 10.1161/CIRCRESAHA.117.303776
Kraft, F., Schmidt, C., Van Aken, H., and Zarbock, A. (2015). Inflammatory response and extracorporeal circulation. Best Pract. Res. Clin. Anaesthesiol. 29, 113–123. doi: 10.1016/j.bpa.2015.03.001
Krötz, F., Sohn, H. Y., Gloe, T., Zahler, S., Riexinger, T., Schiele, T. M., et al. (2002). NAD (P) H oxidase–dependent platelet superoxide anion release increases platelet recruitment. Blood 100, 917–924. doi: 10.1182/blood.V100.3.917
Kul, M., Vurucu, S., Demirkaya, E., Tunc, T., Aydinoz, S., Meral, C., et al. (2009). Enteral glutamine and/or arginine supplementation have favorable effects on oxidative stress parameters in neonatal rat intestine. J. Pediatr. Gastroenterol. Nutr. 49, 85–89. doi: 10.1097/MPG.0b013e318198cd36
Kumar, T. S., Zurakowski, D., Dalton, H., Talwar, S., Allard-Picou, A., Duebener, L. F., et al. (2010). Extracorporeal membrane oxygenation in postcardiotomy patients: factors influencing outcome. J. Thorac. Cardiovasc. Surg. 140, 330–336.e2. doi: 10.1016/j.jtcvs.2010.02.034
Lakshminrusimha, S., Russell, J. A., Wedgwood, S., Gugino, S. F., Kazzaz, J. A., Davis, J. M., et al. (2006). Superoxide dismutase improves oxygenation and reduces oxidation in neonatal pulmonary hypertension. Am. J. Respir. Crit. Care Med. 174, 1370–1377. doi: 10.1164/rccm.200605-676OC
Lamb, N. J., Quinlan, G. J., Westerman, S. T., Gutteridge, J. M., and Evans, T. W. (1999). Nitration of proteins in bronchoalveolar lavage fluid from patients with acute respiratory distress syndrome receiving inhaled nitric oxide. Am. J. Respir. Crit. Care Med. 160, 1031–1034. doi: 10.1164/ajrccm.160.3.9810048
Lambeth, J. D. (2004). NOX enzymes and the biology of reactive oxygen. Nat. Rev. Immunol. 4, 181–189. doi: 10.1038/nri1312
Lambeth, J. D., Krause, K.-H., and Clark, R. A. (2008). NOX enzymes as novel targets for drug development. Semin. Immunopathol. 30, 339–363. doi: 10.1007/s00281-008-0123-6
Laplace, C., Huet, O., Vicaut, E., Ract, C., Martin, L., Benhamou, D., et al. (2005). Endothelial oxidative stress induced by serum from patients with severe trauma hemorrhage. Intensive Care Med. 31, 1174–1180. doi: 10.1007/s00134-005-2737-7
Lee, J. W., Fang, X., Gupta, N., Serikov, V., and Matthay, M. A. (2009). Allogeneic human mesenchymal stem cells for treatment of E. coli endotoxin-induced acute lung injury in the ex vivo perfused human lung. Proc. Natl. Acad. Sci. U.S.A. 106, 16357–16362. doi: 10.1073/pnas.0907996106
Lenz, A., Jorens, P., Meyer, B., De Backer, W., Van Overveld, F., Bossaert, L., et al. (1999). Oxidatively modified proteins in bronchoalveolar lavage fluid of patients with ARDS and patients at-risk for ARDS. Eur. Respir. J. 13, 169–174. doi: 10.1034/j.1399-3003.1999.13a31.x
Levy, J. H., and Tanaka, K. A. (2003). Inflammatory response to cardiopulmonary bypass. Ann. Thorac. Surg. 75, S715–S720. doi: 10.1016/S0003-4975(02)04701-X
Li, P., Gu, Y., Yu, S., Li, Y., Yang, J., and Jia, G. (2014). Assessing the suitability of 8-OHdG and micronuclei as genotoxic biomarkers in chromate-exposed workers: a cross-sectional study. BMJ Open 4:e005979. doi: 10.1136/bmjopen-2014-005979
Libetta, C., Sepe, V., Esposito, P., Galli, F., and Dal Canton, A. (2011). Oxidative stress and inflammation: implications in uremia and hemodialysis. Clin. Biochem. 44, 1189–1198. doi: 10.1016/j.clinbiochem.2011.06.988
Liu, Y.-Y., Chiang, C.-H., Hung, S.-C., Chian, C.-F., Tsai, C.-L., Chen, W.-C., et al. (2017). Hypoxia-preconditioned mesenchymal stem cells ameliorate ischemia/reperfusion-induced lung injury. PLoS One 12:e0187637. doi: 10.1371/journal.pone.0187637
Lobo, V., Patil, A., Phatak, A., and Chandra, N. (2010). Free radicals, antioxidants and functional foods: impact on human health. Pharmacogn. Rev. 4, 118–126. doi: 10.4103/0973-7847.70902
Lou, S., Maclaren, G., Best, D., Delzoppo, C., and Butt, W. (2014). Hemolysis in pediatric patients receiving centrifugal-pump extracorporeal membrane oxygenation: prevalence, risk factors, and outcomes. Crit. Care Med. 42, 1213–1220. doi: 10.1097/CCM.0000000000000128
Lowes, D., Webster, N., Murphy, M., and Galley, H. (2013). Antioxidants that protect mitochondria reduce interleukin-6 and oxidative stress, improve mitochondrial function, and reduce biochemical markers of organ dysfunction in a rat model of acute sepsis. Br. J. Anaesth. 110, 472–480. doi: 10.1093/bja/aes577
Luban, N. L. (1995). Massive transfusion in the neonate. Transfus. Med. Rev. 9, 200–214. doi: 10.1016/S0887-7963(05)80110-5
Mackman, N., Tilley, R. E., and Key, N. S. (2007). Role of the extrinsic pathway of blood coagulation in hemostasis and thrombosis. Arterioscler. Thromb. Vasc. Biol. 27, 1687–1693. doi: 10.1161/ATVBAHA.107.141911
MacMicking, J., Xie, Q.-W., and Nathan, C. (1997). Nitric oxide and macrophage function. Annu. Rev. Immunol. 15, 323–350. doi: 10.1146/annurev.immunol.15.1.323
Maiorino, M., Conrad, M., and Ursini, F. (2018). GPx4, lipid peroxidation, and cell death: discoveries, rediscoveries, and open issues. Antioxid. Redox Signal. 29, 61–74. doi: 10.1089/ars.2017.7115
Maltepe, E., and Saugstad, O. D. (2009). Oxygen in health and disease: regulation of oxygen homeostasis-clinical implications. Pediatr. Res. 65, 261–268. doi: 10.1203/PDR.0b013e31818fc83f
Manja, V., Lakshminrusimha, S., and Cook, D. J. (2015). Oxygen saturation target range for extremely preterm infants: a systematic review and meta-analysis. JAMA Pediatr. 169, 332–340. doi: 10.1001/jamapediatrics.2014.3307
Mantle, D., Falkous, G., and Walker, D. (1999). Quantification of protease activities in synovial fluid from rheumatoid and osteoarthritis cases: comparison with antioxidant and free radical damage markers. Clin. Chim. Acta 284, 45–58. doi: 10.1016/S0009-8981(99)00055-8
Manzanares, W., Dhaliwal, R., Jiang, X., Murch, L., and Heyland, D. K. (2012). Antioxidant micronutrients in the critically ill: a systematic review and meta-analysis. Crit. Care 16:R66. doi: 10.1186/cc11316
Manzanares, W., Lemieux, M., Elke, G., Langlois, P. L., Bloos, F., and Heyland, D. K. (2016). High-dose intravenous selenium does not improve clinical outcomes in the critically ill: a systematic review and meta-analysis. Crit. Care 20:356. doi: 10.1186/s13054-016-1529-5
Margaritelis, N., Kyparos, A., Paschalis, V., Theodorou, A., Panayiotou, G., Zafeiridis, A., et al. (2014). Reductive stress after exercise: the issue of redox individuality. Redox Biol. 2, 520–528. doi: 10.1016/j.redox.2014.02.003
Mascio, C. E., Austin, E. H., Jacobs, J. P., Jacobs, M. L., Wallace, A. S., He, X., et al. (2014). Perioperative mechanical circulatory support in children: an analysis of the Society of Thoracic Surgeons Congenital Heart Surgery Database. J. Thorac. Cardiovasc. Surg. 147, 658–665. doi: 10.1016/j.jtcvs.2013.09.075
Matata, B. M., Sosnowski, A. W., and Galiñanes, M. (2000). Off-pump bypass graft operation significantly reduces oxidative stress and inflammation. Ann. Thorac. Surg. 69, 785–791. doi: 10.1016/S0003-4975(99)01420-4
Matés, J. M., Pérez-Gómez, C., and De Castro, I. N. (1999). Antioxidant enzymes and human diseases. Clin. Biochem. 32, 595–603. doi: 10.1016/S0009-9120(99)00075-2
Mátyás, M., and Zaharie, G. (2018). “Particularities of oxidative stress in newborns,” in Novel Prospects in Oxidative and Nitrosative Stress, ed. P. Atukeren (London: IntechOpen).
McCoy-Pardington, D., Judd, W., Knafl, P., Abruzzo, L. V., Coombes, K., Butch, S., et al. (1990). Blood use during extracorporeal membrane oxygenation. Transfusion 30, 307–309. doi: 10.1046/j.1537-2995.1990.30490273436.x
McDonald, C. I., Fraser, J. F., Coombes, J. S., and Fung, Y. L. (2014a). Oxidative stress during extracorporeal circulation. Eur. J. Cardiothorac. Surg. 46, 937–943. doi: 10.1093/ejcts/ezt637
McDonald, C. I., Fraser, J. F., Shekar, K., Dunster, K. R., Thom, O., and Fung, Y. L. (2014b). Transfusion of packed red blood cells reduces selenium levels and increases lipid peroxidation in an in vivo ovine model. Transfus. Med. 24, 50–54.
McDonald, C. I., Fung, Y. L., and Fraser, J. F. (2012). Antioxidant trace element reduction in an in vitro cardiopulmonary bypass circuit. ASAIO J. 58, 217–222. doi: 10.1097/MAT.0b013e31824cc856
Mcilwain, R. B., Timpa, J. G., Kurundkar, A. R., Holt, D. W., Kelly, D. R., Hartman, Y. E., et al. (2010). Plasma concentrations of inflammatory cytokines rise rapidly during ECMO-related SIRS due to the release of preformed stores in the intestine. Lab. Invest. 90, 128–139. doi: 10.1038/labinvest.2009.119
Meyer, R. J., Brophy, P. D., Bunchman, T. E., Annich, G. M., Maxvold, N. J., Mottes, T. A., et al. (2001). Survival and renal function in pediatric patients following extracorporeal life support with hemofiltration. Pediatr. Crit. Care Med. 2, 238–242. doi: 10.1097/00130478-200107000-00009
Mikolka, P., Kopincova, J., Mikusiakova, L. T., Kosutova, P., Calkovska, A., and Mokra, D. (2016). Antiinflammatory effect of N-acetylcysteine combined with exogenous surfactant in meconium-induced lung injury. Adv. Exp. Med. Biol. 934, 63–75. doi: 10.1007/5584_2016_15
Mildner, R. J., Taub, N., Vyas, J. R., Killer, H. M., Firmin, R. K., Field, D. J., et al. (2005). Cytokine imbalance in infants receiving extracorporeal membrane oxygenation for respiratory failure. Neonatology 88, 321–327. doi: 10.1159/000087630
Millar, J. E., Fanning, J. P., McDonald, C. I., Mcauley, D. F., and Fraser, J. F. (2016). The inflammatory response to extracorporeal membrane oxygenation (ECMO): a review of the pathophysiology. Crit. Care 20:387. doi: 10.1186/s13054-016-1570-4
Mishra, O. P., Pooniya, V., Ali, Z., Upadhyay, R. S., and Prasad, R. (2008). Antioxidant status of children with acute renal failure. Pediatr. Nephrol. 23:2047. doi: 10.1007/s00467-008-0875-1
Mittal, M., Siddiqui, M. R., Tran, K., Reddy, S. P., and Malik, A. B. (2014). Reactive oxygen species in inflammation and tissue injury. Antioxid. Redox Signal. 20, 1126–1167. doi: 10.1089/ars.2012.5149
Mokra, D., Drgova, A., Mokry, J., Antosova, M., Durdik, P., and Calkovska, A. (2015). N-acetylcysteine effectively diminished meconium-induced oxidative stress in adult rabbits. J. Physiol. Pharmacol. 66, 101–110.
Möller, J., Gilman, J., Sussmane, J., Raszynski, A., and Wolfsdorf, J. (1993). Changes in plasma levels of oxygen radical scavenging enzymes during extracorporeal membrane oxygenation in a lamb model. Neonatology 64, 134–139. doi: 10.1159/000243983
Montuschi, P., Barnes, P. J., and Roberts, L. J. (2004). Isoprostanes: markers and mediators of oxidative stress. FASEB J. 18, 1791–1800. doi: 10.1096/fj.04-2330rev
Moore, T. A., Ahmad, I. M., and Zimmerman, M. C. (2018). Oxidative stress and preterm birth: an integrative review. Biol. Res. Nurs. 20, 497–512. doi: 10.1177/1099800418791028
Motoyama, T., Okamoto, K., Kukita, I., Hamaguchi, M., Kinoshita, Y., and Ogawa, H. (2003). Possible role of increased oxidant stress in multiple organ failure after systemic inflammatory response syndrome. Crit. Care Med. 31, 1048–1052. doi: 10.1097/01.CCM.0000055371.27268.36
Murphy, D. A., Hockings, L. E., Andrews, R. K., Aubron, C., Gardiner, E. E., Pellegrino, V. A., et al. (2015). Extracorporeal membrane oxygenation—hemostatic complications. Transfus. Med. Rev. 29, 90–101. doi: 10.1016/j.tmrv.2014.12.001
Nawab, U., and Williams, S. B. (2017). “Transfusion considerations for neonatal extracorporeal membrane oxygenation (ECMO),” in Neonatal Transfusion Practices, ed. D. A. Sesok-Pizzini (Berlin: Springer), 103–121. doi: 10.1007/978-3-319-42764-5_7
Neumar, R. W. (2011). Optimal oxygenation during and after cardiopulmonary resuscitation. Curr. Opin. Crit. Care 17, 236–240. doi: 10.1097/MCC.0b013e3283454c8c
Niki, E., Yoshida, Y., Saito, Y., and Noguchi, N. (2005). Lipid peroxidation: mechanisms, inhibition, and biological effects. Biochem. Biophys. Res. Commun. 338, 668–676. doi: 10.1016/j.bbrc.2005.08.072
Ogilvie, A., Groeneveld, A., Straub, J., and Thijs, L. (1991). Plasma lipid peroxides and antioxidants in human septic shock. Intensive Care Med. 17, 40–44. doi: 10.1007/BF01708408
Oliver, W. C. (2009). Anticoagulation and coagulation management for ECMO. Semin. Cardiothorac. Vasc. Anesth. 13, 154–175. doi: 10.1177/1089253209347384
Omar, H. R., Mirsaeidi, M., Socias, S., Sprenker, C., Caldeira, C., Camporesi, E. M., et al. (2015). Plasma free hemoglobin is an independent predictor of mortality among patients on extracorporeal membrane oxygenation support. PLoS One 10:e0124034. doi: 10.1371/journal.pone.0124034
Orban, J.-C., and Singer, M. (2018). “Oxygen and oxidative stress,” in Metabolic Disorders and Critically Ill Patients, eds C. Ichai, H. Quintard, and J.-C. Orban (Berlin: Springer), 431–439. doi: 10.1007/978-3-319-64010-5_20
Ortiz, L. A., Gambelli, F., Mcbride, C., Gaupp, D., Baddoo, M., Kaminski, N., et al. (2003). Mesenchymal stem cell engraftment in lung is enhanced in response to bleomycin exposure and ameliorates its fibrotic effects. Proc. Natl. Acad. Sci. U.S.A. 100, 8407–8411. doi: 10.1073/pnas.1432929100
Ozaydin, M., Peker, O., Erdogan, D., Kapan, S., Turker, Y., Varol, E., et al. (2008). N-acetylcysteine for the prevention of postoperative atrial fibrillation: a prospective, randomized, placebo-controlled pilot study. Eur. Heart J. 29, 625–631. doi: 10.1093/eurheartj/ehn011
Ozbek, E. (2012). Induction of oxidative stress in kidney. Int. J. Nephrol. 2012:465897. doi: 10.1155/2012/465897
Ozdemir, R., Yurttutan, S., Sarı, F. N., Uysal, B., Unverdi, H. G., Canpolat, F. E., et al. (2012). Antioxidant effects of N-acetylcysteine in a neonatal rat model of necrotizing enterocolitis. J. Pediatr. Surg. 47, 1652–1657. doi: 10.1016/j.jpedsurg.2012.02.016
Pacher, P., Beckman, J. S., and Liaudet, L. (2007). Nitric oxide and peroxynitrite in health and disease. Physiol. Rev. 87, 315–424. doi: 10.1152/physrev.00029.2006
Packer, L., Tritschler, H. J., and Wessel, K. (1997). Neuroprotection by the metabolic antioxidant α-lipoic acid. Free Radic. Biol. Med. 22, 359–378. doi: 10.1016/S0891-5849(96)00269-9
Paden, M. L., Warshaw, B. L., Heard, M. L., and Fortenberry, J. D. (2011). Recovery of renal function and survival after continuous renal replacement therapy during extracorporeal membrane oxygenation. Pediatr. Crit. Care Med. 12, 153–158. doi: 10.1097/PCC.0b013e3181e2a596
Palatianos, G. M., Foroulis, C. N., Vassili, M. I., Astras, G., Triantafillou, K., Papadakis, E., et al. (2003). A prospective, double-blind study on the efficacy of the bioline surface-heparinized extracorporeal perfusion circuit. Ann. Thorac. Surg. 76, 129–135. doi: 10.1016/S0003-4975(03)00338-2
Pammi, M., and Suresh, G. (2017). Enteral lactoferrin supplementation for prevention of sepsis and necrotizing enterocolitis in preterm infants. Cochrane Database Syst. Rev. 6:CD007137. doi: 10.1002/14651858.CD007137.pub5
Perrone, S., Luisa Tataranno, M., Santacroce, A., Negro, S., and Buonocore, G. (2014). The role of oxidative stress on necrotizing enterocolitis in very low birth weight infants. Curr. Pediatr. Rev. 10, 202–207.
Perrone, S., Santacroce, A., Longini, M., Proietti, F., Bazzini, F., and Buonocore, G. (2018). The free radical diseases of prematurity: from cellular mechanisms to bedside. Oxid. Med. Cell. Longev. 2018:7483062. doi: 10.1155/2018/7483062
Perrone, S., Tataranno, M. L., Negro, S., Longini, M., Marzocchi, B., Proietti, F., et al. (2010). Early identification of the risk for free radical-related diseases in preterm newborns. Early Hum. Dev. 86, 241–244. doi: 10.1016/j.earlhumdev.2010.03.008
Perrone, S., Vezzosi, P., Longini, M., Marzocchi, B., Paffetti, P., Bellieni, C. V., et al. (2009). Biomarkers of oxidative stress in babies at high risk for retinopathy of prematurity. Front. Biosci. 1, 547–552.
Petersen, J., Kazakov, A., Böhm, M., Schäfers, H.-J., Laufs, U., and Abdul-Khaliq, H. (2018). Cardiopulmonary bypass reduces myocardial oxidative stress, inflammation and increases c-kit+ CD45- cell population in newborns. J. Transl. Med. 16:111. doi: 10.1186/s12967-018-1478-7
Philippu, A. (2016). Nitric Oxide: a universal modulator of brain function. Curr. Med. Chem. 23, 2643–2652. doi: 10.2174/0929867323666160627120408
Plicner, D., Mazur, P., Sadowski, J., and Undas, A. (2014). Asymmetric dimethylarginine and oxidative stress following coronary artery bypass grafting: associations with postoperative outcome. Eur. J. Cardiothorac. Surg. 45, e136–e141. doi: 10.1093/ejcts/ezt646
Plötz, F., Van Oeveren, W., Bartlett, R., and Wildevuur, C. (1993). Blood activation during neonatal extracorporeal life support. J. Thorac. Cardiovasc. Surg. 105, 823–832.
Poggi, C., and Dani, C. (2018). Sepsis and oxidative stress in the newborn: from pathogenesis to novel therapeutic targets. Oxid. Med. Cell. Longev. 2018:9390140. doi: 10.1155/2018/9390140
Prasad, K., Kalra, J., Bharadwaj, B., and Chaudhary, A. K. (1992). Increased oxygen free radical activity in patients on cardiopulmonary bypass undergoing aortocoronary bypass surgery. Am. Heart J. 123, 37–45. doi: 10.1016/0002-8703(92)90744-G
Prescott, S. M., Zimmerman, G. A., and Mcintyre, T. M. (1984). Human endothelial cells in culture produce platelet-activating factor (1-alkyl-2-acetyl-sn-glycero-3-phosphocholine) when stimulated with thrombin. Proc. Natl. Acad. Sci. U.S.A. 81, 3534–3538. doi: 10.1073/pnas.81.11.3534
Quinlan, G. J., Lamb, N. J., Evans, T. W., and Gutteridge, J. M. (1996). Plasma fatty acid changes and increased lipid peroxidation in patients with adult respiratory distress syndrome. Crit. Care Med. 24, 241–246. doi: 10.1097/00003246-199602000-00010
Quinlan, G. J., Mumby, S., Martin, G. S., Bernard, G. R., Gutteridge, J. M., and Evans, T. W. (2004). Albumin influences total plasma antioxidant capacity favorably in patients with acute lung injury. Crit. Care Med. 32, 755–759. doi: 10.1097/01.CCM.0000114574.18641.5D
Radi, R. (2013). Peroxynitrite, a stealthy biological oxidant. J. Biol. Chem. 288, 26464–26472. doi: 10.1074/jbc.R113.472936
Ramsay, P. L., Demayo, F. J., Hegemier, S. E., Wearden, M. E., Smith, C. V., and Welty, S. E. (2001). Clara cell secretory protein oxidation and expression in premature infants who develop bronchopulmonary dysplasia. Am. J. Respir. Crit. Care Med. 164, 155–161. doi: 10.1164/ajrccm.164.1.2008022
Renke, J., Popadiuk, S., Korzon, M., Bugajczyk, B., and Woźniak, M. (2000). Protein carbonyl groups’ content as a useful clinical marker of antioxidant barrier impairment in plasma of children with juvenile chronic arthritis. Free Radic. Biol. Med. 29, 101–104. doi: 10.1016/S0891-5849(00)00288-4
Richard, C., Lemonnier, F., Thibault, M., Couturier, M., and Auzepy, P. (1990). Vitamin E deficiency and lipoperoxidation during adult respiratory distress syndrome. Crit. Care Med. 18, 4–9. doi: 10.1097/00003246-199001000-00002
Rodell, T. C., Naidoo, Y., and Bhoola, K. D. (1995). Role of kinins in inflammatory responses. Clin. Immunother. 3, 352–361. doi: 10.1007/BF03259501
Romano, A., Serviddio, G., De Matthaeis, A., and Bellanti, F., Vendemiale, G. (2010). Oxidative stress and aging. J. Nephrol. 23, S29–S36.
Romano, R., Cristescu, S. M., Risby, T. H., and Marczin, N. (2018). Lipid peroxidation in cardiac surgery: towards consensus on biomonitoring, diagnostic tools and therapeutic implementation. J. Breath Res. 12:027109. doi: 10.1088/1752-7163/aa9856
Rood, K. L., Teele, S. A., Barrett, C. S., Salvin, J. W., Rycus, P. T., Fynn-Thompson, F., et al. (2011). Extracorporeal membrane oxygenation support after the Fontan operation. J. Thorac. Cardiovasc. Surg. 142, 504–510. doi: 10.1016/j.jtcvs.2010.11.050
Roos, W. P., and Kaina, B. (2006). DNA damage-induced cell death by apoptosis. Trends Mol. Med. 12, 440–450. doi: 10.1016/j.molmed.2006.07.007
Rosa, S. D., Bristot, M. L., Topanotti, M. F., Tomasi, C. D., Felisberto, F., Vuolo, F. S., et al. (2011). Effect of red blood cell transfusion on parameters of inflammation and oxidative stress in critically ill patients. Rev. Bras. Ter. Intensiva 23, 30–35. doi: 10.1590/S0103-507X2011000100006
Rother, R. P., Bell, L., Hillmen, P., and Gladwin, M. T. (2005). The clinical sequelae of intravascular hemolysis and extracellular plasma hemoglobin: a novel mechanism of human disease. JAMA 293, 1653–1662. doi: 10.1001/jama.293.13.1653
Rotruck, J. T., Pope, A. L., Ganther, H., Swanson, A., Hafeman, D. G., and Hoekstra, W. (1973). Selenium: biochemical role as a component of glutathione peroxidase. Science 179, 588–590. doi: 10.1126/science.179.4073.588
Rungatscher, A., Tessari, M., Stranieri, C., Solani, E., Linardi, D., Milani, E., et al. (2015). Oxygenator is the main responsible for leukocyte activation in experimental model of extracorporeal circulation: a cautionary tale. Mediators Inflamm. 2015:484979. doi: 10.1155/2015/484979
Sahoo, S., Meijles, D. N., and Pagano, P. J. (2016). NADPH oxidases: key modulators in aging and age-related cardiovascular diseases? Clin. Sci. 130, 317–335. doi: 10.1042/CS20150087
Salvin, J. W., Laussen, P. C., and Thiagarajan, R. R. (2008). Extracorporeal membrane oxygenation for postcardiotomy mechanical cardiovascular support in children with congenital heart disease. Pediatr. Anesth. 18, 1157–1162. doi: 10.1111/j.1460-9592.2008.02795.x
Saugstad, O. D. (2005). Oxidative stress in the newborn–a 30-year perspective. Neonatology 88, 228–236. doi: 10.1159/000087586
Saugstad, O. D., and Aune, D. (2014). Optimal oxygenation of extremely low birth weight infants: a meta-analysis and systematic review of the oxygen saturation target studies. Neonatology 105, 55–63. doi: 10.1159/000356561
Saugstad, O. D., Sejersted, Y., Solberg, R., Wollen, E. J., and Bjørås, M. (2012). Oxygenation of the newborn: a molecular approach. Neonatology 101, 315–325. doi: 10.1159/000337345
Schieber, M., and Chandel, N. S. (2014). ROS function in redox signaling and oxidative stress. Curr. Biol. 24, R453–R462. doi: 10.1016/j.cub.2014.03.034
Schmidt, B., Whyte, R. K., Asztalos, E. V., Moddemann, D., Poets, C., Rabi, Y., et al. (2013). Effects of targeting higher vs lower arterial oxygen saturations on death or disability in extremely preterm infants: a randomized clinical trial. JAMA 309, 2111–2120. doi: 10.1001/jama.2013.5555
Selewski, D. T., Cornell, T. T., Blatt, N. B., Han, Y. Y., Mottes, T., Kommareddi, M., et al. (2012). Fluid overload and fluid removal in pediatric patients on extracorporeal membrane oxygenation requiring continuous renal replacement therapy. Crit. Care Med. 40, 2694. doi: 10.1097/CCM.0b013e318258ff01
Semenza, G. L. (2009). Regulation of oxygen homeostasis by hypoxia-inducible factor 1. Physiology 24, 97–106. doi: 10.1152/physiol.00045.2008
Shi, J., Chen, Q., Yu, W., Shen, J., Gong, J., He, C., et al. (2014). Continuous renal replacement therapy reduces the systemic and pulmonary inflammation induced by venovenous extracorporeal membrane oxygenation in a porcine model. Artif. Organs 38, 215–223. doi: 10.1111/aor.12154
Shohat, M., Reisner, S. H., Krikler, R., Nissenkorn, I., Yassur, Y., and Ben-Sira, I. (1983). Retinopathy of prematurity: incidence and risk factors. Pediatrics 72, 159–163.
Sies, H. (2018). On the history of oxidative stress: concept and some aspects of current development. Curr. Opin. Toxicol. 7, 122–126. doi: 10.1016/j.cotox.2018.01.002
Signorini, C., Perrone, S., Sgherri, C., Ciccoli, L., Buonocore, G., Leoncini, S., et al. (2008). Plasma esterified F 2-isoprostanes and oxidative stress in newborns: role of nonprotein-bound iron. Pediatr. Res. 63, 287–291. doi: 10.1203/PDR.0b013e318163a1fd
Silvers, K., Gibson, A., Russell, J., and Powers, H. (1998). Antioxidant activity, packed cell transfusions, and outcome in premature infants. Arch. Dis. Child Fetal Neonatal Ed. 78, F214–F219. doi: 10.1136/fn.78.3.F214
Simonyi, A., Serfozo, P., Lehmidi, T. M., Cui, J., Gu, Z., Lubahn, D. B., et al. (2012). The neuroprotective effects of apocynin. Front. Biosci. 4, 2183–2193. doi: 10.2741/e535
Small, D. M., Coombes, J. S., Bennett, N., Johnson, D. W., and Gobe, G. C. (2012). Oxidative stress, anti-oxidant therapies and chronic kidney disease. Nephrology 17, 311–321. doi: 10.1111/j.1440-1797.2012.01572.x
Smith, A., Hardison, D., Bridges, B., and Pietsch, J. (2013). Red blood cell transfusion volume and mortality among patients receiving extracorporeal membrane oxygenation. Perfusion 28, 54–60. doi: 10.1177/0267659112457969
Sonego, G., Abonnenc, M., Tissot, J.-D., Prudent, M., and Lion, N. (2017). Redox proteomics and platelet activation: understanding the redox proteome to improve platelet quality for transfusion. Int. J. Mol. Sci. 18:E387. doi: 10.3390/ijms18020387
Sosa, M. A., Balk, E. M., Lau, J., Liangos, O., Balakrishnan, V. S., Madias, N. E., et al. (2006). A systematic review of the effect of the Excebrane dialyser on biomarkers of lipid peroxidation. Nephrol. Dial. Transplant. 21, 2825–2833. doi: 10.1093/ndt/gfl376
Steinhorn, R. H., Isham-Schopf, B., Smith, C., and Green, T. P. (1989). Hemolysis during long-term extracorporeal membrane oxygenation. J. Pediatr. 115, 625–630. doi: 10.1016/S0022-3476(89)80299-9
Stevens, K. L., Black, A. L., Wells, K. M., Yeo, K. B., Steuart, R. F., Stirling, C. J., et al. (2017). Diminished Ost3-dependent N-glycosylation of the BiP nucleotide exchange factor Sil1 is an adaptive response to reductive ER stress. Proc. Natl. Acad. Sci. U.S.A. 114, 12489–12494. doi: 10.1073/pnas.1705641114
Stiller, B., Lemmer, J., Merkle, F., Alexi-Meskishvili, V., Weng, Y., Hübler, M., et al. (2004). Consumption of blood products during mechanical circulatory support in children: comparison between ECMO and a pulsatile ventricular assist device. Intensive Care Med. 30, 1814–1820. doi: 10.1007/s00134-004-2352-z
Stoppe, C., Spillner, J., Rossaint, R., Coburn, M., Schälte, G., Wildenhues, A., et al. (2013). Selenium blood concentrations in patients undergoing elective cardiac surgery and receiving perioperative sodium selenite. Nutrition 29, 158–165. doi: 10.1016/j.nut.2012.05.013
Stuehr, D. J. (1999). Mammalian nitric oxide synthases. Biochim. Biophys. Acta 1411, 217–230. doi: 10.1016/S0005-2728(99)00016-X
Suliman, H. B., Carraway, M. S., and Piantadosi, C. A. (2003). Postlipopolysaccharide oxidative damage of mitochondrial DNA. Am. J. Respir. Crit. Care Med. 167, 570–579. doi: 10.1164/rccm.200206-518OC
Suresh, G., Davis, J. M., and Soll, R. (2001). Superoxide dismutase for preventing chronic lung disease in mechanically ventilated preterm infants. Cochrane Database Syst. Rev. 1:CD001968. doi: 10.1002/14651858.CD001968
Swaniker, F., Kolla, S., Moler, F., Custer, J., Grams, R., Bartlett, R., et al. (2000). Extracorporeal life support outcome for 128 pediatric patients with respiratory failure. J. Pediatr. Surg. 35, 197–202. doi: 10.1016/S0022-3468(00)90009-5
Sznycer-Taub, N. R., Lowery, R., Yu, S., Owens, S. T., Hirsch-Romano, J. C., and Owens, G. E. (2016). Hyperoxia is associated with poor outcomes in pediatric cardiac patients supported on venoarterial extracorporeal membrane oxygenation. Pediatr. Crit. Care Med. 17, 350–358. doi: 10.1097/PCC.0000000000000655
Takeda, K., Shimada, Y., Amano, M., Sakai, T., Okada, T., and Yoshiya, I. (1984). Plasma lipid peroxides and alpha-tocopherol in critically ill patients. Crit. Care Med. 12, 957–959. doi: 10.1097/00003246-198411000-00007
Takouli, L., Hadjiyannakos, D., Metaxaki, P., Sideris, V., Filiopoulos, V., Anogiati, A., et al. (2010). Vitamin E-coated cellulose acetate dialysis membrane: long-term effect on inflammation and oxidative stress. Ren. Fail. 32, 287–293. doi: 10.3109/08860221003615795
Tappel, A. (1970). Biological antioxidant protection against lipid peroxidation damage. Am. J. Clin. Nutr. 23, 1137–1139. doi: 10.1093/ajcn/23.8.1137
Tarakçıoğlu, M., Erbağci, A. B., Usalan, C., Deveci, R., and Kocabaş, R. (2003). Acute effect of hemodialysis on serum levels of the proinflammatory cytokines. Mediators Inflamm. 12, 15–19. doi: 10.1080/0962935031000096935
Tayama, E., Hayashida, N., Akasu, K., Kosuga, T., Fukunaga, S., Akashi, H., et al. (2000). Biocompatibility of heparin-coated extracorporeal bypass circuits: new heparin bonded bioline system. Artif. Organs 24, 618–623. doi: 10.1046/j.1525-1594.2000.06615.x
Thiagarajan, R. R., Barbaro, R. P., Rycus, P. T., Mcmullan, D. M., Conrad, S. A., Fortenberry, J. D., et al. (2017). Extracorporeal life support organization registry international report 2016. ASAIO J. 63, 60–67. doi: 10.1097/MAT.0000000000000475
Thiara, A., Andersen, V., Videm, V., Mollnes, T., Svennevig, K., Hoel, T., et al. (2010). Comparable biocompatibility of Phisio-and Bioline-coated cardiopulmonary bypass circuits indicated by the inflammatory response. Perfusion 25, 9–16. doi: 10.1177/0267659110362822
Tin, W., and Gupta, S. (2007). Optimum oxygen therapy in preterm babies. Arch. Dis. Child. Fetal Neonatal Ed. 92, F143–F147. doi: 10.1136/adc.2005.092726
Tonelli, M., Wiebe, N., Hemmelgarn, B., Klarenbach, S., Field, C., Manns, B., et al. (2009). Trace elements in hemodialysis patients: a systematic review and meta-analysis. BMC Med. 7:25. doi: 10.1186/1741-7015-7-25
Trittenwein, G., Rotta, A. T., Gunnarsson, B., and Steinhorn, D. M. (1999). Lipid peroxidation during initiation of extracorporeal membrane oxygenation after hypoxia in endotoxemic rabbits. Perfusion 14, 49–57. doi: 10.1177/026765919901400108
Tyson, J. E., Wright, L. L., Oh, W., Kennedy, K. A., Mele, L., Ehrenkranz, R. A., et al. (1999). Vitamin A supplementation for extremely-low-birth-weight infants. N. Engl. J. Med. 340, 1962–1968. doi: 10.1056/NEJM199906243402505
Ulus, A., Aksoyek, A., Ozkan, M., Katircioglu, S., and Basu, S. (2003). Cardiopulmonary bypass as a cause of free radical-induced oxidative stress and enhanced blood-borne isoprostanes in humans. Free Radic. Biol. Med. 34, 911–917. doi: 10.1016/S0891-5849(03)00030-3
Uyar, I. S., Akpinar, M. B., Sahin, V., Uguz, A. C., Onal, S., Gonen, I., et al. (2013). Alpha lipoic acid attenuates inflammatory response during extracorporeal circulation. Cardiovasc. J. Afr. 24, 322–326. doi: 10.5830/CVJA-2013-067
Varan, H. I., Dursun, B., Dursun, E., Ozben, T., and Suleymanlar, G. (2010). Acute effects of hemodialysis on oxidative stress parameters in chronic uremic patients: comparison of two dialysis membranes. Int. J. Nephrol. Renovasc. Dis. 3, 39–45.
Vento, M. (2014). Oxygen supplementation in the neonatal period: changing the paradigm. Neonatology 105, 323–331. doi: 10.1159/000360646
Vento, M., Escobar, J., Cernada, M., Escrig, R., and Aguar, M. (2012). The use and misuse of oxygen during the neonatal period. Clin. Perinatol. 39, 165–176. doi: 10.1016/j.clp.2011.12.014
Visser, J., Labadarios, D., and Blaauw, R. (2011). Micronutrient supplementation for critically ill adults: a systematic review and meta-analysis. Nutrition 27, 745–758. doi: 10.1016/j.nut.2010.12.009
Wachtfogel, Y. T., Hack, C. E., Nuijens, J., Kettner, C., Reilly, T. M., Knabb, R. M., et al. (1995). Selective kallikrein inhibitors alter human neutrophil elastase release during extracorporeal circulation. Am. J. Physiol. Heart Circ. Physiol. 268, H1352–H1357. doi: 10.1152/ajpheart.1995.268.3.H1352
Walski, T., Drohomirecka, A., Bujok, J., Czerski, A., Waż, G., Trochanowska, N., et al. (2018). Low-level light therapy protects red blood cells against oxidative stress and haemolysis during extracorporeal circulation. Front. Physiol. 9:647. doi: 10.3389/fphys.2018.00647
Walski, T., Dyrda, A., Dzik, M., Chludzińska, L., Tomków, T., Mehl, J., et al. (2015). Near infrared light induces post-translational modifications of human red blood cell proteins. Photochem. Photobiol. Sci. 14, 2035–2045. doi: 10.1039/c5pp00203f
Wan, S., Leclerc, J.-L., and Vincent, J.-L. (1997). Inflammatory response to cardiopulmonary bypass: mechanisms involved and possible therapeutic strategies. Chest 112, 676–692. doi: 10.1378/chest.112.3.676
Wang, D., Sun, J., Solomon, S. B., Klein, H. G., and Natanson, C. (2012). Transfusion of older stored blood and risk of death: a meta-analysis. Transfusion 52, 1184–1195. doi: 10.1111/j.1537-2995.2011.03466.x
Wang, S., Krawiec, C., Patel, S., Kunselman, A. R., Song, J., Lei, F., et al. (2015). Laboratory evaluation of hemolysis and systemic inflammatory response in neonatal nonpulsatile and pulsatile extracorporeal life support systems. Artif. Organs 39, 774–781. doi: 10.1111/aor.12466
Wardle, S., Drury, J., Garr, R., and Weindling, A. (2002). Effect of blood transfusion on lipid peroxidation in preterm infants. Arch. Dis. Child. Fetal Neonatal Ed. 86, F46–F48. doi: 10.1136/fn.86.1.F46
Wardle, S., Hughes, A., Chen, S., and Shaw, N. (2001). Randomised controlled trial of oral vitamin A supplementation in preterm infants to prevent chronic lung disease. Arch. Dis. Child. Fetal Neonatal Ed. 84, F9–F13. doi: 10.1136/fn.84.1.F9
Warner, A., Bencosme, A., Healy, D., and Verme, C. (1995). Prognostic role of antioxidant enzymes in sepsis: preliminary assessment. Clin. Chem. 41, 867–871.
Watanabe, N., Sakota, D., Ohuchi, K., and Takatani, S. (2007). Deformability of red blood cells and its relation to blood trauma in rotary blood pumps. Artif. Organs 31, 352–358. doi: 10.1111/j.1525-1594.2007.00392.x
Weber, T. R., Connors, R. H., Tracy, T. F., Bailey, P. V., Stephens, C., and Keenan, W. (1990). Prognostic determinants in extracorporeal membrane oxygenation for respiratory failure in newborns. Ann. Thorac. Surg. 50, 720–723. doi: 10.1016/0003-4975(90)90669-W
Wendel, H. P., Scheule, A. M., Eckstein, F. S., and Ziemer, G. (1999). Haemocompatibility of paediatric membrane oxygenators with heparin-coated surfaces. Perfusion 14, 21–28. doi: 10.1177/026765919901400104
West, A. P., Brodsky, I. E., Rahner, C., Woo, D. K., Erdjument-Bromage, H., Tempst, P., et al. (2011). TLR signalling augments macrophage bactericidal activity through mitochondrial ROS. Nature 472, 476–480. doi: 10.1038/nature09973
Wiesenack, C., Liebold, A., Philipp, A., Ritzka, M., Koppenberg, J., Birnbaum, D. E., et al. (2004). Four years’ experience with a miniaturized extracorporeal circulation system and its influence on clinical outcome. Artif. Organs 28, 1082–1088. doi: 10.1111/j.1525-1594.2004.00030.x
Williams, D. C., Turi, J. L., Hornik, C. P., Bonnadonna, D. K., Williford, W. L., Walczak, R. J., et al. (2015). Circuit oxygenator contributes to extracorporeal membrane oxygenation-lnduced hemolysis. ASAIO J. 61, 190–195. doi: 10.1097/MAT.0000000000000173
Wilson, J. X. (2009). Mechanism of action of vitamin C in sepsis: ascorbate modulates redox signaling in endothelium. Biofactors 35, 5–13. doi: 10.1002/biof.7
Winterbourn, C. C., Buss, I. H., Chan, T. P., Plank, L. D., Clark, M. A., and Windsor, J. A. (2000). Protein carbonyl measurements show evidence of early oxidative stress in critically ill patients. Crit. Care Med. 28, 143–149. doi: 10.1097/00003246-200001000-00024
Winterbourn, C. C., and Hampton, M. B. (2008). Thiol chemistry and specificity in redox signaling. Free Radic. Biol. Med. 45, 549–561. doi: 10.1016/j.freeradbiomed.2008.05.004
Witko-Sarsat, V., Friedlander, M., Capeillère-Blandin, C., Nguyen-Khoa, T., Nguyen, A. T., Zingraff, J., et al. (1996). Advanced oxidation protein products as a novel marker of oxidative stress in uremia. Kidney Int. 49, 1304–1313. doi: 10.1038/ki.1996.186
Wolf, A., Remp, D. G., Kiley, J. E., and Currie, G. D. (1951). Artificial kidney function: kinetics of hemodialysis. J. Clin. Invest. 30, 1062–1070. doi: 10.1172/JCI102526
Wołonciej, M., Milewska, E., and Roszkowska-Jakimiec, W. (2016). Trace elements as an activator of antioxidant enzymes. Postepy Hig. Med. Dosw. 70, 1483–1498. doi: 10.5604/17322693.1229074
Wu, J. H., Marchioli, R., Silletta, M. G., Masson, S., Sellke, F. W., Libby, P., et al. (2015). Oxidative stress biomarkers and incidence of postoperative atrial fibrillation in the omega-3 fatty acids for prevention of postoperative atrial fibrillation (OPERA) Trial. J. Am. Heart Assoc. 4:e001886. doi: 10.1161/JAHA.115.001886
Xia, W., Han, J., Huang, G., and Ying, W. (2010). Inflammation in ischaemic brain injury: current advances and future perspectives. Clin. Exp. Pharmacol. Physiol. 37, 253–258. doi: 10.1111/j.1440-1681.2009.05279.x
Xu, J., Woods, C. R., Mora, A. L., Joodi, R., Brigham, K. L., Iyer, S., et al. (2007). Prevention of endotoxin-induced systemic response by bone marrow-derived mesenchymal stem cells in mice. Am. J. Physiol. Lung Cell. Mol. Physiol. 293, L131–L141. doi: 10.1152/ajplung.00431.2006
Yamamoto, M., Yang, G., Hong, C., Liu, J., Holle, E., Yu, X., et al. (2003). Inhibition of endogenous thioredoxin in the heart increases oxidative stress and cardiac hypertrophy. J. Clin. Invest. 112, 1395–1406. doi: 10.1172/JCI200317700
Yamawaki, H., and Berk, B. C. (2006). Thioredoxin in the cardiovascular system. J. Mol. Med. 84, 997–1003. doi: 10.1007/s00109-006-0109-6
Yang, C.-C., Hsu, S.-P., Wu, M.-S., Hsu, S.-M., and Chien, C.-T. (2006). Effects of vitamin C infusion and vitamin E-coated membrane on hemodialysis-induced oxidative stress. Kidney Int. 69, 706–714. doi: 10.1038/sj.ki.5000109
Yang, S.-K., Xiao, L., Xu, B., Xu, X.-X., Liu, F.-Y., and Sun, L. (2014). Effects of vitamin E-coated dialyzer on oxidative stress and inflammation status in hemodialysis patients: a systematic review and meta-analysis. Ren. Fail. 36, 722–731. doi: 10.3109/0886022X.2014.890858
Yin, H., Xu, L., and Porter, N. A. (2011). Free radical lipid peroxidation: mechanisms and analysis. Chem. Rev. 111, 5944–5972. doi: 10.1021/cr200084z
Zakkar, M., Ascione, R., James, A., Angelini, G., and Suleiman, M. (2015a). Inflammation, oxidative stress and postoperative atrial fibrillation in cardiac surgery. Pharmacol. Ther. 154, 13–20. doi: 10.1016/j.pharmthera.2015.06.009
Zakkar, M., Guida, G., Suleiman, M., and Angelini, G. D. (2015b). Cardiopulmonary bypass and oxidative stress. Oxid. Med. Cell. Longev. 2015:189863. doi: 10.1155/2015/189863
Zimmerman, G. A., Mcintyre, T. M., and Prescott, S. M. (1985). Thrombin stimulates the adherence of neutrophils to human endothelial cells in vitro. J. Clin. Invest. 76, 2235–2246. doi: 10.1172/JCI112232
Zusterzeel, P., Rütten, H., Roelofs, H., Peters, W., and Steegers, E. (2001). Protein carbonyls in decidua and placenta of pre-eclamptic women as markers for oxidative stress. Placenta 22, 213–219. doi: 10.1053/plac.2000.0606
Keywords: antioxidants, cardiopulmonary bypass, CRRT, ECMO, extracorporeal circulation, oxidative stress
Citation: Raffaeli G, Ghirardello S, Passera S, Mosca F and Cavallaro G (2018) Oxidative Stress and Neonatal Respiratory Extracorporeal Membrane Oxygenation. Front. Physiol. 9:1739. doi: 10.3389/fphys.2018.01739
Received: 17 September 2018; Accepted: 19 November 2018;
Published: 04 December 2018.
Edited by:
Murugesan Velayutham, University of Pittsburgh, United StatesReviewed by:
Hitesh Singh Sandhu, The University of Tennessee Health Science Center (UTHSC), United StatesAna Denicola, Universidad de la República, Uruguay
Copyright © 2018 Raffaeli, Ghirardello, Passera, Mosca and Cavallaro. This is an open-access article distributed under the terms of the Creative Commons Attribution License (CC BY). The use, distribution or reproduction in other forums is permitted, provided the original author(s) and the copyright owner(s) are credited and that the original publication in this journal is cited, in accordance with accepted academic practice. No use, distribution or reproduction is permitted which does not comply with these terms.
*Correspondence: Giacomo Cavallaro, Z2lhY29tby5jYXZhbGxhcm9AbWFuZ2lhZ2FsbGkuaXQ=