- Key Laboratory of Integrated Pest Management in Crops, Ministry of Agriculture, Sino-American Biological Control Laboratory, USDA-ARS/Institute of Plant Protection, Chinese Academy of Agricultural Sciences, Beijing, China
Aphidius gifuensis Ashmead (Hymenoptera: Aphidiidae) is a solitary endoparasitoid used in the biological control of various aphids. Diapause plays an important role in the successful production and deployment of A. gifuensis. Diapause can effectively extend the shelf life of biological control agents and solve several practical production problems like long production cycles, short retention periods, and discontinuities between supply and demand. In recent years, studies have been conducted on the environmental regulation and physiological and biochemical mechanisms of diapause in A. gifuensis. Nevertheless, the molecular mechanism of diapause in this species remains unclear. In this study, we compared the transcriptomes and proteomes of diapause and non-diapause A. gifuensis to identify the genes and proteins associated with this process. A total of 557 transcripts and 568 proteins were differentially expressed between the two groups. Among them, (1) genes involved in trehalose synthesis such as glycogen synthase, glycogen phosphorylase, and trehalose 6-phosphate synthase were upregulated in diapause at mRNA or protein level while glycolysis and gluconeogenesis-related genes were downregulated, suggesting that A. gifuensis stores trehalose as an energy resource and cryoprotectant; (2) the expression of immune-related genes like C-type lectins, hemocyanin, and phenoloxidase was increased, which helps to maintain immunity during diapause; (3) a chitin synthase and several cuticular protein genes were upregulated to harden the cuticle of diapausing A. gifuensis larval. These findings improve our understanding of A. gifuensis. diapause and provide the foundation for further pertinent studies.
Introduction
Aphidius gifuensis Ashmead is widely distributed in East Asian countries (Tang and Chen, 1984; Nakata, 1995). It is a common parasitoid of many aphid species. This wasp has high parasitic capacity and adaptability and has been tested as a biological control agent against the aphids Myzus persicae (Sulzer), Sitobion avenae (Fabricius), and Aphis gossypii (Glover) (Pan and Liu, 2014; Khan et al., 2016). It is thought that the release of A. gifuensis in greenhouses or open fields might control aphid populations and prevent catastrophic crop damage. In practice, A. gifuensis has already been used to control M. persicae and has proven to be highly effective (Yang et al., 2009).
Diapause is a form of dormancy that occurs before environmental conditions become unfavorable for development. It ends when favorable environmental conditions return (Denlinger and Armbruster, 2014). The diapause process consists of six phases: induction, preparation, initiation, maintenance, termination, and post-diapause development (Kostál, 2006). When insects enter diapause, their metabolic rates are depressed and their nutrient reserves are increased. In this way, they can survive predictable adverse conditions and synchronize their life cycles with the seasons conducive to growth, development, and reproduction (Hahn and Denlinger, 2011). In general, insect diapause is induced by the low temperatures and short photoperiods of late autumn in preparation for overwintering. However, several insect species enter diapause in summertime to avoid heat and drought (Saulich and Musolin, 2017). Other insects can enter both summer and winter diapause, which results in differential voltinism (Takeda, 1998; Xue et al., 2002; Ding et al., 2003; Ren et al., 2018). Diapause is of great importance in pest management because it enables the prediction of pest emergence, facilitates pest control planning, and increases the shelf life and utility of biological control agents (Denlinger, 2008). In recent years, insect diapause research has elucidated its environmental regulation (Saunders, 2014), maternal effect (Voinovich et al., 2016), energetics (Hahn and Denlinger, 2011), hormonal control (Denlinger et al., 2012), and molecular mechanisms (Hand et al., 2016).
It is believed that the parasitoid wasp A. gifuensis does not enter diapause and can even develop in winter (Ohta and Ohtaishi, 2006). In China, however, several scientists found that A. gifuensis may enter diapause as mature larvae at low temperatures and short day lengths (Li et al., 1999; Xu et al., 2016). Our laboratory successfully induced diapause in A. gifuensis at 8°Cand an 8 h light:16 h dark (L8:D16) photoperiod. We found that temperature played a more vital role than photoperiod in diapause induction (Li et al., 2013). When they enter diapause, the fourth instar larvae turn from white to mustard yellow. This body color change is an important identifying characteristic of diapause in A. gifuensis (Figure 1). Diapausing A. gifuensis have higher total sugar and lower protein levels than non-diapausing individuals. Therefore, A. gifuensis can accumulate nutrients and protective materials when they enter diapause (Li, 2011 ). Nevertheless, the molecular processes occurring in A. gifuensis diapause have not yet been determined.
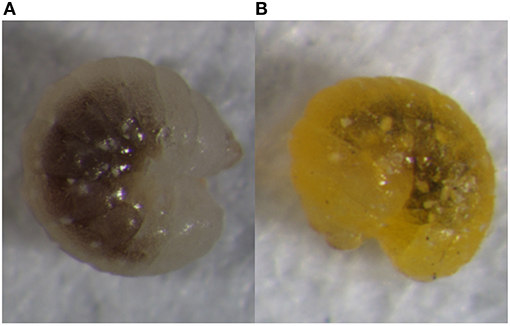
Figure 1. Developing (A) and diapausing (B) fourth-instar larvae of A. gifuensis. Of note is the substantial body color difference between these two states. The raw files of these pictures are shown as Figures S1, S2.
In this study, we used high-throughput mRNA sequencing (RNA-seq) and isobaric tags for relative and absolute quantification (iTRAQ) technology to analyze the transcriptomes and proteomes, respectively, in A. gifuensis diapause (D) and non-diapause (ND) fourth instar larvae. We compared their relative gene and protein expression levels. We determined the functions of their differentially expressed genes (DEGs) and proteins (DEPs) using the gene ontology (GO) and Kyoto Encyclopedia of Genes and Genomes (KEGG) databases. We then compared transcriptome and proteome data between the D and ND groups and selected the DEGs with either the same or inverse change trends. We also ran quantitative real-time PCR (qRT-PCR) to verify the accuracy of the transcriptome analyses.
Methods
Insect Rearing and Treatment
The green peach aphid M. persicae was reared on tobacco (Nicotiana tabacum) seedlings and used as a host for the aphid parasitoid wasp A. gifuensis. The tobacco, aphids, and parasitoid wasps were obtained from Langfang Pilot Scale Base, Institute of Plant Protection, Chinese Academy of Agricultural Sciences, Hebei Province, China. The aphids were reared in homemade cages at 25°C, 80% RH, and L16:D8. Adult A. gifuensis were released at a ratio of one parasitoid to 100 aphids. Daily observations were made and recorded. Mummies were dissected and fourth instar larvae were used as ND samples. Certain aphids were transferred to diapause-inducing conditions (8°C, RH 80%, and L8:D16) 3 d after being parasitized. Once again, mummies were dissected and fourth instar larvae were used as D samples.
RNA Preparation, cDNA Library Construction, and Illumina Sequencing
Three replications were performed under each treatment and 50 larvae were used in each replication. Total RNA was extracted using RNAiso Plus Total RNA extraction reagent (TaKaRa Bio Inc., Kusatsu, Shiga, Japan) following the manufacturer's instructions. An Agilent Bioanalyzer 2100 (Agilent Technologies, Santa Clara, CA, USA) and the RIN number were used to evaluate RNA integrity. Qualified total RNA was further purified with the RNeasy micro kit (Qiagen, Hilden, Germany) and the RNase-Free DNase Set (Qiagen, Hilden, Germany). Total RNA was extracted and oligo (dT) magnetic beads and fragmentation buffer were used to generate short fragments of the isolated poly(A) mRNA. First-strand cDNA was generated using SuperScript II Reverse Transcriptase (Invitrogen, Carlsbad, CA, USA) with these short fragments and random hexamer primers. Second-strand cDNA synthesis was performed using the Second Strand cDNA Synthesis Kit (Beyotime, Shanghai, China). The adapter was ligated immediately after repair of the adenylated 3′ ends and purification with a QIAquick PCR purification kit (Qiagen, Hilden, Germany). A cDNA template enrichment was required for library QC. Concentration and library size were assessed using a Qubit 2.0 fluorometer (Invitrogen, Carlsbad, CA, USA) and an Agilent 2100 Bioanalyzer (Agilent Technologies, Santa Clara, CA, USA), respectively. Three biological replicates of the non-diapause and diapause samples were sequenced with an Illumina HiSeq 2500 platform (Illumina, San Diego, CA, USA). Paired-end sequencing was controlled with the data collection software provided by Illumina using real-time data analysis.
Transcript Identification and Quantification
The cDNA sequencing was performed using the Illumina HiSeq 2500 Sequencing System (Illumina, San Diego, CA, USA) according to the manufacturer's instructions. The clean reads were filtered from the raw reads using fastx v. 0.0.13 (http://hannonlab.cshl.edu/fastx_toolkit/index.html) to eliminate ribosomal RNA reads, adapter sequences, reads with more than 20% low quality bases, reads shorter than 50 bp, and reads with an N (unknown sequences) ratio>5% (Patel and Jain, 2012). De novo clean read assembly was performed using the scaffolding contig algorithm of CLC Genomics Workbench v. 6.0.4 according to the sequencing data of six samples (Bräutigam et al., 2011; Garg et al., 2011; Su et al., 2011). The primary unigenes obtained from the de novo assembly were then expressed sequence tag (EST)-assembled by CAP3. The final unigenes were then searched against the UniProt and Nr database with BLASTx. The best hits from the database were selected as unigene annotations. For quantitative gene expression analysis, reads from each sample were mapped to the final unigenes which acted as reference sequences. The read counts were normalized by trimmed mean M values (TMM; Robinson and Oshlack, 2010), and then a standard calculation was performed based on reads per kilobase of transcript per million mapped reads (RPKM; Mortazavi et al., 2008). The expressions of transcripts are summarized in Table S1.
Protein Extraction
Three replicates (50 larvae for each replication) were used for the D and ND treatments. Samples were quickly ground into a very fine powder in liquid nitrogen and added to centrifuge tubes containing 1 ml ice-cold BPP buffer [100 mm Tris, 100 mm ethylenediaminetetraacetic acid (EDTA), 50 mm borax, 50 mm vitamin C, 1% PVPP w/v, 1% Triton X-100 v/v, 2% β-mercaptoethanol v/v and 30% sucrose w/v, pH 8.0]. After the suspension was vortexed at room temperature for 10 min, 800 μl Trissaturated Phe were added and further vortexed for 10 min. The homogenates were then centrifuged at 15,000 × g and 4°C for 15 min. The supernatants were then transferred to new centrifuge tubes (200 μl supernatants per tube). 1ml ammonium sulfate saturated-methanol was added to each tube and then the mixture was incubated over night at −20°C. After being spun at 15,000 × g and 4°C for 15 min, the precipitates were then re-suspended in 500 μl ice-cold methanol, and then centrifuged at 15,000 × g and 4°C for 5 min. 500 μl ice-cold methanol acetone was added to the precipitates and centrifuged as described above. The protein precipitates were air-dried at room temperature and dissolved in 200 μl lysis buffer [50 mm Tris, 1 mm phenylmethanesulfonyl fluoride (PMSF), 2 mm ethylenediaminetetraacetic acid (EDTA), and 2 mm dithiothreitol (DTT), pH 7.4] at 22°C for more than 2 h. The homogenates were then centrifuged at 17,000 × g and 20°C for 30 min. The protein samples (supernatant) were packed into 1.5-ml microcentrifuge tubes and stored at −80°C. Protein concentrations were determined by the Bradford method (Bradford, 1976). Each treatment included three biological and three technical repetitions.
ITRAQ LABELING and UPLC-QTOF-MS/MS Analysis
200 μg protein from each sample solution was transferred to the centrifuge tube and diluted to 125 μl with 8 M UA buffer (8 M urea and 150 mm Tris-HCl, pH 8.0). 5 μl 1M DTT was added to the homogenates which were then incubated at 37°C. After 1 h, 20 μl1 M iodoacetamide (IAA) were added to each centrifuge tube. These were incubated for 1 h in a dark room. The samples were then transferred to an ultrafiltration centrifuge tube and spun at 14,000 × g and room temperature for 20 min. The supernatants were then discarded. 100 μl 1M UA buffer was added to an ultrafiltration centrifuge tube and spun at 14,000 × g room temperature for 10 min. This step was repeated twice. One hundred microliters of dissolution buffer (AB SCIEX, Framingham, MA, USA) was added to an ultrafiltration centrifuge tube and spun at 14,000 × g room temperature for 20 min. This step was repeated three times. Samples were then transferred to new collection tubes. Protein samples were digested at 37°C overnight with sequencing-grade trypsin (Worthington Biochemical, Lakewood, NJ, USA) at a protein:trypsin ratio of 50:1. After centrifugation at 14,000 × g room temperature for 20 min, the peptides were collected and the remaining homogenates were recentrifuged with 50 μl dissolution buffer. The filtrates were pooled with those from the previous step. The iTRAQ labeling was performed with an iTRAQ Reagent-8plex Multiplex Kit (AB SCIEX, Framingham, MA, USA) according to the manufacturer's protocol. Six samples (three replicates each for ND and D) were labeled with iTRAQ reagent as follows: 113 (ND1), 114 (ND2), 115 (ND3), 116 (D1), 117 (D2), and 118 (D3). After labeling, the peptides were fractionated by reversed phase liquid chromatography (RPLC) on a Gemini-NX C18 (4.6 mm × 150 mm, 5 μm; Phenomenex, Aschaffenburg, Germany) to remove any remaining iTRAQ or other reagents. MS was performed using an AB SCIEX TripleTOFTM 5600(AB SCIEX, Framingham, MA, USA) coupled online with the LC-20AD Nano-HPLC system (Shimadzu Corp., Kyoto, Japan). The gas setting of the MS was as follows: curtain gas = 35 kPa; Gas1 = 4; Gas2 = 0. The ion spray floating voltage was 2,300 V and the collision energy voltage used for collision-induced dissociation (CID) fragmentation for MS/MS spectra acquisitions was 80 V. Each cycle consisted of a TOF-MS spectrum acquisition for 250 ms. The mass-to-charge ratio (m/z) scan range was 350-1,250 Da. There were 30 information-dependent acquisitions (IDA; m/z = 100-1,500 Da). The accumulation time of each IDA was 0.1 s. Masses were dynamically excluded for 25 s when MS/MS fragment spectra were acquired for them. MS was recalibrated at the start of each sample with a β-galactosidase digest standard.
Protein Identification and Quantification
Protein identification and quantification were performed with ProteinPilot 4.5 (AB SCIEX, Framingham, MA, USA). Raw MS/MS data were searched against the UniProt database. Peptides with unused scores ≥1.3 (corresponding to a confidence limit of 95%) were accepted. Predicted proteins with ≥1 unique peptide and a global false discovery rate (FDR) ≤ 1% were counted as identified. The quantitative result was outputted as ratios of proteins in every labeled sample to it in sample 113. A statistical comparison of the differences in protein expression between diapaused and non-diapaused A. gifuensis was conducted with one-way ANOVA in SPSS v. 13.0 (IBM Corp., Armonk, NY, USA). P-values were calibrated by the Benjamini method (Benjamini and Hochberg, 1995).
Bioinformatics Analysis
The identified transcripts and proteins were classified according to the Gene Ontology (GO; Ashburner et al., 2000) database using BLAST (E-value < 1e-5). KAAS (http://www.genome.jp/tools/kaas/) was used to map gene and protein pathways based on the Kyoto Encyclopedia of Genes and Genomes (KEGG; Kanehisa and Goto, 2000) database. Enrichment analyses were performed on the differentially expressed genes (DEGs) and differentially expressed proteins (DEPs) based on the GO and KEGG annotations. DAVID v. 6.7 (https://david-d.ncifcrf.gov/) was used for these analyses.
Quantitative Real-Time PCR
The qRT-PCR was carried out in a 7,900 HT Sequence Detection System (Applied Biosystems, Foster City, CA, USA) using the ABI Power SYBR Green PCR Master Mix (Applied Biosystems, Foster City, CA, USA). Glyceraldehyde-3-phosphate dehydrogenase (GAPDH) was used as a reference gene for relative target gene quantification. The target genes included the upregulated fatty acid synthase (FAS), gamma-interferon-inducible-lysosomal thiol reductase (GILT), cuticle protein 21 (CP21), uncharacterized protein LOC103571003, serine protease homolog 21 precursor (SP21), trehalose phosphate synthase (TPS), troponin C (TnC), sodium/potassium-transporting ATPase (AT1A), and the downregulated venom carboxylesterase-6 (EST6). The primer sequences of GAPDH and the nine target genes are listed in Table 1. Nine replicates (three biological replicates × three technical replicates) were used per sample. The qRT-PCR conditions were as follows: incubation at 50°C for 2 min and 95°C for 10 min followed by 40 cycles of 95°C for 15 s and 60°C for 1 min. To detect non-specific product amplification, the melting curve was analyzed from 60°C to 95°C. The amplification efficiency was automatically calculated using SDS v. 2.4 (Applied Biosystems, Foster City, CA, USA). Relative quantification of the target gene transcripts was determined by the comparative CT method 2−ΔΔCt (Livak and Schmittgen, 2001).
Results
Summary of Transcriptome Data
The RNA-seq experiment was performed on both non-diapaused and diapaused A. gifuensis according to the data obtained by sequencing with the Illumina HiSeq 2,500 platform. Using three biological replicates of two samples, the six libraries yielded 230,886,306 raw reads comprising 154,790,202 for the ND group and 145,249,536 for the D group. After quality filtering and fragment assembly, 47,077 unigenes were obtained. The assembled unigenes were mostly 200-1,000 bp long (70.49%). Another 17.47% were 1,001-2,000 bp long and 12.05% of them were >2,000 bp. Transcriptome data were deposited in the Sequence Read Archive (SRA, https://www.ncbi.nlm.nih.gov/sra) of the National Center for Biotechnology Information (NCBI) under the accession numbers SRR3717246 (ND) and SRR3717412 (D). Transcriptome datasets are also available at NCBI project PRJNA326701 under the accession number SRP077084.
We annotated the unigenes by aligning their sequences against the UniProt database with BLASTX (Altschul et al., 1990). There were 24,431 unigenes matched with a high homology (E-value < 1e-5; Table S2). Since the genome sequence of A. gifuensis was not yet determined, we performed a sequence alignment with known genomes of other species to establish whether the unigene belonged to A. gifuensis. BLAST results showed the greatest number of hits for Apis mellifera (19.03%) and Nasonia vitripennis (18.36%), followed by Cerapachys biroi (10.60%), Harpegnathos saltator (9.47%), Camponotus floridanus (8.37%), and Acromyrmex octospinosus (5.39%). Another 9.48% of the unigenes were found for species not belonging to the Hymenoptera (Figure 2A). These were treated as contaminants and filtered out.
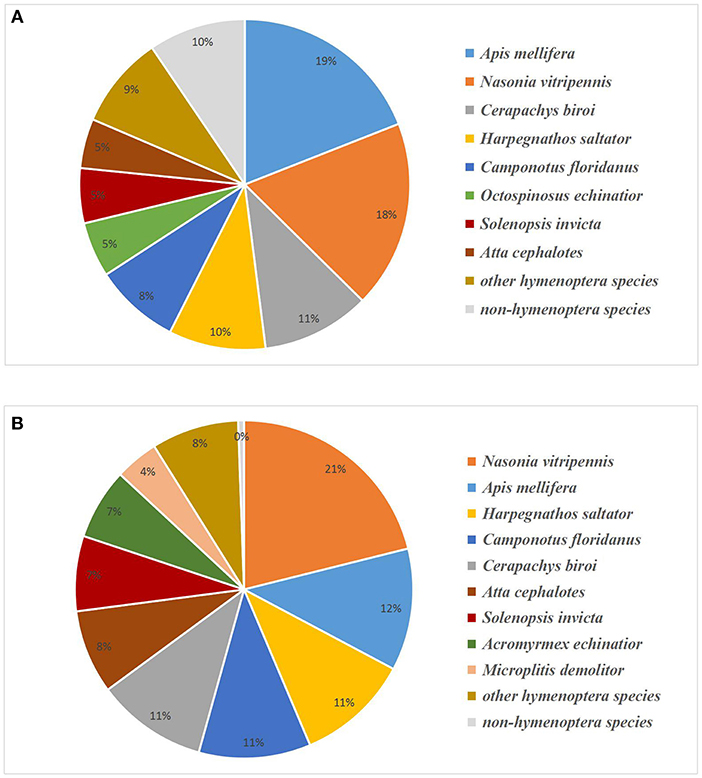
Figure 2. Species distribution of BLASTX hits of experimental unigenes (A) and proteins (B). Unigenes or proteins homologous to Hymenoptera were assumed to belong to A. gifuensis.
In the differential expression analysis, transcripts with ≥ 1.25-fold change between ND and D and FDR (false discovery rate) ≤ 0.05 were considered DEGs. The expression levels of 557 transcripts were significantly changed; 389 were upregulated and 168 were downregulated.
Protein Identification and Quantification
We identified 4,931 proteins from a total of 30,332 unique peptides using ProteinPilot v. 4.5. All of them had ≥1 unique peptide and 80.17% of them had ≥2 unique peptides. Of these, 2,852 proteins were quantified with iTRAQ ratios (Table S3). Species annotation showed that most of the matches belonged to Nasonia vitripennis (21.11%), Apis mellifera (11.68%), Harpegnathos saltator (10.83%), Camponotus floridanus (10.69%), and Cerapachys biroi (10.59%). Another 0.56% of the proteins were filtered out as contaminants (Figure 2B). The proteome datasets are available at Peptide Atlas (http://www.peptideatlas.org) under submission number PASS00902.
Correlations between biological replicates were evaluated before the differential expression analysis. Sample 113, which acted as the reference sample in protein quantification, was used as the denominator. The ratios from same treatment (ND or D) were log-transformed and plotted against each other. All correlations between the two biological replicates were >0.6 (Figure 3). Therefore, reproducibility among the three replicates per treatment was satisfactory. Proteins with the same trend in all six comparisons and with average ≥1.25-fold change and FDR ≤ 0.05 were deemed DEPs. There were 253 upregulated and 315 downregulated DEPs.
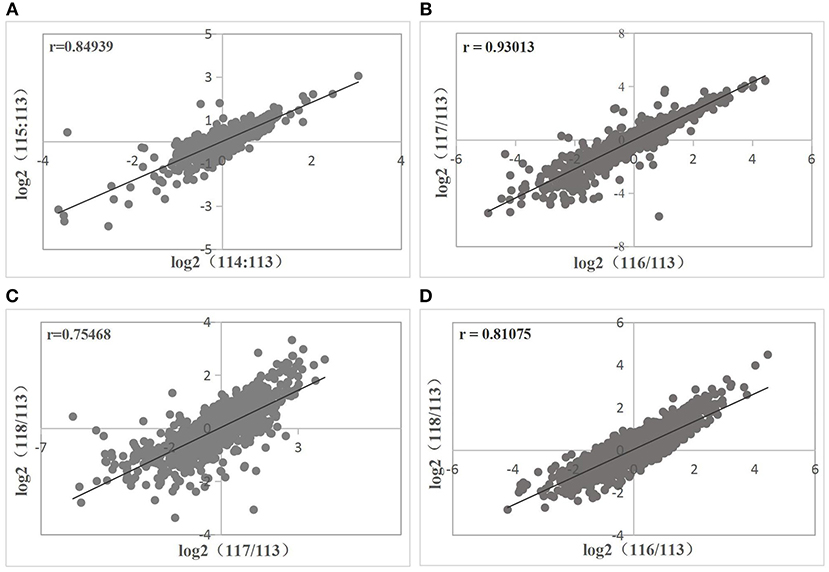
Figure 3. Correlations between biological replicates in ND group (A) and D group (B–D). Correlations are described by the Pearson correlation coefficient r, where r > 0.6 means a strong correlation between two sets of variates.
Correlation Analysis of Transcriptome and Proteome Data
There were 2,527 out of 2,852 proteins matching the corresponding genes by sequence alignment. The expression change ratios (D/ND) between transcriptome and proteome level were compared with correlation analysis. The result showed a low Pearson correlation coefficient between these two levels (r = 0.0046; Figure 4). All DEPs with ≥1.25-fold change matched their corresponding genes, while most of them did not significantly change. As shown in Table 2, 28 of the DEPs had the same change trend as their corresponding DEGs. Of these, 25 were upregulated and 3 were downregulated. Twelve DEPs had change trends that were the inverse of those for their corresponding DEGs.
Functional Analysis of Differentially Expressed Transcripts and Proteins
To explore the biochemical reactions involved in A. gifuensis diapause, an enrichment analysis based on GO annotations was performed using hypergeometric testing to categorize the DEPs and DEGs. There were 168 (62 biological processes, 94 molecular functions, and 12 cellular components) and 167 (53 biological processes, 86 molecular functions, and 28 cellular components) enriched GO terms. These classified 152 DEGs and 181 DEPs, respectively. One gene or protein could have >1 GO annotation (Tables S4, S5). The same enrichment analysis was performed based on the KEGG annotation. At the mRNA level, 80 differentially expressed genes mapped to 143 pathways (Table S6). The greatest number of DEGs were linked to “metabolic pathways,” (20) followed by “biosynthesis of secondary metabolites” (12) and “biosynthesis of antibiotics” (11). At the protein level, eight differentially expressed proteins (DEPs) mapped to 37 pathways (Table S7). The pathways with the most DEPs were “metabolic pathways,” (7) “biosynthesis of secondary metabolites,” (6) and “glycolysis/gluconeogenesis” (4). The aforementioned results suggest that glycometabolism, immunoreaction, and secondary metabolite accumulation play important roles in diapause.
Validation of Differentially Expressed Genes Using QRT-PCR
A qRT-PCR analysis was performed to investigate the transcriptional patterns of nine selected genes. As shown in Figure 5, the expression levels of all target genes were consistent between the qRT-PCR and RNA-seq data. This showed that the quality of transcriptome data was reliable.
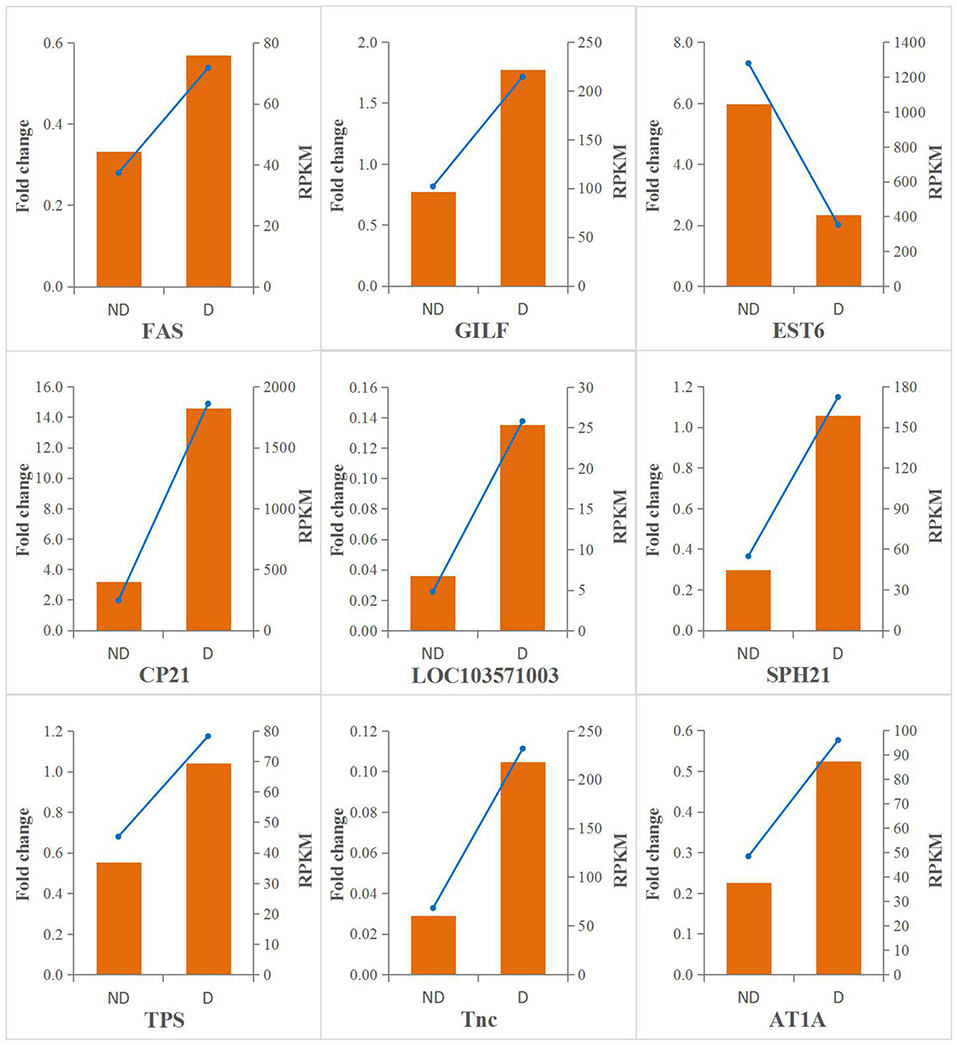
Figure 5. qRT-PCR validation of differentially expressed genes. The qRT-PCR results are shown in orange, and the values are shown on the left y-axis; the R result are shown with blue lines, and the value correspond to the right y-axis.
Discussion
Metabolite Accumulation in Diapause
During diapause, an insect may feed little or not at all. For this reason, insects must store nutrients in preparation for diapause (Hahn and Denlinger, 2007). Certain metabolites like polyhydric alcohols and sugars can also serve as cryoprotectants that improve cold endurance in diapausing insects (Block, 1990). The insulin-signaling pathway may be a major player in diapause metabolite regulation (Hahn and Denlinger, 2011). The transcriptome analysis showed that the expression of the 3-phosphoinositide-dependent protein kinase-1 (PDK1) gene (PDK1, contig_31661) was upregulated 4.08-fold in the D group. PDK1 is an indispensable component of the PI3K-AKT signaling axis which, in turn, is a part of the insulin-signaling pathway. PDK1 phosphorylates and activates serine/threonine-protein kinase (AKT), which regulates glucose homeostasis (Sarbassov et al., 2005). The forkhead of the transcription factor Foxo1 is downstream of AKT and may regulate glucose homeostasis in insects (Sim and Denlinger, 2013). In the nucleus, FOXO1 binds the promoter of the gene encoding phosphoenolpyruvate carboxykinase (PEPCK) and activates the expression of this enzyme (Puigserver et al., 2003). Activated AKT phosphorylates FOXO1. Thence, FOXO1 is exported from the nucleus and retained in the cytoplasm, and target gene expression is repressed (Biggs et al., 1999). PEPCK converts oxaloacetate to phosphoenolpyruvate. This reaction is generally considered to be the first committed step in gluconeogenesis (Rognstad, 1979). At the mRNA level, the expression of PEPCK (contig_30482) was halved in the D group. Therefore, gluconeogenesis was probably inhibited in them. Another important gene downstream from AKT is glycogen synthase kinase 3 beta (GSK3β), which is involved in glycogen biosynthesis and metabolism. GSK3β inhibits glycogen synthase (GYS) by phosphorylating it. In this way, it inhibits glycogen biosynthesis (Cohen and Frame, 2001). By inhibiting GSK3β, AKT can overcome this repression and increase GYS activity (Beaulieu et al., 2009). In this study, GSK3β (K7JBD0_NASVI) was downregulated at the protein level and GYS (contig_31002) was upregulated at the mRNA level in the D group. Following this, glycogen synthesis was increased during A. gifuensis diapause.
It is generally accepted that glycogen is converted into polyhydric alcohols or trehalose in diapausing insects (Hayakawa and Chino, 1982). This transformation starts with phosphorolysis catalyzed by glycogen phosphorylase (GP). The product is D-glucose-1-phosphate (Steel, 1982). There are two alternative metabolic pathways for D-glucose-1-phosphate. In one scenario, it is converted into D-glucose-6-phosphate via phosphoglucomutase (PGM). In turn, D-glucose-6-phosphate may be transformed via glycolysis into pyruvate which would enter the TCA cycle. Glycerol and sorbitol may also be produced by glycolysis in diapausing insects (Chion, 1958). Contrarily, D-glucose-1-phosphate could be converted into trehalose via UTP-glucose-1-phosphate uridylyltransferase (UGP), trehalose 6-phosphate synthase (TPS), and trehalose 6-phosphate phosphatase (TPP). Several studies have shown that independent TPP genes were absent in insects whereas there were two conserved regions corresponding to TPS and TPP, respectively (Cui and Xia, 2009; Xu et al., 2009; Tang et al., 2010). In the D group, the expression levels of GP (contig_3475) and TPS (contig_3499) were 1.29-fold and 1.73-fold upregulated, respectively, while the protein pgm (K7IN84_NASVI) was downregulated 0.80-fold. Therefore, we speculate that diapausing A. gifuensis decreases energy expenditure and stores nutrients in the form of trehalose rather than glycerol or sorbitol.
Several insects like Colaphellus bowringi (Tan et al., 2017), Pieris napi (Lehmann et al., 2016), Arimania comaroffi (Bemani et al., 2012), and Ectomyelois ceratoniae (Heydari and Izadi, 2014) accumulate lipids in diapause whereas others like Cymbalophora pudica (Kostál et al., 1998) and Eurytoma amygdali (Khanmohamadi et al., 2016) do not. In group D, fatty acid synthase (FAS, K7IM26_NASVI) and acetyl-CoA carboxylase (ACC, E2B9B3_HARSA) were non-significantly downregulated at the protein level (0.88- and 0.89-fold change, respectively). The result suggested that A. gifuensis relies on sugars like trehalose rather than lipids as energy sources and cryoprotectants in diapause. The aforementioned signals and metabolic processes are summarized in Figure 6.
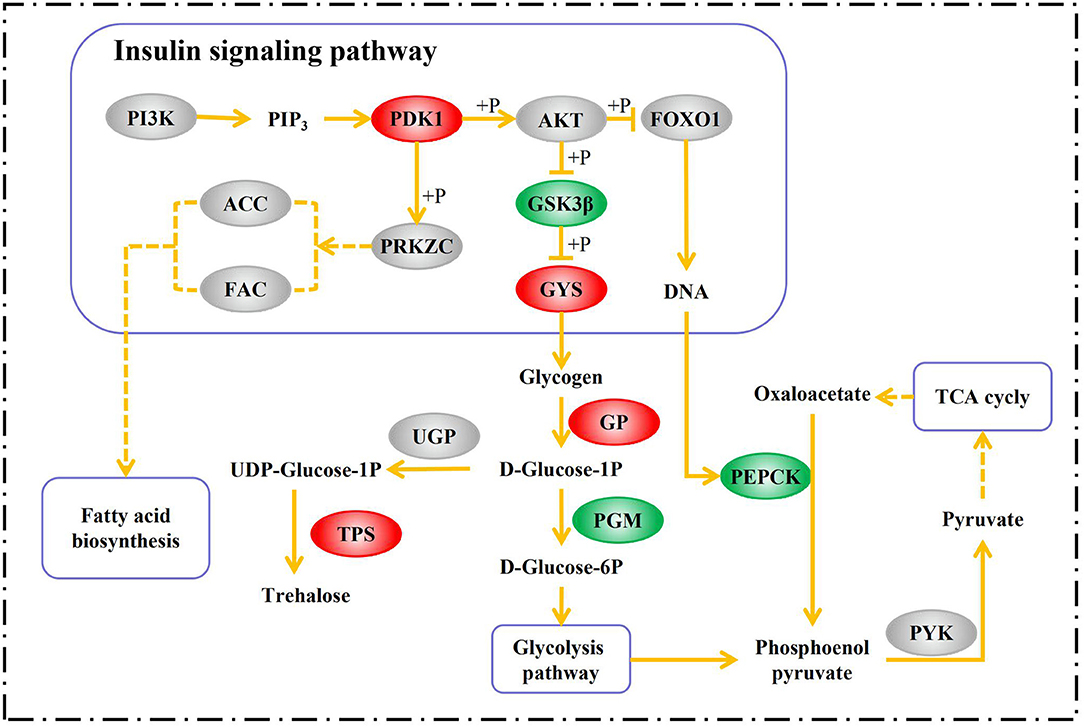
Figure 6. Summary of the pathways involved in metabolite accumulation in A. gifuensis diapause. Red boxes indicate genes/proteins upregulated in the D group relative to the ND group. Green boxes indicate genes/proteins downregulated in the D group compared with the ND group. Gray boxes indicate genes/proteins not differently expressed in the D group in comparison with the ND group. PI3K, phosphatidylinositol-4,5-bisphosphate 3-kinase; PIP3, Phosphatidylinositol-3,4,5-trisphosphate; PRKZC, atypical protein kinase C zeta type; PYK, pyruvate kinase.
Immune Responses in Diapause
Comparatively little research has been conducted on immune responses in diapausing insects. Metabolic and developmental retardation in diapause are expected to weaken insect immune systems. However, diapause must not lower insect defenses against pathogens if it is to ensure insect survival under harsh environmental conditions. A report on Samia cynthia suggested that immunity continues to work well-during diapause (Nakamura et al., 2011). Insects may maintain immunity during diapause by regulating the expression of certain proteins. In the D group, pattern recognition proteins, C-type lectins (CTL, A0A088ACU2_APIME), and the immune globulin hemocyanin (HC, F4X264_ACREC) were all upregulated at the protein level and the humoral immune-related protein phenoloxidase (PO, contig_33789) was upregulated at the mRNA level.
C-type lectins are a superfamily of proteins binding and agglutinating bacterial lipopolysaccharides and lipoteichoic acids (Dodd and Drickamer, 2001). Reports on endoparasitoid wasps indicated that CTL binds parasitoid eggs to mask their hemocyte-binding sites. In this way, the wasp could evade detection by the host immune system (Glatz et al., 2003; Lee et al., 2008; Nalini et al., 2008). Since A. gifuensis enter diapause as fourth instar larvae, however the host is already dead by that time. Therefore, the role of CTL upregulation in A. gifuensis diapause is to improve pathogen defense.
Hemocyanin is the major protein component of invertebrate hemolymph. It participates in non-specific invertebrate immunity. A study on Scylla serrata suggested that HC agglutinates bacteria in preparation for host cell defensive measures (Yan et al., 2011). Zhang et al. (2004) reported that purified HC had non-specific antiviral properties. In response to microbial infection, HC can also be converted by proteolytic cleavage into antimicrobial peptides (Destoumieux-Garzón et al., 2001). Since hemocyanin is multifunctional, the 8.45-fold upregulation of HC expression in diapausing A. gifuensis may have been required for protein storage (Jaenicke et al., 1999), ecdysis regulation (Paul et al., 1994), and other processes besides immunity enhancement.
Phenoloxidase is an important enzyme in melanization. It oxidizes phenolic molecules to produce melanin around invading pathogens and wounds (Lu et al., 2014). Melanin and the melanization intermediates may be cytotoxic to microorganisms and impede pathogen invasion. They may also participate in insect wound healing (Eleftherianos and Revenis, 2011). In the D group, there was a 2.93-fold upregulation of phenoloxidase, which was manifested through increased melanin biosynthesis. These findings suggest that insects regulate immunity in diapause by accumulating immune-related proteins. Nevertheless, the precise nature of the changes in cellular immunity that occur during diapause remains unknown.
Melanism and Cuticle Sclerotization in Diapause
The cuticle is the protective barrier between the internal tissues and the external environment in holometabolous larval insects. It is composed of chitin fibers and cuticle proteins (Charles, 2010). Cross-links between the fibers and proteins result in hardening, dehydration, and close packing. This process and cuticle pigmentation are collectively referred to as tanning (Arakane et al., 2005). PO is present during the formation of the cuticulin layer. The quinones generated by PO cross-link the protein chains and stabilize the cuticle (Locke and Krishnan, 1971). A study on Bombyx mori reported that melanization occurred in the cuticle (Ashida and Bery, 1995). Therefore, melanin can form from quinones in the cuticle. Phenoloxidase upregulation, then, may explain the body color darkening observed in diapausing A. gifuensis. Several insect cuticular proteins were upregulated at the protein (A0A232F5H6_9HYME) and mRNA (contig_17805, contig_2099, contig_3789, contig_9417) levels in the D group. Transcriptome analysis also identified a chitin synthase (contig_135) that was upregulated during diapause. These modifications thickened and hardened the larval A. gifuensis cuticle to improve its resistance to mechanical damage.
Correlation Between Transcriptome and Proteome
In this study, the correlation between the transcriptome and proteome of the studied species was found to be very low. This phenomenon has been reported in several other studies as well (Tian et al., 2004; Huang et al., 2013). Transcription is an intermediate step in gene expression, and mRNA is the template for protein synthesis. In theory, protein and mRNA expression should be highly consistent. In practice, however, they may not align because of mRNA instability (Waggoner and Liebhaber, 2003), mRNA-ribosome binding (Zong et al., 1999), protein degradation (Beyer et al., 2004), and post-translational protein modification (Futcher et al., 1999). Proteins directly regulate metabolism and physiology. Transcriptome analysis cannot completely reveal protein expression because of post-transcriptional regulation. Proteome studies may also overlook information for degraded or consumed proteins. Transcriptome and proteome analyses are both incomplete, but they could complement each other. For this reason, comprehensive transcriptome and proteome studies have been conducted in diverse research fields in recent years (Vogel and Marcotte, 2012; Kumar et al., 2016).
Conclusion
In this study, we presented a comprehensive transcriptome and proteome analysis to explore possible molecular processes in A. gifuensis diapaus. As a result, we found three important molecular events (carbohydrate accumulation, immune enhancing and cuticle tanning) that may play roles in survival and stress resistance during diapause. Although the conclusions may need further metabology and immunological tests, and numerous other genes and pathways associated with diapause were not analyzed in this study, these findings still provide foundations and directions for the researche of diapause in Aphidius gifuensis and, more broadly, even all parasitic wasps.
Author Contributions
H-ZZ, TA, F-XH, and L-SZ designed the research. H-ZZ, TA, and F-XH performed research. Y-YL, M-QW, C-XL, and J-JM provided assistance. H-ZZ, TA, and F-XH analyzed data. H-ZZ wrote the manuscript. Y-YL and L-SZ revised the manuscript.
Conflict of Interest Statement
The authors declare that the research was conducted in the absence of any commercial or financial relationships that could be construed as a potential conflict of interest.
Acknowledgments
This research was supported by the National Key R&D Program of China (2017YFD0201000), National Natural Science Foundation of China (31572062), and the Major Science and Technology Project of China National Tobacco Corporation [110201601021(LS-01)]. We are grateful for the assistance of all staff and students in the Key Laboratory of Integrated Pest Management in Crops, Ministry of Agriculture, Sino-American Biological Control Laboratory, USDA-ARS/Institute of Plant Protection, Chinese Academy of Agricultural Sciences, Beijing, China. Additionally, we would like to thank Editage (www.editage.com) for English language editing.
Supplementary Material
The Supplementary Material for this article can be found online at: https://www.frontiersin.org/articles/10.3389/fphys.2018.01697/full#supplementary-material
Figure S1. Original file of Figure 1A.
Figure S2. Original file of Figure 1B.
Table S1. Expression of the quantified transcript.
Table S2. GO enrichement of differentially expressed genes.
Table S3. GO enrichment of differentially expressed proteins.
Table S4. KEGG enrichment of differentially expressed genes.
Table S5. KEGG enrichment of differentially expressed proteins.
Table S6. UniProt annotation of transcript.
Table S7. Expression of the quantified proteins.
Abbreviations
D, diapause; ND, non-diapause; PD, post-diapause; DEG, differentially expressed gene; DEP, differentially expressed protein; GO, Gene Ontology; iTRAQ, isobaric tags for relative and absolute quantification; KEGG, Kyoto Encyclopedia of Genes and Genomes.
References
Altschul, S. F., Gish, W., Miller, W., Myers, E. W., and Lipman, D. J. (1990). Basic local alignment search tool. J. Mol. Biol. 215, 403–410. doi: 10.1016/S0022-2836(05)80360-2
Arakane, Y., Muthukrishnan, S., Beeman, R. W., Kanost, M. R., and Kramer, K. J. (2005). Laccase 2 is the phenoloxidase gene required for beetle cuticle tanning. Proc. Natl. Acad. Sci. U.S.A. 102, 11337–11342. doi: 10.1073/pnas.0504982102
Ashburner, M., Ball, C. A., Blake, J. A., Botstein, D., Butler, H., Cherry, J. M., et al. (2000). Gene ontology: tool for the unification of biology. The gene ontology consortium. Nat. Genet. 25, 25–29. doi: 10.1038/75556
Ashida, M., and Bery, P. T. (1995). Role of the integument in insect defense: pro-phenol oxidase cascade in the cuticular matrix. Proc. Natl. Acad. Sci. USA. 92, 10698–10702. doi: 10.1073/pnas.92.23.10698
Beaulieu, J. M., Gainetdionv, R. R., and Caron, M. G. (2009). Akt/GSK3 signaling in the action of psychotropic drugs. Annu. Rev. Pharmacol. Toxicol. 49, 327–347. doi: 10.1146/annurev.pharmtox.011008.145634
Bemani, M., Izadi, H., Mahdian, K., Khani, A., and Samih, M. A. (2012). Study on the physiology of diapause, cold hardiness and supercooling point of overwintering pupae of the pistachio fruit hull borer, Arimania comaroffi. J. Insect. Physiol. 58, 89–902. doi: 10.1016/j.jinsphys.2012.04.003
Benjamini, Y., and Hochberg, Y. (1995). Controlling the false discovery rate: a practical and powerful approach to multiple testing. J. R. Stat. Soc. 57, 289–300. doi: 10.2307/2346101
Beyer, A., Hollunder, J., Nasheuer, H. P., and Wilhelm, T. (2004). Post-transcriptional expression regulation in the yeast Saccharomyces cerevisiae on a genomic scale. Mol. Cell. Porteomics. 3, 1083–1092. doi: 10.1074/mcp.M400099-MCP200
Biggs, W. H. 3rd, Meisenhelder, J., Hunter, T., Cavenee, W. K., and Arden, K. C. (1999). Protein kinase B/Akt-mediated phosphorylation promotes nuclear exclusion of the winged helix transcription factor FKHR1. Proc. Natl. Acad. Sci. U.S.A. 96, 7421–7426.
Block, W. (1990). Cold tolerance of insects and other arthropods. Philos. T. R. Soc. B. 326, 613–633. doi: 10.1098/rstb.1990.0035
Bradford, M. M. (1976). A rapid and sensitive method for the quantitation of microgram quantities of protein utilizing the principle of protein-dye binding. Anal. Biochem. 72, 248–254. doi: 10.1006/abio.1976.9999
Bräutigam, A., Mullick, T., Schliesky, S., and Weber, A. P. (2011). Critical assessment of assembly strategies for non-model species mRNA-Seq data and application of next-generation sequencing to the comparison of C(3) and C(4) species. J. Exp. Bot. 62, 3093–3102. doi: 10.1093/jxb/err029
Charles, J. P. (2010). The regulation of expression of insect cuticle protein genes. Insect. Biochem. Mol. Biol. 40, 205–213. doi: 10.1016/j.ibmb.2009.12.005.
Chion, H. (1958). Carbohydrate metabolism in the diapause egg of the silkworm, Bombyx mori-II: conversion of glycogen into sorbitol and glycerol during diapause. J. Insect. Physiol. 2, 1–12. doi: 10.1016/0022-1910(58)90024-6
Cohen, P., and Frame, S. (2001). The renaissance of GSK3. Nat. Rev. Mol. Cell. Biol. 2, 769–776. doi: 10.1038/35096075
Cui, S. Y., and Xia, Y. X. (2009). solation and characterization of the trehalose-6-phosphate synthase gene from Locusta migratoria manilensis. Insect. Sci. 16, 287–295. doi: 10.1111/j.1744-7917.2009.01268.x
Denlinger, D. L. (2008). Why study diapause? Entomol. Res. 38, 1–9. doi: 10.1111/j.1748-5967.2008.00139.x
Denlinger, D. L., and Armbruster, P. A. (2014). Mosquito diapause. Annu. Rev. Entomol. 59, 73–93. doi: 10.1146/annurev-ento-011613-162023
Denlinger, D. L., Yocum, G. D., and Rinehart, J. P. (2012). “Hormonal control of diapause”, in Insect Endocrinology, ed L. I. Gilbert (London: Academic Press), 430–463. doi: 10.1016/B978-0-12-384749-2.10010-X
Destoumieux-Garzón, D., Saulnier, D., Garnier, J., Jouffrey, C., Bulet, P., and Bachère, E. (2001). Crustacean immunity-antifungal peptides are generated from the Cterminus of shrimp hemocyanin in response to microbial challenge. J. Biol. Chem. 276, 47070–47077. doi: 10.1074/jbc.M103817200
Ding, L., Li, Y., and Goto, M. (2003). Physiological and biochemical changes in summer and winter diapause and non-diapause pupae of the cabbage armyworm, Mamestra brassicae L. during long-term cold acclimation. J. Insect. Physiol. 49, 1153–1159. doi: 10.1016/j.jinsphys.2003.08.012
Dodd, R. B., and Drickamer, K. (2001). Lectin-like proteins in model organisms: implications for evolution of carbohydrate-binding activity. Glycobiology 11, 71R−79R. doi: 10.1093/glycob/11.5.71R
Eleftherianos, I., and Revenis, C. (2011). Role and importance of phenoloxidase in insect hemostasis. J. Innate. Immun. 3, 28–33. doi: 10.1159/000321931
Futcher, B., Latter, G. L., Monardo, P., McLaughlin, C. S., and Garrels, J. I. (1999). A samping of the yeast proteome. Mol. Cell. Biol. 19, 7375–7368.
Garg, R., Patel, R. K., Tyagi, A. K., and Jain, M. (2011). De novo assembly of chickpea transcriptome using short reads for gene discovery and marker identification. DNA Res. 18, 53–63. doi: 10.1093/dnares/dsq028
Glatz, R., Schmidit, O., and Asgari, S. (2003). Characterization of a novel protein with homology to C-type lectins expressed by the Cotesia rubecula bracovirus in larvae of the lepidopteran host, Pieris rapae. J. Bio. Chem. 278, 19743–19750. doi: 10.1074/jbc.M301396200
Hahn, D. A., and Denlinger, D. L. (2007). Meeting the energetic demands of insect diapause: nutrient storage and utilization. J. Insect. Physiol. 53, 760–773. doi: 10.1016/j.jinsphys.2007.03.018
Hahn, D. A., and Denlinger, D. L. (2011). Energetics of Insect Diapause. Annu. Rev. Entomol. 56, 103–121. doi: 10.1146/annurev-ento-112408-085436
Hand, S. C., Denlinger, D. L., Podrabsky, J. E., and Roy, R. (2016). Mechanisms of animal diapause: recent developments from nematodes, crustaceans, insects, and fish. Am. J. Physiol. Regul. Integr. Comp. Physiol. 310, R1193–R1211. doi: 10.1152/ajpregu.00250.2015
Hayakawa, Y., and Chino, H. (1982). Phosphofructokinase as a possible enzyme regulating glycerol or trehalose accumulation in diapausing insects. Insect. Biochem. 12, 760–773. doi: 10.1016/0020-1790(82)90050-6
Heydari, M., and Izadi, H. (2014). Effects of seasonal acclimation on cold tolerance and biochemical status of the carob moth, Ectomyelois ceratoniae Zeller, last instar larvae. Bull. Entomol. Res. 104, 592–600. doi: 10.1017/S0007485314000364
Huang, S. Q., Chen, L., Te, R. G., Qiao, J. J., Wang, J. X., and Zhang, W. W. (2013). Complementary iTRAQ proteomics and RNA-seq transcriptomics reveal multiple levels of regulation in response to nitrogen starvation in Synechocystis sp. PCC 6803. Mol. Biosyst. 9, 2565–2574. doi: 10.1039/c3mb70188c
Jaenicke, E., Föll, R., and Decker, H. (1999). Spider hemocyanin binds ecdysone and 20-OH-ecdysone. J. Bio. Chem. 374, 34267–34271. doi: 10.1074/jbc.274.48.34267
Kanehisa, M., and Goto, S. (2000). KEGG: Kyoto Encyclopedia of Genes and Genomes. Nucleic. Acids. Res. 28, 27–30. doi: 10.1093/nar/28.1.27
Khan, M. A. Z., Liang, Q., Maria, M. S. M., and Liu, T. X. (2016). Effect of temperature on functional response of Aphidius gifuensis (Hymenoptera: Braconidae) parasitizing Myzus persicae (Hemiptera: Aphididae). Fla. Entomol. 99, 696–702. doi: 10.1653/024.099.0419
Khanmohamadi, F., Khajehali, J., and Izadi, H. (2016). Diapause and cold hardiness of the almond wasp, Eurytoma amygdali (Hymenoptera: Eurytomidae), two independent phenomena. J. Econ. Entomol. 44, 165–173. doi: 10.1093/jee/tow150
Kostál, V. (2006). Eco-physiological phases of insect diapause. J. Insect. Physiol. 52, 113–127. doi: 10.1016/j.jinsphys.2005.09.008
Kostál, V., Sula, J., and Simek, P. (1998). Physiology of drought tolerance and cold hardiness of the Mediterranean tiger moth, Cymbalophora pudica during summer diapause. J. Insect Physiol. 44, 165–173. doi: 10.1016/S0022-1910(97)00047-4
Kumar, D., Bansal, G., Narang, A., Basak, T., Abbas, T., and Dash, D. (2016). Integrating transcriptome and proteome profiling: strategies and applications. Proteomics 16, 2533–2544. doi: 10.1002/pmic.201600140
Lee, S., Nalini, M., and Kim, Y. (2008). A viral lectin encoded in Cotesia plutellae bracovirus and its immunosuppressive effect on host hemocytes. Comp. Biochem. Physiol. A. Mol. Integr. Physiol. 149, 351–361. doi: 10.1016/j.cbpa.2008.01.007
Lehmann, P., Pruisscher, P., Posledovich, D., Carlsson, M., Käkel,ä, R., Tang, P., et al. (2016). Energy and lipid metabolism during direct and diapause development in a pierid butterfly. J. Exp. Bio. 219(Pt 19), 3049–3060. doi: 10.1242/jeb.142687
Li, X. R., Hu, C., and Xin, Y. F. (1999). Artificial induction of Aphidius gifuensis diapause. J Chejiang Univ. 25, 435–438.
Li, Y. Y. (2011). Temperature and Photoperiodic Response of Diapause Induction and Duapause Physiology in Aphidius gifuensis Ashmead. [master's thesis]. Beijing: Chinese Academy of Agricultural Sciences.
Li, Y. Y., Zhang, L. S., Chen, H. Y., Wang, W., and Zhang, J., (2013).Temperature and photoperiodic response of diapause inductuin in Aphidius gifuensis. Chin. J. Appl. Entomol. 50, 718–726. doi: 10.7679/j.issn.2095-1353.2013.100
Livak, K. J., and Schmittgen, T. D. (2001). Analysis of relative gene expression data using real-time quantitative PCR and the2-ΔΔCT method. Methods 25, 402–408. doi: 10.1006/meth.2001.1262
Locke, M., and Krishnan, N. (1971). The distribution of phenoloxidases and polyphenols during cuticle formation. Tissue. Cell. 3, 103–126. doi: 10.1016/S0040-8166(71)80034-4
Lu, A., Zhang, Q., Zhang, J., Yang, B., Wu, K., Xie, W., et al. (2014). Insect prophenoloxidase: the view beyond immunity. Front. Physiol. 5:252. doi: 10.3389/fphys.2014.00252
Mortazavi, A., Williams, B. A., McCue, K., Schaeffer, L., and Wold, B. (2008). Mapping and quantifying mammalian transcriptomes by RNA-Seq. Nat. Methods. 5, 621–628. doi: 10.1038/nmeth.1226
Nakamura, A., Miyado, K., Takezawa, Y., Ohnami, N., Sato, M., Ono, C., et al. (2011). Innate immune system still works at diapause, a physiological state of dormancy in insects. Biochem. Bioph. Res. Co. 410, 351–357. doi: 10.1016/j.bbrc.2011.06.015
Nakata, T. (1995). Population fluctuations of aphis and their natural enemies on potato in Hokkaido, Japan. Appl. Entomol. Zool. 30, 129–138.
Nalini, M., Choi, J. Y., Je, Y. H., Hwang, I., and Kim, Y. (2008). Immunoevasive property of a polydnaviral product, CpBV-lectin, protects the parasitoid egg from hemocytic encapsulation of Plutella xylostella (Lepidoptera: Yponomeutidae). J. Insect. Physiol. 54, 1125–1131. doi: 10.1016/j.jinsphys.2008.04.023
Ohta, I., and Ohtaishi, M. (2006). Effect of low temperature and short day length exposure on the development of Aphidius gifuensis Ashmead (Hymenoptera: Braconidae). Appl. Entomol. Zool. 41, 555–559. doi: 10.1303/aec.2006.555
Pan, M. Z., and Liu, T. X. (2014). Suitability of three aphid species for Aphidius gifuensis, (hymenoptera: braconidae): parasitoid performance varies with hosts of origin. Bio. Control. 69, 90–96. doi: 10.1016/j.biocontrol.2013.11.007
Patel, R. K., and Jain, M. (2012). NGS QC toolkit: a toolkit for quality control of next generation sequencing data. PLoS ONE. 7:e30619. doi: 10.1371/journal.pone.0030619
Paul, R., Bergner, B., Pfefferseidl, A., Decker, H., Efinger, R., and Storz, G. (1994). Gas-transport in the hemolymph of arachnids.1. Oxygen-transport and the physiological-role of hemocyanin. J. Exp. Biol. 188, 25–46.
Puigserver, P., Rhee, J., Donovan, J., Walkey, C. J., Yoon, J. C., Oriente, F., et al. (2003). Insulin-regulated hepatic gluconeogenesis through FOXO1-PGC-1alpha interaction. Nature 423, 550–555. doi: 10.1038/nature01667
Ren, S., Hao, Y. J., Chen, B., and Yin, Y. P. (2018). Global transcriptome sequencing reveals molecular profiles of summer diapause induction stage of onion maggot, Delia antiqua (Diptera: Anthomyiidae). G3 8, 207–217. doi: 10.1534/g3.117.300393
Robinson, M. D., and Oshlack, A. A. (2010). Scaling normalization method for differential expression analysis of RNA-seq data. Genome. Biol. 11:R25. doi: 10.1186/gb-2010-11-3-r25
Sarbassov, D. D., Guertin, D. A., Ali, S. M., and Sabatini, D. M. (2005). Phosphorylation and regulation of Akt/PKB by the rictor-mTOR complex. Science 307, 1098–1101. doi: 10.1126/science.1106148
Saulich, A. K., and Musolin, D. L. (2017). Summer diapause as a special seasonal adaptation in insects: diversity of forms, control mechanisms, and ecological importance. Entomol. Rev. 97, 1183–1212. doi: 10.1134/S0013873817090019
Saunders, D. S. (2014). Insect photoperiodism: effects of temperature on the induction of insect diapause and diverse roles for the circadian system in the photoperiodic response. Entomol. Sci. 17, 25–40. doi: 10.111/ens.12059
Sim, C., and Denlinger, D. L. (2013). Insulin signaling and the regulation of insect diapause. Front. Physiol. 4:186. doi: 10.3389/fphys.2013.00189
Steel, J. E. (1982). Glycogen phosphorylase in insects. Insect Biochem. 12, 131–147. doi: 10.1016/0020-1790(82)90001-4
Su, C. L., Chao, Y. T., Chang, Y. C. A., Chen, W. C., Chen, C. Y., Lee, A. Y., et al. (2011). De novo assembly of expressed transcripts and global analysis of the Phalaenopsis aphrodite transcriptome. Plant. Cell. Physiol. 52, 1501–1514. doi: 10.1093/pcp/pcr097
Takeda, M. (1998). Genetic basis of photoperiodic control of summer and winter diapause in georaphic ecotypes of the rice stem maggot, Chlorops oryzae. Entomol. Exp. Appl. 86, 59–70. doi: 10.1023/A:1003195727467
Tan, Q. Q., Liu, W., Zhu, F., Lei, C. L., Hahn, D. A., and Wang, X. P. (2017). Describing the diapause-preparatory proteome of the beetle Colaphellus bowringi and identifying candidates affecting lipid accumulation using isobaric tags for mass spectrometry-based proteome quantification (iTRAQ). Front. Physiol. 8:251. doi: 10.3389/fphys.2017.00251
Tang, B., Chen, J., Yao, Q., Pan, Z. Q., Xu, W. H., Wang, S. G., et al. (2010). Characterization of a trehalose-6-phosphate synthase gene from Spodoptera exigua and its function identification through RNA interference. J. Insect. Physiol. 56, 813–821. doi: 10.1016/j.jinsphys.2010.02.009
Tang, Y., and Chen, Z. M. (1984). Preliminary study on the biology and the ecology of Aphidius gifuensis (Ashmead). J. Fujian Agricul. Forest. Univ. 13, 119–125.
Tian, Q., Stepaniants, S. B., Mao, M., Weng, L., Feetham, M. C., Doyle, M. J., et al. (2004). Integrated genomic and proteomic analyses of gene expression in Mammalian cells. Mol. Cell. Proteomics. 3, 960–969. doi: 10.1074/mcp.M400055-MCP200
Vogel, C., and Marcotte, E. W. (2012). Insights into the regulation of protein abundance from proteomic and transcriptomic analyses. Nat. Rev. Genet. 13, 227–232. doi: 10.1038/nrg3185
Voinovich, N. D., Reznik, S. Y., and Vaghina, N. P. (2016). Maternal thermal effect on diapause in Trichogramma species (Hymenoptera: Trichogrammatidae). J. Appl. Entomol. 139, 783–790. doi: 10.1111/jen.12214
Waggoner, S. A., and Liebhaber, S. A. (2003). Regulation of alpha-globin mRNA stability. Exp. Biol. Med. 228, 387–395. doi: 10.1177/153537020322800409
Xu, J., Bao, B., Zhang, Z. G., Yi, Y. Z., and Xu, W. H. (2009). Identification of a novel gene encoding the trehalose phosphate synthase in the cotton bollworm, Helicoverpa armigera. Glycobiogoly 19, 250–257. doi: 10.1093/glycob/cwn127
Xu, L., Zheng, L. X., Zhang, C. Q., Zhang, Z. G., and Wei, H. Y. (2016). Effects of temperature and photoperiod on diapause of Aphidius gifuensis Ashmead. Tobacco Sci. Technol. 49, 21–25. doi: 10.16135/j.issn1002-0861.2016.0211
Xue, F., Spieth, H. R., Aiqing, L., and Ai, H. (2002). The role of photoperiod and temperature in determination of summer and winter diapause in the cabbage beetle, Colaphellus Bowringi (coleoptera: chrysomelidae). J. Insect. Physiol. 48, 279–286. doi: 10.1016/S0022-1910(01)00172-X
Yan, F., Qiao, J., Zhang, Y., Zhou, N., Liu, Y., Guo, L., et al. (2011). Hemolytic properties of hemocyanin from mud crab Scylla serrata. J. Shellfish. Res. 30, 957–962. doi: 10.2983/035.030.0338
Yang, S., Yang, S. Y., Zhang, C. P., Wei, J. N., and Kuang, R. P. (2009). Population dynamics of Myzus persicae on tobacco in yunnan province, China, before and after augmentative releases of Aphidius gifuensis. Biocontrol. Sci. Techn. 19, 219–228. doi: 10.1080/09583150802696525
Zhang, X., Huang, C., and Qin, Q. (2004). Anti-viral properties of hemocyanin isolated from shrimp Penaeus monodon. Antivir. Res. 61, 93–99. doi: 10.1016/j.antiviral.2003.08.019
Keywords: aphidius gifuensis, diapause, molecular mechanism, transcriptome, proteome
Citation: Zhang H-Z, Li Y-Y, An T, Huang F-X, Wang M-Q, Liu C-X, Mao J-J and Zhang L-S (2018) Comparative Transcriptome and iTRAQ Proteome Analyses Reveal the Mechanisms of Diapause in Aphidius gifuensis Ashmead (Hymenoptera: Aphidiidae). Front. Physiol. 9:1697. doi: 10.3389/fphys.2018.01697
Received: 28 July 2018; Accepted: 12 November 2018;
Published: 30 November 2018.
Edited by:
Bin Tang, Hangzhou Normal University, ChinaReviewed by:
Yifan Zhai, Shandong Academy of Agricultural Sciences, ChinaHamzeh Izadi, Vali-E-Asr University of Rafsanjan, Iran
Copyright © 2018 Zhang, Li, An, Huang, Wang, Liu, Mao and Zhang. This is an open-access article distributed under the terms of the Creative Commons Attribution License (CC BY). The use, distribution or reproduction in other forums is permitted, provided the original author(s) and the copyright owner(s) are credited and that the original publication in this journal is cited, in accordance with accepted academic practice. No use, distribution or reproduction is permitted which does not comply with these terms.
*Correspondence: Li-Sheng Zhang, emhhbmdsZWVzaGVuZ0AxNjMuY29t