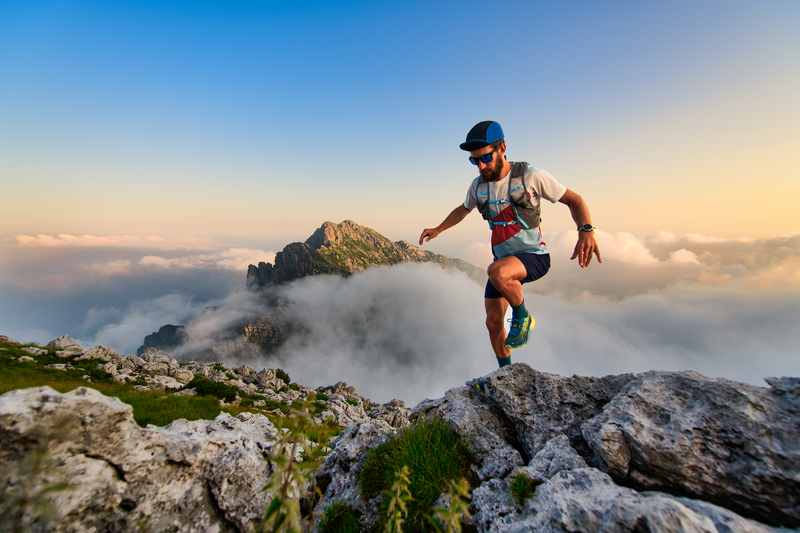
95% of researchers rate our articles as excellent or good
Learn more about the work of our research integrity team to safeguard the quality of each article we publish.
Find out more
REVIEW article
Front. Physiol. , 20 November 2018
Sec. Lipid and Fatty Acid Research
Volume 9 - 2018 | https://doi.org/10.3389/fphys.2018.01663
Tay-Sachs disease belongs to the group of autosomal-recessive lysosomal storage metabolic disorders. This disease is caused by β-hexosaminidase A (HexA) enzyme deficiency due to various mutations in α-subunit gene of this enzyme, resulting in GM2 ganglioside accumulation predominantly in lysosomes of nerve cells. Tay-Sachs disease is characterized by acute neurodegeneration preceded by activated microglia expansion, macrophage and astrocyte activation along with inflammatory mediator production. In most cases, the disease manifests itself during infancy, the “infantile form,” which characterizes the most severe disorders of the nervous system. The juvenile form, the symptoms of which appear in adolescence, and the most rare form with late onset of symptoms in adulthood are also described. The typical features of Tay-Sachs disease are muscle weakness, ataxia, speech, and mental disorders. Clinical symptom severity depends on residual HexA enzymatic activity associated with some mutations. Currently, Tay-Sachs disease treatment is based on symptom relief and, in case of the late-onset form, on the delay of progression. There are also clinical reports of substrate reduction therapy using miglustat and bone marrow or hematopoietic stem cell transplantation. At the development stage there are methods of Tay-Sachs disease gene therapy using adeno- or adeno-associated viruses as vectors for the delivery of cDNA encoding α and β HexA subunit genes. Effectiveness of this approach is evaluated in α or β HexA subunit defective model mice or Jacob sheep, in which Tay-Sachs disease arises spontaneously and is characterized by the same pathological features as in humans. This review discusses the possibilities of new therapeutic strategies in Tay-Sachs disease therapy aimed at preventing neurodegeneration and neuroinflammation.
GM2-gangliosidoses are a group of autosomal-recessive lysosomal storage disorders (LSDs). These diseases result from a deficiency of lysosomal enzyme β-hexosaminidase (Hex), which is responsible for GM2 ganglioside degradation (Ferreira and Gahl, 2017). Gangliosides are the main glycolipids of neuronal cell plasma membranes which ensure normal cellular activities (Sandhoff and Harzer, 2013). There are two major β-hexosaminidase isoenzymes: HexA consists of two subunits, α and β; HexB is a homodimer consisting of two β-subunits (Ferreira and Gahl, 2017). The two subunits of HexA enzyme, α and β, are synthesized at the endoplasmic reticulum (ER) where glycosylation, the formation of intramolecular disulfide bonds and dimerization take place (Weitz and Proia, 1992; Maier et al., 2003). Besides HexA and HexB isoenzymes a homodimer consisting of two α-subunits, called HexS, is also found (Hou et al., 1996).
After the dimerization of subunits in ER, β-hexosaminidase is transported to the Golgi complex, where it undergoes post-translational modification. The most important of these is the addition of mannose-6-phosphate (M6P) to the side chains of the oligosaccharide (Sonderfeld-Fresko and Proia, 1989). The residues of phosphorylated mannose can be considered as an address mark recognized by specific receptors found on the inner surface of the Golgi complex’s membranes. With the aid of this mark a lysosome recognizes the enzyme and absorbs it (Weitz and Proia, 1992).
Inside lysosomes α and β subunits are proteolytically processed into a mature form (Hubbes et al., 1989). Also in the lysosome, the presentation of the GM2 ganglioside substrate from the bilayer to the HexA active site requires the presence of GM2 activator protein (GM2A) (Martino et al., 2002b; Cachon-Gonzalez et al., 2006). GM2A a co-factor and is necessary in order to make lipophilic GM2 ganglioside available for hydrolysis in the hydrophilic medium of the lysosome (Renaud and Brodsky, 2016; Sandhoff, 2016; Figure 1). HexA and HexB can hydrolyze a wide range of substrates with terminal N-acetylglucosamine residues (GlcNAc) to β-bonds. Only the HexA isoenzyme can interact with a GM2 ganglioside-GM2A complex (Lemieux et al., 2006). Although only HexA hydrolyzes GM2 ganglioside, both isoenzymes can hydrolyze glycoproteins, glycosaminoglycans, and glycolipids (Ferreira and Gahl, 2017).
HexA, HexB, and HexS in the absence of GM2A can also hydrolyze synthetic substrates, for example, 4-methylumbelliferone-GlcNAc fluorescent substrate (4MUG). Another compound related to 4MUG is 4-methylumbelliferyl-GlcNAc-6-sulfate (4MUGS) which is only hydrolyzed by isoenzymes HexA and HexS. These compounds are used in GM2-gangliosidoses diagnosis and detection of HEXA and HEXB gene mutation carriers (Cachon-Gonzalez et al., 2012).
The HexA enzyme is a product of HEXA and HEXB genes that encode, respectively, the α and β subunits, the amino acid sequence identity of which is about 60% (Mark et al., 2003; Dersh et al., 2016).
GM2-gangliosidosis can be caused by mutations in three genes: HEXA (15th chromosome), HEXB (5th chromosome), and GM2A (5th chromosome) (Mahuran, 1999; Ferreira and Gahl, 2017). GM2-gangliosidosis includes (I) Tay-Sachs disease (TSD, OMIM 272800), at which mutations occur in the HEXA gene and only HexA activity is disrupted (variant B); (II) Sandhoff disease (SD; OMIM 268800), caused by mutations in HEXB gene, at which the activity of HexA and HexB is disrupted (variant O); and (III) GM2 activator protein deficiency (OMIM 272750), at which mutations take place in the GM2A gene (variant AB) (Mahuran, 1999).
In patients with HexA deficiency GM2 ganglioside accumulates inside lysosomes, which form characteristic inclusions within the cells, so called membranous cytoplasmic bodies, which are enlarged lysosomes filled with gangliosides (Ferreira and Gahl, 2017; Figure 1). The highest concentration of GM2 ganglioside is found in neuronal cells, therefore, the HexA deficiency primarily affects the nervous system, causing mental and motor developmental delay in patients (Myerowitz, 1997). Later, progressive destruction of neurons, proliferation of microglia and accumulation of complex lipids in macrophages are observed in the brain tissue. A similar process develops in the neurons of the cerebellum, basal ganglia, brain stem, spinal cord, spinal ganglia, and also in neurons of the autonomic nervous system. Ganglion cells in the retina also swell and contain GM2 gangliosides, particularly, along the edges of the macula. As a result, a cherry red spot appears in the macula and emphasizes the normal color of the actual choroid, contrasting with the pale, swollen ganglion cells in the affected part of the retina (Ferreira and Gahl, 2017).
An inflammatory response is also observed in patients with GM2-gangliosidosis. Wada et al. (2000) offered a model of acute neurodegeneration in GM2-gangliosidosis and showed that massive death of neurons is preceded by activated microglia expansion. The activation of macrophages and astrocytes along with inflammatory mediators production is also observed (Myerowitz et al., 2002). This inflammatory response can occur before the clinical manifestation of symptoms and aggravates the neurological dysfunction (Wu and Proia, 2004).
In the CNS of GM2-gangliosidosis mouse model, microglial cell activation, and infiltration of inflammatory cells are also observed (Jeyakumar et al., 2003). Hayase et al. (2010) showed that TSD patient cerebrospinal fluid has significantly increased levels of TNF-α pro-inflammatory cytokine, which is involved in the induction of inflammatory response. The authors suggested that an increase in TNF-α level indicates inflammation in the CNS and may contribute to disease progression (Hayase et al., 2010). Utz et al. (2015) identified five possible inflammatory biomarkers ENA-78, MCP-1, MIP-1α, MIP-1β, and TNFR2, increased levels of these in the cerebrospinal fluid is associated with infantile gangliosidosis.
Tay-Sachs disease is caused by mutations in the HEXA gene. The incidence of this disease is one in 100,000 live births (carrier frequency of about one in 250) (Lew et al., 2015). TSD, SD, and GM2A deficiency are clinically similar (Seyrantepe et al., 2018). More than 130 different mutations in HEXA gene are described (partial deletion, splicing mutations, nonsense mutations, missense mutations) leading to disruption of transcription, translation, folding, dimerization of monomers and catalytic dysfunction of HexA protein (Myerowitz, 1997; Sakuraba et al., 2006; Mistri et al., 2012). TSD heterogeneity in severity of clinical symptoms and the age at disease onset is determined by residual HexA enzymatic activity that occurs with some mutations (Kaback and Desnick, 1993). Only 10–15% of HexA activity is required in order to prevent the accumulation of GM2 ganglioside (Osher et al., 2015). The three different forms of TSD are classified by severity of clinical symptoms and the age of onset (Patterson, 2013).
Clinical symptoms and course of the infantile form of TSD, which occurs more often than others, are the most studied. The infantile form, which is characterized by onset around 6 months of age and very low HexA activity levels (<0.5%), rapidly manifests with mental and motor developmental delay (Sandhoff and Christomanou, 1979). In the most severe infantile forms symptoms may occur a few months after childbirth (Nestrasil et al., 2018). Common neurodegenerative symptoms in infants are hypotension, inability to sit or hold their head unsupported, eye movement abnormalities, dysphagia, spasms, and hypomyelination (Jarnes Utz et al., 2017). Most patients with the infantile TSD do not survive past 4 years of age (Bley et al., 2011).
The juvenile form of the disease strikes in early childhood, usually at the age of 3–10 years. Common symptoms are ataxia, dysarthria, dysphagia development, hypotension, and spasm progression. Most patients do not live past the age of 15 years (Nestrasil et al., 2018). In adolescent patients the disease is less severe but has a wider range of symptoms (Regier et al., 2016). Common symptoms are limb muscle weakness and abnormal gait which are observed in 88% of patients (Maegawa et al., 2006).
In contrast to the infantile and juvenile forms, the later-onset form is less aggressive, characterized by small mutations and higher residual activity level of HexA, which is 5–20% of normal activity. Symptoms typically appear in adolescence or early adulthood, but can appear later (20–30 years) (Sandhoff and Christomanou, 1979). Therefore, neurodegeneration progression is slowed presenting with delayed onset and gradual decline in motor, cerebral and spinocerebellar function (Deik and Saunders-Pullman, 2014; Osher et al., 2015).
Currently there are several therapy approaches to these diseases, including substrate reduction (Bembi et al., 2006), bone marrow transplantation (Jacobs et al., 2005), hematopoietic (Stepien et al., 2017) or neural stem cell transplantation (Gonzalez et al., 2016), use of anti-inflammatory drugs (Hayase et al., 2010), administration of purified enzyme (Tsuji et al., 2011), and gene therapy to restore expression of a dysfunctional protein (Cachon-Gonzalez et al., 2012).
To investigate the efficacy of new TSD therapies Liu and Zhao (2016) proposed an in vitro model of induced pluripotent stem cells (iPSCs) derived from infantile TSD patient fibroblasts. These cells expressed OCT4, SOX2, NANOG, Tra-1-60, and alkaline phosphatase pluripotency factors and had the ability to differentiate into tissues from all three germ layers (Liu and Zhao, 2016).
The main in vivo models of TSD include mice and sheep. The first mouse TSD model was created in 1995 by knockout of the HEXA gene. This line of mice lacked HexA activity, however, GM2 ganglioside accumulation and membrane cytoplasmic body formation in neurons occurred only in certain regions of the brain, excluding the olfactory bulb, the cerebral cortex and the anterior horn of the brain. Also, HEXA knockout mice had a normal lifespan and no clinical symptoms of TSD (Taniike et al., 1995). A number of other studies show that HEXA-defective mice exhibit biochemical and pathological features of TSD without obvious neurological dysfunction (Cohen-Tannoudji et al., 1995; Sango et al., 1995). The difference in the distribution of neuronal storage delineates a difference in ganglioside metabolism between humans and mice. It was shown that mice have one or more sialidases that remove sialic acid from GM2 ganglioside, which can later be hydrolyzed by HexB in HEXA-deficient TSD model mice (Yuziuk et al., 1998; Seyrantepe et al., 2018).
In contrast to HEXA-deficient mice HEXB-deficient TSD mice, develop CNS neurodegeneration, with spasticity, muscle weakness, rigidity, tremor, and ataxia (Sango et al., 1995; Phaneuf et al., 1996). Thus HEXB-deficient mice can be useful for the initial evaluation of potential GM2-gangliosidosis treatment.
A strain of mice deficient in HEXA and sialidase NEU3 genes have been developed with a lifespan of 1.5–4.5 months (Seyrantepe et al., 2018). An abnormal accumulation of GM2 ganglioside in the brains of these mice and the presence of membrane cytoplasmic bodies in neurons were found. HEXA/NEU3-deficient mice have progressive neurodegeneration, bone structure anomalies, and neurologic abnormalities such as ataxia, tremor and slow movement. The described pathologies and symptoms in HEXA/NEU3-deficient mice mimic those observed in patients with early onset TSD. This strain of mouse is a suitable model to investigate new TSD therapies (Seyrantepe et al., 2018).
Tay-Sachs disease is also described in other animal species, for example flamingo Phoenicopterus ruber (Zeng et al., 2008) and Jacob sheep (Torres et al., 2010). In these species TSD develops spontaneously and is characterized by HexA enzymatic activity deficiency and GM2 ganglioside accumulation (Lawson and Martin, 2016). It was shown that the nucleotide and amino acid sequences identity of the coding region of HEXA gene in flamingo and humans is about 70% (Zeng et al., 2008). However, a serious interspecies difference limits the use of flamingos as a model to investigate pathogenesis and therapy of human TSD. In terms of research, one of the most useful species with spontaneously developing TSD is a Jacob sheep. Torres et al. (2010) showed that in Jacob sheep, TSD clinical manifestations are closest to the pathological features in humans. Sheep with TSD also suffered from ataxia, proprioceptive defects and cortical blindness (Porter et al., 2011). Genetic studies showed that HexA activity deficiency in these sheep is associated with a single nucleotide substitution in exon 11 of the HEXA gene, which leads to glycine-to-arginine substitution (Torres et al., 2010).
Substrate reduction therapy (SRT) utilizes small molecules to slow the rate of glycolipid biosynthesis (Platt et al., 2003). Efficacy of miglustat (N-butyldeoxynojirimycin, NB-DNJ) in the prevention of GM2 ganglioside accumulation was demonstrated in TSD murine models (Platt et al., 1997; Bembi et al., 2006). NB-DNJ is a small iminosugar competitive inhibitor of glucosylceramide synthase. It catalyzes the first committed step of glycosphingolipid synthesis and can penetrate the blood-brain barrier (Boomkamp et al., 2010). It has been shown that that NB-DNJ added to the food of TSD model mice reduces GM2 ganglioside accumulation in the brain by 50% (Platt et al., 1997). Bembi et al. (2006) assessed the clinical efficacy of NB-DNJ in two patients with TSD infantile form. The authors observed a significant concentration of NB-DNJ in the cerebrospinal fluid of the patients. The use of SRT did not stop the neurologic dysfunction progression in patients, however, the authors recommend the use of NB-DNJ for macrocephaly prevention (Bembi et al., 2006). Similar results were described in a clinical trial (NCT00672022) in five patients (Maegawa et al., 2009).
The development of enzyme replacement therapy (ERT) is a promising option for the treatment of lysosomal storage diseases. After ERT therapy many somatic symptoms are decreased, but it is less effective in preventing CNS neurodegeneration since intravenous administration doesn’t allow the enzyme molecule to cross the blood-brain barrier (Jakobkiewicz-Banecka et al., 2007; Sorrentino et al., 2013). ERT is clinically approved for the following diseases: Gaucher disease (Barton et al., 1991; Connock et al., 2006), Fabry disease (Eng et al., 2001), Pompe disease (Klinge et al., 2005), and mucopolysaccharidosis type I (Wraith et al., 2004), type II (Muenzer et al., 2002) and type VI (Harmatz et al., 2004).
The major challenge in creating HexA-based ERT is the need to synthesize both of the enzyme subunits (Tropak et al., 2016). Methylotrophic yeast Ogataea minuta (Om) culture, simultaneously expressing the HEXA and HEXB genes, can be used for the production of recombinant HexA enzymes. The purified HexA was treated with α-mannosidase to expose mannose-6-phosphate (M6P) residues on the N-glycans (Akeboshi et al., 2007; Tsuji et al., 2011). The therapeutic efficacy of recombinant HexA was demonstrated in the SD mouse model (hexb-/- mice) and improvement of motor function, increase of survival rate and inhibition of the induction of MIP-1α were noted (Tsuji et al., 2011). Tropak et al. (2016) created a hybrid μ subunit combining the critical characteristics of α and β HexA subunits. The hybrid μ subunit contains an active α subunit site, a stable β subunit interface, and unique regions of each subunit necessary for interaction with GM2A. To purify the HexM μ-homodimer HEK239 cells with CRISPR deleted HEXA and HEXB genes and also stably expressing the μ subunit were used. The authors showed that, in combination with GM2A, HexM hydrolyzes the derivative of GM2-ganglioside both in cellulo and in vitro (Tropak et al., 2016).
Matsuoka et al. (2011) modified the nucleotide sequence of the human HEXB gene encoding the chimeric β subunit to contain the partial amino acid sequence of the α subunit. Chinese hamster ovary (CHO) cell lines were modified with the chimeric HEXB gene to obtain a cell line with the chimeric HexB stable expression. It was shown that chimeric HexB can degrade artificial anionic substrates and GM2 ganglioside in vitro, and also maintain the thermal stability of the wild-type HexB enzyme in plasma. In TSD patient derived fibroblasts it was shown that the treatment of cells with the chimeric HexB led to the incorporation of the enzyme into the cells and the degradation of the accumulated GM2-ganglioside. Intracerebroventricular administration of chimeric HexB to SD model mice restored Hex activity in the brain and reduced the accumulation of GM2-ganglioside in the parenchyma (Matsuoka et al., 2011).
Jacobs et al. (2005) published the clinical case of the application of bone marrow transplantation (BMT) with the following substrate reduction therapy to treat the patient with TSD. The use of BMT and Zavesca® (miglustat) led to an increase in HexA activity in leukocytes and plasma 23 months after transplantation, but did not prevent the development of neurological dysfunction.
A case of BMT from a HLA-matched sibling to a 15-year-old patient with late-onset TSD has been described where 8 years after BMT complete graft retention remained unchanged. HexA activity in leukocytes was 187 nmol/mg/h, which is comparable to the enzyme activity in control group leukocytes. HexA activity in plasma was 15 nmol/mg/h, which is three times lower than the lower limit of HexA normal activity (50–250 nmol/mg/h). There was also no intentional tremor progression after BMT (Stepien et al., 2017).
There are cases of BMT in in vivo studies on SD mouse models which showed that BMT prolongs the survival rate (from 4.5 to 8 months) (Norflus et al., 1998) and improves neurologic manifestations in laboratory animals (Wada et al., 2000).
An alternative approach for patients who do not have a suitable bone marrow donor is transplantation of hematopoietic stem cells from umbilical cord blood obtained from partially HLA-matched unrelated donors (Martin et al., 2006). Human umbilical cord blood is an important source of stem cells and progenitor cells capable of providing neuroprotective effect in degenerative disease caused by various factors. Transplantation of umbilical cord blood cells is considered to be a promising approach to treat neurodegenerative disease in ischemic or traumatic spinal cord injury (Galieva et al., 2017).
Attempts to correct mutations in HEXA gene by gene and cell engineering began in the mid-1990s. The first vectors for the delivery of wild-type HEXA gene were adenoviruses. Akli et al. (1996) first produced TSD patient skin-derived fibroblasts expressing the HEXA gene by adenovirus transduction. The enzyme activity in the transduced fibroblasts was 40–84% of the norm. The secretion level of an enzyme α-subunit was 25 times higher than the patient’s untreated control fibroblasts (Akli et al., 1996).
In vivo studies with HEXA knockout mice showed that in case of intravenous co-administration, HEXA and HEXB gene expressing adenoviral vectors preferentially transduced liver cells. Delivery of both HexA subunits promoted enzyme secretion in the serum, as well as the enzymatic activity restoration in peripheral tissues (Guidotti et al., 1999). The main limiting factor for successful use of adenoviral vectors in CNS disease treatment is the inability to overcome the blood-brain barrier (Gray et al., 2010). Another disadvantage is the immunogenicity of adenoviral vectors and their tropism to liver cells which leads to a high accumulation of the vector and overexpression of the transgene in this organ which risks development of hepatocellular carcinoma (Nakamura et al., 2003).
Guidotti et al. (1998) also constructed a retroviral vector encoding the human HEXA gene cDNA and produced a stable line of hexa-/- mouse fibroblasts with overexpression of the human HEXA gene. The resulting fibroblast line secreted the interspecies HexA enzyme: human α-subunit and mouse β-subunit. The cultivation of fibroblasts with HexA deficiency in the culture medium from transduced fibroblasts resulted in restoration of intracellular HexA activity in non-transduced cells. Absorption of the enzyme by non-transduced fibroblasts from the culture medium was the result of receptor-mediated transfer analagous to lysosomal uptake of the enzyme. Thus HexA can pass from the overexpressing cell to neighboring cells that have a receptor essential for recognizing of M6P (Guidotti et al., 1998). This method based on the ability of non-transduced cells to take up the enzyme is termed cross-correction.
The possibility of cross-correction to restore the metabolism of gangliosides in TSD patient-derived fibroblasts in vitro has been investigated. A stable NIH3T3 mouse fibroblast cell line with HEXA gene overexpression was made using retroviral transduction. It was shown that when cultured in transduced NIH3T3 cells conditioned medium TSD-fibroblasts absorbed a large amount of soluble enzyme, however, there was not a sufficient amount of the enzyme necessary for the degradation of GM2 ganglioside in lysosomes (Martino et al., 2002b). Therefore, the cross-correction-based delivery of HexA is insufficient to change the phenotype of TSD-fibroblasts.
The major challenges in TSD gene therapy are the choice of the vector and delivery method of therapeutic genes in order to overcome the blood-brain barrier along with minimal side effects (Kyrkanides et al., 2005). Martino et al. (2005) proposed an in vivo gene transfer strategy for the production and distribution of the HEXA gene in the CNS in TSD model animals. A replication-defective herpes simplex virus type 1 (HSV-1) encoding HEXA gene cDNA was made. HSV-1 is able to infect various types of non-dividing cells, including neurons, and is transferred in a retrograde fashion to motor and/or sensory neuron bodies after peripheral inoculation (Wolfe et al., 1999). It was shown that the injection of HSV-1-HEXA into the inner capsule of the left cerebral hemisphere of TSD model mice restored HexA activity. GM2 ganglioside accumulation disappeared both in the injected and in the control (right) hemispheres, as well as in the cerebellum and spinal cord of the studied animals within a month after the injection (Martino et al., 2005). Thus when the viral vector is directly delivered to the brain of laboratory animals, a high efficiency of cell transduction is shown. However, due to the large size of a human brain, this approach has limitations for the uniform distribution of the viral vector throughout the central nervous system and would require a large number of injections.
Great progress has been achieved in developing approaches for GM2 gangliosidoses gene therapy using adeno-associated virus (AAV)-based vectors (Gray-Edwards et al., 2018). Intracranial administration of recombinant AAV (rAAV) serotypes 2/1 or 2/2 encoding the HEXA and HEXB gene cDNA to SD model mice led to a wide spread of HexA in the nervous system without apparent cytotoxicity associated with rAAV use and an increase in mouse survival rate (Cachon-Gonzalez et al., 2006, 2012). Defects in myelination occur at an early age and so there are time limits within which gene therapy can lead to positive results and slow the progression of neurological worsening (Cachon-Gonzalez et al., 2014).
Adeno-associated virus-based vectors are limited in their capacity (from 2.1 to 4.5 kb) and cannot carry the cDNA of both HEXA and HEXB genes, and the efficiency of co-transduction is significantly less than transduction with a single construct. This is a limiting factor in the application of these vectors since the effective recovery of the secretion of the absent heterodimeric HexA isoenzyme requires the expression of both subunits, α and β (Tropak et al., 2016). Tropak et al. (2016) designed the self-complementary AAV9.47 encoding a hybrid μ subunit (scAAV9.47-HEXM) and showed that intracranial injection of scAAV9.47-HEXM decreased GM2 ganglioside accumulation in the brain of TSD model mice. Intravenous administration of scAAV9.47-HEXM to newborn TSD model mice showed a long-term decrease in GM2 ganglioside accumulation in the CNS and a decreased distribution of this vector in the liver compared to AAV9, AAVrh10 or AAVrh8 (Karumuthil-Melethil et al., 2016). Intravenous injection of scAAV9.47-HEXM results in effective transduction of CNS cells and an 2.5-fold increase in survival rate of newborn SD model mice compared with the control group (Osmon et al., 2016).
The therapeutic efficacy of AAVrh8-based gene therapy on the Jacob sheep TSD model has been studied. Sheep aged 2–4 months received intracranial injections of only AAVrh8 encoding α subunit (AAVrh8-HEXA), or two vectors encoding α or β subunit (AAVrh8-HEXA + AAVrh8-HEXB). It was shown that all sheep after AAV injection had a delay in the appearance of symptoms and/or a decrease in the acquired symptoms of the disease. When AAVrh8-HEXA + AAVrh8-HEXB were injected, an excellent distribution of HexA in the sheep brain was noted, unlike the injection of AAVrh8-HEXA. However, HexA distribution in the sheep spinal cord was low in all groups and a decrease in the activation and proliferation of microglia in the sheep brain after gene therapy was noted (Gray-Edwards et al., 2018). The data were confirmed by studies with SD cats that demonstrated safety and a wide spread of Hex in the CNS, after intracranial injection of AAVrh8 encoding the species-specific α and β Hex subunits (Bradbury et al., 2013).
A study of the safety of AAVrh8 encoding the α and β Hex subunit of normal cynomolgus macaques (cm) showed that dyskinesia, ataxia, and loss of dexterity developed in most of the monkeys with intracranial injection of AAVrh8-cmHexα/β (Golebiowski et al., 2017). Animals that received a high dose of AAVrh8-cmHexα/β eventually became apathetic. The time of symptom onset depended on the dose, with the highest dose causing symptoms within a month after the infusion. Histological analyses showed severe necrosis of white and gray matter along the injection pathway, the reactive vasculature and the presence of neurons with granular eosinophilic material. Despite neurotoxicity, a sharp increase in Hex activity was noted in the thalamus (Golebiowski et al., 2017). The authors suggested that severe neurotoxicity may be associated with Hex overexpression. The data about TSD gene therapy are generalized in Table 1.
The transplantation of ex vivo modified multipotent neural cells (MNCs) in the CNS is another therapeutic strategy. An MNC line with human HEXA gene overexpression (MNCs-HEXA) has been produced by retroviral transduction Lacorazza et al. (1996). MNCs-HEXA stably secreted the biologically active HexA enzyme and cross-corrected the metabolic defect in TSD patient-derived fibroblast culture in vitro. Intracranial injection of MNCs-HEXA to mice resulted in expression of a significant quantity of the human HexA subunit transcript and active HexA enzyme production (Lacorazza et al., 1996). It has been shown that transduction of stromal cells obtained from the bone marrow of TSD model mice, with retrovirus encoding HEXA gene cDNA, results in an increase in secretion of the active HexA enzyme capable of hydrolyzing GM2 ganglioside (Martino et al., 2002a).
Currently, the number of available treatments for patients with LSDs is increasing. For example, BMT (Lange et al., 2006; Rovelli, 2008), SRT (Coutinho et al., 2016), and ERT (Li, 2018) are used for therapy of Gaucher disease, Fabry disease, mucopolysaccharidoses (MPS), Pompe disease, Niemann-Pick disease, etc. ERT and SRT methods are approved for these diseases in Europe, United States, and other countries (Beck, 2018). The previously described drug Zavesca® is also used for the treatment of Niemann-Pick disease type C (Hassan et al., 2018; Pineda et al., 2018) and GM1-gangliosidosis (Deodato et al., 2017). It is worth mentioning that, similarly to TSD, early diagnosis is necessary for successful LSD therapy in order to prevent organ damage that aggravates the disease progression (Wasserstein et al., 2018).
Lifelong prescription of ERT with recombinant glucocerebrosidase showed good results in the treatment of Gaucher disease. ERT stops the main clinical manifestations of the disease, improves the quality of life of patients and has no pronounced adverse effects (Zimran et al., 2018). However, for some LSDs, such as MPSI, II and VI, Pompe disease and diseases with CNS damage, ERT is not effective as the large molecules of recombinant enzymes are unable to penetrate damaged tissues to achieve therapeutic levels (Wraith, 2006; Begley et al., 2008; Parenti et al., 2013).
Non-TSD LSDs caused by missense mutations and small deletions without frameshift can be treated with the use of small molecules of pharmacological chaperones to increase active enzyme concentration (Pereira et al., 2018). If the mutation does not affect the active or binding site of the enzyme and only leads to disruption of the protein conformation then pharmacological chaperones can be used as protein stabilizers to form a stable structure and maintain catalytic activity (Parenti, 2009). The molecular chaperones thereby increase the intracellular pool of active enzymes and can partially restore metabolic homeostasis. The application of this approach is under investigation for Gaucher disease (Goddard-Borger et al., 2012), Fabry disease (Kato et al., 2010), Pompe disease (Porto et al., 2009), and Krabbe disease (Berardi et al., 2014).
For many LSDs gene therapy approaches [comprehensively discussed in the review (Biffi, 2016)] as well as genome editing techniques using zinc-finger nucleases (ZFN) for MPSI and MPSII (Harmatz et al., 2018) are being actively explored. Improvement of LSD treatment methods, in particular, using pharmacological chaperones and genome editing technologies can also contribute to the development of new approaches to TSD treatment which is important since the current approved treatment options for this disease have low efficiency.
To achieve a therapeutic effect in the treatment of TSD the production and distribution of the absent HexA enzyme in CNS is required. SRT, ERT, and BMT showed low efficacy to prevent neurodegeneration in the CNS although these methods can partially restore HexA activity and reduce GM2 ganglioside accumulation in cells (Table 2). It is important to remember that in order to achieve the maximum therapeutic effect, it is necessary to start TSD therapy from the time of its early manifestations, since myelination defects appear at early stages and are aggravated with time.
TABLE 2. Efficacy of various therapeutic approaches for TSD treatment in pre-clinical and clinical trials.
Currently there are methods of TSD gene therapy being developed using viral vectors for the delivery of cDNA of encoding α and β HexA subunit genes. In humans, it is necessary for viral vectors to successfully cross the blood-brain barrier since the injection of genetic constructs directly into the CNS is not feasible due to the large size of the human brain. In addition, severe neurotoxic effects due to HexA overexpression in case of direct viral delivery to the brain of cynomolgus macaques gives rise to concern.
Of particular interest are studies using the scAAV9.47 vector encoding the HEXM gene of the hybrid μ subunit that contains the α subunit active site, the stable β subunit interface, and also the unique regions in each subunit that are required for interaction with GM2A. This vector is able to cross the blood-brain barrier and the HEXM gene circumvents the capacity limitation of AAV vectors. However, studies of the efficacy of this viral construction are currently limited to in vivo experiments in TSD or SD model mice.
Further investigation of the therapeutic potential of genetically modified stem cells is important, since in addition to restoring the activity of the deficient enzyme, these cells can have a neuroprotective effect to limit the degenerative processes that are observed in TSD patients. This, combined with stem cells can prevent the activation of microglia guarding against further neurodegeneration.
VS and AR conceived the idea. VS wrote the manuscript and made the tables. AS and DC collected the data of TSD gene therapy. KK created the figure. LC and AR edited the manuscript and tables. All authors contributed to read and commented on the manuscript.
The work was performed according to the Russian Government Program of Competitive Growth of Kazan Federal University. AR was supported by state assignment 20.5175.2017/6.7 of the Ministry of Education and Science of Russian Federation and the President of the Russian Federation grant HШ-3076.2018.4.
The authors declare that the research was conducted in the absence of any commercial or financial relationships that could be construed as a potential conflict of interest.
Akeboshi, H., Chiba, Y., Kasahara, Y., Takashiba, M., Takaoka, Y., Ohsawa, M., et al. (2007). Production of recombinant beta-hexosaminidase A, a potential enzyme for replacement therapy for Tay-Sachs and Sandhoff diseases, in the methylotrophic yeast Ogataea minuta. Appl. Environ. Microbiol. 73, 4805–4812. doi: 10.1128/AEM.00463-07
Akli, S., Guidotti, J. E., Vigne, E., Perricaudet, M., Sandhoff, K., Kahn, A., et al. (1996). Restoration of hexosaminidase A activity in human Tay-Sachs fibroblasts via adenoviral vector-mediated gene transfer. Gene Ther. 3, 769–774.
Barton, N. W., Brady, R. O., Dambrosia, J. M., Di Bisceglie, A. M., Doppelt, S. H., Hill, S. C., et al. (1991). Replacement therapy for inherited enzyme deficiency–macrophage-targeted glucocerebrosidase for Gaucher’s disease. N. Engl. J. Med. 324, 1464–1470. doi: 10.1056/NEJM199105233242104
Beck, M. (2018). Treatment strategies for lysosomal storage disorders. Dev. Med. Child Neurol. 60, 13–18. doi: 10.1111/dmcn.13600
Begley, D. J., Pontikis, C. C., and Scarpa, M. (2008). Lysosomal storage diseases and the blood-brain barrier. Curr. Pharm. Des. 14, 1566–1580. doi: 10.2174/138161208784705504
Bembi, B., Marchetti, F., Guerci, V. I., Ciana, G., Addobbati, R., Grasso, D., et al. (2006). Substrate reduction therapy in the infantile form of Tay-Sachs disease. Neurology 66, 278–280. doi: 10.1212/01.wnl.0000194225.78917.de
Berardi, A. S., Pannuzzo, G., Graziano, A., Costantino-Ceccarini, E., Piomboni, P., and Luddi, A. (2014). Pharmacological chaperones increase residual beta-galactocerebrosidase activity in fibroblasts from Krabbe patients. Mol. Genet. Metab. 112, 294–301. doi: 10.1016/j.ymgme.2014.05.009
Biffi, A. (2016). Gene therapy for lysosomal storage disorders: a good start. Hum. Mol. Genet. 25, R65–R75. doi: 10.1093/hmg/ddv457
Bley, A. E., Giannikopoulos, O. A., Hayden, D., Kubilus, K., Tifft, C. J., and Eichler, F. S. (2011). Natural history of infantile G(M2) gangliosidosis. Pediatrics 128, e1233–e1241. doi: 10.1542/peds.2011-0078
Boomkamp, S. D., Rountree, J. S., Neville, D. C., Dwek, R. A., Fleet, G. W., and Butters, T. D. (2010). Lysosomal storage of oligosaccharide and glycosphingolipid in imino sugar treated cells. Glycoconj. J. 27, 297–308. doi: 10.1007/s10719-010-9278-1
Bradbury, A. M., Cochran, J. N., McCurdy, V. J., Johnson, A. K., Brunson, B. L., Gray-Edwards, H., et al. (2013). Therapeutic response in feline sandhoff disease despite immunity to intracranial gene therapy. Mol. Ther. 21, 1306–1315. doi: 10.1038/mt.2013.86
Cachon-Gonzalez, M. B., Wang, S. Z., Lynch, A., Ziegler, R., Cheng, S. H., and Cox, T. M. (2006). Effective gene therapy in an authentic model of Tay-Sachs-related diseases. Proc. Natl. Acad. Sci. U.S.A. 103, 10373–10378. doi: 10.1073/pnas.0603765103
Cachon-Gonzalez, M. B., Wang, S. Z., McNair, R., Bradley, J., Lunn, D., Ziegler, R., et al. (2012). Gene transfer corrects acute GM2 gangliosidosis–potential therapeutic contribution of perivascular enzyme flow. Mol. Ther. 20, 1489–1500. doi: 10.1038/mt.2012.44
Cachon-Gonzalez, M. B., Wang, S. Z., Ziegler, R., Cheng, S. H., and Cox, T. M. (2014). Reversibility of neuropathology in Tay-Sachs-related diseases. Hum. Mol. Genet. 23, 730–748. doi: 10.1093/hmg/ddt459
Cohen-Tannoudji, M., Marchand, P., Akli, S., Sheardown, S. A., Puech, J. P., Kress, C., et al. (1995). Disruption of murine Hexa gene leads to enzymatic deficiency and to neuronal lysosomal storage, similar to that observed in Tay-Sachs disease. Mamm. Genome 6, 844–849. doi: 10.1007/BF00292433
Connock, M., Burls, A., Frew, E., Fry-Smith, A., Juarez-Garcia, A., McCabe, C., et al. (2006). The clinical effectiveness and cost-effectiveness of enzyme replacement therapy for Gaucher’s disease: a systematic review. Health Technol. Assess 10, iii–136. doi: 10.3310/hta10240
Coutinho, M. F., Santos, J. I., and Alves, S. (2016). Less is more: substrate reduction therapy for lysosomal storage disorders. Int. J. Mol. Sci. 17:E1065. doi: 10.3390/ijms17071065
Deik, A., and Saunders-Pullman, R. (2014). Atypical presentation of late-onset Tay-Sachs disease. Muscle Nerve 49, 768–771. doi: 10.1002/mus.24146
Deodato, F., Procopio, E., Rampazzo, A., Taurisano, R., Donati, M. A., Dionisi-Vici, C., et al. (2017). The treatment of juvenile/adult GM1-gangliosidosis with Miglustat may reverse disease progression. Metab. Brain Dis. 32, 1529–1536. doi: 10.1007/s11011-017-0044-y
Dersh, D., Iwamoto, Y., and Argon, Y. (2016). Tay-Sachs disease mutations in HEXA target the alpha chain of hexosaminidase A to endoplasmic reticulum-associated degradation. Mol. Biol. Cell 27, 3813–3827. doi: 10.1091/mbc.E16-01-0012
Eng, C. M., Guffon, N., Wilcox, W. R., Germain, D. P., Lee, P., Waldek, S., et al. (2001). Safety and efficacy of recombinant human alpha-galactosidase A replacement therapy in Fabry’s disease. N. Engl. J. Med. 345, 9–16. doi: 10.1056/NEJM200107053450102
Ferreira, C. R., and Gahl, W. A. (2017). Lysosomal storage diseases. Transl. Sci. Rare Dis. 2, 1–71. doi: 10.3233/TRD-160005
Galieva, L. R., Mukhamedshina, Y. O., Arkhipova, S. S., and Rizvanov, A. A. (2017). Human umbilical cord blood cell transplantation in neuroregenerative strategies. Front. Pharmacol. 8:628. doi: 10.3389/fphar.2017.00628
Goddard-Borger, E. D., Tropak, M. B., Yonekawa, S., Tysoe, C., Mahuran, D. J., and Withers, S. G. (2012). Rapid assembly of a library of lipophilic iminosugars via the thiol-ene reaction yields promising pharmacological chaperones for the treatment of Gaucher disease. J. Med. Chem. 55, 2737–2745. doi: 10.1021/jm201633y
Golebiowski, D., van der Bom, I. M. J., Kwon, C. S., Miller, A. D., Petrosky, K., Bradbury, A. M., et al. (2017). Direct intracranial injection of AAVrh8 encoding monkey beta-N-Acetylhexosaminidase causes neurotoxicity in the primate brain. Hum. Gene Ther. 28, 510–522. doi: 10.1089/hum.2016.109
Gonzalez, R., Hamblin, M. H., and Lee, J. P. (2016). Neural stem Cell transplantation and CNS diseases. CNS Neurol Disord Drug Targets 15, 881–886. doi: 10.2174/1871527315666160815164247
Gray, S. J., Woodard, K. T., and Samulski, R. J. (2010). Viral vectors and delivery strategies for CNS gene therapy. Ther. Deliv. 1, 517–534. doi: 10.4155/tde.10.50
Gray-Edwards, H. L., Randle, A. N., Maitland, S. A., Benatti, H. R., Hubbard, S. M., Canning, P. F., et al. (2018). Adeno-associated virus gene therapy in a sheep model of Tay-Sachs disease. Hum. Gene Ther. 29, 312–326. doi: 10.1089/hum.2017.163
Guidotti, J., Akli, S., Castelnau-Ptakhine, L., Kahn, A., and Poenaru, L. (1998). Retrovirus-mediated enzymatic correction of Tay-Sachs defect in transduced and non-transduced cells. Hum. Mol. Genet. 7, 831–838. doi: 10.1093/hmg/7.5.831
Guidotti, J. E., Mignon, A., Haase, G., Caillaud, C., McDonell, N., Kahn, A., et al. (1999). Adenoviral gene therapy of the Tay-Sachs disease in hexosaminidase A-deficient knock-out mice. Hum. Mol. Genet. 8, 831–838. doi: 10.1093/hmg/8.5.831
Harmatz, P., Muenzer, J., Burton, B. K., Ficicioglu, C., Lau, H. A., Leslie, N. D., et al. (2018). Update on phase 1/2 clinical trials for MPS I and MPS II using ZFN-mediated in vivo genome editing. Mol. Genet. Metab. 123, S59–S60. doi: 10.1016/j.ymgme.2017.12.143
Harmatz, P., Whitley, C. B., Waber, L., Pais, R., Steiner, R., Plecko, B., et al. (2004). Enzyme replacement therapy in mucopolysaccharidosis VI (Maroteaux-Lamy syndrome). J. Pediatr. 144, 574–580. doi: 10.1016/j.jpeds.2004.03.018
Hassan, S. S., Trenado, C., Elben, S., Schnitzler, A., and Groiss, S. J. (2018). Alteration of cortical excitability and its modulation by Miglustat in Niemann-Pick disease type C. J. Clin. Neurosci. 47, 214–217. doi: 10.1016/j.jocn.2017.10.011
Hayase, T., Shimizu, J., Goto, T., Nozaki, Y., Mori, M., Takahashi, N., et al. (2010). Unilaterally and rapidly progressing white matter lesion and elevated cytokines in a patient with Tay-Sachs disease. Brain Dev. 32, 244–247. doi: 10.1016/j.braindev.2009.01.007
Hou, Y., Tse, R., and Mahuran, D. J. (1996). Direct determination of the substrate specificity of the alpha-active site in heterodimeric beta-hexosaminidase A. Biochemistry 35, 3963–3969. doi: 10.1021/bi9524575
Hubbes, M., Callahan, J., Gravel, R., and Mahuran, D. (1989). The amino-terminal sequences in the pro-alpha and -beta polypeptides of human lysosomal beta-hexosaminidase A and B are retained in the mature isozymes. FEBS Lett. 249, 316–320. doi: 10.1016/0014-5793(89)80649-0
Jacobs, J. F., Willemsen, M. A., Groot-Loonen, J. J., Wevers, R. A., and Hoogerbrugge, P. M. (2005). Allogeneic BMT followed by substrate reduction therapy in a child with subacute Tay-Sachs disease. Bone Marrow Transplant. 36, 925–926. doi: 10.1038/sj.bmt.1705155
Jakobkiewicz-Banecka, J., Wegrzyn, A., and Wegrzyn, G. (2007). Substrate deprivation therapy: a new hope for patients suffering from neuronopathic forms of inherited lysosomal storage diseases. J. Appl. Genet. 48, 383–388. doi: 10.1007/BF03195237
Jarnes Utz, J. R., Kim, S., King, K., Ziegler, R., Schema, L., Redtree, E. S., et al. (2017). Infantile gangliosidoses: mapping a timeline of clinical changes. Mol. Genet. Metab. 121, 170–179. doi: 10.1016/j.ymgme.2017.04.011
Jeyakumar, M., Thomas, R., Elliot-Smith, E., Smith, D. A., van der Spoel, A. C., d’Azzo, A., et al. (2003). Central nervous system inflammation is a hallmark of pathogenesis in mouse models of GM1 and GM2 gangliosidosis. Brain 126(Pt 4), 974–987. doi: 10.1093/brain/awg089
Kaback, M. M., and Desnick, R. J. (1993). “Hexosaminidase a deficiency,” in GeneReviews((R)), eds M. P. Adam, H. H. Ardinger, R. A. Pagon, S. E. Wallace, L. J. H. Bean, K. Stephens, et al. (Seattle, WA: University of Washington).
Karumuthil-Melethil, S., Nagabhushan Kalburgi, S., Thompson, P., Tropak, M., Kaytor, M. D., Keimel, J. G., et al. (2016). Novel vector design and hexosaminidase variant enabling self-complementary adeno-associated virus for the treatment of Tay-Sachs disease. Hum. Gene Ther. 27, 509–521. doi: 10.1089/hum.2016.013
Kato, A., Yamashita, Y., Nakagawa, S., Koike, Y., Adachi, I., Hollinshead, J., et al. (2010). 2,5-Dideoxy-2,5-imino-d-altritol as a new class of pharmacological chaperone for Fabry disease. Bioorg. Med. Chem. 18, 3790–3794. doi: 10.1016/j.bmc.2010.04.048
Klinge, L., Straub, V., Neudorf, U., and Voit, T. (2005). Enzyme replacement therapy in classical infantile pompe disease: results of a ten-month follow-up study. Neuropediatrics 36, 6–11. doi: 10.1055/s-2005-837543
Kyrkanides, S., Miller, J. H., Brouxhon, S. M., Olschowka, J. A., and Federoff, H. J. (2005). beta-hexosaminidase lentiviral vectors: transfer into the CNS via systemic administration. Brain Res. Mol. Brain Res. 133, 286–298. doi: 10.1016/j.molbrainres.2004.10.026
Lacorazza, H. D., Flax, J. D., Snyder, E. Y., and Jendoubi, M. (1996). Expression of human beta-hexosaminidase alpha-subunit gene (the gene defect of Tay-Sachs disease) in mouse brains upon engraftment of transduced progenitor cells. Nat. Med. 2, 424–429. doi: 10.1038/nm0496-424
Lange, M. C., Teive, H. A., Troiano, A. R., Bitencourt, M., Funke, V. A., Setubal, D. C., et al. (2006). Bone marrow transplantation in patients with storage diseases: a developing country experience. Arq. Neuropsiquiatr. 64, 1–4. doi: 10.1590/S0004-282X2006000100001
Lawson, C. A., and Martin, D. R. (2016). Animal models of GM2 gangliosidosis: utility and limitations. Appl. Clin. Genet. 9, 111–120. doi: 10.2147/TACG.S85354
Lemieux, M. J., Mark, B. L., Cherney, M. M., Withers, S. G., Mahuran, D. J., and James, M. N. (2006). Crystallographic structure of human beta-hexosaminidase A: interpretation of Tay-Sachs mutations and loss of GM2 ganglioside hydrolysis. J. Mol. Biol. 359, 913–929. doi: 10.1016/j.jmb.2006.04.004
Lew, R. M., Burnett, L., Proos, A. L., and Delatycki, M. B. (2015). Tay-Sachs disease: current perspectives from Australia. Appl. Clin. Genet. 8, 19–25. doi: 10.2147/TACG.S49628
Li, M. (2018). Enzyme replacement therapy: a review and its role in treating lysosomal storage diseases. Pediatr. Ann. 47, e191–e197. doi: 10.3928/19382359-20180424-01
Liu, Z., and Zhao, R. (2016). Generation of HEXA-deficient hiPSCs from fibroblasts of a Tay-Sachs disease patient. Stem Cell Res. 17, 289–291. doi: 10.1016/j.scr.2016.08.010
Maegawa, G. H., Banwell, B. L., Blaser, S., Sorge, G., Toplak, M., Ackerley, C., et al. (2009). Substrate reduction therapy in juvenile GM2 gangliosidosis. Mol. Genet. Metab. 98, 215–224. doi: 10.1016/j.ymgme.2009.06.005
Maegawa, G. H., Stockley, T., Tropak, M., Banwell, B., Blaser, S., Kok, F., et al. (2006). The natural history of juvenile or subacute GM2 gangliosidosis: 21 new cases and literature review of 134 previously reported. Pediatrics 118, e1550–e1562. doi: 10.1542/peds.2006-0588
Mahuran, D. J. (1999). Biochemical consequences of mutations causing the GM2 gangliosidoses. Biochim. Biophys. Acta 1455, 105–138. doi: 10.1016/S0925-4439(99)00074-5
Maier, T., Strater, N., Schuette, C. G., Klingenstein, R., Sandhoff, K., and Saenger, W. (2003). The X-ray crystal structure of human beta-hexosaminidase B provides new insights into Sandhoff disease. J. Mol. Biol. 328, 669–681. doi: 10.1016/S0022-2836(03)00311-5
Mark, B. L., Mahuran, D. J., Cherney, M. M., Zhao, D., Knapp, S., and James, M. N. (2003). Crystal structure of human beta-hexosaminidase B: understanding the molecular basis of Sandhoff and Tay-Sachs disease. J. Mol. Biol. 327, 1093–1109. doi: 10.1016/S0022-2836(03)00216-X
Martin, P. L., Carter, S. L., Kernan, N. A., Sahdev, I., Wall, D., Pietryga, D., et al. (2006). Results of the cord blood transplantation study (COBLT): outcomes of unrelated donor umbilical cord blood transplantation in pediatric patients with lysosomal and peroxisomal storage diseases. Biol. Blood Marrow Transplant. 12, 184–194. doi: 10.1016/j.bbmt.2005.09.016
Martino, S., Cavalieri, C., Emiliani, C., Dolcetta, D., Cusella De Angelis, M. G., Chigorno, V., et al. (2002a). Restoration of the GM2 ganglioside metabolism in bone marrow-derived stromal cells from Tay-Sachs disease animal model. Neurochem. Res. 27, 793–800.
Martino, S., Emiliani, C., Tancini, B., Severini, G. M., Chigorno, V., Bordignon, C., et al. (2002b). Absence of metabolic cross-correction in Tay-Sachs cells: implications for gene therapy. J. Biol. Chem. 277, 20177–20184. doi: 10.1074/jbc.M106164200
Martino, S., Marconi, P., Tancini, B., Dolcetta, D., De Angelis, M. G., Montanucci, P., et al. (2005). A direct gene transfer strategy via brain internal capsule reverses the biochemical defect in Tay-Sachs disease. Hum. Mol. Genet. 14, 2113–2123. doi: 10.1093/hmg/ddi216
Matsuoka, K., Tamura, T., Tsuji, D., Dohzono, Y., Kitakaze, K., Ohno, K., et al. (2011). Therapeutic potential of intracerebroventricular replacement of modified human beta-hexosaminidase B for GM2 gangliosidosis. Mol. Ther. 19, 1017–1024. doi: 10.1038/mt.2011.27
Mistri, M., Tamhankar, P. M., Sheth, F., Sanghavi, D., Kondurkar, P., Patil, S., et al. (2012). Identification of novel mutations in HEXA gene in children affected with Tay Sachs disease from India. PLoS One 7:e39122. doi: 10.1371/journal.pone.0039122
Muenzer, J., Lamsa, J. C., Garcia, A., Dacosta, J., Garcia, J., and Treco, D. A. (2002). Enzyme replacement therapy in mucopolysaccharidosis type II (Hunter syndrome): a preliminary report. Acta Paediatr. Suppl. 91, 98–99. doi: 10.1111/j.1651-2227.2002.tb03118.x
Myerowitz, R. (1997). Tay-Sachs disease-causing mutations and neutral polymorphisms in the Hex A gene. Hum. Mutat. 9, 195–208. doi: 10.1002/(SICI)1098-1004(1997)9:3<195::AID-HUMU1>3.0.CO;2-7
Myerowitz, R., Lawson, D., Mizukami, H., Mi, Y., Tifft, C. J., and Proia, R. L. (2002). Molecular pathophysiology in Tay-Sachs and Sandhoff diseases as revealed by gene expression profiling. Hum. Mol. Genet. 11, 1343–1350. doi: 10.1093/hmg/11.11.1343
Nakamura, T., Sato, K., and Hamada, H. (2003). Reduction of natural adenovirus tropism to the liver by both ablation of fiber-coxsackievirus and adenovirus receptor interaction and use of replaceable short fiber. J. Virol. 77, 2512–2521. doi: 10.1128/JVI.77.4.2512-2521.2003
Nestrasil, I., Ahmed, A., Utz, J. M., Rudser, K., Whitley, C. B., and Jarnes-Utz, J. R. (2018). Distinct progression patterns of brain disease in infantile and juvenile gangliosidoses: volumetric quantitative MRI study. Mol. Genet. Metab. 123, 97–104. doi: 10.1016/j.ymgme.2017.12.432
Norflus, F., Tifft, C. J., McDonald, M. P., Goldstein, G., Crawley, J. N., Hoffmann, A., et al. (1998). Bone marrow transplantation prolongs life span and ameliorates neurologic manifestations in Sandhoff disease mice. J. Clin. Invest. 101, 1881–1888. doi: 10.1172/JCI2127
Osher, E., Fattal-Valevski, A., Sagie, L., Urshanski, N., Sagiv, N., Peleg, L., et al. (2015). Effect of cyclic, low dose pyrimethamine treatment in patients with Late Onset Tay Sachs: an open label, extended pilot study. Orphanet J. Rare Dis. 10:45. doi: 10.1186/s13023-015-0260-7
Osmon, K. J., Woodley, E., Thompson, P., Ong, K., Karumuthil-Melethil, S., Keimel, J. G., et al. (2016). Systemic gene transfer of a Hexosaminidase variant using an scaav9.47 vector corrects GM2 gangliosidosis in sandhoff mice. Hum. Gene Ther. 27, 497–508. doi: 10.1089/hum.2016.015
Parenti, G. (2009). Treating lysosomal storage diseases with pharmacological chaperones: from concept to clinics. EMBO Mol. Med. 1, 268–279. doi: 10.1002/emmm.200900036
Parenti, G., Pignata, C., Vajro, P., and Salerno, M. (2013). New strategies for the treatment of lysosomal storage diseases (review). Int. J. Mol. Med. 31, 11–20. doi: 10.3892/ijmm.2012.1187
Patterson, M. C. (2013). Gangliosidoses. Handb. Clin. Neurol. 113, 1707–1708. doi: 10.1016/B978-0-444-59565-2.00039-3
Pereira, D. M., Valentao, P., and Andrade, P. B. (2018). Tuning protein folding in lysosomal storage diseases: the chemistry behind pharmacological chaperones. Chem. Sci. 9, 1740–1752. doi: 10.1039/c7sc04712f
Phaneuf, D., Wakamatsu, N., Huang, J. Q., Borowski, A., Peterson, A. C., Fortunato, S. R., et al. (1996). Dramatically different phenotypes in mouse models of human Tay-Sachs and Sandhoff diseases. Hum. Mol. Genet. 5, 1–14. doi: 10.1093/hmg/5.1.1
Pineda, M., Walterfang, M., and Patterson, M. C. (2018). Miglustat in Niemann-Pick disease type C patients: a review. Orphanet J. Rare Dis. 13:140. doi: 10.1186/s13023-018-0844-0
Platt, F. M., Jeyakumar, M., Andersson, U., Heare, T., Dwek, R. A., and Butters, T. D. (2003). Substrate reduction therapy in mouse models of the glycosphingolipidoses. Philos. Trans. R. Soc. Lond. B Biol. Sci. 358, 947–954. doi: 10.1098/rstb.2003.1279
Platt, F. M., Neises, G. R., Reinkensmeier, G., Townsend, M. J., Perry, V. H., Proia, R. L., et al. (1997). Prevention of lysosomal storage in Tay-Sachs mice treated with N-butyldeoxynojirimycin. Science 276, 428–431. doi: 10.1126/science.276.5311.428
Porter, B. F., Lewis, B. C., Edwards, J. F., Alroy, J., Zeng, B. J., Torres, P. A., et al. (2011). Pathology of GM2 gangliosidosis in Jacob sheep. Vet. Pathol. 48, 807–813. doi: 10.1177/0300985810388522
Porto, C., Cardone, M., Fontana, F., Rossi, B., Tuzzi, M. R., Tarallo, A., et al. (2009). The pharmacological chaperone N-butyldeoxynojirimycin enhances enzyme replacement therapy in Pompe disease fibroblasts. Mol. Ther. 17, 964–971. doi: 10.1038/mt.2009.53
Regier, D. S., Proia, R. L., D’Azzo, A., and Tifft, C. J. (2016). The GM1 and GM2 gangliosidoses: natural history and progress toward therapy. Pediatr. Endocrinol. Rev. 13(Suppl. 1), 663–673.
Renaud, D., and Brodsky, M. (2016). GM2-gangliosidosis, AB variant: clinical, ophthalmological, MRI, and molecular findings. JIMD Rep. 25, 83–86. doi: 10.1007/8904_2015_469
Rovelli, A. M. (2008). The controversial and changing role of haematopoietic cell transplantation for lysosomal storage disorders: an update. Bone Marrow Transplant. 41(Suppl. 2), S87–S89. doi: 10.1038/bmt.2008.62
Sakuraba, H., Sawada, M., Matsuzawa, F., Aikawa, S., Chiba, Y., Jigami, Y., et al. (2006). Molecular pathologies of and enzyme replacement therapies for lysosomal diseases. CNS Neurol Disord. Drug Targets 5, 401–413. doi: 10.2174/187152706777950738
Sandhoff, K. (2016). Neuronal sphingolipidoses: membrane lipids and sphingolipid activator proteins regulate lysosomal sphingolipid catabolism. Biochimie 130, 146–151. doi: 10.1016/j.biochi.2016.05.004
Sandhoff, K., and Christomanou, H. (1979). Biochemistry and genetics of gangliosidoses. Hum. Genet. 50, 107–143. doi: 10.1007/BF00390234
Sandhoff, K., and Harzer, K. (2013). Gangliosides and gangliosidoses: principles of molecular and metabolic pathogenesis. J. Neurosci. 33, 10195–10208. doi: 10.1523/JNEUROSCI.0822-13.2013
Sango, K., Yamanaka, S., Hoffmann, A., Okuda, Y., Grinberg, A., Westphal, H., et al. (1995). Mouse models of Tay-Sachs and Sandhoff diseases differ in neurologic phenotype and ganglioside metabolism. Nat. Genet. 11, 170–176. doi: 10.1038/ng1095-170
Seyrantepe, V., Demir, S. A., Timur, Z. K., Von Gerichten, J., Marsching, C., Erdemli, E., et al. (2018). Murine Sialidase Neu3 facilitates GM2 degradation and bypass in mouse model of Tay-Sachs disease. Exp. Neurol. 299(Pt A), 26–41. doi: 10.1016/j.expneurol.2017.09.012
Sonderfeld-Fresko, S., and Proia, R. L. (1989). Analysis of the glycosylation and phosphorylation of the lysosomal enzyme, beta-hexosaminidase B, by site-directed mutagenesis. J. Biol. Chem. 264, 7692–7697.
Sorrentino, N. C., D’Orsi, L., Sambri, I., Nusco, E., Monaco, C., Spampanato, C., et al. (2013). A highly secreted sulphamidase engineered to cross the blood-brain barrier corrects brain lesions of mice with mucopolysaccharidoses type IIIA. EMBO Mol. Med. 5, 675–690. doi: 10.1002/emmm.201202083
Stepien, K. M., Lum, S. H., Wraith, J. E., Hendriksz, C. J., Church, H. J., Priestman, D., et al. (2017). Haematopoietic stem cell transplantation arrests the progression of neurodegenerative disease in late-onset tay-sachs disease. JIMD Rep. 41, 17–23. doi: 10.1007/8904_2017_76
Taniike, M., Yamanaka, S., Proia, R. L., Langaman, C., Bone-Turrentine, T., and Suzuki, K. (1995). Neuropathology of mice with targeted disruption of Hexa gene, a model of Tay-Sachs disease. Acta Neuropathol. 89, 296–304. doi: 10.1007/BF00309622
Torres, P. A., Zeng, B. J., Porter, B. F., Alroy, J., Horak, F., Horak, J., et al. (2010). Tay-Sachs disease in Jacob sheep. Mol. Genet. Metab. 101, 357–363. doi: 10.1016/j.ymgme.2010.08.006
Tropak, M. B., Yonekawa, S., Karumuthil-Melethil, S., Thompson, P., Wakarchuk, W., Gray, S. J., et al. (2016). Construction of a hybrid beta-hexosaminidase subunit capable of forming stable homodimers that hydrolyze GM2 ganglioside in vivo. Mol. Ther. Methods Clin. Dev. 3:15057. doi: 10.1038/mtm.2015.57
Tsuji, D., Akeboshi, H., Matsuoka, K., Yasuoka, H., Miyasaki, E., Kasahara, Y., et al. (2011). Highly phosphomannosylated enzyme replacement therapy for GM2 gangliosidosis. Ann. Neurol. 69, 691–701. doi: 10.1002/ana.22262
Utz, J. R., Crutcher, T., Schneider, J., Sorgen, P., and Whitley, C. B. (2015). Biomarkers of central nervous system inflammation in infantile and juvenile gangliosidoses. Mol. Genet. Metab. 114, 274–280. doi: 10.1016/j.ymgme.2014.11.015
Wada, R., Tifft, C. J., and Proia, R. L. (2000). Microglial activation precedes acute neurodegeneration in Sandhoff disease and is suppressed by bone marrow transplantation. Proc. Natl. Acad. Sci. U.S.A. 97, 10954–10959. doi: 10.1073/pnas.97.20.10954
Wasserstein, M. P., Caggana, M., Bailey, S. M., Desnick, R. J., Edelmann, L., Estrella, L., et al. (2018). The New York pilot newborn screening program for lysosomal storage diseases: report of the First 65,000 Infants. Genet. Med. doi: 10.1038/s41436-018-0129-y [Epub ahead of print].
Weitz, G., and Proia, R. L. (1992). Analysis of the glycosylation and phosphorylation of the alpha-subunit of the lysosomal enzyme, beta-hexosaminidase A, by site-directed mutagenesis. J. Biol. Chem. 267, 10039–10044.
Wolfe, D., Goins, W. F., Yamada, M., Moriuchi, S., Krisky, D. M., Oligino, T. J., et al. (1999). Engineering herpes simplex virus vectors for CNS applications. Exp. Neurol. 159, 34–46. doi: 10.1006/exnr.1999.7158
Wraith, J. E. (2006). Limitations of enzyme replacement therapy: current and future. J. Inherit. Metab. Dis. 29, 442–447. doi: 10.1007/s10545-006-0239-6
Wraith, J. E., Clarke, L. A., Beck, M., Kolodny, E. H., Pastores, G. M., Muenzer, J., et al. (2004). Enzyme replacement therapy for mucopolysaccharidosis I: a randomized, double-blinded, placebo-controlled, multinational study of recombinant human alpha-L-iduronidase (laronidase). J. Pediatr. 144, 581–588. doi: 10.1016/j.jpeds.2004.01.046
Wu, Y. P., and Proia, R. L. (2004). Deletion of macrophage-inflammatory protein 1 alpha retards neurodegeneration in Sandhoff disease mice. Proc. Natl. Acad. Sci. U.S.A. 101, 8425–8430. doi: 10.1073/pnas.0400625101
Yuziuk, J. A., Bertoni, C., Beccari, T., Orlacchio, A., Wu, Y. Y., Li, S. C., et al. (1998). Specificity of mouse GM2 activator protein and beta-N-acetylhexosaminidases A and B. Similarities and differences with their human counterparts in the catabolism of GM2. J. Biol. Chem. 273, 66–72. doi: 10.1074/jbc.273.1.66
Zeng, B. J., Torres, P. A., Viner, T. C., Wang, Z. H., Raghavan, S. S., Alroy, J., et al. (2008). Spontaneous appearance of Tay-Sachs disease in an animal model. Mol. Genet. Metab. 95, 59–65. doi: 10.1016/j.ymgme.2008.06.010
Keywords: lysosomal storage diseases, GM2-gangliosidosis, β-hexosaminidase, Tay-Sachs disease, neurodegeneration, inflammation, gene therapy, bone marrow transplantation
Citation: Solovyeva VV, Shaimardanova AA, Chulpanova DS, Kitaeva KV, Chakrabarti L and Rizvanov AA (2018) New Approaches to Tay-Sachs Disease Therapy. Front. Physiol. 9:1663. doi: 10.3389/fphys.2018.01663
Received: 28 September 2018; Accepted: 05 November 2018;
Published: 20 November 2018.
Edited by:
Anna Maria Giudetti, University of Salento, ItalyReviewed by:
Daniele Vergara, University of Salento, ItalyCopyright © 2018 Solovyeva, Shaimardanova, Chulpanova, Kitaeva, Chakrabarti and Rizvanov. This is an open-access article distributed under the terms of the Creative Commons Attribution License (CC BY). The use, distribution or reproduction in other forums is permitted, provided the original author(s) and the copyright owner(s) are credited and that the original publication in this journal is cited, in accordance with accepted academic practice. No use, distribution or reproduction is permitted which does not comply with these terms.
*Correspondence: Albert A. Rizvanov, cml6dmFub3ZAZ21haWwuY29t
Disclaimer: All claims expressed in this article are solely those of the authors and do not necessarily represent those of their affiliated organizations, or those of the publisher, the editors and the reviewers. Any product that may be evaluated in this article or claim that may be made by its manufacturer is not guaranteed or endorsed by the publisher.
Research integrity at Frontiers
Learn more about the work of our research integrity team to safeguard the quality of each article we publish.