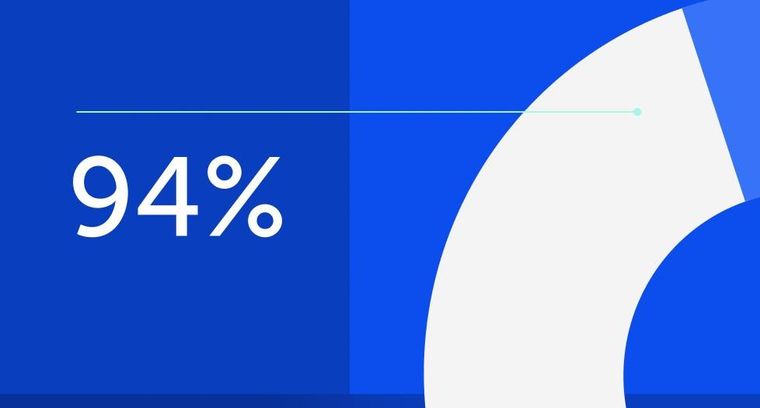
94% of researchers rate our articles as excellent or good
Learn more about the work of our research integrity team to safeguard the quality of each article we publish.
Find out more
REVIEW article
Front. Physiol., 22 August 2018
Sec. Integrative Physiology
Volume 9 - 2018 | https://doi.org/10.3389/fphys.2018.01130
This article is part of the Research TopicPhysico-Chemical Control of Cell FunctionView all 20 articles
Classical approaches to engineer skeletal muscle tissue based on current regenerative and surgical procedures still do not meet the desired outcome for patient applications. Besides the evident need to create functional skeletal muscle tissue for the repair of volumetric muscle defects, there is also growing demand for platforms to study muscle-related diseases, such as muscular dystrophies or sarcopenia. Currently, numerous studies exist that have employed a variety of biomaterials, cell types and strategies for maturation of skeletal muscle tissue in 2D and 3D environments. However, researchers are just at the beginning of understanding the impact of different culture settings and their biochemical (growth factors and chemical changes) and biophysical cues (mechanical properties) on myogenesis. With this review we intend to emphasize the need for new in vitro skeletal muscle (disease) models to better recapitulate important structural and functional aspects of muscle development. We highlight the importance of choosing appropriate system components, e.g., cell and biomaterial type, structural and mechanical matrix properties or culture format, and how understanding their interplay will enable researchers to create optimized platforms to investigate myogenesis in healthy and diseased tissue. Thus, we aim to deliver guidelines for experimental designs to allow estimation of the potential influence of the selected skeletal muscle tissue engineering setup on the myogenic outcome prior to their implementation. Moreover, we offer a workflow to facilitate identifying and selecting different analytical tools to demonstrate the successful creation of functional skeletal muscle tissue. Ultimately, a refinement of existing strategies will lead to further progression in understanding important aspects of muscle diseases, muscle aging and muscle regeneration to improve quality of life of patients and enable the establishment of new treatment options.
The field of regenerative medicine and tissue engineering (TE) is still one of the fastest growing research areas in biomedical science. Previous TE efforts mostly focused on tissues and organs that are associated with diseases occurring at high frequencies in 1st world countries, such as the heart and the musculoskeletal apparatus with a strong emphasis on bone, cartilage, and ligaments. Muscle tissue, which for long has been relatively neglected, has gained more attention in the TE community recently. The view on muscle evolved from being the tissue mainly responsible for locomotion, thermogenesis and postural support to an endocrine organ able to secrete cytokines (termed myokines) that exert beneficial effects on surrounding tissues (Pedersen, 2011).
Tissue-specific stem cells, termed satellite cells (Beauchamp et al., 2000; Zammit et al., 2004; Yin et al., 2013; Han et al., 2016) are responsible for maintaining the regenerative capacity of skeletal muscle. Upon injury, satellite cells can re-enter the cell cycle, proliferate and either fuse to existing myofibers or generate myofibers de novo. Since their discovery in 1961 (Katz, 1961; Mauro, 1961), extensive research has been conducted on the regulatory mechanisms guiding satellite cell activity and their role in healthy and diseased muscle (Seale and Rudnicki, 2000; Zammit et al., 2004, 2006; Yin et al., 2013).
Skeletal muscle TE (SMTE) aims at the functional restoration of either lost, atrophic or impaired muscle tissue. Of late, the field has particularly emphasized using cellular and acellular therapeutic approaches for pathological muscle states such as muscular dystrophies, sarcopenia, or traumatic volumetric muscle loss. In the young, regeneration generally occurs efficiently as skeletal muscle can cope with slight injuries due to its high regenerative potential. However, regeneration is inefficient when trauma causes extensive damage or when the muscle is affected by a chronic pathology. This is especially severe in the elderly, where the regenerative capacity of muscle is diminished due to a decrease in the muscle stem cell pool. This leads to progressive replacement of muscle with scar and fat tissue, causing substantial deteriorations in muscle function and motility and thus quality of life. In addition, the loss of muscle associated with aging (sarcopenia) affects a growing number of patients as the global increase in life expectancy leads to population aging. Thus there is an unmet clinical need for approaches to restore or maintain muscle function, especially in the older population which is highly affected by muscle wasting and atrophy (Chargé and Rudnicki, 2004; Ryall et al., 2008; Carosio et al., 2011; Blau et al., 2015). In contrast to sarcopenia, genetic muscle diseases, such as muscular dystrophies (MDs), result in progressive muscle weakening and breakdown starting already in childhood or middle age. MDs are a group of more than 30 rare hereditary diseases caused by mutations leading to either a dysfunction in, or lack of proteins essential for muscle stability (Theadom et al., 2014; Smith et al., 2016). MDs greatly vary in the type of muscle affected (some forms of MD may affect cardiac muscle), extent of muscle weakness, the age of onset, the rate of progression and the pattern of inheritance (Theadom et al., 2014).
Duchenne muscular dystrophy (DMD) is the most common MD affecting approximately 1 in 5,000 males (Goyenvalle et al., 2011; Mah et al., 2014; Romitti et al., 2015; Stark, 2015; Yiu and Kornberg, 2015). DMD is caused by the absence of functional dystrophin, either through deletion, point mutations, insertions or duplication. Dystrophin is a structural protein, which acts as a linker between the cytoskeleton (via the dystroglycan complex) and the surrounding extracellular matrix (ECM). Dystrophin stabilizes muscle cells under mechanical load and is essential for the maintenance of the intracellular structural organization of muscle cytoskeletal proteins in the contractile apparatus (Ervasti and Sonnemann, 2008; Constantin, 2014; Gawlik et al., 2014). Thus, lack of dystrophin predisposes muscle fibers to fragility in response to mechanical forces, leading to continuous cycles of muscle de- and regeneration (Serrano et al., 2011). Recent evidence additionally suggests that dystrophin is directly involved in regulating satellite cell behavior and that satellite cells from dystrophin knock out animals show lower proliferation rates as well as functional impairment (Sacco et al., 2010; Dumont et al., 2015b; Almada and Wagers, 2016; Dumont and Rudnicki, 2016). As a result, the muscle stem cell (satellite cell) pool is prematurely exhausted, a phenomenon somewhat analogous with aging (Webster and Blau, 1990), which eventually leads to muscle weakness, loss of motility and, in the worst case, premature death (Emery, 1993). Other MD types include Becker MD, a less severe variant of DMD, Emery-Dreifuss MD, facioscapulohumeral MD, congenital MD, limb-girdle MD or myotonic MD (Theadom et al., 2014).
To date, there is no cure for MDs. Although symptomatic treatments such as physical or drug therapies are used to delay disease progression, the prognosis for people with chronic muscle pathologies is poor. This creates a considerable world-wide socioeconomic burden for health systems, patients and caregivers alike. Sarcopenia accounts for roughly $18.5 billion per year in direct healthcare costs in the U.S. (Janssen et al., 2004; Beaudart et al., 2014). A cross-sectional study in 2014 reported the mean annual direct costs per DMD patient to range from 23,920$ to 54,270$ in Europe and the U.S., which is 7 to 16 times higher than the mean annual per capita health expenditures in these countries (Landfeldt et al., 2014). A more recent study focusing on European DMD patients and their caregivers provided similar figures but identified direct non-healthcare costs as the main part of total annual costs (Cavazza et al., 2016).
In the past, research on regenerative therapies for diseased skeletal muscle mostly focused on methods to deliver healthy myogenic cells or to restore the endogenous myogenic potential of satellite cells (Dumont et al., 2015a). Although satellite cell transplantation holds great therapeutic potential for MDs, the vast number of cells needed for treatment and their phenotypic changes after prolonged in vitro culture limit this approach. In addition to the restoration of the stem cell pool and host myofiber repair, healthy myogenic donor cells can also act as vectors to (re)establish expression of normal (wild-type) alleles in the muscle fibers they fuse to (Partridge et al., 1989). However, the pathomechanisms leading to MD phenotypes, muscle wasting, and atrophy are still not fully understood. In addition, the fact that some MD animal models do not faithfully recapitulate the respective disease creates another burden for translation of novel therapies into clinics. Therefore, tissue engineered in vitro muscle (disease) model systems can serve as an alternate pre-clinical approach to gain further insight into the molecular causes and potential treatments of chronic pathological muscle states.
Current clinical strategies to restore muscle function are limited to symptomatic treatments and, consequently, healthcare costs are progressively rising; e.g., healthcare costs of direct and indirect traumatic injury in the year 2000 was greater than $400 billion in the US (Corso et al., 2006). SMTE constitutes a promising tool to lower this immense socioeconomic burden, as it enables the creation of new muscle to replace lost tissue without the need of donor tissue. Furthermore, SMTE can be used to study muscle development, and the impact of biomaterials and mechanical cues on myogenesis and muscular disorders in in vitro (disease) models (Juhas et al., 2015). Conducting traditional studies on muscle biology in 3D settings, which more closely mimic the physiological microenvironment of the whole organ (Bursac et al., 2015), is the new state of the art in this rapidly growing field (Figure 1). However, so far, TE only successfully entered clinics when it comes to skin, bone or cartilage replacement and regeneration (Horch et al., 2000; Chang et al., 2003; Kojima et al., 2003; Kopp et al., 2004; Oakes, 2004; Vangsness et al., 2004).
Figure 1. Advances in skeletal muscle tissue engineering—from classic to functional approaches. Until recently, the classic tissue engineering approach was the combination of the following components: biomaterials, cells, and growth factors. In recent years, this classic triad was combined with novel methodologies allowing for more biomimetic approaches. Advances in cross-linking chemistry made it possible to link growth factors to the biomaterial or to provide growth factor binding sites. In addition, guidance cues like patterning or alignment of the biomaterial, as well as the mechanical properties, have been demonstrated to significantly influence cell behavior such as adhesion, migration, and maturation. Likewise, the number of cell types that can potentially be used has increased ranging from cell lines and primary cells to muscle stem cells and cells with mesenchymal stem cells characteristics. One of the major advances in the past has been the incorporation of dynamic culture systems into existing SMTE approaches to improve tissue maturation. In this respect, the most commonly used techniques are electrical or mechanical stimulation via sophisticated bioreactor systems. These bioreactors allow controlled provision of different mechanical or electrical stimuli to drive both early myogenesis and functional maturation. GF, growth factor; 2D, 2-dimensional; 3D, 3-dimensional; SCs, stem cells; IGF, insulin growth factor; FGF, fibroblast growth factor; PDGF, platelet derived growth factor; VEGF, vascular endothelial growth factor.
Current clinical approaches to compensate for lost skeletal muscle tissue are to transfer skeletal muscle tissue from other sites of the body to the area of injury (free functional muscle transfer). However, this causes donor site morbidity and an extra surgical procedure resulting in additional stress for the patient (Qazi et al., 2015). The gold standard is the use of freestanding flaps which include functional vessels as tissue grafts. Although free functional muscle transfer is still considered the best option for restoring function in otherwise non-reconstructable muscles, a return to pre-injury levels of muscle strength and functionality does not usually occur. Thus, many research groups are now focusing on in vitro SMTE, providing new remarkable data for this field, some of which will be discussed in more detail in the subsequent sections (Engler et al., 2004; Huang et al., 2004; Matsumoto et al., 2007; Lam et al., 2009; van der Schaft et al., 2013; Kurth et al., 2015; Bersini et al., 2016). To date, the majority of in vitro SMTE strategies aim at creating functional skeletal muscle tissue in the lab to offer new therapeutic possibilities for patients suffering from volumetric muscle loss, sarcopenia or genetic muscle disorders (Law et al., 1993; Guettier-Sigrist et al., 1998). Given the current clinical treatment limitations and the rising prevalence of pathological muscle states (especially sarcopenia), these patients would greatly benefit from further research on alternative therapeutic approaches.
Another approach is in vivo SMTE which involves introducing cells with myogenic potential (Bach et al., 2004), either as bolus injections or in combination with a scaffold biomaterial, into the site of injury to form and regenerate new muscle tissue (McCullen et al., 2011). However, this strategy is limited by the vast amount of cells needed (Bach et al., 2004). Alternatively, the cell-free approach of in situ SMTE has been introduced (Jana et al., 2013; Wang et al., 2014), where instructive biomaterials are grafted into a muscle defect to trigger the endogenous regenerative potential and regenerate the diseased tissue via release of bioactive signaling molecules from the biomaterial implanted into the patient (Qazi et al., 2015). Ex vivo SMTE demonstrates an alternative strategy to in vivo approaches, where autologous cells are expanded in cell culture beforehand and eventually reintroduced into the defect site for regeneration (Barrilleaux et al., 2006; Stern et al., 2009).
With this review we would like to highlight the state of the art in SMTE, trigger ideas for refinements and provide the scientific community with putative strategies and criteria to increase the performance and maturity of tissue engineered muscle. Additionally, we give an outlook on future challenges and general considerations for SMTE applications in healthy and diseased muscle.
In vitro SMTE relies on efficient maturation strategies to generate functional 3D skeletal muscle constructs, which firstly requires biomaterials as scaffolds. These scaffold matrices should offer adequate physicochemical properties as well as bioactive cues like incorporated growth factors to enhance myogenic differentiation or cell adhesion motives to improve cellular attachment. Additionally, potent myogenic cells that are able to differentiate into mature myotubes under appropriate environmental conditions are a prerequisite (Bursac et al., 2015). Finally, effective stimulation strategies in the form of mechanical, electrical or electromechanical stimulation are needed to trigger cell alignment, fusion, and differentiation (Figure 2). After densely packed arrays of aligned myotubes are generated, the ultimate goal is to implement methods to (pre)vascularize and innervate such muscle constructs before they can serve as functional transplants.
Figure 2. Differences in experimental design of skeletal muscle tissue engineering approaches influence outcome. The choice of the biomaterial and its biophysical properties influence the TE construct in terms of cell adhesion, migration, morphology, proliferation, and differentiation. Notably, differentiation of muscle cells into contractile myofibers is highly dependent on factors such as matrix elasticity, porosity or the availability of growth factors within the construct. The selection of the appropriate cell type is of equal importance as it partially predetermines which scientific questions can be answered using a given SMTE approach. Thus, changing cell types within the same SMTE setup can increase its application range, from studies on different stages in myogenesis or disease modeling to transplantation or cellular gene therapy. Finally, application of external stimuli to cells embedded in biomaterials greatly enhances myogenic maturation. Patterning of the biomaterial via provision of defined topographical cues can drive cell differentiation and further enables control over cell/myofiber arrangement. As engineered muscles are required to create sufficiently large contractile forces upon transplantation, the importance of dynamic culture systems using such stimulation strategies has been unambiguously shown. GF, growth factors; ESCs, embryonic stem cells; iPSCs, induced pluripotent stem cells; MSCs, mesenchymal stem cells; SM interstitial cells, skeletal muscle interstitial cells.
Natural biomaterials are biocompatible and biodegradable, and thus constitute favorable biomaterials for SMTE. They possess tunable mechanical and structural properties such as porosity, topographical cues, and the option of functionalization with growth factors and/or cell adhesion motives. Furthermore, natural hydrogel materials can be molded into different shapes, which is advantageous for repairing volumetric muscle defects that usually have irregular shapes. However, natural biomaterials harbor potential immunogenicity and sometimes lack of mechanical strength (ASM International, 2003; Qazi et al., 2015). The most commonly used natural biomaterials in SMTE are collagen (Vandenburgh et al., 1988; Shansky et al., 1997; Okano and Matsuda, 1998a,b; Powell et al., 2002; Cheema et al., 2003; Kroehne et al., 2008; Bian and Bursac, 2009; Rhim et al., 2010; Hinds et al., 2011; Ma et al., 2011; Smith et al., 2012), fibrin (Huang et al., 2004; Beier et al., 2006; Borschel et al., 2006; Matsumoto et al., 2007; Bian and Bursac, 2009, 2012; Lam et al., 2009; Hinds et al., 2011; Page et al., 2011; Liu et al., 2012, 2013; Heher et al., 2015), alginate (Shapiro and Cohen, 1997; Hill et al., 2006a,b; Borselli et al., 2010, 2011; Liu et al., 2012; Wang et al., 2014), Matrigel® (Grefte et al., 2012; Juhas and Bursac, 2014), hyaluronic acid (HA) (Wang et al., 2009; Rossi et al., 2011; Monge et al., 2012), gelatin (Hosseini et al., 2012; Yang et al., 2014), silk fibroin (Mandal and Kundu, 2009), chitosan (Jana et al., 2013), and decellularized tissues (Borschel et al., 2004; Conconi et al., 2005; De Coppi et al., 2006; Mase et al., 2010; Merritt et al., 2010; Machingal et al., 2011; Perniconi et al., 2011; DeQuach et al., 2012; Wolf et al., 2012; Corona et al., 2014; Sicari et al., 2014). Common synthetic biomaterials are manufactured from biodegradable polyesters of polyglycolic acid, polyethylene glycol (PEG), polycaprolactone, poly(lactic-co-glycolic acid), and poly-l-lactic acid (Huang et al., 2006a; Choi et al., 2008; Jun et al., 2009; Aviss et al., 2010; Kim et al., 2010a,b; Ku et al., 2012; Chen et al., 2013; Yang et al., 2014). These synthetic biomaterials are versatile in use as they are degradable (over weeks to years, depending on the formulation and degree of cross-linking), allow for precise control over their physicochemical properties (e.g., degradation rate, stiffness/elasticity or the presence of topographical or biochemical cues) and usually are considerably cheaper than natural biomaterials. Additionally, they can be used in the form of hydrogels (Grizzi et al., 1995). However, they do not always support cell attachment and adhesion, can potentially cause inflammatory responses (after degradation or through prolonged persistence at the injury site in vivo) and lack biomimicry of the native ECM (Kim et al., 2010b). Therefore, they are often combined or coated with natural biomaterials to present biological recognition cues e.g. integrin-binding motives like Arg-Gly-Asp to increase cell attachment (Qazi et al., 2015). An overview of commonly used biomaterials for SMTE and their advantages and disadvantages is given in Table 1.
Hydrogels are particularly popular in SMTE due to their tunability regarding structure, shape and mechanical stability as well as their amenability to incorporate contact guidance and biochemical cues. Additionally, hydrogels can be functionalized with growth factors or other bioactive molecules to enhance regeneration (Hill et al., 2006a; Ostrovidov et al., 2014; Qazi et al., 2015). 3D hydrogels promote a spatially uniform cell distribution after encapsulation, enabling the generation of dense tissue constructs through high initial cell seeding densities and hydrogel compaction by the cells over time. The high amount of cell-cell contacts promotes and enhances myogenic fusion and increases myofiber length and thickness (Heher et al., 2015). Furthermore, 3D environments mimic the physiological conditions of the tissue more closely than 2D cultures. The use of hydrogel-based biomaterials is a promising strategy to introduce therapeutic myogenic precursor cells into a defect for subsequent formation of new muscle tissue in vivo (Han et al., 2016). Notably, hydrogels can be injected in a minimally invasive manner to support or fill void spaces after muscle trauma or disease (Qazi et al., 2015).
Collagen is the most abundant protein in the human body and the main constituent of natural ECM, which is why it has been used in a multitude of TE applications (Lee et al., 2001). However, if muscle satellite cells (MuSCs) are used, laminin has to be added to match the specific integrin complex formed by α7 and β1 isoforms (Blanco-Bose et al., 2001). In a pioneering study, Vandenburgh et al used collagen gels to incorporate and differentiate avian myoblasts into contractile myotubes with structural characteristics similar to neonatal myofibers (Vandenburgh et al., 1988). Since then, many other groups have used myogenic precursor cells combined with collagen hydrogels (Cheema et al., 2003; Rhim et al., 2007; Ma et al., 2011; Smith et al., 2012). Okano et al. highlighted that C2C12 myoblasts combined with 3D collagen gels led to differentiation into multinucleated aligned myotubes, successful capillary infiltration in vivo, and remodeling after implantation (Okano and Matsuda, 1998b).
Another natural biomaterial for hydrogel production is alginate, a polysaccharide found in seaweed, rendering it a feasible and cheap hydrogel source (Boontheekul et al., 2005; Andrejecsk et al., 2013). An advantage of alginate hydrogels is the possibility to modify them, for example by introducing cell adhesive ligands or adjusting stiffness and degradability (Shapiro and Cohen, 1997; Hill et al., 2006a,b; Borselli et al., 2010; Liu et al., 2012). Alginate hydrogels are used in many medical applications, including wound healing management or the delivery of bioactive molecules due to their low toxicity and good biocompatibility (Lee and Mooney, 2012).
The ECM component HA is also used for the fabrication of hydrogels by photo cross-linking via UV light treatment (Han et al., 2016) or by chemical cross-linking (Luo et al., 2000; Collinsworth et al., 2002; Zhang et al., 2016). HA enhances myoblast proliferation and differentiation. However, degradation by hyaluronidases in vivo is difficult to control, which may lead to apoptosis of the introduced cells due to loss of attachment to the material (Han et al., 2016).
Fibrin is a favored biomaterial to produce hydrogels. It is the end-product of the blood clotting cascade, formed when fibrinogen is cleaved by thrombin (Helgerson et al., 2004). As fibrin is a natural component of the human body like collagen and HA, it provides attractive features, including biocompatibility, biodegradability and non-toxicity. Encapsulating myogenic cells in fibrin hydrogels provides cues to trigger growth and differentiation into myotubes and eventually to myofibers (Juhas et al., 2015). Further advantageous features include tunability of its structural network, modifiable polymerization (Han et al., 2016) and the potential for incorporating growth factors (Ahmed et al., 2008). Some studies claim fibrin to be superior to other biomaterials (e.g., collagen I) due to the strong integrin binding (integrin α7 and α5) of myotubes to fibrin (Morishima-Kawashima et al., 1995; Papers and Mayer, 2003). This effect is more pronounced in fibrin, as myotubes do not have the collagen I specific integrin α2 receptor. Therefore, a fibrin environment is more conducive to distributing contractile forces from myocytes (Juhas et al., 2015). The major drawback of fibrin is finding an appropriate material density that balances the required material integrity to mimic natural stiffness and sufficient porosity for nutrient transport and cell migration (Helgerson et al., 2004; Brown and Barker, 2014). Fibrin hydrogels have been used successfully in numerous SMTE approaches using different stimuli to enhance differentiation (Huang et al., 2004; Borschel et al., 2006; Matsumoto et al., 2007; Lam et al., 2009; Liu et al., 2013; Heher et al., 2015).
Injectable hydrogels derived from decellularized muscle ECM may offer a more flexible approach than whole decellularized muscles (DeQuach et al., 2012). Although muscle ECM breakdown and subsequent processing into hydrogels destroys all existing architectural cues of the ECM (such as the vascular bed), the hydrogel formulation's composition in terms of proteins, growth factors and cytokines is preserved and can still instruct endogenous regenerative processes. Importantly, hydrogels can be produced from xenogeneic ECM sources, such as porcine dermis, submucosa or urinary bladder (Wolf et al., 2012; Badylak et al., 2016), circumventing the need for autologous muscle ECM which would be inapplicable in clinics due to donor site morbidity.
Synthetic hydrogels based on PEG are cytocompatible and offer tremendous variability for chemical manipulation. For SMTE applications, PEG can be tailored to mimic the natural stiffness of skeletal muscle tissue (Juhas et al., 2015) and seems to be a promising biomaterial for myogenic differentiation (Han et al., 2016). Laminin-coated PEG hydrogels, as an example of combined synthetic and natural materials, favored MuSC proliferation and differentiation in vitro (Han et al., 2016). PEG combined with fibrinogen constitutes a promising scaffold to embed skeletal muscle-derived pericytes (Fuoco et al., 2014) or mesoangioblasts (Fuoco et al., 2012, 2015) and favors differentiation of cells and regeneration of muscle tissue. Moreover, PEG-based hydrogels can be functionalized with different growth factors to directly promote muscle regeneration in situ, recruit endogenous stem cells to the site of injury, or enhance differentiation of muscle progenitor cells on/in the gel (Hammers et al., 2012; Rybalko et al., 2015).
Another essential factor influencing the myogenic outcome is choosing appropriate cells when generating functional muscle tissue constructs. The pool of cell types scientists can choose from has grown enormously in recent years and a variety of cell populations that are able to differentiate along the myogenic lineage have been identified (Fishman et al., 2013). In addition, new techniques, such as the generation of patient-specific induced pluripotent stem cells (iPSCs) or gene editing via the CRISPR/Cas9 technology have opened new therapeutic possibilities, especially for the treatment of MDs.
The two main groups of cells potentially being used for SMTE are either freshly isolated and expanded primary cells or immortalized cell lines. The main application of immortalized cells is the establishment of model systems, whereas primary cells are used in clinical applications and for implant studies. Myoblasts, satellite cells and stem cells from various sources are employed in different therapeutic approaches to improve muscle regeneration and function (Bach et al., 2004). The most prominent type of primary myogenic cells are MuSCs, which demonstrate a high proliferative capacity, have the ability to self-renew and differentiate into myotubes (Zammit et al., 2004; Qazi et al., 2015). Autologous MuSCs cultured with homologous acellular muscular matrices enhances their engraftment, and subsequently those matrices can be used as transplants to compensate for tissue loss (Marzaro et al., 2002). A drawback is that they have poor survival and engraftment rates after injection into damaged tissue (Mouly et al., 2005). MuSCs can be isolated either via enzymatic digestion of muscle tissue or via cellular outgrowth by plating single muscle fibers onto protein-coated dishes, which serve as a niche for satellite cells (Zammit et al., 2006; Juhas et al., 2015). However, a drawback of satellite cells is that once activated and differentiated into myotubes they cannot be brought back to a self-renewing state. Thus, the pool of cells able to proliferate and build new myotubes is eventually exhausted (Shadrin et al., 2016).
Over the years, other tissue resident cells have been discovered, namely interstitial skeletal muscle progenitor cells, which constitute a heterogeneous cell pool and seem to derive from the interstitium near the blood vessels (Shadrin et al., 2016). They offer a great regenerative potential and have already been used in studies of rodent and human SMTE. Pw1 interstitial cells, a fraction of interstitial skeletal muscle progenitor cells (Relaix et al., 1996), originate upstream of MuSCs in the muscle precursor lineage and can induce the formation of MuSCs. Therefore, their presence is a key factor in the satellite cell niche (Malecova and Puri, 2012). In the murine model, Pw1 interstitial cells enhanced muscle regeneration by releasing paracrine growth factors. Other subsets of skeletal muscle interstitial cells that play important roles in inducing muscle differentiation are fibroadipogenic progenitors, pericytes, and mesoangioblasts. All three cell populations are promising for SMTE approaches, since they are capable of ameliorating myogenic regeneration, offer high proliferative rates, and can be genetically modified (Minasi et al., 2002; Dellavalle et al., 2007; Tonlorenzi et al., 2007; Crisan et al., 2008; Joe et al., 2010; Birbrair et al., 2013; Ostrovidov et al., 2015). These characteristics also increase their relevance for potential MD treatments (Sampaolesi et al., 2003, 2006; Tedesco and Cossu, 2012; Meregalli et al., 2013). Furthermore, these cells are suitable for regenerative medicine approaches due to their good survival rates and their ability to fuse to preexisting myofibers, thereby promoting muscle regeneration in vivo (De Angelis et al., 1999; Minasi et al., 2002; Dellavalle et al., 2007; Tonlorenzi et al., 2007; Joe et al., 2010; Tedesco and Cossu, 2012).
Another cell type with great potential for regenerative medicine is mesenchymal stem cells (MSCs). MSCs are multipotent cells capable of migrating to the site of injury to promote tissue repair (Ferrari et al., 1998; Dezawa et al., 2005) and reducing inflammation (Sassoli et al., 2012). MSCs are able to differentiate into the myogenic lineage (Dezawa et al., 2005). Furthermore, they enhance muscle fiber formation and regeneration in vivo (Ferrari et al., 1998; De Bari et al., 2003; Koponen et al., 2007; Lee et al., 2011; Sassoli et al., 2012). This might be due to their support of functional satellite cells when implanted in murine muscle tissue (De Bari et al., 2003) and through recovery of expressed mechano growth factor, which is crucial for skeletal muscle maintenance and repair (Goldspink, 1999). This positive effect on muscle regeneration has been validated in in vivo disease models, where autologous MSCs were transplanted into crush trauma injuries in rats (von Roth et al., 2012; Qazi et al., 2015). MSCs' therapeutic effects may also stem from their ability to secrete soluble paracrine factors (Gnecchi et al., 2008; Sassoli et al., 2012) including Interleukin (IL)-6, IL-10, stromal cell-derived factor (Gnecchi et al., 2005; Kortesidis et al., 2005; Zhang et al., 2007; Yin et al., 2011), vascular endothelial growth factor, fibroblast growth factor (FGF), IL-1, matrix metalloproteinases (MMPs), platelet derived growth factor, transforming growth factor ß, angiopoyetin (Kinnaird et al., 2004), hepatocyte growth factor, and adrenomedullin (Ohnishi et al., 2007; Wynn, 2008; Tang et al., 2010). Via secretion of these factors, MSCs assert substantial anti-inflammatory effects by modulating the immune response (Le Blanc and Mougiakakos, 2012). However, a study by Ferrari et al reported that bone-marrow transplantation did not ameliorate the dystrophic phenotype in mdx mice, a widely used mouse model for DMD (Ferrari et al., 2001). One suggested reason for the low regenerative potential of MSCs in this setting was that a vast number of cell types is present in the bone-marrow, which resulted in relatively low numbers of MSCs actually being transplanted in the course of a bone-marrow transplantation (reviewed by Forcales, 2015). Alternative cells used for SMTE are L6 rat myoblasts, neonatal muscle-derived progenitor cells and xenogeneic cells derived from adult muscles from other species (van der Schaft et al., 2013).
Human or murine embryonic stem cells represent another regularly used source for obtaining skeletal myoblasts. It is possible to obtain CD73+ multipotent mesenchymal precursors, which can be differentiated into myoblasts by co-culturing them with C2C12 cells (Barberi et al., 2005). Since their generation by Yamanaka and Takahashi (2006), iPSCs have been widely implemented in different research areas. This technique makes it possible to reprogram cells directly from patients for autologous cell therapy of MDs (Meregalli et al., 2014; Quattrocelli et al., 2015). Since iPSCs can be derived from healthy or diseased patients, they offer great potential in TE for disease modeling and drug testing (Wobma and Vunjak-Novakovic, 2016). Such autologous patient-derived cells are non-immunogenic and, in addition, genetic defects can be corrected during ex vivo culture using tools such as CRISPR/Cas9. Interestingly, iPSCs generated from mesoangioblasts were shown to fuse to existing muscle with higher efficiency than iPSCs generated from fibroblasts (Quattrocelli et al., 2015). An important proof-of-concept study was performed by Tedesco et al., who used genetically corrected iPSCs derived from myoblasts or fibroblasts of limb-girdle MD patients, differentiated them into mesoangioblasts and grafted them into affected muscles in a humanized limb-girdle MD mouse model (Tedesco et al., 2012). This not only ameliorated the dystrophic phenotype and restored the depleted satellite cell pool, but importantly also demonstrated that treatment with patient-specific iPSC-derived cells can be utilized for stem cell therapy in MDs. However, it has to be noted that there are still limitations regarding both the use of embryonic stem cells, which raise ethical concerns (An and Li, 2014), and iPSCs, which entail the risk of genetic recombination and tumor formation. To date, iPSC-based regenerative stem cell therapies have not entered clinics due to these safety considerations (Lee et al., 2009; Cittadella Vigodarzere and Mantero, 2014).
Finally, one of the most widely used cell line in SMTE are C2C12 murine myoblasts, established in 1977 from MuSCs derived from a C3H mouse (Yaffe and Saxel, 1977). Many researchers start their initial experiments with these cells, as they are easy to cultivate, proliferate rapidly, and differentiate well upon serum deprivation. Thus, they represent an ideal tool to evaluate new biomaterials or bioreactor systems for the generation of skeletal muscle tissue. However, due to the immortalization of the cells, translation into clinical use is not feasible. Human cell lines, however, may still serve as attractive cells for in vitro studies. A recent transcriptomics analysis revealed that immortalization of C25 human myoblasts neither interferes with their myogenic potential, nor with any other aspect of cell physiology—apart from the elicited protection against senescence (Thorley et al., 2016). Biomimetic in vitro skeletal muscle disease models employing patient-derived human myoblast lines may therefore provide a higher predictive capability than rodent in vivo models.
Besides choosing the appropriate biomaterial and cell type, another key element that needs to be addressed is suitable stimulation strategies (either mechanical-, electrical-, or electromechanical-stimulation), which are indispensable for enhanced muscle maturation in vitro. Cells are highly responsive to their microenvironment such as the surrounding ECM, mechanical forces, and biochemical signals. Furthermore, the mechanical properties of biomaterials, such as the material stiffness or the presence of distinct microarchitectural features, can influence cellular behavior tremendously (Engler et al., 2006, 2007; Cittadella Vigodarzere and Mantero, 2014). The stiffness/elasticity of a material is usually assessed by measuring the Young's modulus (elastic modulus) which is determined by a material's composition and capability for deformation.
One strategy to mimic the natural environment is the application of biochemical and/or biophysical stimulation to engineered constructs. Exercise can be simulated by the application of mechanical stimuli, such as cyclic and/or static strain. Exercise leads to the activation of satellite cells and subsequent fusion to already existing myofibers in vitro (Tatsumi et al., 2001) through triggering the release of hepatocyte growth factor and nitric oxide (NO) radicals, which in turn activate the satellite cells. NO is produced by nitric oxide synthases which are up-regulated by exercised or injured muscle tissue in vitro and in vivo (Tatsumi, 2010).
Regarding myogenesis, passive (e.g., bone elongation during development) as well as active (e.g., exercising during sport) mechanical stretching is essential for the development of skeletal muscle from embryonic to adult tissue (Goldspink et al., 1992; Heher et al., 2015). An appropriate stimulation protocol can exert a positive effect on gene regulation, protein expression and thus proliferation and differentiation of cells (Goldspink et al., 1992; Powell et al., 2002; Goldspink, 2003). Furthermore, exercise training improves fusion and alignment of myofibers (Vandenburgh and Karlisch, 1989; Corona et al., 2012; Heher et al., 2015), and enhances the generation of mature myofibers (Goldspink, 2003). Morphologically, mature skeletal muscle tissue is characterized by widespread sarcomeric patterning, which is indispensable for contraction. Moreover, mechanical stimulation causes an increase in the cross-striations of the tissue and a switch of myosin heavy chain isoforms from embryonic to adult (Juhas et al., 2015).
One of the first studies implementing mechanical stimulation was conducted by Goldberg et al. in which hypertrophy was induced by overloading of synergistic muscle within just 24 h (Goldberg, 1967; Armstrong et al., 1979). Further sophisticated mechanical stimulation protocols were conducted using bioreactors with mechanical stimuli to create dynamic 2D or 3D culture systems. These studies are listed in more detail in Table 2 (Vandenburgh and Karlisch, 1989; Okano and Matsuda, 1998b; Powell et al., 2002; Auluck et al., 2005; Cheema et al., 2005; Matsumoto et al., 2007; Liao et al., 2008; Moon et al., 2008; Candiani et al., 2010; Machingal et al., 2011; Corona et al., 2012; Smith et al., 2012; Heher et al., 2015; Qazi et al., 2015).
Muscle tissue can also be stimulated with electrical stimulation, which positively affects myogenic gene regulation as well as protein expression (Goldspink et al., 1992; Powell et al., 2002; Goldspink, 2003). Motor neurons are responsible for innervating muscle fibers and the signal inducing contraction of the muscle tissue is distributed via branched axons (Purves et al., 2001). Electrical stimulation aims to recapitulate the processes of innervation by fast and slow motor neurons, which are responsible for the switch of muscle fiber types (Wehrle et al., 1994; Khodabukus et al., 2015). Electrical stimulation of mouse myoblasts improves myogenic differentiation (Park et al., 2008) and enhances their contractile properties compared to unstimulated controls (Salmons et al., 2005; Fujita et al., 2007). In monolayer myogenic cultures, twitches happen spontaneously after the formation of myotubes, but electrical stimulation is needed for a controlled and sustained contraction. Chronic periods of electrical stimulation are relevant for the formation of mature phenotypes in muscle tissue constructs as well as to improve their contractile properties (Kasper et al., 2017). Many groups have applied sophisticated electrical stimulation protocols to muscle cells in vitro (Stern-Straeter et al., 2005; Huang et al., 2006b; Fujita et al., 2007; Donnelly et al., 2010; Langelaan et al., 2011; Khodabukus and Baar, 2012) (Table 3).
Table 3. Summary of bioreactor systems with corresponding electrical and electro-mechanical stimulation protocols.
To the best of our knowledge, so far there is only one published study combining both electro-and mechanical stimulation for engineering mature muscle constructs (Liao et al., 2008) (Table 3). In literature, there is only one bioreactor system reported, which combines the application of electrical and mechanical stimulation of 3D constructs. It is a commercially available system from EBERS Medical Technology, Spain, and allows for media perfusion under sterile conditions (Kasper et al., 2017). An overview of bioreactor systems used in SMTE with their used electrical stimulation protocols and the observed outcome is given in Table 3.
Myokines are another factor influencing muscle as well as other tissues and therefore might offer an interesting therapeutic option to treat patients in future. They are released by muscle tissue in response to exercise training. It is known that regular exercise has beneficial effects on overall health status. Accumulating epidemiologic evidence suggests that physical activity plays an independent role in preventing frequent chronic diseases like osteoporosis, diabetes, Alzheimer's, osteoarthritis or degenerative muscle conditions, and that the beneficial effects of exercise training are partially due to secreted myokines (Dunstan, 2011; Pedersen, 2011; Egan and Zierath, 2013).
Skeletal muscle has been recognized as an endocrine organ due to its ability to produce, store and secrete hormones and myokines. In particular, myokines are able to affect and regulate inflammatory and metabolic processes in muscle and in many other tissues in an endocrine or paracrine manner (Pedersen and Febbraio, 2008; Pedersen, 2009; Girgis et al., 2014; Tagliaferri et al., 2015). To date, there are 69 putative myokines, which are released via exercise training (Catoire et al., 2014).
So far, the most prominently investigated myokines are IL-6 (Pedersen, 2009), IL-7 (Haugen et al., 2010), IL-8 (Nielsen and Pedersen, 2007), IL-15 (Quinn et al., 2008), leukemia inhibitory factor (Broholm et al., 2011), FGF-21 (Izumiya et al., 2008; Hojman et al., 2009), insulin-like 6 (Zeng et al., 2010), follistatin-like 1 (Ouchi et al., 2008), musculin (Nishizawa et al., 2004), irisin (Boström et al., 2012), myonectin (Seldin et al., 2012), secreted protein acidic rich in cysteine (SPARC) (Songsorn et al., 2016), and Meteorin-like 1 (Rao et al., 2014).
IL-6 is a pleiotropic myokine and acts on muscle tissue by influencing satellite cell activation and differentiation, which is usually triggered by stress due to injury or mechanical stimulation (Muñoz-Cánoves et al., 2013). It is the first myokine to be released after acute exercise (Agarwal, 2017). Besides primarily acting on muscle, other organs such as adipose tissue, the liver and the brain are responsive to secreted IL-6 (Pedersen et al., 2001). Secondly, it negatively regulates pro-inflammatory cytokines (Pedersen and Febbraio, 2008; Benatti and Pedersen, 2014) such as tumor necrosis factor alpha and elevates levels of anti-inflammatory cytokines e.g., IL-10 and IL-1 receptor antagonist released from leukocytes (Pedersen et al., 2001). On the other hand, IL-6 is also considered a pro-inflammatory cytokine. Therefore, further investigations are needed to identify the exact role of IL-6 in muscle and other influenced tissues (Abeywardena et al., 2009).
Leukemia inhibitory factor belongs to the IL-6 superfamily (Rose-John et al., 2006) and is secreted by hypertrophic muscle (Spangenburg and Booth, 2006; Serrano et al., 2008; Guerci et al., 2012). It is also released upon resistance training in human muscle and in electrically stimulated cultured human myoblasts (Broholm et al., 2011). Studies in rodents demonstrated that production of IL-6 and leukemia inhibitory factor help to regenerate muscle tissue after injury by activating satellite cells (Barnard et al., 1994; Kurek et al., 1996, 1997; Zhang et al., 2013).
Irisin is a hormone-like myokine secreted during exercise (Boström et al., 2012). It plays an important role in bone-muscle cross talk, and supposedly influences both tissues (Colaianni et al., 2017). This might explain why diseases like osteoporosis and sarcopenia are linked to each other (Reginster et al., 2015). Studies suggest that myokines like IL-6, IL-8, and IL-15 indirectly influence bone via acting on other tissues, while irisin affects bone tissue directly by increasing the differentiation of osteoblasts in vitro as well as enhancing cortical bone mass in vivo (Colaianni et al., 2014, 2015, 2017; Colaianni and Grano, 2015). Irisin also reduces body weight when administered to obese patients (Colaianni et al., 2017). Another study showed that irisin uptake reduces body weight due to increased adipocyte and glucose metabolism and even elevated oxygen intake levels in an animal model (Boström et al., 2012). Irisin has the potential to transform white adipose tissue into brown adipose tissue, which is metabolically very active. This is supposed to ameliorate obesity and is called browning (Bartelt and Heeren, 2013). Furthermore, Colaianni et al conducted a study in which they analyzed conditioned media from muscle cells of mice performing exercise training. They found that Irisin levels in the media caused a stronger differentiation of bone marrow stromal cells into osteoblasts (Colaianni et al., 2014).
Another myokine, Meteorin-like 1, also induces adipose tissue browning (Rao et al., 2014). The myokines IL-8 and Fstl-1 both induce angiogenesis (Nielsen and Pedersen, 2007), the latter by inducing endothelial cell-mediated neovascularization in ischemic tissue (Ouchi et al., 2008).
IL-15 as well as IL-15 receptor alpha are involved in anabolic/catabolic regulation of skeletal muscle tissue (Quinn et al., 1995; Quinn, 2002; Furmanczyk and Quinn, 2003; Riechman et al., 2004; Busquets et al., 2005; Pistilli et al., 2007). IL-15 enhances the expression of myosin heavy chain in differentiating myocytes (Quinn et al., 1995) and myotubes (Quinn, 2002; Furmanczyk and Quinn, 2003). One session of resistance exercise is sufficient to increase IL-15 levels in trained and untrained humans (Riechman et al., 2004). Furthermore, Quinn et al found high levels of IL-15 to reduce fat mass and therefore lower adiposity in mice (Quinn et al., 2008).
In 2012, Seldin et al. identified another myokine called myonectin. Higher levels of myonectin were secreted into the media by differentiated C2C12 compared to non-differentiated cells. Furthermore, exercise elevated the expression of myonectin in muscle and it is putatively involved in the cross talk between muscle and other tissues like liver and adipose tissue (Seldin et al., 2012).
Another exercise-induced myokine, musculin, is activated by calcium signaling via the AKT pathway (Subbotina et al., 2015). Its function is to enhance mitochondrial biogenesis, which improves physical perseverance (Nishizawa et al., 2004; Subbotina et al., 2015).
SPARC is a myokine found in humans and mice and secreted during muscle contraction (Songsorn et al., 2016). Catoire et al. first identified SPARC to be released upon exercise training through secretome analysis (Catoire et al., 2014). SPARC affects many crucial mechanisms in the cell such as regulation of cell shape, differentiation and adhesion (Murphy-Ullrich and Sage, 2014). SPARC additionally affects insulin secretion in humans (Harries et al., 2013) and erythropoiesis in mice (Luo et al., 2012). Interestingly, the duration of the exercise seems to be more important than the intensity of the exercise for its secretion (Songsorn et al., 2016).
To this day there are no effective cures for muscular dystrophies, hence there is an urgent need for models mimicking them. Novel biomimetic disease modeling platforms could offer a way to understand and study underlying mechanisms of such diseases and furthermore to test potential treatment options. Muscular dystrophy disease models have been used to study the underlying mechanisms, course of the disease over time, as well as therapeutic agents. Some of these will be discussed in the next sections. Animal models are the cornerstone of research on elucidating the mechanisms underlying dystrophies and on developing new treatment strategies. To date, there are around 50 in vivo animal models for studying muscular dystrophies in various species ranging from invertebrates (e.g., Caenorhabditis elegans), non-mammalian vertebrates, especially zebrafish (Guyon et al., 2007), to mammals (e.g., mice, rats, dogs, and pigs) (McGreevy et al., 2015). The most commonly utilized mammalian DMD models are the mdx mouse model, the mdx utrophin double mutant mouse model (mdx:utrn−/−) and the canine x-linked MD model (cxmd) (Banks and Chamberlain, 2008). A frequently used model system is the mdx mouse that carries a mutation in the dystrophin gene, resulting in a DMD phenotype. However, there are significant differences in the course of the disease in human DMD patients and mdx mice regarding characteristics, such as lifespan, severity, timeline, body weight, impact on other physiological functions and many more (Partridge, 2010). Canine DMD models offer a way to overcome these obstacles, as they present fewer differences to the human DMD pathology regarding the aforementioned characteristics. Furthermore, dystrophic dogs are more suitable for studies using gene therapy approaches than mice, since the former presents a closer simulation of the human immune response toward introduction of vectors for gene repair and replacement (McGreevy et al., 2015). Thus, development of new strategies to introduce vectors evading the immune system is facilitated (Duan, 2015).
Despite the immense amount of knowledge gained on the pathophysiology of skeletal muscle diseases, animal models entail certain disadvantages and ethical considerations. They cannot recapitulate the exact manifestation of the disease in regard of physiological, biochemical and clinical conditions as they appear in the human body. This has prompted research trying to find appropriate time and cost effective alternatives (Banks and Chamberlain, 2008; Benam et al., 2015). Furthermore, results gained from in vivo drug testing setups frequently fail when translated to the clinics, due to major differences in underlying molecular mechanisms between different species. In vitro human disease models are a potential way to overcome these limitations. They can more closely mimic human pathological conditions concerning tissue and organ specific cell types (Benam et al., 2015) through the possibility of using patient-derived cells, which reflects the patient's individual skeletal muscle physiology and the disease progression in the dystrophic state (Smith et al., 2016). Moreover, it is possible to change single parameters within these systems and study the resulting effects, which constitutes another advantage of in vitro disease modeling (Bersini et al., 2016).
In general, miniaturizing disease models has gained attraction in the past years, as it allows reduction in cell number and reagents (and, thus, overall costs), while maintaining the quality of results. Additionally, the assessment of results and provision of external stimuli can be carried out in a precise and controlled manner. Thus, research on microfluidic devices for disease modeling has emerged in recent years (Bersini et al., 2016). One microfluidic system by Ferreira et al used a different approach in modeling dystrophy. Instead of using cells with a diseased phenotype, they established a device that mimicked the cellular environment of dystrophies. This was done by using different ECM compositions and applying a concentration gradient of basic FGF (bFGF), which is known to be released upon muscle injury. Thereby, it was possible to assess the influence of bFGF and different substrates on myoblast recruitment in normal or DMD simulating environments (Ferreira et al., 2015).
Another miniaturized 2D in vitro disease model was published by Serena et al, who created myotubes derived from primary myoblasts from healthy donors as well as patients suffering from DMD cultured on polyacrylamide hydrogels. This was achieved through adequate substrate design, including appropriate mechanical properties (i.e., a Young's modulus of 15 kPa). Furthermore, micro-patterning the substrate in parallel lanes to enhance myotube alignment and coatings with the adhesion molecules laminin, fibronectin and Matrigel® were utilized as well. Thereby, it was possible to generate myotubes positive for myosin heavy chain II and α-actinin that developed a highly ordered sarcomeric patterning. Furthermore, myotubes generated from healthy donors exhibited dystrophin expression. This is a key aspect for assessing the functionality of DMD therapies, as they often aim at restoring dystrophin expression (Serena et al., 2010). The basic principle of this test system was recently used to study the potential of mesoangioblasts in DMD treatment as they ameliorated dystrophin distribution in DMD myoblasts (Serena et al., 2016).
The course of dystrophies varies widely from one patient to another, as the mutations causing the disease are very heterogeneous, ranging from severe forms completely lacking dystrophin to a partially functioning truncated form of the protein. This variance cannot be considered in animal or standard in vitro models. Creating iPSCs from patient-derived cells offers a solution to this problem, since it allows direct comparison of the pathological phenotype of the patient and the cultured cells. This makes drug screening results and the evaluation of specific genetic aberrations more reliable. Therefore, they present a promising tool for modeling a variety of diseases. Also, recent advances in the field of iPSC research have boosted the efficiency of reprogramming. Myogenic progenitor-derived iPSCs showed good engraftment after transplantation, were able to regenerate myofibers and could repopulate the stem cell niche (Darabi et al., 2012; Meregalli et al., 2014; Kodaka et al., 2017). Nevertheless, this approach also bears certain disadvantages such as the lengthy processes involved in generating iPSCs and inducing differentiation into iPSC-derived myogenic progenitors, or the need to integrate so-called reprogramming factors, which could have unknown implications on the phenotype of the disease (Smith et al., 2016). Tanaka et al. were able to create myotubes from human iPSCs derived from Miyoshi Myopathy patients through inducible MyoD1 expression. These myotubes exhibited hallmarks of the disease, such as the role of Dysferlin during this disease. A lack of Dysferlin expression led to inefficient membrane repair, which could be overcome by an induced overexpression of Dysferlin, rescuing dystrophic myotubes and leading to a healthy phenotype. These results suggest that this model has the potential to shed light on the pathology of the disease, and may be applicable to other types of dystrophies (Tanaka et al., 2013). Another study using human iPSCs from DMD and Becker MD patients was published by Abujarour et al. Human iPSCs were subjected to MyoD1 overexpression, inducing myogenic commitment and finally yielding myotubes. To investigate whether this model has the potential to be used for drug testing, dystrophic myotubes were subjected to IGF-1 and Wnt7a treatment, factors that elicit skeletal muscle hypertrophy. A treatment with these two factors resulted in significant increase in fiber diameter, suggesting usability of this model for drug testing (Abujarour et al., 2014). Nevertheless, to date these models have not been used to test drugs or other therapies for DMD.
However, it is not possible to accurately mimic the complex organization of tissues in vivo using 2D disease models. Thus, drug-screening results gained from these systems cannot directly be translated for the use in clinical studies. To overcome the limitations of 2D cell-based systems, more recent research has focused on the development of 3D systems that more adequately reflect the in vivo situation (Nam et al., 2015), where cells can interact with the matrix they are embedded in and form 3D structures (Bersini et al., 2016). A 3D drug testing platform was established by Madden and colleagues using human primary myoblasts grown in so-called myobundles generated by incorporation in fibrinogen and Matrigel® frames using polydimethylsiloxane molds. The bundles differentiated into chemically and electrically responsive muscle-like constructs capable of contraction. To prove their suitability for drug screening, the myobundles were treated with three different drugs, namely statins that induce muscle weakness, chloroquine that induces autophagy and clenbuterol which increases hypertrophy in low doses but leads to apoptosis and necrosis at higher concentrations. Overall, treatment with these compounds resulted in the expected outcomes. Therefore, this model appears suitable for drug testing. However, its usability as a disease model to study the pathophysiology of dystrophies remains to be established, as it has only been examined with cells derived from healthy donors (Madden et al., 2015). Thus, there is only one actual in vitro skeletal muscle disease model reported in 3D so far. This model used dystrophic myoblasts from mdx mice that were incorporated in natural hydrogels (collagen type I or fibrin) that were cast around posts. The resultant myotubes were electrically stimulated and contractile force generation was measured. In addition, 31 compounds that have the potential to serve as DMD drugs were screened by measuring changes in force generation upon treatment. Since this system works semi-automatically and in a 96-well culture format it is considered a potential high-throughput system for testing novel drugs for MD treatment. The major drawback of this model, however, is that it used murine cells. Thus, the results do not account for possible differences in drug response between humans and mice. Furthermore, the phenotype of the engineered constructs appeared to be closer to neonatal than adult according to the myosin heavy chain profiles (Vandenburgh et al., 2008).
In summary, there is still a great need for further research in the field of 3D skeletal muscle disease modeling. The creation of mature and functional in vitro muscle constructs could help enhance our fundamental understanding of the skeletal muscle physiology. Hence, the next step would be to create appropriate and translatable disease model systems to bring in vitro research one step closer to the in homine situation.
When it comes to SMTE approaches, the fact that 2D culture systems behave fundamentally different from 3D systems has often been overlooked. Hence, results from 2D experiments may not be directly compared or even translated to 3D settings. Identifying applicable treatment options will require engineering of functional 3D muscle tissue constructs. In this respect, several questions need to be addressed: (I) When does one look at gene expression levels or signaling pathways involved in muscle development or differentiation? (II) What are representative time points for the evidence of mature and functional muscle tissue? (III) Which analytical tools and methods can be applied for the morphological and functional assessment of skeletal muscle tissue constructs? (IV) How can a given biomaterial recapitulate the physiological environment supporting the myogenic potential of the cells?
Therefore, there is urgent need for standardized dynamic 3D model systems to enable comparability of results. Additionally, careful deliberation of the choice of biomaterial, cell type and the external stimuli, prior to the start of the actual experimental SMTE approach, may help to improve the outcome and save valuable time (Figure 2). The field of SMTE would greatly benefit from a workflow of criteria, factors, and analytical methods, which could be utilized by researchers globally. Here we provide a putative example of such a workflow that displays different experimental and developmental stages in in vitro SMTE culture systems, and produces results that are translatable to in vivo settings (Figure 3). It suggests analytical tools for endpoint analysis and evaluation of requirements for achieving SMTE constructs with desirable properties (e.g., determination of elastic modulus, activation of involved signaling pathways and expression of myogenic markers and functional characteristics). Optimization of dynamic culture conditions comprises a thorough cell biological analysis including investigation of signaling pathways involved in myogenesis, muscle hypertrophy and proliferation, myogenic gene expression profiling, morphological analysis through immunofluorescence staining for contractile proteins, calculation of the fusion index and the quantification of sarcomeric striations—the latter indicating a certain degree of muscle maturity. Finally, environmental culture conditions should be fine-tuned, e.g., applying external stimuli including appropriate training. Such stimuli are commonly applied via bioreactor systems, which contribute to the desired outcome of engineering 3D skeletal muscle constructs by recapitulating physiological or pathophysiological muscle states. A unified SMTE approach following certain design criteria would render results between groups more comparable, possibly accelerating and streamlining new therapeutic discoveries and advancements in the field of SMTE.
Figure 3. Envisioned future of skeletal muscle tissue engineering—a suggested workflow. This schematic presents a skeletal muscle tissue engineering workflow including stage-specific experimental considerations. Initially, the compatibility of biomaterials with potent myogenic cells has to be evaluated. This first step also involves the decision whether the cells will be cultured and grown in a 2D (monolayer on a pliant matrix) or 3D (encapsulation into a pliant matrix) environment. This still represents a static cell culture, where only the first steps in the SMTE approach are addressed. Evaluation of the biophysical matrix properties, biocompatibility and effects of the biomaterial on cell proliferation/differentiation can be evaluated via this process. The second step involves dynamic culture of the evaluated biomaterial and cells, where the main consideration is which stimulation strategy will be implemented into the culture system—ranging from mechanical to electrical stimulation or a combination of both. The third step addresses the functional analysis of the engineered muscle construct via twitch force measurements. At this point, contractile muscle constructs can furthermore be tested for their response to drugs with known effects, which is a prerequisite for later application of engineered muscle tissue in drug screening studies. An ideal setup would involve co-cultures to engineer muscle tissue with built-in vascular and neuronal structures to further enhance muscle maturity and contractility. After successful in vitro evaluation, the final step is the translation into animal models to test for the contribution of the engineered muscle to myogenesis and regeneration in healthy and/or diseased muscle. Ultimately, the knowledge gained from in vivo experiments can also be transferred back to in vitro setups for the generation of disease models.
Numerous sophisticated SMTE strategies exist, ranging from basic 2D to complex dynamic 3D setups, and researchers have a plethora of biomaterials and cell types to choose from. Nevertheless, to date the clear majority of SMTE approaches have failed to achieve broad clinical utility due to several reasons: (I) Systemic elucidation of suitable cell types and biomaterials as well as stimulation protocols (to induce muscle maturation) are still ongoing. (II) The pathomechanisms of a variety of MDs are still poorly understood which limits the clinical success of cell therapeutic approaches. Hence, model systems for developmental/mechanistic and pathophysiological studies (disease models) are urgently needed to perform drug screenings for potential new treatment options. Currently, the focus is on finding reliable physiological models to further understand and study the pathophysiological processes in MDs. (III) Although acellular approaches bypass the general risks associated with (stem) cell therapy, many seemingly promising biomaterials have ultimately failed to meet the physical and native requirements to drive muscle regeneration.
ESC- and iPSC-derived myogenic precursors are increasingly used for drug screening purposes in disease models, while immortalized cell lines are used for initial testing of novel biomaterials and/or bioreactor systems. In an optimal scenario, autologous primary muscle (stem) cells directly derived from the patient would be used for personalized therapeutic approaches or disease models that involve the use of either undifferentiated or preconditioned cells. Although the current pool of applicable cells permits many different methodologies, each cell type has its limitations. However, advances in cell biology will establish adequate culture conditions in the future which will ideally diminish the phenotypic changes of cell types suitable for SMTE during ex vivo culture. Biomaterial systems that can serve as artificial satellite cell niches have already improved the efficiency of cell grafting in in vivo studies, and a more thorough evaluation of the satellite cell niche composition and microarchitecture will further improve current cell-based therapies. Finally, strategies for in vitro pre-vascularization and innervation will likely enhance the functional contribution of engineered muscle transplants to repair muscle in vivo. In addition, co-culture systems will allow studies on the interface between the different cell types in the muscle construct. Furthermore, myokines might offer novel therapeutic opportunities in the future, due to their positive effects on muscle as well as on other tissues. In in vitro culture systems, they might also be useful as supplements which can act as supportive factors for myogenesis, thereby improving the myogenic outcome of engineered muscle tissue constructs.
Therefore, elevating SMTE to the next level will require a thorough re-evaluation of biomaterial and cell sources as well as fine-tuning of stimulation techniques. Additionally, taking the above-mentioned criteria into account and implementing them into current research strategies will yield novel skeletal muscle (disease) model systems helping to improve therapeutic approaches to finally translate them into clinical setups.
CF: PI of the project, preparation of the manuscript, design of the figures; BM: preparation of the manuscript and tables; JT: contributed to cell part and disease model part of manuscript, revising the manuscript; PH: preparation of the manuscript and help in figure design, revising the manuscript; OS: PhD supervisor of BM, corrections of the manuscript and input on figures and tables; DR: finances the project, head of department of biochemical engineering, corrections of the manuscript and input on figures and tables.
This work was supported by the Signal Tissue (MA23#18-08) and the FFG COIN Disease Tissue (FFG #845443) Project.
The authors declare that the research was conducted in the absence of any commercial or financial relationships that could be construed as a potential conflict of interest.
The reviewer SG and handling Editor declared their shared affiliation.
We would like to acknowledge DI Dr. Andreas Teuschl, DI Dr. Karl H. Schneider, Carina Hromada, and Prof. Dr. Heinz Redl for their valuable input.
Abeywardena, M. Y., Leifert, W. R., Warnes, K. E., Varghese, J. N., and Head, R. J. (2009). Cardiovascular biology of interleukin-6. Curr. Pharmaceut. Des. 15, 1809–1821. doi: 10.2174/138161209788186290
Abujarour, R., Bennett, M., Valamehr, B., Lee, T. T., Robinson, M., Robbins, D., et al. (2014). Myogenic differentiation of muscular dystrophy-specific induced pluripotent stem cells for use in drug discovery. STEM CELLS Transl. Med. 3, 149–160. doi: 10.5966/sctm.2013-0095
Agarwal, M. (2017). Cardiovascular response and serum interleukin-6 level in concentric vs. eccentric exercise. J. Clin. Diagn. Res. 11, 4–8. doi: 10.7860/JCDR/2017/25281.9703
Ahmed, T. A., Dare, E. V., and Hincke, M. (2008). Fibrin: a versatile scaffold for tissue engineering applications. Tissue Engineering B Rev. 14, 199–215. doi: 10.1089/ten.teb.2007.0435
Almada, A. E., and Wagers, A. J. (2016). Molecular circuitry of stem cell fate in skeletal muscle regeneration, ageing and disease. Nat. Rev. Mol. Cell Biol. 17, 267–279. doi: 10.1038/nrm.2016.7
An, Y., and Li, D. (2014). Engineering skeletal muscle tissue in bioreactor systems. Chin. Med. J. 127, 4130–4139.
Andrejecsk, J. W., Cui, J., Chang, W. G., Devalliere, J., Pober, J. S., and Saltzman, W. M. (2013). Paracrine exchanges of molecular signals between alginate-encapsulated pericytes and freely suspended endothelial cells within a 3D protein gel. Biomaterials 34, 8899–8908. doi: 10.1016/j.biomaterials.2013.08.008
Armstrong, R. B., Marum, P., Tullson, P., and Saubert, C. W. (1979). Acute hypertrophic response of skeletal muscle to removal of synergists. J. Appl. Physiol. 46, 835–842. doi: 10.1152/jappl.1979.46.4.835
ASM International (2003). Overview of Biomaterials and Their Use in Medical Devices. ASM International.
Auluck, A., Mudera, V., Hunt, N. P., and Lewis, M. P. (2005). A three-dimensional in vitro model system to study the adaptation of craniofacial skeletal muscle following mechanostimulation. Eur. J. Oral Sci. 113, 218–224. doi: 10.1111/j.1600-0722.2005.00215.x
Aviss, K. J., Gough, J. E., and Downes, S. (2010). Aligned electrospun polymer fibres for skeletal muscle regeneration. Eur. Cells Mater. 19, 193–204. doi: 10.22203/eCM.v019a19
Bach, A. D., Beier, J. P., Stern-Staeter, J., and Horch, R. E. (2004). Skeletal muscle tissue engineering. J. Cell. Mol. Med. 8, 413–422. doi: 10.1111/j.1582-4934.2004.tb00466.x
Badylak, S. F., Dziki, J. L., Sicari, B. M., Ambrosio, F., and Boninger, M. L. (2016). Mechanisms by which acellular biologic scaffolds promote functional skeletal muscle restoration. Biomaterials 103, 128–136. doi: 10.1016/j.biomaterials.2016.06.047
Banks, G. B., and Chamberlain, J. S. (2008). “Chapter 9: The value of mammalian models for duchenne muscular dystrophy in developing therapeutic strategies,” in Current Topics in Developmental Biology, eds R. S. Krauss. Elesvier Inc. doi: 10.1016/S0070-2153(08)00609-1
Bao Ha, T., Le, M. T., Nguyen, D., and Minh, D. (2013). “Naturally derived biomaterials: preparation and application,” in Regenerative Medicine and Tissue Engineering. doi: 10.5772/55668
Barberi, T., Willis, L. M., Socci, N. D., and Studer, L. (2005). Derivation of multipotent mesenchymal precursors from human embryonic stem cells. PLoS Med. 2:e161. doi: 10.1371/journal.pmed.0020161
Barnard, W., Bower, J., Brown, M. A., Murphy, M., and Austin, L. (1994). Leukemia inhibitory factor (LIF) infusion stimulates skeletal muscle regeneration after injury: injured muscle expresses lif mRNA. J. Neurol. Sci. 123, 108–113. doi: 10.1016/0022-510X(94)90211-9
Barrilleaux, B., Phinney, D. G., Prockop, D. J., and O'Connor, K. C. (2006). Review: ex vivo engineering of living tissues with adult stem cells. Tiss. Eng. 12, 3007–3019. doi: 10.1089/ten.2006.12.3007
Bartelt, A., and Heeren, J. (2013). Adipose tissue browning and metabolic health. Nat. Rev. Endocrinol. 10, 24–36. doi: 10.1038/nrendo.2013.204
Beauchamp, J. R., Heslop, L., Yu, D. S., Tajbakhsh, S., Kelly, R. G., Wernig, A., et al. (2000). Expression of CD34 and Myf5 defines the majority of quiescent adult skeletal muscle satellite cells. J. Cell Biol. 151, 1221–1233. doi: 10.1083/jcb.151.6.1221
Beaudart, C., Rizzoli, R., Bruyère, O., Reginster, J.-Y., and Biver, E. (2014). Sarcopenia: burden and challenges for public health. Arch. Public Health 72:45. doi: 10.1186/2049-3258-72-45
Beier, J. P., Stern-Straeter, J., Foerster, V. T., Kneser, U., Stark, G. B., and Bach, A. D. (2006). Tissue engineering of injectable muscle: three-dimensional myoblast-fibrin injection in the syngeneic rat animal model. Plastic Reconstr. Surg. 118, 1113–1121. doi: 10.1097/01.prs.0000221007.97115.1d
Benam, K. H., Dauth, S., Hassell, B., Herland, A., Jain, A., Jang, K.-J., et al. (2015). Engineered in vitro Disease Models. Annu. Rev. Pathol. 10, 195–262. doi: 10.1146/annurev-pathol-012414-040418
Benatti, F. B., and Pedersen, B. K. (2014). Exercise as an anti-inflammatory therapy for rheumatic diseases—myokine regulation. Nat. Rev. Rheumatol. 11, 86–97. doi: 10.1038/nrrheum.2014.193
Bersini, S., Arrigoni, C., Lopa, S., Bongio, M., Martin, I., and Moretti, M. (2016). Engineered miniaturized models of musculoskeletal diseases. Drug Discovery Today 21, 1429–1436. doi: 10.1016/j.drudis.2016.04.015
Bian, W., and Bursac, N. (2009). Engineered skeletal muscle tissue networks with controllable architecture. Biomaterials 30, 1401–1412. doi: 10.1016/j.biomaterials.2008.11.015
Bian, W., and Bursac, N. (2012). Soluble miniagrin enhances contractile function of engineered skeletal muscle. FASEB J. 26, 955–965. doi: 10.1096/fj.11-187575
Birbrair, A., Zhang, T., Wang, Z.-M., Messi, M. L., Enikolopov, G. N., Mintz, A., et al. (2013). Role of pericytes in skeletal muscle regeneration and fat accumulation. Stem Cells Dev. 22, 2298–2314. doi: 10.1089/scd.2012.0647
Blanco-Bose, W. E., Yao, C.-C., Kramer, R. H., and Blau, H. M. (2001). Purification of mouse primary myoblasts based on α7 integrin expression. Exp. Cell Res. 265, 212–220. doi: 10.1006/excr.2001.5191
Blau, H. M., Cosgrove, B. D., and Ho, A. T. (2015). The central role of muscle stem cells in regenerative failure with aging. Nat. Med. 21, 854–862. doi: 10.1038/nm.3918
Boontheekul, T., Kong, H.-J., and Mooney, D. J. (2005). Controlling alginate gel degradation utilizing partial oxidation and bimodal molecular weight distribution. Biomaterials 26, 2455–2465. doi: 10.1016/j.biomaterials.2004.06.044
Borschel, G. H., Dennis, R. G., and Kuzon, W. M. (2004). Contractile skeletal muscle tissue-engineered on an acellular scaffold. Plastic Reconstr. Surg. 113, 595–602. doi: 10.1097/01.PRS.0000101064.62289.2F
Borschel, G. H., Dow, D. E., Dennis, R. G., and Brown, D. L. (2006). Tissue-engineered axially vascularized contractile skeletal muscle. Plastic Reconstr. Surg. 117, 2235–2242. doi: 10.1097/01.prs.0000224295.54073.49
Borselli, C., Cezar, C. A., Shvartsman, D., Vandenburgh, H. H., and Mooney, D. J. (2011). The role of multifunctional delivery scaffold in the ability of cultured myoblasts to promote muscle regeneration. Biomaterials 32, 8905–8914. doi: 10.1016/j.biomaterials.2011.08.019
Borselli, C., Storrie, H., Benesch-Lee, F., Shvartsman, D., Cezar, C., Lichtman, J. W., et al. (2010). Functional muscle regeneration with combined delivery of angiogenesis and myogenesis factors. Proc. Natl. Acad. Sci. U.S.A. 107, 3287–3292. doi: 10.1073/pnas.0903875106
Boström, P., Wu, J., Jedrychowski, M. P., Korde, A., Ye, L., Lo, J. C., et al. (2012). A PGC1 alpha dependent myokine that drives brown fat like development of white fat and thermogenesis. Nature 481, 463–468. doi: 10.1038/nature10777
Broholm, C., Laye, M. J., Brandt, C., Vadalasetty, R., Pilegaard, H., Pedersen, B. K., et al. (2011). LIF is a contraction-induced myokine stimulating human myocyte proliferation. J. Appl. Physiol. 111, 251–259. doi: 10.1152/japplphysiol.01399.2010
Brown, A. C., and Barker, T. H. (2014). Fibrin-based biomaterials: Modulation of macroscopic properties through rational design at the molecular level. Acta Biomat. 10, 1502–1514. doi: 10.1016/j.actbio.2013.09.008
Bursac, N., Juhas, M., and Rando, T. A. (2015). Synergizing engineering and biology to treat and model skeletal muscle injury and disease. Annu. Rev. Biomed. Eng. 17, 217–242. doi: 10.1146/annurev-bioeng-071114-040640
Busquets, S., Figueras, M. T., Meijsing, S., Carbó, N., Quinn, L. S., Almendro, V., et al. (2005). Interleukin-15 decreases proteolysis in skeletal muscle: a direct effect. Int. J. Mol. Med. 16, 471–476. doi: 10.3892/ijmm.16.3.471
Candiani, G., Riboldi, S. A., Sadr, N., Lorenzoni, S., Neuenschwander, P., Montevecchi, F. M., et al. (2010). Cyclic mechanical stimulation favors myosin heavy chain accumulation in engineered skeletal muscle constructs. J. Appl. Biomat. Biomech. 8, 68–75.
Carosio, S., Berardinelli, M. G., Aucello, M., and Musarò, A. (2011). Impact of ageing on muscle cell regeneration. Ageing Res. Rev. 10, 35–42. doi: 10.1016/j.arr.2009.08.001
Catoire, M., Mensink, M., Kalkhoven, E., Schrauwen, P., and Kersten, S. (2014). Identification of human exercise-induced myokines using secretome analysis. Physiol. Gen. 46, 256–267. doi: 10.1152/physiolgenomics.00174.2013
Cavazza, M., Kodra, Y., Armeni, P., De Santis, M., López-Bastida, J., Linertová, R., et al. (2016). Social/economic costs and health-related quality of life in patients with Duchenne muscular dystrophy in Europe. Eur. J. Health Econ. 17, 19–29. doi: 10.1007/s10198-016-0782-5
Chang, S. C., Tobias, G., Roy, A. K., Vacanti, C. A., and Bonassar, L. J. (2003). Tissue engineering of autologous cartilage for craniofacial reconstruction by injection molding. Plastic Reconstr. Surg. 112, 793–799. doi: 10.1097/01.PRS.0000069711.31021.94
Chargé, S. B., and Rudnicki, M. A. (2004). Cellular and molecular regulation of muscle regeneration. Physiol. Rev. 84, 209–238. doi: 10.1152/physrev.00019.2003
Cheema, U., Brown, R., Mudera, V., Yang, S. Y., McGrouther, G., and Goldspink, G. (2005). Mechanical signals and IGF-I gene splicing in vitro in relation to development of skeletal muscle. J. Cell. Physiol. 202, 67–75. doi: 10.1002/jcp.20107
Cheema, U., Yang, S.-Y., Mudera, V., Goldspink, G. G., and Brown, R. A. (2003). 3-D in vitro model of early skeletal muscle development. Cell Motility Cytoskeleton 54, 226–236. doi: 10.1002/cm.10095
Chen, M.-C., Sun, Y.-C., and Chen, Y.-H. (2013). Electrically conductive nanofibers with highly oriented structures and their potential application in skeletal muscle tissue engineering. Acta Biomaterialia 9, 5562–5572. doi: 10.1016/j.actbio.2012.10.024
Choi, J. S., Lee, S. J., Christ, G. J., Atala, A., and Yoo, J. J. (2008). The influence of electrospun aligned poly(ε-caprolactone)/collagen nanofiber meshes on the formation of self-aligned skeletal muscle myotubes. Biomaterials 29, 2899–2906. doi: 10.1016/j.biomaterials.2008.03.031
Cittadella Vigodarzere, G., and Mantero, S. (2014). Skeletal muscle tissue engineering: strategies for volumetric constructs. Front. Physiol. 5:362. doi: 10.3389/fphys.2014.00362
Colaianni, G., Cinti, S., Colucci, S., and Grano, M. (2017). Irisin and musculoskeletal health. Ann. N. Y. Acad. Sci. 1402, 5–9. doi: 10.1111/nyas.13345
Colaianni, G., Cuscito, C., Mongelli, T., Oranger, A., Mori, G., Brunetti, G., et al. (2014). Irisin enhances osteoblast differentiation in vitro. Int. J. Endocrinol. 2014:902186. doi: 10.1155/2014/902186
Colaianni, G., Cuscito, C., Mongelli, T., Pignataro, P., Buccoliero, C., Liu, P., et al. (2015). The myokine irisin increases cortical bone mass. Proc. Natl. Acad. Sci. U.S.A. 112, 12157–12162. doi: 10.1073/pnas.1516622112
Colaianni, G., and Grano, M. (2015). Role of Irisin on the bone – muscle functional unit. BoneKEy Rep. 4, 1–4. doi: 10.1038/bonekey.2015.134
Collinsworth, A. M., Zhang, S., Kraus, W. E., and Truskey, G. A. (2002). Apparent elastic modulus and hysteresis of skeletal muscle cells throughout differentiation. AJP Cell Physiol. 283, C1219–C1227. doi: 10.1152/ajpcell.00502.2001
Conconi, M. T., De Coppi, P., Bellini, S., Zara, G., Sabatti, M., Marzaro, M., et al. (2005). Homologous muscle acellular matrix seeded with autologous myoblasts as a tissue-engineering approach to abdominal wall-defect repair. Biomaterials 26, 2567–2574. doi: 10.1016/j.biomaterials.2004.07.035
Constantin, B. (2014). Dystrophin complex functions as a scaffold for signalling proteins. Biochim. Biophys. Acta 1838, 635–642. doi: 10.1016/j.bbamem.2013.08.023
Corona, B. T., Machingal, M. A., Criswell, T., Vadhavkar, M., Dannahower, A. C., Bergman, C., et al. (2012). Further development of a tissue engineered muscle repair construct in vitro for enhanced functional recovery following implantation in vivo in a murine model of volumetric muscle loss injury. Tissue Eng. 18, 1213–1228. doi: 10.1089/ten.tea.2011.0614
Corona, B. T., Ward, C. L., Baker, H. B., Walters, T. J., and Christ, G. J. (2014). Implantation of in vitro tissue engineered muscle repair constructs and bladder acellular matrices partially restore in vivo skeletal muscle function in a rat model of volumetric muscle loss injury. Tissue Eng. 20, 705–715. doi: 10.1089/ten.TEA.2012.0761
Corso, P., Finkelstein, E., Miller, T., Fiebelkorn, I., and Zaloshnja, E. (2006). Incidence and lifetime costs of injuries in the United States. Inj. Prev. 12, 212–218. doi: 10.1136/ip.2005.010983
Crisan, M., Yap, S., Casteilla, L., Chen, C.-W., Corselli, M., Park, T. S., et al. (2008). A perivascular origin for mesenchymal stem cells in multiple human organs. Cell Stem Cell 3, 301–313. doi: 10.1016/j.stem.2008.07.003
Darabi, R., Arpke, R. W., Irion, S., Dimos, J. T., Grskovic, M., Kyba, M., et al. (2012). Human ES- and iPS-derived myogenic progenitors restore DYSTROPHIN and improve contractility upon transplantation in dystrophic mice. Cell Stem Cell 10, 610–619. doi: 10.1016/j.stem.2012.02.015
De Angelis, L., Berghella, L., Coletta, M., Lattanzi, L., Zanchi, M., Cusella-De Angelis, M. G., et al. (1999). Skeletal myogenic progenitors originating from embryonic dorsal aorta coexpress endothelial and myogenic markers and contribute to postnatal muscle growth and regeneration. J. Cell Biol. 147, 869–878. doi: 10.1083/jcb.147.4.869
De Bari, C., Dell'Accio, F., Vandenabeele, F., Vermeesch, J. R., Raymackers, J.-M., and Luyten, F. P. (2003). Skeletal muscle repair by adult human mesenchymal stem cells from synovial membrane. J. Cell Biol. 160, 909–918. doi: 10.1083/jcb.200212064
De Coppi, P., Bellini, S., Conconi, M. T., Sabatti, M., Simonato, E., Gamba, P. G., et al. (2006). Myoblast–acellular skeletal muscle matrix constructs guarantee a long-term repair of experimental full-thickness abdominal wall defects. Tissue Eng. 12, 1929–1936. doi: 10.1089/ten.2006.12.1929
Dellavalle, A., Sampaolesi, M., Tonlorenzi, R., Tagliafico, E., Sacchetti, B., et al. (2007). Pericytes of human skeletal muscle are myogenic precursors distinct from satellite cells. Nat. Cell Biol. 9, 255–267. doi: 10.1038/ncb1542
DeQuach, J. A., Lin, J. E., Cam, C., Hu, D., Salvatore, M. A., Sheikh, F., et al. (2012). Injectable skeletal muscle matrix hydrogel promotes neovascularization and muscle cell infiltration in a hindlimb ischemia model. Eur. Cells Mater. 23, 400–412; discussion 412. doi: 10.22203/eCM.v023a31
Dezawa, M., Ishikawa, H., Itokazu, Y., Yoshihara, T., Hoshino, M., Takeda, S., et al. (2005). Bone marrow stromal cells generate muscle cells and repair muscle degeneration. Science 309, 314–317. doi: 10.1126/science.1110364
Donnelly, K., Ph, D., Khodabukus, A., Philp, A., Deldicque, L., Dennis, R. G., Baar, K., et al. (2010). A novel bioreactor for stimulating skeletal muscle in vitro. Tissue Eng. Part C Methods 16, 711–718. doi: 10.1089/ten.TEC.2009.0125
Duan, D. (2015). Duchenne muscular dystrophy gene therapy in the canine model. Hum. Gene Ther. Clin. Dev. 26, 57–69. doi: 10.1089/humc.2015.006
Dumont, N. A., Bentzinger, C. F., Sincennes, M.-C., and Rudnicki, M. A. (2015a). Satellite cells and skeletal muscle regeneration. Compr. Physiol. 5, 1027–1059. doi: 10.1002/cphy.c140068
Dumont, N. A., and Rudnicki, M. A. (2016). Targeting muscle stem cell intrinsic defects to treat Duchenne muscular dystrophy. NPJ Regen. Med. 1:16006. doi: 10.1038/npjregenmed.2016.6
Dumont, N. A., Wang, Y. X., von Maltzahn, J., Pasut, A., Bentzinger, C. F., Brun, C. E., et al. (2015b). Dystrophin expression in muscle stem cells regulates their polarity and asymmetric division. Nat. Med. 21, 1455–1463. doi: 10.1038/nm.3990
Dunstan, D. (2011). Diabetes: exercise and T2DM—move muscles more often! Nat. Rev. Endocrinol. 7, 189–190. doi: 10.1038/nrendo.2011.35
Egan, B., and Zierath, J. R. (2013). Exercise metabolism and the molecular regulation of skeletal muscle adaptation. Cell Metab. 17, 162–184. doi: 10.1016/j.cmet.2012.12.012
Engler, A. J., Griffin, M. A., Sen, S., Bönnemann, C. G., Sweeney, H. L., and Discher, D. E. (2004). Myotubes differentiate optimally on substrates with tissue-like stiffness: pathological implications for soft or stiff microenvironments. J. Cell Biol. 166, 877–887. doi: 10.1083/jcb.200405004
Engler, A. J., Sen, S., Sweeney, H. L., and Discher, D. E. (2006). Matrix elasticity directs stem cell lineage specification. Cell 126, 677–689. doi: 10.1016/j.cell.2006.06.044
Engler, A. J., Sweeney, H. L., Discher, D. E., and Schwarzbauer, J. E. (2007). Extracellular matrix elasticity directs stem cell differentiation. J. Musculoskeletal Neuronal Interact. 7:335.
Ervasti, J. M., and Sonnemann, K. J. (2008). Biology of the striated muscle dystrophin-glycoprotein complex. Int. Rev. Cytol. 265, 191–225. doi: 10.1016/S0074-7696(07)65005-0
Ferrari, G., Cusella-De Angelis, G., Coletta, M., Paolucci, E., Stornaiuolo, A., Cossu, G., et al. (1998). Muscle regeneration by bone marrow-derived myogenic progenitors. Science 279, 1528–1530. doi: 10.1126/science.279.5356.1528
Ferrari, G., Stornaiuolo, A., and Mavilio, F. (2001). Bone-marrow transplantation: failure to correct murine muscular dystrophy. Nature 411, 1014–1015. doi: 10.1038/35082631
Ferreira, M. M., Dewi, R. E., and Heilshorn, S. C. (2015). Microfluidic analysis of extracellular matrix-bFGF crosstalk on primary human myoblast chemoproliferation, chemokinesis, and chemotaxis. Int. Biol. 7, 569–579. doi: 10.1039/C5IB00060B
Fishman, J. M., Tyraskis, A., Maghsoudlou, P., Urbani, L., Totonelli, G., Birchall, M. A., et al. (2013). Skeletal muscle tissue engineering: which cell to use? Tissue Eng. Part B Rev. 19, 503–515. doi: 10.1089/ten.teb.2013.0120
Forcales, S.-V. (2015). Potential of adipose-derived stem cells in muscular regenerative therapies. Front. Aging Neurosci. 7:123. doi: 10.3389/fnagi.2015.00123
Fujita, H., Nedachi, T., and Kanzaki, M. (2007). Accelerated de novo sarcomere assembly by electric pulse stimulation in C2C12 myotubes. Exp. Cell Res. 313, 1853–1865. doi: 10.1016/j.yexcr.2007.03.002
Fuoco, C., Rizzi, R., Biondo, A., Longa, E., Mascaro, A., Shapira-Schweitzer, K., et al. (2015). In vivo generation of a mature and functional artificial skeletal muscle. EMBO Mol. Med. 7, 411–422. doi: 10.15252/emmm.201404062
Fuoco, C., Salvatori, M. L., Biondo, A., Shapira-Schweitzer, K., Santoleri, S., Antonini, S., et al. (2012). Injectable polyethylene glycol-fibrinogen hydrogel adjuvant improves survival and differentiation of transplanted mesoangioblasts in acute and chronic skeletal-muscle degeneration. Skel. Muscle 2:24. doi: 10.1186/2044-5040-2-24
Fuoco, C., Sangalli, E., Vono, R., Testa, S., Sacchetti, B., Latronico, M. V., et al. (2014). 3D hydrogel environment rejuvenates aged pericytes for skeletal muscle tissue engineering. Front. Physiol. 5:203. doi: 10.3389/fphys.2014.00203
Furmanczyk, P. S., and Quinn, L. S. (2003). Interleukin-15 increases myosin accretion in human skeletal myogenic cultures. Cell Biol. Int. 27, 845–851. doi: 10.1016/S1065-6995(03)00172-0
Gawlik, K. I., Holmberg, J., and Durbeej, M. (2014). Loss of dystrophin and β-sarcoglycan significantly exacerbates the phenotype of laminin α2 chain-deficient animals. Am. J. Pathol. 184, 740–752. doi: 10.1016/j.ajpath.2013.11.017
Girgis, C. M., Mokbel, N., and DiGirolamo, D. J. (2014). Therapies for musculoskeletal disease: can we treat two birds with one stone? Curr. Osteoporosis Rep. 12, 142–153. doi: 10.1007/s11914-014-0204-5
Gnecchi, M., He, H., Liang, O. D., Melo, L. G., Morello, F., Mu, H., et al. (2005). Paracrine action accounts for marked protection of ischemic heart by Akt-modified mesenchymal stem cells. Nat. Med. 11, 367–368. doi: 10.1038/nm0405-367
Gnecchi, M., Zhang, Z., Ni, A., and Dzau, V. J. (2008). Paracrine mechanisms in adult stem cell signaling and therapy. Circ. Res. 103, 1204–1219. doi: 10.1161/CIRCRESAHA.108.176826
Goldberg, A. (1967). Work-induced growth of skeletal muscle in normal and hypophysectomized rats. Am. J. Physiol. 213, 1193–1198.
Goldspink, G. (1999). Changes in muscle mass and phenotype and the expression of autocrine and systemic growth factors by muscle in response to stretch and overload. J. Anat. 194 (Pt 3), 323–334. doi: 10.1046/j.1469-7580.1999.19430323.x
Goldspink, G. (2003). Gene expression in muscle in response to exercise. J. Muscle Res. Cell Motility 24, 121–126. doi: 10.1023/A:1026041228041
Goldspink, G., Scutt, A., Loughna, P. T., Wells, D. J., Jaenicke, T., and Gerlach, G. F. (1992). Gene expression in skeletal muscle in response to stretch and force generation. Am. J. Physiol. 262, R356–R363. doi: 10.1152/ajpregu.1992.262.3.R356
Goyenvalle, A., Seto, J. T., Davies, K. E., and Chamberlain, J. (2011). Therapeutic approaches to muscular dystrophy. Hum. Mol. Genet. 20, R69–R78. doi: 10.1093/hmg/ddr105
Grefte, S., Vullinghs, S., Kuijpers-Jagtman, A. M., Torensma, R., and Von den Hoff, J. W. (2012). Matrigel, but not collagen I, maintains the differentiation capacity of muscle derived cells in vitro. Biomed. Materials 7:55004. doi: 10.1088/1748-6041/7/5/055004
Grizzi, I., Garreau, H., Li, S., and Vert, M. (1995). Hydrolytic degradation of devices based on poly(DL-lactic acid) size-dependence. Biomaterials 16, 305–11. doi: 10.1016/0142-9612(95)93258-F
Guerci, A., Lahoute, C., Hébrard, S., Collard, L., Graindorge, D., Favier, M., et al. (2012). Srf-dependent paracrine signals produced by myofibers control satellite cell-mediated skeletal muscle hypertrophy. Cell Metab. 15, 25–37. doi: 10.1016/j.cmet.2011.12.001
Guettier-Sigrist, S., Coupin, G., Braun, S., Warter, J.-M., and Poindron, P. (1998). Muscle could be the therapeutic target in SMA treatment. J. Neurosci. Res. 53, 663–669. doi: 10.1002/(SICI)1097-4547(19980915)53:6<663::AID-JNR4>3.0.CO;2-3
Gunatillake, P. A., Adhikari, R., and Gadegaard, N. (2003). Biodegradable synthetic polymers for tissue engineering. Eur. Cells Materials 5, 1–16. doi: 10.22203/eCM.v005a01
Guyon, J. R., Steffen, L. S., Howell, M. H., Pusack, T. J., Lawrence, C., and Kunkel, L. M. (2007). Modeling human muscle disease in zebrafish. Biochim. Biophys. Acta 1772, 205–215. doi: 10.1016/j.bbadis.2006.07.003
Hammers, D. W., Sarathy, A., Pham, C. B., Drinnan, C. T., Farrar, R. P., and Suggs, L. J. (2012). Controlled release of IGF-I from a biodegradable matrix improves functional recovery of skeletal muscle from ischemia/reperfusion. Biotechnol. Bioeng. 109, 1051–1059. doi: 10.1002/bit.24382
Han, W. M., Jang, Y. C., and García, A. J. (2016). Engineered matrices for skeletal muscle satellite cell engraftment and function. Matrix Biol. 60–61, 96–109. doi: 10.1016/j.matbio.2016.06.001
Harries, L. W., McCulloch, L. J., Holley, J. E., Rawling, T. J., Welters, H. J., and Kos, K. (2013). A role for SPARC in the moderation of human insulin secretion. PLoS ONE 8:68253. doi: 10.1371/journal.pone.0068253
Haugen, F., Norheim, F., Lian, H., Wensaas, A. J., Dueland, S., Berg, O., et al. (2010). IL-7 is expressed and secreted by human skeletal muscle cells. Am. J. Physiol Cell Physiol. 298, C807–C816. doi: 10.1152/ajpcell.00094.2009
Heher, P., Maleiner, B., Prüller, J., Teuschl, A. H., Kollmitzer, J., Monforte, X., et al. (2015). A novel bioreactor for the generation of highly aligned 3D skeletal muscle-like constructs through orientation of fibrin via application of static strain. Acta Biomaterialia 24, 251–265. doi: 10.1016/j.actbio.2015.06.033
DiOrio, J., Tawil, J. B., Bittner, K., and Spaethe, R. (2004). Fibrin, Encyclopedia of Biomaterials and Biomedical Engineering. (New York, NY: Marcel Dekker), 603–610.
Hill, E., Boontheekul, T., and Mooney, D. J. (2006a). Designing scaffolds to enhance transplanted myoblast survival and migration. Tissue Eng. 12, 1295–1304. doi: 10.1089/ten.2006.12.1295
Hill, E., Boontheekul, T., and Mooney, D. J. (2006b). Regulating activation of transplanted cells controls tissue regeneration. Proc. Natl. Acad. Sci. U.S.A. 103, 2494–2499. doi: 10.1073/pnas.0506004103
Hinds, S., Bian, W., Dennis, R. G., and Bursac, N. (2011). The role of extracellular matrix composition in structure and function of bioengineered skeletal muscle. Biomaterials 32, 3575–3583. doi: 10.1016/j.biomaterials.2011.01.062
Hojman, P., Pedersen, M., Nielsen, A. R., Krogh-Madsen, R., Yfanti, C., Akerstrom, T., et al. (2009). Fibroblast growth factor-21 is induced in human skeletal muscles by hyperinsulinemia. Diabetes 58, 2797–2801. doi: 10.2337/db09-0713
Horch, R. E., Debus, M., Wagner, G., and Stark, G. B. (2000). Cultured human keratinocytes on type I collagen membranes to reconstitute the epidermis. Tissue Eng. 6, 53–67. doi: 10.1089/107632700320892
Hosseini, V., Ahadian, S., Ostrovidov, S., Camci-Unal, G., Chen, S., Kaji, H., et al. (2012). Engineered contractile skeletal muscle tissue on a microgrooved methacrylated gelatin substrate. Tissue Eng. Part A 18, 2453–2465. doi: 10.1089/ten.tea.2012.0181
Huang, N. F., Patel, S., Thakar, R. G., Wu, J., Hsiao, B. S., Chu, B., et al. (2006a). Myotube assembly on nanofibrous and micropatterned polymers. Nano Lett. 6, 537–542. doi: 10.1021/nl060060o
Huang, Y.-C., Dennis, R. G., and Baar, K. (2006b). Cultured slow vs. fast skeletal muscle cells differ in physiology and responsiveness to stimulation. AJP Cell Physiol. 291, C11–C17. doi: 10.1152/ajpcell.00366.2005
Huang, Y.-C., Dennis, R. G., Larkin, L., and Baar, K. (2004). Rapid formation of functional muscle in vitro using fibrin gels. J. Appl. Physiol. 98, 706–713. doi: 10.1152/japplphysiol.00273.2004
Izumiya, Y., Bina, H. A., Ouchi, N., Akasaki, Y., Kharitonenkov, A., and Walsh, K. (2008). FGF21 is an Akt-regulated myokine. FEBS Lett. 582, 3805–3810. doi: 10.1016/j.febslet.2008.10.021
Jana, S., Cooper, A., and Zhang, M. (2013). Chitosan scaffolds with unidirectional microtubular pores for large skeletal myotube generation. Adv. Healthcare Mater. 2, 557–561. doi: 10.1002/adhm.201200177
Janssen, I., Shepard, D. S., Katzmarzyk, P. T., and Roubenoff, R. (2004). The healthcare costs of sarcopenia in the United States. J. Am. Geriatrics Soc. 52, 80–85. doi: 10.1111/j.1532-5415.2004.52014.x
Joe, A. W., Yi, L., Natarajan, A., Le Grand, F., So, L., Wang, J., et al. (2010). Muscle injury activates resident fibro/adipogenic progenitors that facilitate myogenesis. Nat. Cell Biol. 12, 153–163. doi: 10.1038/ncb2015
Juhas, M., and Bursac, N. (2014). Roles of adherent myogenic cells and dynamic culture in engineered muscle function and maintenance of satellite cells. Biomaterials 35, 9438–9446. doi: 10.1016/j.biomaterials.2014.07.035
Juhas, M., Ye, J., and Bursac, N. (2015). Design, evaluation, and application of engineered skeletal muscle. Methods 99, 81–90. doi: 10.1016/j.ymeth.2015.10.002
Jun, I., Jeong, S., and Shin, H. (2009). The stimulation of myoblast differentiation by electrically conductive sub-micron fibers. Biomaterials 30, 2038–2047. doi: 10.1016/j.biomaterials.2008.12.063
Kasper, A. M., Turner, D. C., Martin, N. R. W., and Sharples, A. P. (2017). Mimicking exercise in three-dimensional bioengineered skeletal muscle to investigate cellular and molecular mechanisms of physiological adaptation. J. Cell. Physiol. 233, 1985–1998. doi: 10.1002/jcp.25840
Katz, B. (1961). The termination of the afferent nerve fibre in the muscle spindle of the frog. Biol. Sci. 243, 222–240. doi: 10.1098/rstb.1961.0001
Khodabukus, A., and Baar, K. (2012). Defined electrical stimulation emphasizing excitability for the development and testing of engineered skeletal muscle. Tissue Eng. Part C Methods 18, 349–357. doi: 10.1089/ten.tec.2011.0364
Khodabukus, A., Baehr, L. M., Bodine, S. C., and Baar, K. (2015). Role of contraction duration in inducing fast-to-slow contractile and metabolic protein and functional changes in engineered muscle. J. Cell. Physiol. 230, 2489–2497. doi: 10.1002/jcp.24985
Kim, M. H., Hong, H. N., Hong, J. P., Park, C. J., Kwon, S. W., Kim, S. H., et al. (2010a). The effect of VEGF on the myogenic differentiation of adipose tissue derived stem cells within thermosensitive hydrogel matrices. Biomaterials 31, 1213–1218. doi: 10.1016/j.biomaterials.2009.10.057
Kim, M. S., Jun, I., Shin, Y. M., Jang, W., Kim, S. I., and Shin, H. (2010b). The development of genipin-crosslinked poly(caprolactone) (PCL)/gelatin nanofibers for tissue engineering applications. Macromol. Biosci. 10, 91–100. doi: 10.1002/mabi.200900168
Kinnaird, T., Stabile, E., Burnett, M. S., Lee, C. W., Barr, S., Fuchs, S., et al. (2004). Marrow-derived stromal cells express genes encoding a broad spectrum of arteriogenic cytokines and promote in vitro and in vivo arteriogenesis through paracrine mechanisms. Circulation Res. 94, 678–685. doi: 10.1161/01.RES.0000118601.37875.AC
Kodaka, Y., Rabu, G., and Asakura, A. (2017). Skeletal muscle cell induction from pluripotent stem cells. Stem Cells Int. 2017, 1–16. doi: 10.1155/2017/1376151
Kojima, K., Bonassar, L. J., Ignotz, R. A., Syed, K., Cortiella, J., and Vacanti, C. A. (2003). Comparison of tracheal and nasal chondrocytes for tissue engineering of the trachea. Ann. Thoracic Surg. 76, 1884–1888. doi: 10.1016/S0003-4975(03)01193-7
Koponen, J. K., Kekarainen, T., Heinonen, S. E., Laitinen, A., Nystedt, J., Laine, J., et al. (2007). Umbilical cord blood–derived progenitor cells enhance muscle regeneration in mouse hindlimb ischemia model. Mol. Ther. 15, 2172–2177. doi: 10.1038/sj.mt.6300302
Kopp, J., Jeschke, M. G., Bach, A. D., Kneser, U., and Horch, R. E. (2004). Applied tissue engineering in the closure of severe burns and chronic wounds using cultured human autologous keratinocytes in a natural fibrin matrix. Cell Tiss. Banking 5, 81–87. doi: 10.1023/B:CATB.0000034082.29214.3d
Kortesidis, A., Zannettino, A., Isenmann, S., Shi, S., Lapidot, T., and Gronthos, S. (2005). Stromal-derived factor-1 promotes the growth, survival, and development of human bone marrow stromal stem cells. Blood 105, 3793–3801. doi: 10.1182/blood-2004-11-4349
Kroehne, V., Heschel, I., Schügner, F., Lasrich, D., Bartsch, J. W., and Jockusch, H. (2008). Use of a novel collagen matrix with oriented pore structure for muscle cell differentiation in cell culture and in grafts. J. Cell. Mol. Med. 12, 1640–1648. doi: 10.1111/j.1582-4934.2008.00238.x
Ku, S. H., Lee, S. H., and Park, C. B. (2012). Synergic effects of nanofiber alignment and electroactivity on myoblast differentiation. Biomaterials 33, 6098–6104. doi: 10.1016/j.biomaterials.2012.05.018
Kurek, J. B., Bower, J. J., Romanella, M., Koentgen, F., Murphy, M., and Austin, L. (1997). The role of leukemia inhibitory factor in skeletal muscle regeneration. Muscle Nerve 20, 815–822. doi: 10.1002/(SICI)1097-4598(199707)20:7<815::AID-MUS5>3.0.CO;2-A
Kurek, J. B., Nouri, S., Kannourakis, G., Murphy, M., and Austin, L. (1996). Leukemia inhibitory factor and interleukin-6 are produced by diseased and regenerating skeletal muscle. Muscle Nerve 19, 1291–1301. doi: 10.1002/(SICI)1097-4598(199610)19:10<1291::AID-MUS6>3.0.CO;2-9
Kurth, F., Franco-Obregón, A., Casarosa, M., Küster, S. K., Wuertz-Kozak, K., and Dittrich, P. S. (2015). Transient receptor potential vanilloid 2-mediated shear-stress responses in C2C12 myoblasts are regulated by serum and extracellular matrix. FASEB J. 29, 4726–4737. doi: 10.1096/fj.15-275396
Lam, M. T., Huang, Y. C., Birla, R. K., and Takayama, S. (2009). Microfeature guided skeletal muscle tissue engineering for highly organized 3-dimensional free-standing constructs. Biomaterials 30, 1150–1155. doi: 10.1016/j.biomaterials.2008.11.014
Landfeldt, E., Lindgren, P., Bell, C. F., Schmitt, C., Guglieri, M., Straub, V., et al. (2014). The burden of Duchenne muscular dystrophy: an international, cross-sectional study. Neurology 83, 529–536. doi: 10.1212/WNL.0000000000000669
Langelaan, M. L., Boonen, K. J., Rosaria-Chak, K. Y., van der Schaft, D. W., Post, M. J., and Baaijens, F. P. (2011). Advanced maturation by electrical stimulation: Differences in response between C2C12 and primary muscle progenitor cells. J. Tissue Eng. Regenerat. Med. 5, 529–539. doi: 10.1002/term.345
Law, P. K., Goodwin, T. G., Fang, Q., Deering, M. B., Duggirala, V., Larkin, C., et al. (1993). Cell transplantation as an experimental treatment for Duchenne muscular dystrophy. Cell Transplant. 2, 485–505. doi: 10.1177/096368979300200607
Le Blanc, K., and Mougiakakos, D. (2012). Multipotent mesenchymal stromal cells and the innate immune system. Nat. Rev. Immunol. 12, 383–396. doi: 10.1038/nri3209
Lee, C. H., Singla, A., and Lee, Y. (2001). Biomedical applications of collagen. Int. J. Pharmaceut. 221, 1–22. doi: 10.1016/S0378-5173(01)00691-3
Lee, H., Park, J., Forget, B. G., and Gaines, P. (2009). Induced pluripotent stem cells in regenerative medicine: an argument for continued research on human embryonic stem cells. Regen. Med. 4, 759–769. doi: 10.2217/rme.09.46
Lee, J. W., Fang, X., Krasnodembskaya, A., Howard, J. P., and Matthay, M. A. (2011). Concise review: mesenchymal stem cells for acute lung injury: role of paracrine soluble factors. Stem Cells 29, 913–919. doi: 10.1002/stem.643
Lee, K. Y., and Mooney, D. J. (2012). Alginate: properties and biomedical applications. Prog. Polymer Sci. 37, 106–126. doi: 10.1016/j.progpolymsci.2011.06.003
Liao, I.-C., Liu, J. B., Bursac, N., and Leong, K. W. (2008). Effect of electromechanical stimulation on the maturation of myotubes on aligned electrospun fibers. Cell. Mol. Bioeng. 1, 133–145. doi: 10.1007/s12195-008-0021-y
Liu, J., Xu, H. H., Zhou, H., Weir, M. D., Chen, Q., and Trotman, C. A. (2013). Human umbilical cord stem cell encapsulation in novel macroporous and injectable fibrin for muscle tissue engineering. Acta Biomaterialia 9, 4688–4697. doi: 10.1016/j.actbio.2012.08.009
Liu, J., Zhou, H., Weir, M. D., Xu, H. H., Chen, Q., and Trotman, C. A. (2012). Fast-degradable microbeads encapsulating human umbilical cord stem cells in alginate for muscle tissue engineering. Tissue Eng. Part A 18, 2303–2314. doi: 10.1089/ten.tea.2011.0658
Luo, Y., Kirker, K. R., and Prestwich, G. D. (2000). Cross-linked hyaluronic acid hydrogel films: new biomaterials for drug delivery. J. Controlled Release 69, 169–184. doi: 10.1016/S0168-3659(00)00300-X
Luo, Z., Luo, P., Yu, Y., Zhao, Q., Zhao, X., and Cheng, L. (2012). SPARC promotes the development of erythroid progenitors. Exp. Hematol. 40, 828–836. doi: 10.1016/j.exphem.2012.06.002
Ma, J., Holden, K., Zhu, J., Pan, H., and Li, Y. (2011). The application of three-dimensional collagen-scaffolds seeded with myoblasts to repair skeletal muscle defects. J. Biomed. Biotechnol. 2011:812135. doi: 10.1155/2011/812135
Machingal, M. A., Corona, B. T., Walters, T. J., Kesireddy, V., Koval, C. N., Dannahower, A., et al. (2011). A tissue-engineered muscle repair construct for functional restoration of an irrecoverable muscle injury in a murine model. Tissue Eng. Part A 17, 2291–2303. doi: 10.1089/ten.tea.2010.0682
Madden, L., Juhas, M., Kraus, W. E., Truskey, G. A., and Bursac, N. (2015). Bioengineered human myobundles mimic clinical responses of skeletal muscle to drugs. eLife 4:e04885. doi: 10.7554/eLife.04885
Mah, J. K., Korngut, L., Dykeman, J., Day, L., Pringsheim, T., and Jette, N. (2014). A systematic review and meta-analysis on the epidemiology of Duchenne and Becker muscular dystrophy. Neuromuscul. Disord. 24, 482–491. doi: 10.1016/j.nmd.2014.03.008
Malecova, B., and Puri, P. L. (2012). “Mix of Mics”- phenotypic and biological heterogeneity of “multipotent” muscle interstitial cells (MICs). J. Stem Cell Res. Ther. (Suppl 11):004.
Mandal, B. B., and Kundu, S. C. (2009). Cell proliferation and migration in silk fibroin 3D scaffolds. Biomaterials 30, 2956–2965. doi: 10.1016/j.biomaterials.2009.02.006
Marzaro, M., Conconi, M. T., Perin, L., Giuliani, S., Gamba, P., De Coppi, P., et al. (2002). Autologous Satellite Cell Seeding Improves in vivo Biocompatibility of Homologous Muscle Acellular Matrix Implants. [Accessed April 6, 2017].Available online at: http://www.ingentaconnect.com/content/sp/ijmm/2002/00000010/00000002/art00009
Mase, V. J., Hsu, J. R., Wolf, S. E., Wenke, J. C., Baer, D. G., Owens, J., et al. (2010). Clinical application of an acellular biologic scaffold for surgical repair of a large, traumatic quadriceps femoris muscle defect. Orthopedics 33:511. doi: 10.3928/01477447-20100526-24
Matsumoto, T., Sasaki, J., Alsberg, E., Egusa, H., Yatani, H., and Sohmura, T. (2007). Three-dimensional cell and tissue patterning in a strained fibrin gel system. PLoS ONE 2:1211. doi: 10.1371/journal.pone.0001211
Mauro, A. (1961). Satellite cell of skeletal muscle fibers. J. Biophys. Biochem. Cytol. 9, 493–495. doi: 10.1083/jcb.9.2.493
McCullen, S. D., Chow, A. G., and Stevens, M. M. (2011). In vivo tissue engineering of musculoskeletal tissues. Curr. Opin. Biotechnol. 22, 715–720. doi: 10.1016/j.copbio.2011.05.001
McGreevy, J. W., Hakim, C. H., McIntosh, M. A., and Duan, D. (2015). Animal models of Duchenne muscular dystrophy: from basic mechanisms to gene therapy. Dis. Model. Mech. 8, 195–213. doi: 10.1242/dmm.018424
Meregalli, M., Farini, A., Belicchi, M., Parolini, D., Cassinelli, L., Razini, P., et al. (2013). Perspectives of stem cell therapy in Duchenne muscular dystrophy. FEBS J. 280, 4251–4262. doi: 10.1111/febs.12083
Meregalli, M., Farini, A., Sitzia, C., and Torrente, Y. (2014). Advancements in stem cells treatment of skeletal muscle wasting. Front. Physiol. 5:48. doi: 10.3389/fphys.2014.00048
Merritt, E. K., Cannon, M. V., Hammers, D. W., Le, L. N., Gokhale, R., Sarathy, A., et al. (2010). Repair of traumatic skeletal muscle injury with bone-marrow-derived mesenchymal stem cells seeded on extracellular matrix. Tissue Eng. Part A 16, 2871–2881. doi: 10.1089/ten.tea.2009.0826
Minasi, M. G., Riminucci, M., De Angelis, L., Borello, U., Berarducci, B., Innocenzi, A., et al. (2002). The meso-angioblast: a multipotent, self-renewing cell that originates from the dorsal aorta and differentiates into most mesodermal tissues. Development 129, 2773–2783.
Monge, C., Ren, K., Berton, K., Guillot, R., Peyrade, D., and Picart, C. (2012). Engineering muscle tissues on microstructured polyelectrolyte multilayer films. Tissue Eng. Part A 18, 1664–1676. doi: 10.1089/ten.tea.2012.0079
Moon, D. G., Christ, G., Stitzel, J. D., Atala, A., and Yoo, J. J. (2008). Cyclic mechanical preconditioning improves engineered muscle contraction. Tissue Eng. Part A 14, 473–482. doi: 10.1089/tea.2007.0104
Morishima-Kawashima, M., Hasegawa, M., Takio, K., Suzuki, M., Yoshida, H., Titani, K., et al. (1995). Proline-directed and non-proline-directed phosphorylation of PHF-tau. J. Biol. Chem. 270, 823–829. doi: 10.1074/jbc.270.2.823
Mouly, V., Aamiri, A., Périé, S., Mamchaoui, K., Barani, A., Bigot, A., et al. (2005). Myoblast transfer therapy: is there any light at the end of the tunnel? Acta Myologica 24, 128–133.
Muñoz-Cánoves, P., Scheele, C., Pedersen, B. K., and Serrano, A. L. (2013). Interleukin-6 myokine signaling in skeletal muscle: a double-edged sword? FEBS J. 280, 4131–4148. doi: 10.1111/febs.12338
Murphy-Ullrich, J. E., and Sage, E. H. (2014). Revisiting the matricellular concept. Matrix Biol. 37, 1–14. doi: 10.1016/j.matbio.2014.07.005
Nam, K.-H., Smith, A. S., Lone, S., Kwon, S., and Kim, D.-H. (2015). Biomimetic 3D tissue models for advanced high-throughput drug screening. J. Lab. Automat. 20, 201–215. doi: 10.1177/2211068214557813
Nielsen, A. R., and Pedersen, B. K. (2007). The biological roles of exercise-induced cytokines: IL-6, IL-8, and IL-15. Appl. Physiol. Nutr. Metab. 32, 833–839. doi: 10.1139/H07-054
Nishizawa, H., Matsuda, M., Yamada, Y., Kawai, K., Suzuki, E., Makishima, M., et al. (2004). Musclin, a novel skeletal muscle-derived secretory factor. J. Biol. Chem. 279, 19391–19395. doi: 10.1074/jbc.C400066200
Oakes, B. W. (2004). Orthopaedic tissue engineering: from laboratory to the clinic. Med. J. Aust. 180(5 Suppl.), S35–S38.
Ohnishi, S., Sumiyoshi, H., Kitamura, S., and Nagaya, N. (2007). Mesenchymal stem cells attenuate cardiac fibroblast proliferation and collagen synthesis through paracrine actions. FEBS Lett. 581, 3961–3966. doi: 10.1016/j.febslet.2007.07.028
Okano, T., and Matsuda, T. (1998a). Muscular tissue engineering: capillary-incorporated hybrid muscular tissues in vivo tissue culture. Cell Transplant. 7, 435–442.
Okano, T., and Matsuda, T. (1998b). Tissue engineered skeletal muscle: preparation of highly dense, highly oriented hybrid muscular tissues. Cell Transplant. 7, 71–82.
Ostrovidov, S., Hosseini, V., Ahadian, S., Fujie, T., Parthiban, S. P., Ramalingam, M., et al. (2014). Skeletal muscle tissue engineering: methods to form skeletal myotubes and their applications. Tissue Eng. B 20. doi: 10.1089/ten.teb.2013.0534
Ostrovidov, S., Shi, X., Sadeghian, R. B., Salehi, S., Fujie, T., Bae, H., et al. (2015). Stem cell differentiation toward the myogenic lineage for muscle tissue regeneration: a focus on muscular dystrophy. Stem Cell Rev. Rep. 11, 866–884. doi: 10.1007/s12015-015-9618-4
Ouchi, N., Oshima, Y., Ohashi, K., Higuchi, A., Ikegami, C., Izumiya, Y., et al. (2008). Follistatin-like 1, a secreted muscle protein, promotes endothelial cell function and revascularization in ischemic tissue through a nitric-oxide synthase-dependent mechanism. J. Biol. Chem. 283, 32802–32811. doi: 10.1074/jbc.M803440200
Page, R. L., Malcuit, C., Vilner, L., Vojtic, I., Shaw, S., Hedblom, E., et al. (2011). Restoration of skeletal muscle defects with adult human cells delivered on fibrin microthreads. Tissue Eng. Part A 17, 2629–2640. doi: 10.1089/ten.tea.2011.0024
Papers, J. B. C., and Mayer, U. (2003). Integrins: redundant or important players in skeletal muscle. J. Biol. Chem. 17, 14587–14590. doi: 10.1074/jbc.R200022200
Park, H., Bhalla, R., Saigal, R., Radisic, M., Watson, N., Langer, R., et al. (2008). Effects of electrical stimulation in C2C12 muscle constructs. J. Tiss. Eng. Regenerat. Med. 2, 279–287. doi: 10.1002/term.93
Partridge, T. (2010). The potential of exon skipping for treatment for duchenne muscular dystrophy. J. Child Neurol. 25, 1165–1170. doi: 10.1177/0883073810371130
Partridge, T. A., Morgan, J. E., Coulton, G. R., Hoffman, E. P., and Kunkel, L. M. (1989). Conversion of mdx myofibres from dystrophin-negative to -positive by injection of normal myoblasts. Nature 337, 176–179. doi: 10.1038/337176a0
Pedersen, B. K. (2009). Edward F. Adolph distinguished lecture: muscle as an endocrine organ: IL-6 and other myokines. J. Appl. Physiol. 107, 1006–1014. doi: 10.1152/japplphysiol.00734.2009
Pedersen, B. K. (2011). Muscles and their myokines. J. Exp. Biol. 214, 337–346. doi: 10.1242/jeb.048074
Pedersen, B. K., and Febbraio, M. A. (2008). Muscle as an endocrine organ.pdf. Physiol. Rev. 88, 1379–1406. doi: 10.1152/physrev.90100.2007
Pedersen, B. K., Steensberg, A., and Schjerling, P. (2001). Muscle-derived interleukin-6: Possible biological effects. J. Physiol. 536, 329–337. doi: 10.1111/j.1469-7793.2001.0329c.xd
Perniconi, B., Costa, A., Aulino, P., Teodori, L., Adamo, S., and Coletti, D. (2011). The pro-myogenic environment provided by whole organ scale acellular scaffolds from skeletal muscle. Biomaterials 32, 7870–7882. doi: 10.1016/j.biomaterials.2011.07.016
Pistilli, E. E., Siu, P. M., and Alway, S. E. (2007). Interleukin-15 responses to aging and unloading-induced skeletal muscle atrophy. Am. J. Physiol. Cell Physiol. 292, C1298–C1304. doi: 10.1152/ajpcell.00496.2006
Powell, C. A., Smiley, B. L., Mills, J., and Vandenburgh, H. H. (2002). Mechanical stimulation improves tissue-engineered human skeletal muscle. AJP Cell Physiol. 283, C1557–C1565. doi: 10.1152/ajpcell.00595.2001
Purves, D., Augustine, G. J., Fitzpatrick, D., Katz, L. C., LaMantia, A. S., McNamara, J. O., et al. (2001). Neuroscience, 2nd Edn. Sunderland, MA: Sinauer Associates.
Qazi, T. H., Mooney, D. J., Pumberger, M., Geissler, S., and Duda, G. N. (2015). Biomaterials based strategies for skeletal muscle tissue engineering: existing technologies and future trends. Biomaterials 53, 502–521. doi: 10.1016/j.biomaterials.2015.02.110
Quattrocelli, M., Swinnen, M., Giacomazzi, G., Camps, J., Barthélemy, I., Ceccarelli, G., et al. (2015). Mesodermal iPSC – derived progenitor cells functionally regenerate cardiac and skeletal muscle. J. Clin. Invest.125, 4463–4482. doi: 10.1172/JCI82735
Quinn, L. (2002). Overexpression of interleukin-15 induces skeletal muscle hypertrophy in vitro: implications for treatment of muscle wasting disorders. Exp. Cell Res. 280, 55–63. doi: 10.1006/excr.2002.5624
Quinn, L. S., Anderson, B. G., Strait-Bodey, L., Stroud, A. M., and Argilés, J. M. (2008). Oversecretion of interleukin-15 from skeletal muscle reduces adiposity. AJP Endocrinol. Metab. 296, E191–E202. doi: 10.1152/ajpendo.90506.2008
Quinn, L. S., Haugk, K. L., and Grabstein, K. H. (1995). Interleukin-15: a novel anabolic cytokine for skeletal muscle. Endocrinology 136, 3669–3672. doi: 10.1210/endo.136.8.7628408
Rao, R. R., Long, J. Z., White, J. P., Svensson, K. J., Lou, J., Lokurkar, I., et al. (2014). Meteorin-like is a hormone that regulates immune-adipose interactions to increase beige fat thermogenesis. Cell 157, 1279–1291. doi: 10.1016/j.cell.2014.03.065
Reginster, J.-Y., Beaudart, C., Buckinx, F., and Bruyère, O. (2015). Osteoporosis and sarcopenia: two diseases or one?. Curr. Opin. Clin. Nutr. Metab. Care 19, 31–36. doi: 10.1097/MCO.0000000000000230
Relaix, F., Weng, X., Marazzi, G., Yang, E., Copeland, N., Jenkins, N., et al. (1996). Pw1, a novel zinc finger gene implicated in the myogenic and neuronal lineages. Dev. Biol. 177, 383–396. doi: 10.1006/dbio.1996.0172
Rhim, C., Cheng, C. S., Kraus, W. E., and Truskey, G. A. (2010). Effect of microRNA modulation on bioartificial muscle function. Tiss. Eng. 16, 3589–3597. doi: 10.1089/ten.tea.2009.0601
Rhim, C., Lowell, D. A., Reedy, M. C., Slentz, D. H., Zhang, S. J., Kraus, W. E., et al. (2007). Morphology and ultrastructure of differentiating three-dimensional mammalian skeletal muscle in a collagen gel. Musc. Nerve 36, 71–80. doi: 10.1002/mus.20788
Riechman, S. E., Balasekaran, G., Roth, S. M., and Ferrell, R. E. (2004). Association of interleukin-15 protein and interleukin-15 receptor genetic variation with resistance exercise training responses. J. Appl. Physiol. 97, 2214–2219. doi: 10.1152/japplphysiol.00491.2004
Romitti, P. A., Zhu, Y., Puzhankara, S., James, K. A., Nabukera, S. K., Zamba, G. K. D., et al. (2015). Prevalence of Duchenne and Becker muscular dystrophies in the United States. Pediatrics 135, 513–521. doi: 10.1542/peds.2014-2044
Rose-John, S., Scheller, J., Elson, G., and Jones, S. A. (2006). Interleukin-6 biology is coordinated by membrane-bound and soluble receptors: role in inflammation and cancer. J. Leukocyte Biol. 80, 227–236. doi: 10.1189/jlb.1105674
Rossi, C. A., Flaibani, M., Blaauw, B., Pozzobon, M., Figallo, E., Reggiani, C., et al. (2011). In vivo tissue engineering of functional skeletal muscle by freshly isolated satellite cells embedded in a photopolymerizable hydrogel. FASEB J. 25, 2296–2304. doi: 10.1096/fj.10-174755
Ryall, J. G., Schertzer, J. D., and Lynch, G. S. (2008). Cellular and molecular mechanisms underlying age-related skeletal muscle wasting and weakness. Biogerontology 9, 213–228. doi: 10.1007/s10522-008-9131-0
Rybalko, V. Y., Pham, C. B., Hsieh, P.-L., Hammers, D. W., Merscham-Banda, M., Suggs, L. J., et al. (2015). Controlled delivery of SDF-1α and IGF-1: CXCR4(+) cell recruitment and functional skeletal muscle recovery. Biomat. Sci. 3, 1475–1486. doi: 10.1039/C5BM00233H
Sacco, A., Mourkioti, F., Tran, R., Choi, J., Llewellyn, M., Kraft, P., et al. (2010). Short telomeres and stem cell exhaustion model duchenne muscular dystrophy in mdx/mTR Mice. Cell 143, 1059–1071. doi: 10.1016/j.cell.2010.11.039
Salmons, S., Ashley, Z., Sutherland, H., Russold, M. F., Li, F., and Jarvis, J. C. (2005). Functional electrical stimulation of denervated muscles: basic issues. Artificial Organs 29, 199–202. doi: 10.1111/j.1525-1594.2005.29034.x
Sampaolesi, M., Blot, S., D'Antona, G., Granger, N., Tonlorenzi, R., Innocenzi, A., et al. (2006). Mesoangioblast stem cells ameliorate muscle function in dystrophic dogs. Nature 444, 574–579. doi: 10.1038/nature05282
Sampaolesi, M., Torrente, Y., Innocenzi, A., Tonlorenzi, R., D'Antona, G., Pellegrino, M. A., et al. (2003). Cell therapy of α-sarcoglycan null dystrophic mice through intra-arterial delivery of mesoangioblasts. Science 301, 487–492. doi: 10.1126/science.1082254
Sassoli, C., Zecchi-Orlandini, S., and Formigli, L. (2012). Trophic actions of bone marrow-derived mesenchymal stromal cells for muscle repair/regeneration. Cells 1, 832–850. doi: 10.3390/cells1040832
Seale, P., and Rudnicki, M. A. (2000). A new look at the origin, function, and “stem-cell” status of muscle satellite cells. Dev. Biol. 218, 115–124. doi: 10.1006/dbio.1999.9565
Seldin, M. M., Peterson, J. M., Byerly, M. S., Wei, Z., and Wong, G. W. (2012). Myonectin (CTRP15), a novel myokine that links skeletal muscle to systemic lipid homeostasis. J. Biol. Chem. 287, 11968–11980. doi: 10.1074/jbc.M111.336834
Serena, E., Zatti, S., Reghelin, E., Pasut, A., Cimetta, E., and Elvassore, N. (2010). Soft substrates drive optimal differentiation of human healthy and dystrophic myotubes. Integr. Biol. 2, 193–201. doi: 10.1039/b921401a
Serena, E., Zatti, S., Zoso, A., Lo Verso, F., Tedesco, F. S., Cossu, G., et al. (2016). Skeletal muscle differentiation on a chip shows human donor mesoangioblasts' efficiency in restoring dystrophin in a Duchenne muscular dystrophy model. Stem Cells Transl. Med. 5, 1676–1683. doi: 10.5966/sctm.2015-0053
Serrano, A. L., Baeza-Raja, B., Perdiguero, E., Jard,í, M., and Muñoz-Cánoves, P. (2008). Interleukin-6 is an essential regulator of satellite cell-mediated skeletal muscle hypertrophy. Cell Metab. 7, 33–44. doi: 10.1016/j.cmet.2007.11.011
Serrano, A. L., Mann, C. J., Vidal, B., Ardite, E., Perdiguero, E., and Muñoz-Cánoves, P. (2011). Cellular and molecular mechanisms regulating fibrosis in skeletal muscle repair and disease. Curr. Top Dev. Biol. 2096, 167–201. doi: 10.1016/B978-0-12-385940-2.00007-3
Shadrin, I. Y., Khodabukus, A., and Bursac, N. (2016). Striated muscle function, regeneration, and repair. Cell. Mol. Life Sci. 73, 4175–4202. doi: 10.1007/s00018-016-2285-z
Shansky, J., Chromiak, J., Del Tatto, M., and Vandenburgh, H. (1997). A simplified method for tissue engineering skeletal muscle organoids in vitro. In Vitro Cell. Devel. Biol. Animal 33, 659–661. doi: 10.1007/s11626-997-0118-y
Shapiro, L., and Cohen, S. (1997). Novel alginate sponges for cell culture and transplantation. Biomaterials 18, 583–590. doi: 10.1016/S0142-9612(96)00181-0
Sicari, B. M., Rubin, J. P., Dearth, C. L., Wolf, M. T., Ambrosio, F., Boninger, M., et al. (2014). An acellular biologic scaffold promotes skeletal muscle formation in mice and humans with volumetric muscle loss. Sci. Transl. Med. 6, 234ra58–234ra58. doi: 10.1126/scitranslmed.3008085
Smith, A. S., Passey, S., Greensmith, L., Mudera, V., and Lewis, M. P. (2012). Characterization and optimization of a simple, repeatable system for the long term in vitro culture of aligned myotubes in 3D. J. Cell. Biochem. 113, 1044–1053. doi: 10.1002/jcb.23437
Smith, A. S. T., Davis, J., Lee, G., Mack, D. L., and Kim, D. H. (2016). Muscular dystrophy in a dish: engineered human skeletal muscle mimetics for disease modeling and drug discovery. Drug Discovery Today 21, 1387–1398. doi: 10.1016/j.drudis.2016.04.013
Songsorn, P., Ruffino, J., and Vollaard, N. B. (2016). No effect of acute and chronic supramaximal exercise on circulating levels of the myokine SPARC. Eur. J. Sport Sci. 17, 447–452. doi: 10.1080/17461391.2016.1266392
Spangenburg, E. E., and Booth, F. W. (2006). Leukemia inhibitory factor restores the hypertrophic response to increased loading in the LIF(–/–) mouse. Cytokine 34, 125–130. doi: 10.1016/j.cyto.2006.05.001
Stark, A. E. (2015). Determinants of the incidence of Duchenne muscular dystrophy. Ann. Transl. Med. 3:287. doi: 10.3978/j.issn.2305-5839.2015.10.45
Stern, M. M., Myers, R. L., Hammam, N., Stern, K. A., Eberli, D., Kritchevsky, S. B., et al. (2009). The influence of extracellular matrix derived from skeletal muscle tissue on the proliferation and differentiation of myogenic progenitor cells ex vivo. Biomaterials 30, 2393–2399. doi: 10.1016/j.biomaterials.2008.12.069
Stern-Straeter, J., Bacha, A. D., Stangenberg, L., Foerster, V. T., Horch, R. E., Stark, G. B., et al. (2005). Impact of electrical stimulation on three-dimensional myoblast cultures - a real-time RT-PCR study. J. Cell. Mol. Med. 9, 883–892. doi: 10.1111/j.1582-4934.2005.tb00386.x
Subbotina, E., Sierra, A., Zhu, Z., Gao, Z., Koganti, S. R., Reyes, S., et al. (2015). Musclin is an activity-stimulated myokine that enhances physical endurance. Proc. Natl. Acad. Sci. U.S.A. 112, 16042–16047. doi: 10.1073/pnas.1514250112
Tagliaferri, C., Wittrant, Y., Davicco, M. J., Walrand, S., and Coxam, V. (2015). Muscle and bone, two interconnected tissues. Ageing Res. Rev. 21, 55–70. doi: 10.1016/j.arr.2015.03.002
Tanaka, A., Woltjen, K., Miyake, K., Hotta, A., Ikeya, M., Yamamoto, T., et al. (2013). Efficient and reproducible myogenic differentiation from human ips cells: prospects for modeling miyoshi myopathy in vitro. PLoS ONE 8. doi: 10.1371/annotation/63972dc9-3a31-43d0-ad52-bc46fd948c03
Tang, J., Wang, J., Guo, L., Kong, X., Yang, J., Zheng, F., et al. (2010). Mesenchymal stem cells modified with stromal cell-derived factor 1α improve cardiac remodeling via paracrine activation of hepatocyte growth factor in a rat model of myocardial infarction. Mol. Cells 29, 9–19. doi: 10.1007/s10059-010-0001-7
Tatsumi, R. (2010). Mechano-biology of skeletal muscle hypertrophy and regeneration: possible mechanism of stretch-induced activation of resident myogenic stem cells. Animal Sci. J. 81, 11–20. doi: 10.1111/j.1740-0929.2009.00712.x
Tatsumi, R., Sheehan, S. M., Iwasaki, H., Hattori, A., and Allen, R. E. (2001). Mechanical stretch induces activation of skeletal muscle satellite cells in vitro. Exp. Cell Res. 267, 107–114. doi: 10.1006/excr.2001.5252
Tedesco, F. S., and Cossu, G. (2012). Stem cell therapies for muscle disorders. Curr. Opinion Neurol. 25, 597–603. doi: 10.1097/WCO.0b013e328357f288
Tedesco, F. S., Gerli, M. F., Perani, L., Benedetti, S., Ungaro, F., Cassano, M., et al. (2012). Transplantation of genetically corrected human ipsc-derived progenitors in mice with limb-girdle muscular dystrophy. Sci. Transl. Med. 4, 140ra89–140ra89. doi: 10.1126/scitranslmed.3003541
Theadom, A., Rodrigues, M., Roxburgh, R., Balalla, S., Higgins, C., Bhattacharjee, R., et al. (2014). Prevalence of muscular dystrophies: a systematic literature review. Neuroepidemiology 43, 259–268. doi: 10.1159/000369343
Thorley, M., Duguez, S., Mazza, E. M. C., Valsoni, S., Bigot, A., Mamchaoui, K., et al. (2016). Skeletal muscle characteristics are preserved in hTERT/cdk4 human myogenic cell lines. Skeletal Muscle 6:43. doi: 10.1186/s13395-016-0115-5
Tonlorenzi, R., Dellavalle, A., Schnapp, E., Cossu, G., and Sampaolesi, M. (2007). “Isolation and characterization of Mesoangioblasts from Mouse, Dog, and Human tissues,” in Current Protocols in Stem Cell Biology (Hoboken, NJ: John Wiley and Sons, Inc.), Unit 2B.1.
van der Schaft, D. W., van Spreeuwel, A. C., Boonen, K. J., Langelaan, M. L., Bouten, C. V., and Baaijens, F. P. (2013). Engineering skeletal muscle tissues from murine myoblast progenitor cells and application of electrical stimulation. J. Vis. Exp. 73:e4267. doi: 10.3791/4267
Vandenburgh, H., Shansky, J., Benesch-Lee, F., Barbata, V., Reid, J., Thorrez, L., et al. (2008). Drug-screening platform based on the contractility of tissue-engineered muscle. Muscle Nerve 37, 438–447. doi: 10.1002/mus.20931
Vandenburgh, H. H., and Karlisch, P. (1989). Longitudinal growth of skeletal myotubes in vitro in a new horizontal mechanical cell stimulator. In Vitro Cell. Devel. Biol. J. Tissue Cult. Assoc. 25, 607–616.
Vandenburgh, H. H., Karlisch, P., and Farr, L. (1988). Maintenance of highly contractile tissue-cultured avian skeletal myotubes in collagen gel. In Vitro Cell. Dev. Biol. 24, 166–174.
Vangsness, C. T., Kurzweil, P. R., and Lieberman, J. R. (2004). Restoring articular cartilage in the knee. Am. J. Orthopedics 33, 29–34.
von Roth, P., Duda, G. N., Radojewski, P., Preininger, B., Strohschein, K., Röhner, E., et al. (2012). Intra-arterial msc transplantation restores functional capacity after skeletal muscle trauma. The Open Orthopaedics J. 6, 352–356. doi: 10.2174/1874325001206010352
Wang, L., Cao, L., Shansky, J., Wang, Z., Mooney, D., and Vandenburgh, H. (2014). Minimally invasive approach to the repair of injured skeletal muscle with a shape-memory scaffold. Mol. Ther. 22, 1441–1449. doi: 10.1038/mt.2014.78
Wang, W., Fan, M., Zhang, L., Liu, S. H., Sun, L., and Wang, C. Y. (2009). Compatibility of hyaluronic acid hydrogel and skeletal muscle myoblasts. Biomed. Materials 4:25011. doi: 10.1088/1748-6041/4/2/025011
Webster, C., and Blau, H. M. (1990). Accelerated age-related decline in replicative life-span of Duchenne muscular dystrophy myoblasts: Implications for cell and gene therapy. Somatic Cell Mol. Genet. 16, 557–565. doi: 10.1007/BF01233096
Wehrle, U., Düsterhöft, S., and Pette, D. (1994). Effects of chronic electrical stimulation on myosin heavy chain expression in satellite cell cultures derived from rat muscles of different fiber-type composition. Differentiation 58, 37–46. doi: 10.1046/j.1432-0436.1994.5810037.x
Wobma, H., and Vunjak-Novakovic, G. (2016). Tissue engineering and regenerative medicine 2015: a year in review. Tissue Eng. 20, 1–16. doi: 10.1089/ten.TEB.2015.0535
Wolf, M. T., Daly, K. A., Reing, J. E., and Badylak, S. F. (2012). Biologic scaffold composed of skeletal muscle extracellular matrix. Biomaterials 33, 2916–2925. doi: 10.1016/j.biomaterials.2011.12.055
Wynn, T. (2008). Cellular and molecular mechanisms of fibrosis. J. Pathol. 214, 199–210. doi: 10.1002/path.2277
Yaffe, D., and Saxel, O. (1977). Serial passaging and differentiation of myogenic cells isolated from dystrophic mouse muscle. Nature 270, 725–727. doi: 10.1038/270725a0
Yamanaka, S., and Takahashi, K. (2006). Induction of pluripotent stem cells from mouse embryonic and adult fibroblast cultures by defined factors. Cell 126, 663–676. doi: 10.1016/j.cell.2006.07.024
Yang, H. S., Ieronimakis, N., Tsui, J. H., Kim, H. N., Suh, K.-Y., Reyes, M., et al. (2014). Nanopatterned muscle cell patches for enhanced myogenesis and dystrophin expression in a mouse model of muscular dystrophy. Biomaterials 35, 1478–1486. doi: 10.1016/j.biomaterials.2013.10.067
Yin, H., Price, F., and Rudnicki, M. A. (2013). Satellite cells and the muscle stem cell niche. Physiol. Rev. 93, 23–67. doi: 10.1152/physrev.00043.2011
Yin, Q., Jin, P., Liu, X., Wei, H., Lin, X., Chi, C., et al. (2011). SDF-1α inhibits hypoxia and serum deprivation-induced apoptosis in mesenchymal stem cells through PI3K/Akt and ERK1/2 signaling pathways. Mol. Biol. Rep. 38, 9–16. doi: 10.1007/s11033-010-0071-9
Yiu, E. M., and Kornberg, A. J. (2015). Duchenne muscular dystrophy. J. Paediatr. Child Health 51, 759–764. doi: 10.1111/jpc.12868
Zammit, P. S., Golding, J. P., Nagata, Y., Hudon, V., Partridge, T. A., and Beauchamp, J. R. (2004). Muscle satellite cells adopt divergent fates: a mechanism for self-renewal? J. Cell Biol. 166, 347–357. doi: 10.1083/jcb.200312007
Zammit, P. S., Partridge, T. A., and Yablonka-Reuveni, Z. (2006). The skeletal muscle satellite cell: the stem cell that came in from the cold. J. Histochem. Cytochem. 54, 1177–1191. doi: 10.1369/jhc.6R6995.2006
Zeng, L., Akasaki, Y., Sato, K., Ouchi, N., Izumiya, Y., and Walsh, K. (2010). Insulin-like 6 is induced by muscle injury and functions as a regenerative factor. J. Biol. Chem. 285, 36060–36069. doi: 10.1074/jbc.M110.160879
Zhang, C., Li, Y., Wu, Y., Wang, L., Wang, X., and Du, J. (2013). Interleukin-6/signal transducer and activator of transcription 3 (STAT3) pathway is essential for macrophage infiltration and myoblast proliferation during muscle regeneration. J. Biol. Chem. 288, 1489–1499. doi: 10.1074/jbc.M112.419788
Zhang, M., Mal, N., Kiedrowski, M., Chacko, M., Askari, A. T., Popovic, Z. B., et al. (2007). SDF-1 expression by mesenchymal stem cells results in trophic support of cardiac myocytes after myocardial infarction. FASEB J. 21, 3197–3207. doi: 10.1096/fj.06-6558com
Keywords: skeletal muscle tissue engineering, stimulation strategies, bioreactors, myokines, skeletal muscle disease models, biomaterials, myogenesis
Citation: Maleiner B, Tomasch J, Heher P, Spadiut O, Rünzler D and Fuchs C (2018) The Importance of Biophysical and Biochemical Stimuli in Dynamic Skeletal Muscle Models. Front. Physiol. 9:1130. doi: 10.3389/fphys.2018.01130
Received: 18 December 2017; Accepted: 30 July 2018;
Published: 22 August 2018.
Edited by:
Alberto Rainer, Università Campus Bio-Medico, ItalyReviewed by:
Cesare Gargioli, Università degli Studi di Roma Tor Vergata, ItalyCopyright © 2018 Maleiner, Tomasch, Heher, Spadiut, Rünzler and Fuchs. This is an open-access article distributed under the terms of the Creative Commons Attribution License (CC BY). The use, distribution or reproduction in other forums is permitted, provided the original author(s) and the copyright owner(s) are credited and that the original publication in this journal is cited, in accordance with accepted academic practice. No use, distribution or reproduction is permitted which does not comply with these terms.
*Correspondence: Christiane Fuchs, Y2Z1Y2hzMUBtZ2guaGFydmFyZC5lZHU=
†Present Address: Christiane Fuchs, Wellman Center for Photomedicine, Massachusetts General Hospital, Boston, MA, United States
Disclaimer: All claims expressed in this article are solely those of the authors and do not necessarily represent those of their affiliated organizations, or those of the publisher, the editors and the reviewers. Any product that may be evaluated in this article or claim that may be made by its manufacturer is not guaranteed or endorsed by the publisher.
Research integrity at Frontiers
Learn more about the work of our research integrity team to safeguard the quality of each article we publish.