- 1Fundación Oceanografic de la Comunidad Valenciana, Gran Vía Marques del Turia, Valencia, Spain
- 2Department of Life Sciences, Texas A&M University–Corpus Christi, Corpus Christi, TX, United States
- 3Woods Hole Oceanographic Institution, Woods Hole, MA, United States
- 4Chicago Zoological Society’s Sarasota Dolphin Research Program, Mote Marine Laboratory, Sarasota, FL, United States
- 5Duke University Marine Lab, Beaufort, NC, United States
- 6Dolphin Quest, Waikoloa, HI, United States
- 7Wildlife Consulting Service, Currumbin, QLD, Australia
- 8Aarhus Institute of Advanced Studies, Aarhus University, Aarhus, Denmark
Diving mammals have evolved a suite of physiological adaptations to manage respiratory gases during extended breath-hold dives. To test the hypothesis that offshore bottlenose dolphins have evolved physiological adaptations to improve their ability for extended deep dives and as protection for lung barotrauma, we investigated the lung function and respiratory physiology of four wild common bottlenose dolphins (Tursiops truncatus) near the island of Bermuda. We measured blood hematocrit (Hct, %), resting metabolic rate (RMR, l O2 ⋅ min-1), tidal volume (VT, l), respiratory frequency (fR, breaths ⋅ min-1), respiratory flow (l ⋅ min-1), and dynamic lung compliance (CL, l ⋅ cmH2O-1) in air and in water, and compared measurements with published results from coastal, shallow-diving dolphins. We found that offshore dolphins had greater Hct (56 ± 2%) compared to shallow-diving bottlenose dolphins (range: 30–49%), thus resulting in a greater O2 storage capacity and longer aerobic diving duration. Contrary to our hypothesis, the specific CL (sCL, 0.30 ± 0.12 cmH2O-1) was not different between populations. Neither the mass-specific RMR (3.0 ± 1.7 ml O2 ⋅ min-1 ⋅ kg-1) nor VT (23.0 ± 3.7 ml ⋅ kg-1) were different from coastal ecotype bottlenose dolphins, both in the wild and under managed care, suggesting that deep-diving dolphins do not have metabolic or respiratory adaptations that differ from the shallow-diving ecotypes. The lack of respiratory adaptations for deep diving further support the recently developed hypothesis that gas management in cetaceans is not entirely passive but governed by alteration in the ventilation-perfusion matching, which allows for selective gas exchange to protect against diving related problems such as decompression sickness.
Introduction
Marine mammals live a life of dual constraints: On one hand, they need to find and exploit prey in varying densities underwater, but on the other hand as air breathing animals they need to return to the surface to replenish O2 stores and remove CO2 produced by aerobic metabolism. The total time spent on a single dive influences both the types of prey that can be exploited as well as the efficiency with which food can be obtained, and this dive performance ultimately depends on the amount of O2 available, the size of the O2 stores, and how quickly they are utilized, the metabolic cost.
To increase dive capacity, many marine mammals have therefore evolved to lower energy consumption over the dive and to increase available O2 stores. Oxygen stores are typically expanded by having a greater proportion of blood relative to body mass, by increasing the amount of red blood cells per volume of blood (the hematocrit), and by increasing the amount of myoglobin (Ponganis, 2015). In addition, it has been proposed that deep divers have relatively larger muscle mass, large muscle fibers, and low mitochondrial volume (Kooyman and Ponganis, 1998; Pabst et al., 2016). A greater proportion of blood volume and muscle mass would enhance available O2, and the latter would also help to reduce the overall rate of O2 consumption as the basal metabolic rate of muscle is lower than many other tissues (Pabst et al., 2016). The lung volume, on the other hand, does not seem to be increased, and it has been proposed that deep diving species have lungs that are relatively small as compared with shallow diving species (Scholander, 1940; Piscitelli et al., 2010). It is suggested that the reduced lung volume minimizes gas exchange at depth and minimizes uptake of N2 and thereby the risk of diving-related problems such as decompression sickness or N2 narcosis (Scholander, 1940; Kooyman, 1973; Bostrom et al., 2008).
In addition to reduced lung volume in deep divers, it has been suggested that marine mammals have respiratory adaptations that help prevent excessive uptake of N2. The respiratory adaptations include a highly compliant alveolar space and stiff airways that would allow the alveolar gas to empty into the conducting airways as the pressure increases during descent (Scholander, 1940). In addition, the minimum air volume (MAV), the amount of air left in the relaxed lung, would be minimal to avoid barotrauma (Fahlman et al., 2017b). In a recent study, it was shown that the static lung compliance in pinnipeds that had been housed under human care was lower as compared with wild animals undergoing rehabilitation (Fahlman et al., 2014). It was hypothesized that these differences could be evidence that lung conditioning, where repeated collapse and recruitment of the alveoli, increased the compliance (Fahlman et al., 2014). However, the study subjects in the previous study were of different ages, species, and health and we are unaware of any study that has specifically tested the hypothesis that dive behavior alters lung compliance.
In the current study, we wanted to test the hypothesis that offshore bottlenose dolphins have lower metabolic rate, and tidal volume (VT), and greater lung compliance (CL) as traits that correlate with deep diving. To test these hypotheses, we measured the resting metabolic rate (RMR), VT, respiratory frequency (fR), respiratory flow, and dynamic CL in 4 common bottlenose dolphins near the island of Bermuda. The dolphins were of the offshore ecotype (Wells and Scott, 2018), a variant occupying deeper, offshore waters, and differing from the more commonly studied coastal ecotype in distribution and morphology (Mead and Potter, 1995). Previous research found that offshore dolphins near Bermuda exploited prey at depths of several hundred meters in dives lasting more than 5 min, well beyond the depths and dive durations previously recorded for coastal bottlenose dolphins (Klatsky et al., 2007). Our aim was to compare these measurements with data from coastal ecotype bottlenose dolphins (Fahlman et al., 2018a) to test the hypothesis that deeper-diving offshore ecotype bottlenose dolphins have a lower mass-specific metabolic rate, a lower VT, and a greater CL as part of traits to improve the ability for extended deep dives and as protection against lung barotrauma.
Materials and Methods
Animals
A total of 3 male and 1 female common bottlenose dolphins (Tursiops truncatus, Table 1) were studied. The animals were captured by a break-away hoop netting technique (Asper, 1975), and briefly restrained for a health assessment, tagging, and sampling. Once in the net, swimmers managed the animal and moved it onto a buoyant foam mat. The animal was moved onto a sling and then brought onto the sampling boat using a pulley system. Once on the boat, the dolphin was weighed (± 0.2 kg, Ohaus 3000 Series industrial floor scale) and underwent a basic health examination (e.g., Wells et al., 2004). During the health assessment, a custom-made breath-by-breath respirometry system, including a custom-built pneumotachometer and fast-response O2 and CO2 analyzers allowed assessment of lung function and resting rates of O2 consumption (O2) and CO2 production (CO2). All spirometry trials (breath-by-breath lung function and end-expired O2 and CO2) were measured with the dolphin lying on a shaded, padded mat or being lightly restrained while partially submerged in water and breathing voluntarily. Spirometry trials while resting on the foam mat ranged from 35 to 45 min, and while restrained in water for up to 10 min.
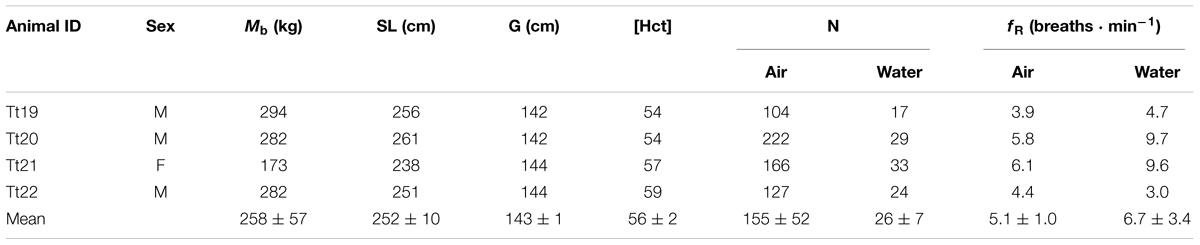
TABLE 1. Animal identification, sex (M-male, F-female), body mass (Mb), straight length (SL), maximum girth (G), blood hematocrit concentration (Hct, %), number of breaths collected (N), and breathing frequency (fR), while in air or in water.
All works were approved by the IACUC at Texas A&M University Corpus Christi (TAMUCC-IACUC AUP#04-11) and by a research permit issued by the Bermuda Government, Department of Environment and Natural Resources (Research permit number SP160401r).
Blood Hematocrit (Hct)
Blood samples were obtained from the venous rete on the ventral side of the tail fluke for measuring the packed cell volumes, or blood hematocrit (Hct).
Respiratory Flows (Lung Function)
The procedures and equipment used were identical to those used in previous studies on the same species under human care, or during similar conditions on restrained wild dolphins (Fahlman et al., 2015, 2018a), briefly summarized below. Respiratory flows were measured using a custom-made Fleisch type pneumotachometer (Mellow Design, Valencia, Spain), which housed a low-resistance laminar flow matrix (Item # Z9A887-2, Merriam Process Technologies, Cleveland, OH, United States). A diffuser was inserted to improve the flow characteristics during expiration, which reduced the need for different calibration factors for expiratory and inhalatory flow. A differential pressure transducer (Spirometer Pod, ML 311, ADInstruments, Colorado Springs, CO, United States) was connected to the pneumotachometer with two, 310 cm lengths of 2 mm i.d., firm walled, flexible tubing. The differential pressure transducer was connected to a data acquisition system (Powerlab 8/35, ADInstruments, Colorado Springs, CO, United States), and the data were captured at 400 Hz and displayed on a computer running LabChart (v. 8.1, ADInstruments, Colorado Springs, CO, United States). The differential pressure was used to determine flow and was calibrated using a 7.0 l calibration syringe (Series 4900, Hans Rudolph, Inc., Shawnee, KS, United States). The signal was integrated and the flow determined as detailed previously (Fahlman et al., 2015, 2018a).
Lung Compliance
We estimated the dynamic lung compliance (CL, l ⋅ cmH2O-1) as previously described by dividing the VT by the change in transpulmonary pressure (PL = Pao – Peso; Pao = airway opening pressure, Peso = esophageal pressure), measured at zero flow of the expiratory phase and inspiratory phases (a and b, respectively, in Figure 2C in Fahlman et al., 2015). To enable comparisons between animals of different body sizes, we computed the mass-specific lung compliance (sCL, cmH2O-1) by dividing CL by the MAV, estimated to be 7% of the estimated total lung capacity (TLC; Fahlman et al., 2011, 2015).
To learn more about the respiratory adaptations in marine mammals, we measured trans-pulmonary and trans-thoracic static compliance of a stranded and deceased common (Delphinus delphis) and Atlantic white-sided dolphin (Lagenorhynchus acutus) obtained from the Marine Mammal Rescue and Research Division of the International Fund for Animal Welfare (IFAW). The specimen was intubated and the transthoracic pressure of the intact cadaver (PT = Pao - Pamb, Pamb = ambient pressure) or the PL of the excised lungs (PL = Pao - Pamb) as previously detailed (Fahlman et al., 2011, 2014). These former value represent the compliance of the entire respiratory system, including lung and chest, and the latter the compliance of the lungs only. If the chest has high compliance, PL = PT.
Respiratory Gas Composition
The concentrations of expired O2 and CO2 were subsampled via a port in the pneumotachometer and passed through a 310 cm length of 2 mm i.d., firm walled, flexible tubing and a 30 cm length of 1.5 mm i.d., Nafion tubing, to a fast-response O2 and CO2 analyzer (Gemini respiratory monitor, CWE Inc.) at a flow rate of 200 ml ⋅ min-1. The gas analyzer was connected to the data acquisition system and sampled at 400 Hz. The gas analyzer was calibrated before and after the experiment using a commercial mixture of 5% O2, 5% CO2, and 90% N2 (Product No. 17L-340, GASCO, Oldsmar, FL, United States). Ambient air and pure O2 were used to check the calibration before and after each experimental trial. Mean (± SEM) air temperature and humidity during trials were 31.3 ± 0.3°C (n = 4, range 31–32°C) and 71.8 ± 3.6% (65–78%). The average ( ± SE, n = 4) surface water temperature around the sampling boat was 27.3 ± 0.3°C (range: 27–28°C).
Metabolic Rates
The metabolic rates were estimated as detailed previously (Fahlman et al., 2015, 2018a) and briefly summarized here. The respiratory gas signals were phase-corrected for CO2 and O2, to match the respirations, and the expiratory flow-rate and expired O2 and CO2 content were multiplied to calculate the instantaneous O2 consumption rate (O2) and CO2 production rate (CO2). The instantaneous O2 and CO2 were integrated over each breath to yield the total volume of O2 and CO2 exchanged during each breath. The volumes were summed for each trial period and divided by the duration of the trial to provide an estimate of the O2 and CO2 rates for that time period.
We compared metabolic rate in water and on land to determine if the effect of gravity would affect the measurements. Measurements on land are easier to perform during health assessments, but in fully aquatic species, the effect of gravity on respiration may increase the metabolic cost. However, we found no evidence for this in the current study. Still, comparisons with the coastal ecotype were made with the in-water measurements.
Data Processing and Statistical Analysis
All gas volumes were converted to standard temperature pressure dry (STPD, Quanjer et al., 1993). Exhaled air was assumed saturated at 37°C, inhaled air volume was corrected for ambient temperature and relative humidity. Metabolic data are reported as the average O2 consumption rate for an entire trial. With the limited sample size, we made comparisons between positions (air vs. water) or respiratory direction (inspiration vs. expiration) using paired t-tests. In this study, P-values ≤ 0.05 were considered as significant and P ≤ 0.1 were considered potentially suggestive of a trend. Data are presented as the mean ± standard deviation (SD), unless otherwise stated.
Results
Data from 722 spontaneous breaths were collected for 4 bottlenose dolphins (Table 1) sampled near the island of Bermuda.
Blood Hematocrit (Hct)
The blood Hct ranged from 54 to 59% between animals and are reported in Table 1.
Respiratory Flows and Timing
The average fR during trials was 5.9 ± 2.2 breaths ⋅ min-1 (Table 1), and did not differ whether held in or out of water (paired t-test, t-value: 1.37, df = 3, P = 0.26).
The average maximum spontaneous inspiratory flow (insp) was 16.1 ± 3.6 l ⋅ s-1, and was not different for animals in air vs. in water (range: 2.5–34.2 l ⋅ s-1, paired t-test, t-value: 0.79, df = 3, P = 0.49). Similarly, the maximum spontaneous expiratory flow (exp) did not differ in water vs. in air (21.0 ± 5.2 l ⋅ sec-1, range: 7.5–39.4 l ⋅ s-1, paired t-test, t-value: 1.5, df = 3, P = 0.24). exp was significantly higher as compared with insp (paired t-test, t-value: 3.2, df = 3, P = 0.04). The average expiratory (e.g., 514 ± 109 s vs. 458 ± 110 s; paired t-test, t-value: 4.66, P = 0.02), average inspiratory (t-value: 3.47, P = 0.04), and total breath durations (t-value: 5.17, P = 0.01) were shorter in water as compared with air (Table 2). The average expiratory or inspiratory breath duration did not differ either in air or water (e.g., 514 ± 109 s vs. 653 ± 209 s; air: t-value: 2.4, df = 3, P = 0.1, water: t-value: 1.2, df = 3, P = 0.31). The expiratory (t-value: 1,25, df = 3, P = 0.30) or inspiratory VT (t-value: 0.89, df = 3, P = 0.43) did not differ in air vs. water, and there were no differences in inspiratory VT (VTinsp = 6.0 ± 0.8 l) or expiratory VT (VTexp = 5.5 ± 0.9 l, t-value: 1.28, df = 3, P = 0.29). The average VTinsp was 27 ± 1% of the estimated total lung capacity (TLCest, 8).
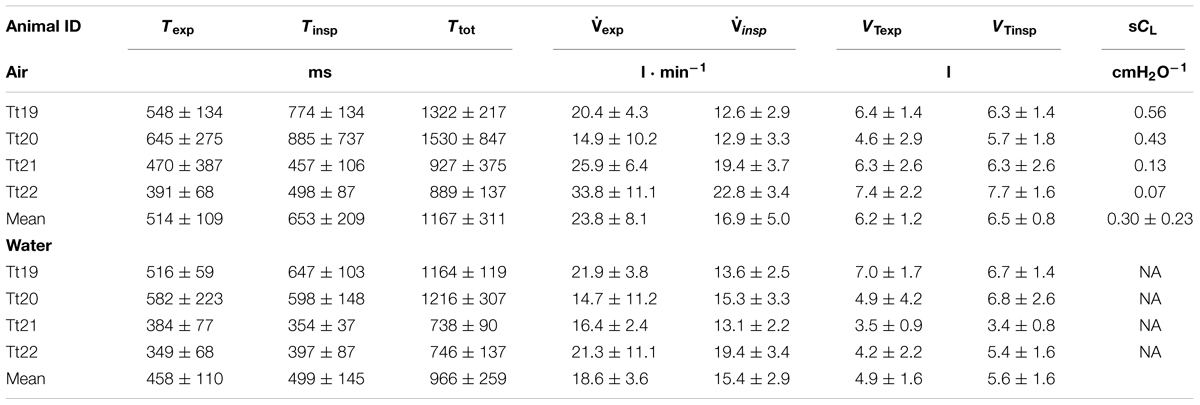
TABLE 2. Animal identification, expiratory (Texp), inspiratory (Tinsp) and total breath duration (Ttot), expiratory and inspiratory flow (exp and insp) and tidal volume (VTexp and VTinsp), specific dynamic lung compliance (sCL).
Respiratory Compliance
The average ( ± SEM) CL and sCL were 0.50 ± 0.22 l ⋅ cmH2O-1 (range: 0.12–0.98 l ⋅ cmH2O-1) and 0.30 ± 0.12 cmH2O-1 (range: 0.07–0.56 cmH2O-1), respectively. We used previously published pressure-volume (compliance) data for the excised lungs (Fahlman et al., 2011), and unpublished chest compliance data from the same individuals to compare differences in lung and chest compliance in carcasses of a common (Delphinus delphis) and an Atlantic white-sided dolphin (Lagenorhynchus acutus, Figure 1). These data show that the chest is not infinitely compliant and provide resistance to inhalation.
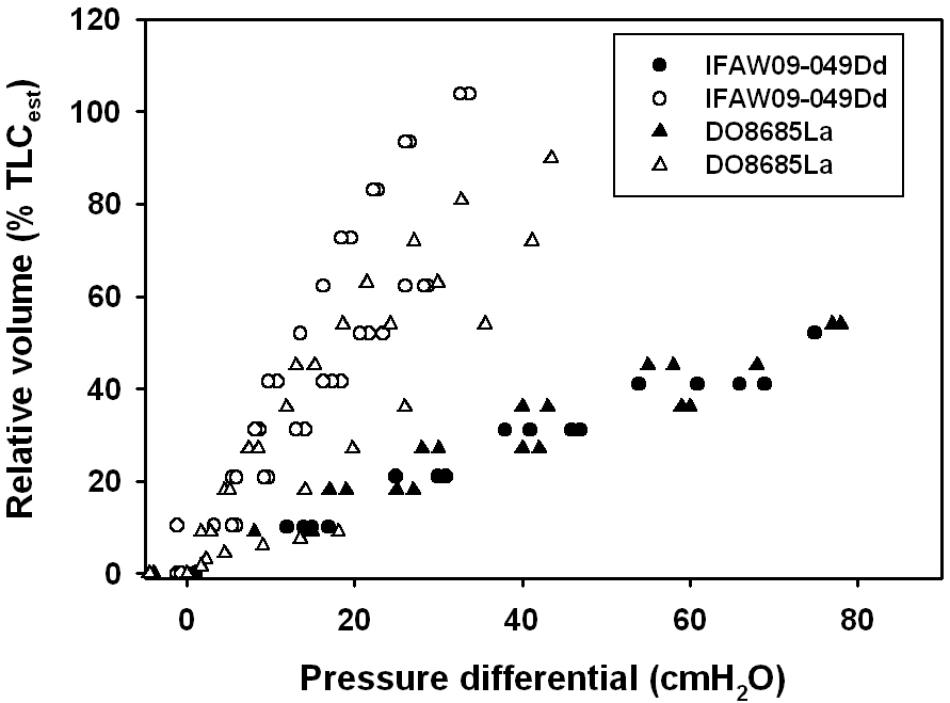
FIGURE 1. Lung volume expressed as a percentage of estimated total lung capacity (TLCest, Kooyman, 1973; Fahlman et al., 2011) vs. trans-thoracic (whole animals, filled symbols) or trans-pulmonary (excised, open symbols) pressure in a common (Dd: Delphinus delphis) and Atlantic white-sided dolphin (La: Lagenorhynchus acutus). Measurements shown are during deflation of the lung.
Gas Exchange
The end-expiratory O2 was significantly higher in water as compared with air (t-value: 3.6, P = 0.04), and there was a suggestion of a trend for a lower end-expiratory CO2 in water as compared to on land (t-value: 2.9, P = 0.06, Table 3).
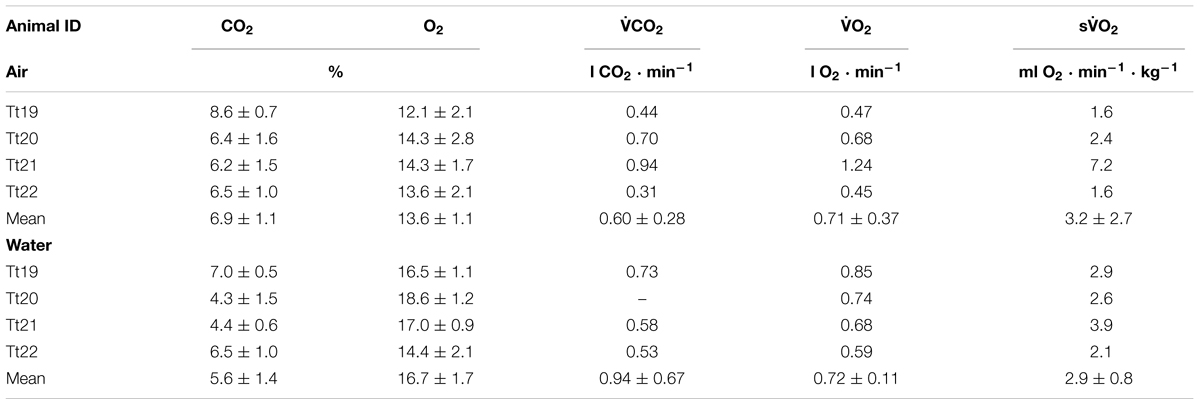
TABLE 3. Animal ID, end-expired CO2 and O2, CO2 production rate (CO2), O2 consumption rate (O2), and mass-specific O2 (sO2) while in air or in water.
Metabolic Rates
The resting O2 was estimated for periods during which the dolphin remained calm that were at least 3 min and up to 15 min in duration (Table 3). Neither the O2 (t-value: 0.02, df = 3, P = 0.98), nor the CO2 (t-value: 0.24, df = 2, P = 0.83) differed on land vs. in water. The mass-specific metabolic rate (sO2) ranged from 1.6 to 7.2 ml O2 ⋅ min-1 ⋅ kg-1. A comparison with previous data from adult males showed that the sO2 in the offshore ecotype (2.53 ± 0.40 ml O2 ⋅ min-1 ⋅ kg-1, n = 3) did not differ with measurements in the near shore dolphins [2.69 ± 1.20 ml O2 ⋅ min-1 ⋅ kg-1, n = 9, (Fahlman et al., 2018a), t-test, t-value: 0.20, P = 0.85].
Discussion
We estimated resting metabolic rate and lung function in four offshore bottlenose dolphins. This limited data set provides an important comparison with published values for animals under human care and free-ranging coastal bottlenose dolphins. We hypothesized that the deep-diving offshore population would have a lower sO2, sVT, and a higher sCL as physiological adaptations required for deep-diving.
Physiological Adaptations for Longer Foraging Dives
In the current study, the measured resting sO2 was within the same range as values previously published for bottlenose dolphins of the coastal ecotype (Table 3; Yazdi et al., 1999; Yeates and Houser, 2008; Noren et al., 2013; Fahlman et al., 2015, 2018a). The measured resting metabolic in free ranging coastal dolphins made in our previous study allowed intra-specific comparison of animals under similar conditions (Fahlman et al., 2018a). The O2 consumption rate of offshore dolphin males (range: 1.6–2.9 ml O2 ⋅ min-1 ⋅ kg-1) and the single female (3.9 ml O2 ⋅ min-1 ⋅ kg-1) while not different were at the lower end of values previously reported from both managed care and shallow-diving dolphins [0.8–9.5 ml O2 ⋅ min-1 ⋅ kg-1 (Yazdi et al., 1999; Yeates and Houser, 2008; Noren et al., 2013; Fahlman et al., 2015, 2018a)]. A lower metabolic rate would support the hypothesis that deep divers are built with a greater proportional muscle mass with large fibers and lower mitochondrial density (Pabst et al., 2016), which helps to increase the O2 stores and reducing the sO2, thereby increasing aerobic dive durations. However, we were unable to detect a significant difference in resting sO2 in adult males in water, which could be caused by the inability to control for digestive status or that the reduction is smaller than we could measure with our limited sample size. We had limited ability to control the sex, age, and size of the animal in the current study, but the goal was to select non-calf animals of medium size. We specifically avoided very young animals, and did not attempt to catch larger animals for safety reasons. Given that we were studying free-ranging animals, we could not determine their digestive status. As these variables affect the metabolic rate, this could have increased the variation of our measurements. Alternatively, the increased dive durations (average: 70 ± 10 s, maximum 539 s) seen in the offshore ecotype (Fahlman et al., 2018b) may be largely possible by increased O2 storage capacity.
We propose that the offshore ecotype has a greater O2 storage capacity to extend dive durations, and the greater blood Hct in the deep diving dolphins (Bermuda: 56 ± 2%) as compared with the shallow diving ecotype (values ranging from 37 to 47% for adult males and females) provide partial evidence of this (Table 1; Ridgway and Johnston, 1966; Hall et al., 2007; Klatsky et al., 2007; Schwacke et al., 2009). However, this greater blood Hct is not sufficient to increase the calculated aerobic dive limit (cADL) to support the longest dives seen in the Bermuda dolphins (Fahlman et al., 2018b). To further increase cADL, we previously proposed that the offshore ecotype have, in addition to reduced RMR and blood Hct, increased blood volume, muscle mass and muscle myoglobin as compared with the coastal ecotype (Pabst et al., 2016). A greater muscle and blood O2 capacity would make them less dependent on pulmonary O2 during deeper dives, which may explain the trend toward a lower mass-specific VT (sVT). The longer and deeper dives performed by the offshore ecotype could allow them to further reduce the metabolic cost of foraging at depth by employing cost-saving swimming strategies that reduce the overall activity and metabolic cost (Williams, 2001; Fahlman et al., 2013; Martín López et al., 2015). Thus, offshore dolphins may have a reduced cost of foraging compared to coastal ecotype that increases their cADL. Comparing activity among these populations and ecotypes, as a proxy for field metabolic rate (acceleration, Fahlman et al., 2008, 2013; Enstipp et al., 2011) could be useful to assess how energy is partitioned in these populations.
Breathing Strategies for Deep Foraging Dives
The measured VT collected in this study agrees well with previous measurements in cetaceans (Fahlman et al., 2017b). The average sVT recorded in the current study was 24.4 ± 2.6 ml ⋅ kg-1 (range: 21.1–32.1 ml ⋅ kg-1), which is within the range of previous estimates for the bottlenose dolphin (13.6–58.8 ml ⋅ kg-1, Irving et al., 1941; Ridgway et al., 1969; Fahlman et al., 2015, 2018a), and a gray whale (12.0–35.3 ml ⋅ kg-1, Wahrenbrock et al., 1974), but slightly lower than the harbor porpoise (39.3–52.6 ml ⋅ kg-1, Reed et al., 2000). The breathing frequency (5.1 ± 1.0 breaths ⋅ min-1 in air; 6.7 ± 3.4 breaths ⋅ min-1 in water), on the other hand, was significantly higher as compared with previous studies (Dolphin Quest: 3.4 ± 1.1 breaths ⋅ min-1, Sarasota: 2.9 ± 1.1 breaths ⋅ min-1, t-value > 2.9 for both, P < 0.05, t-test, Fahlman et al., 2015, 2018a). This variation may reflect differences in breathing strategy between deep- and shallow-diving dolphins. It is also possible that the differences in breathing frequency may indicate stress, where dolphins under managed care participate voluntarily and those in Sarasota have been handled previously.
The breathing strategy in marine mammals, with a low fR, high VT, and extended respiratory pause between inspirations has been hypothesized to help with gas exchange or aid in buoyancy (Mortola and Limoges, 2006; Mortola and Sequin, 2009; Fahlman et al., 2017b). A higher fR may indicate a different breathing strategy in the offshore animals, with lower VT to maintain the same alveolar ventilation. Still, while the average sVT was not significantly different from previous studies of coastal ecotype bottlenose dolphins, the volumes were at the lower end and with high variability, and we may not have been able to detect a difference given our small sample size. Thus, we cannot determine whether offshore dolphins may choose to breathe more frequently with lower VT. A shallower VT would support the suggestion that deep-diving cetaceans dive with a lower lung volume (Miller et al., 2004; Piscitelli et al., 2010), which would help minimize N2 uptake (Bostrom et al., 2008; Fahlman et al., 2009). A lower sVT may indicate a lower TLC as has been suggested as a potential adaptation to reduce N2 uptake and risk of gas emboli in deep-diving species (Scholander, 1940; Miller et al., 2004; Bostrom et al., 2008; Piscitelli et al., 2010). Additional measurements on deep-diving dolphins may confirm this hypothesis, and alternative methods to assess respiratory capacity or diving lung volume using phonospirometry (Sumich, 2001; Sumich and May, 2009; van der Hoop et al., 2014), respiratory sinus arrhythmias, or body acceleration/movement (Fahlman et al., 2017c) may provide alternatives to explore these physiological traits for free-ranging individuals.
Mechanisms to Avoid Lung Barotrauma
Scholander (1940) proposed that the anatomy of the respiratory system in marine mammals, with a compliant alveolar space and stiff airways, would help reduce gas exchange and the risk of decompression sickness. A number of studies have evaluated the respiratory mechanics in marine mammals and concluded that the sCL is greater as compared with terrestrial mammals (Fahlman et al., 2017b). In a previous study, it was found that the sCL in pinnipeds undergoing rehabilitation was higher than in animals raised under human care (Fahlman et al., 2014). One proposed explanation was that lung conditioning, by repeated diving, results in increased sCL to reduce the risk for lung barotrauma. In human apnea divers, increased vital capacity has been reported following long-term training that included repeated expansion and compression of the thoracic space, similar to what happens during a breath-hold dive (Johansson and Schagatay, 2012). Consequently, frequent compression collapse/atelectasis and recruitment may result in more compliant chest or lung parenchyma. We therefore hypothesized that the offshore, deep-diving dolphins should exhibit a higher sCL as compared with the dolphins that had been housed under managed care and with limited opportunity to perform deep dives. Our results indicate that the sCL appears to be significantly higher as compared with terrestrial species, including humans, but there was no difference compared to dolphins that have lived for extended durations in zoological facilities (Fahlman et al., 2015, 2017b).
While sCL may be an important variable to reduce lung barotrauma during diving, a compliant chest/thorax is probably more important. Chest compliance has been measured in pinnipeds, and it was shown that it was considerably more compliant than the lungs (Leith, 1976; Fahlman et al., 2014), and the flexible rib cage allows the lungs to empty to very low lung volumes. In cetaceans, on the other hand, no study has measured the compliance of the thorax in live animals at this point. It has been shown photographically that the chest in dolphins is very compressible (Ridgway et al., 1969). However, compliance measurements on stranded and deceased common (Delphinus delphis) and Atlantic white-sided (Lagenorhynchus acutus) dolphins indicate that unlike the pinnipeds, the chest is considerably stiffer than the lungs (Figure 1). The high sCL, and reinforced airways may help generate the high respiratory flow seen in cetaceans, and the elastic recoil may help aid compression of the lung to low volumes during diving (Kooyman and Cornell, 1973, Kooyman and Cornell, 1981; Fahlman et al., 2011, 2015, 2017b; Moore et al., 2014). While similar measurements should be repeated in live animals, these data provide an understanding about the extent that respiratory compliance has on deep-diving adaptations.
Recent work has resulted in a new hypothesis how cetaceans manage gases during diving and it has been suggested that they have specialized lung architecture, including collateral ventilation, and volitional control of autonomic cardiac responses (Fahlman et al., 2017b; García-Párraga et al., 2018). The lung architecture would help create two pulmonary regions with distinctly different levels of alveolar ventilation (A) and perfusion (). By varying the level of ventilation-perfusion matching, the lung would be able to selectively exchange gases with different gas solubilities (West, 1962; Farhi and Yokoyama, 1967), thereby providing exchange of O2 and CO2 while minimizing N2 exchange (García-Párraga et al., 2018). Stressful situations could alter the ventilation-perfusion match, causing increased N2 exchange and risk for gas emboli. This provides avenues for new areas of research, offering an alternative explanation for how marine vertebrates can avoid the diving-related problems observed in human divers, and how failure of this adaptation may result in diving-related trauma (Frantzis, 1998; Fernandez et al., 2005, García-Párraga et al., 2014; Fernández et al., 2017; Fahlman et al., 2017a). Thus, if active management of pulmonary shunt and gas exchange is important, it makes sense that the sCL is not different between deep-diving and shallow-diving bottlenose dolphins. Still, the hypothesis made by Scholander (1940) that there should be significant differences in the compliance between the upper and lower airways is important as it allows compression and variation of the A/ to alter exchange of the different gases (Hodanbosi et al., 2016; Fahlman et al., 2018b).
In addition, both ecotypes may have similar traits to deal with pressure changes. The largest relative difference in pressure occurs close to the surface. At a depth of 10 m, the lung volume is reduced to half that at the surface, and the change in volume decreases exponentially with depth. Thus, to prevent pulmonary barotrauma all diving marine mammals should have a compliant lung/alveolar space, and a chest that is either highly compliant or recoils inward to low volumes. Evolutionary pressure may have resulted in different solutions to a common problem. It appears that all diving marine mammals measured to date have a similar sCL, regardless of ecotype, that is greater than that measured in land mammals (Fahlman et al., 2017b). Chest compliance, on the other hand, appears to differ among orders, where measurements on live pinnipeds suggest that chest compliance is high, which allows pressure to compress the lung without resistance (Fahlman et al., 2014). In cetaceans, on the other hand, the need for high respiratory flow seems to have created a chest that is stiffer than the lung, but that recoils strongly inward to low volumes (Figure 1). Thus, both strategies provide a respiratory system with a lung that can compress, an alveolar space that can empty to low volumes, and vary A to allow matching with to allow management of gas exchange (García-Párraga et al., 2018).
Conclusion
The measured resting sO2 in offshore bottlenose dolphins did not differ from measurements done for shallow-diving dolphins or measurements in other marine mammal species (Yazdi et al., 1999; Noren et al., 2013; Fahlman et al., 2015, 2017b). We showed that the offshore dolphins have greater O2 storage capacity (greater blood Hct), and propose that increased blood and muscle volume, and increased muscle myoglobin may also be important to increase O2 storage capacity. The RMR was on the low end but not different from RMR of coastal bottlenose dolphins. Thus, additional data on RMR of offshore dolphins will help resolve if increased muscle mass results in reduced basal metabolic rate in deep divers (Pabst et al., 2016). The sCL measurements for the offshore ecotype were similar to those in shallow-diving dolphins, suggesting a lack of specialized adaptations to lung compliance. This supports the idea that since the most extreme pressure changes happen within the first 10–100 m of diving, and further specializations to enable longer and deeper dives do not add much pulmonary stress. These data suggest that the structural properties of the lung itself may not differ substantially between ecotypes within a marine mammal species and perhaps across species, but that chest compliance is most likely different in cetaceans versus pinnipeds. In both cases, the structural properties allow increasing pressure to compress the alveolar space to very low volumes, preventing barotrauma. This supports a recent hypothesis that cetaceans vary A/ to selectively manage gas exchange (García-Párraga et al., 2018). If this hypothesis is correct, we would not expect to see major differences in the sCL between deep and shallow divers as during natural dives they would selectively control their gas exchange, making maximum dive depth per se a relatively minor determinant of sCL.
Author Contributions
All the authors participated in the field-work and gave final approval for the publication. AF, MJM, RW, JS, and RS conceived of the study, and designed the experiments. AF, MJM, RW, JS, FHJ, RFT, and RS collected the data. AF and AA analyzed the data. AF carried out the statistical analysis, and drafted the paper, with feedback from the co-authors.
Funding
Funding for this project was provided by the Office of Naval Research (ONR YIP Award No. N000141410563, and Dolphin Quest, Inc. FHJ was supported by the Office of Naval Research (Award No. N00014-1410410) and an AIAS-COFUND fellowship from Aarhus Institute of Advanced Studies under the FP7 program of the EU (Agreement No. 609033).
Conflict of Interest Statement
The authors declare that the research was conducted in the absence of any commercial or financial relationships that could be construed as a potential conflict of interest.
Acknowledgments
A special thanks to the many volunteers and staff at Dolphin Quest. A special thanks to Nigel Pollard, the Bermuda Government, the Bermuda Aquarium, Museum, and Zoo (BAMZ), and NOAA. We also thank Andres Jabois and Teresa Lorenzo from the Oceanografic, and Sunnie Brenneman of the Sarasota Dolphin Research Program for their assistance in the field.
Abbreviation
CL, dynamic lung compliance; fR, breathing frequency; Mb, body mass; sCL, mass-specific lung compliance; STPD, standard temperature pressure dry; TLC, total lung capacity; TLCest, estimated total lung capacity; O2, oxygen consumption rate; VT, tidal volume; A, Alveolar ventilation; , Alveolar perfusion.
References
Asper, E. D. (1975). Techniques of live capture of smaller cetacea. J. Fish. Res. Board Can. 32, 1191–1196. doi: 10.1139/f75-138
Bostrom, B. L., Fahlman, A., and Jones, D. R. (2008). Tracheal compression delays alveolar collapse during deep diving in marine mammals. Respir. Physiol. Neurobiol. 161, 298–305. doi: 10.1016/j.resp.2008.03.003
Enstipp, M. R., Ciccione, S., Gineste, B., Milbergue, M., Ballorain, K., Ropert-Coudert, Y., et al. (2011). Energy expenditure of freely swimming adult green turtles (Chelonia mydas) and its link with body acceleration. J. Exp. Biol. 214, 4010–4020. doi: 10.1242/jeb.062943
Fahlman, A., Brodsky, M., Wells, R., McHugh, K., Allen, J., Barleycorn, A., Sweeney, J. C., et al. (2018a). Field energetics and lung function in wild bottlenose dolphins, Tursiops truncatus, in Sarasota Bay Florida. R. Soc. Open Sci. 5:171280. doi: 10.1098/rsos.171280
Fahlman, A., Crespo Picazo, J. L., Sterba-Boatwright, B., Stacy, B. A., and Garcia-Parraga, D. (2017a). Defining risk variables causing gas embolism in loggerhead sea turtles (Caretta caretta) caught in trawls and gillnets. Sci. Rep. 7:2739. doi: 10.1038/s41598-017-02819-5
Fahlman, A., Hooker, S. K., Olszowka, A., Bostrom, B. L., and Jones, D. R. (2009). Estimating the effect of lung collapse and pulmonary shunt on gas exchange during breath-hold diving: the Scholander and Kooyman legacy. Respir. Physiol. Neurobiol. 165, 28–39. doi: 10.1016/j.resp.2008.09.013
Fahlman, A., Jensen, F., Tyack, P. L., and Wells R. (2018b). Modeling tissue and blood gas kinetics in coastal and offshore common bottlenose dolphins, Tursiops truncatus. Front. Physiol. 9, 1–13. doi: 10.3389/fphys.2018.00838
Fahlman, A., Loring, S. H., Ferrigno, M., Moore, C., Early, G., Niemeyer, M., et al. (2011). Static inflation and deflation pressure-volume curves from excised lungs of marine mammals. J. Exp. Biol. 214, 3822–3828. doi: 10.1242/jeb.056366
Fahlman, A., Loring, S. H., Levine, G., Rocho-Levine, J., Austin, T., and Brodsky, M. (2015). Lung mechanics and pulmonary function testing in cetaceans J. Exp. Biol. 218, 2030–2038. doi: 10.1242/jeb.119149
Fahlman, A., Loring, S. H., Johnson, S., Haulena, M., Trites, A. W., Fravel, V. A., et al. (2014). Inflation and deflation pressure-volume loops in anesthetized pinnipeds confirms compliant chest and lungs. Front. Physiol. 5:433. doi: 10.3389/fphys.2014.00433
Fahlman, A., Moore, M. J., and Garcia-Parraga, D. (2017b). Respiratory function and mechanics in pinnipeds and cetaceans. J. Exp. Biol. 220, 1761–1763. doi: 10.1242/jeb.126870
Fahlman, A., Svärd, C., Rosen, D. A. S., Wilson, R. S., and Trites, A. W. (2013). Activity as a proxy to estimate metabolic rate and to partition the metabolic cost of diving vs. breathing in pre- and post-fasted Steller sea lions. Aquat. Biol. 18, 175–184. doi: 10.3354/ab00500
Fahlman, A., Wilson, R., Holton, M., Archibald, E., Redcliffe, J., Lacave, G., et al. (2017c). “Physiology and movement to assess breathing frequency and tidal volume in marine mammals,” in Proceedings of the 6th International Bio-Logging Science Symposium (Lake Constance: International Bio-logging Society)
Fahlman, A., Wilson, R., Svärd, C., Rosen, D. A. S., and Trites, A. W. (2008). Activity and diving metabolism correlate in Steller sea lion Eumetopias jubatus. Aquat. Biol. 2, 75–84. doi: 10.3354/ab00039
Farhi, L. E., and Yokoyama, T. (1967). Effects of ventilation-perfusion inequality on elimination of inert gases. Respir. Physiol. 3, 12–20. doi: 10.1016/0034-5687(67)90019-9
Fernandez, A., Edwards, J. F., Rodruiquez, F., Espinosa de los Monteros, A., Herraez, M. P., Castro, P., et al. (2005). Gas and fat embolic syndrome involving a mass stranding of beaked whales (family ziphiidae) exposed to anthropogenic sonar signals. Vet. Pathol. 42, 446–457. doi: 10.1354/vp.42-4-446
Fernández, A., Sierra, E., Díaz-Delgado, J., Sacchini, S., Sánchez-Paz, Y., Suárez-Santana, C., et al. (2017). Deadly acute decompression sickness in Risso’s dolphins. Sci. Rep. 7:13621. doi: 10.1038/s41598-017-14038-z
García-Párraga, D., Crespo-Picazo, J. L., Bernaldo de Quirós, Y., Cervera, V., Martí-Bonmati, L., Díaz-Delgado, A. M., et al. (2014). Decompression sickness (“the bends”) in sea turtles. Dis. Aquat. Org. 111, 191–205. doi: 10.3354/dao02790
García-Párraga, D., Moore, M., and Fahlman, A. (2018). Pulmonary ventilation– perfusion mismatch: a novel hypothesis for how diving vertebrates may avoid the bends. Proc. Biol. Sci. 285:20180482. doi: 10.1098/rspb.2018.0482
Hall, A. J., Wells, R. S., Sweeney, J. C., Townsend, F. I., Balmer, B. C., Hohn, A. A., et al. (2007). Annual, seasonal and individual variation in hematology and clinical blood chemistry profiles in bottlenose dolphins (Tursiops truncatus) from Sarasota Bay, Florida. Comp. Biochem. Physiol. A Mol. Integr. Physiol. 148, 266–277. doi: 10.1016/j.cbpa.2007.04.017
Hodanbosi, M., Sterba-Boatwright, B., and Fahlman, A. (2016). Updating a gas dynamics model using estimates for California sea lions (Zalophus californianus). Respir. Physiol. Neurobiol. 234, 1–8. doi: 10.1016/j.resp.2016.08.006
Irving, L., Scholander, P. F., and Grinnell, S. W. (1941). The respiration of the porpoise, Tursiops truncatus. J. Cell. Comp. Physiol. 17, 145–168. doi: 10.1002/jcp.1030170203
Johansson, O., and Schagatay, E. (2012). Lung-Packing and Stretching Increases Vital Capacity in Recreational Freedivers. Vienna: European Respiratory Society.
Klatsky, L. J., Wells, R. S., and Sweeney, J. C. (2007). Offshore bottlenose dolphins (Tursiops truncatus): movement and dive behavior near the Bermuda pedestal. J. Mammal. 88, 59–66. doi: 10.1644/05-MAMM-A-365R1.1
Kooyman, G. L. (1973). Respiratory adaptations in marine mammals. Am. Zool. 13, 457–468. doi: 10.1093/icb/13.2.457
Kooyman, G. L., and Cornell, L. H. (1981). Flow properties of expiration and inspiration in a trained bottle-nosed porpoise. Physiol. Zool. 54, 55–61. doi: 10.1086/physzool.54.1.30155804
Kooyman, G. L., and Ponganis, P. J. (1998). The physiological basis of diving to depth: birds and mammals. Ann. Rev. Physiol. 60, 19–32. doi: 10.1146/annurev.physiol.60.1.19
Martín López, L. M., Miller, P. J. O., Aguilar de Soto, N., and Johnson, M. (2015). Gait switches in deep-diving beaked whales: biomechanical strategies for long-duration dives. J. Exp. Biol. 218, 1325–1338. doi: 10.1242/jeb.106013
Mead, J. G., and Potter, C. W. (1995). Recognizing two populations off the bottlenose dolphin (Tursiops Truncatus) of the Atlantic coast of North America-Morphologic and Ecologic Considerations. IBI Rep. 5, 32–44.
Miller, P. J., Johnson, M. P., Tyack, P. L., and Terray, E. A. (2004). Swimming gaits, passive drag and buoyancy of diving sperm whales Physeter macrocephalus. J. Exp. Biol. 207, 1953–1967. doi: 10.1242/jeb.00993
Moore, C., Moore, M. J., Trumble, S., Niemeyer, M., Lentell, B., McLellan, W., et al. (2014). Comparative analysis of marine mammal tracheas. J. Exp. Biol. 217, 1154–1166. doi: 10.1242/jeb.093146
Mortola, J. P., and Limoges, M. J. (2006). Resting breathing frequency in aquatic mammals: a comparative analysis with terrestrial species. Respir. Physiol. Neurobiol. 154, 500–514. doi: 10.1016/j.resp.2005.12.005
Mortola, J. P., and Sequin, J. (2009). End-tidal CO2 in some aquatic mammals of large size. Zoology 112, 77–85. doi: 10.1016/j.zool.2008.06.001
Noren, D. P., Holt, M. M., Dunkin, R. C., and Williams, T. M. (2013). The metabolic cost of communicative sound production in bottlenose dolphins (Tursiops truncatus). J. Exp. Biol. 216, 1624–1629. doi: 10.1242/jeb.083212
Pabst, D. A., McLellan, W. A., and Rommel, S. A. (2016). How to build a deep diver: the extreme morphology of mesoplodonts. Int. Comp. Biol. 56, 1337–1348. doi: 10.1093/icb/icw126
Piscitelli, M. A., McLellan, W. A., Rommel, S. A., Blum, J. E., Barco, S. G., et al. (2010). Lung size and thoracic morphology in shallow- and deep-diving cetaceans. J. Morphol. 271, 654–673. doi: 10.1002/jmor.10823
Ponganis, P. J. (2015). Diving Physiology of Marine Mammals and Seabirds. Cambridge: University Press. doi: 10.1017/CBO9781139045490
Quanjer, P. H., Tammeling, G. J., Cotes, J. E., Pedersen, O. F., Peslin, R., and Yernault, J. C. (1993). Lung volumes and forced ventilatory flows. Eur. Respir. J. Suppl. 6, 5–40. doi: 10.1183/09041950.005s1693
Reed, J. Z., Chambers, C., Hunter, C. J., Lockyer, C., Kastelein, R., Fedak, M. A., et al. (2000). Gas exchange and heart rate in the harbour porpoise, Phocoena phocoena. J. Comp. Physiol. B 170, 1–10. doi: 10.1007/s003600050001
Ridgway, S. H., and Johnston, D. G. (1966). Blood oxygen and ecology of porpoises of three genera. Science 151, 456–458. doi: 10.1126/science.151.3709.456
Ridgway, S. H., Scronce, B. L., and Kanwisher, J. W. (1969). Respiration and deep diving in the bottlenose porpoise. Science 166, 1651–1654. doi: 10.1126/science.166.3913.1651
Scholander, P. F. (1940). Experimental investigations on the respiratory function in diving mammals and birds. Hvalrådets Skrifter 22, 1–131.
Schwacke, L. H., Hall, A. J., Townsend, F. I., Wells, R. S., Hansen, L. J., Hohn, A. A., et al. (2009). Hematologic and serum biochemical reference intervals for free-ranging common bottlenose dolphins (Tursiops truncatus) and variation in the distributions of clinicopathologic values related to geographic sampling site. Am. J. Vet. Res. 70, 973–985. doi: 10.2460/ajvr.70.8.973
Sumich, J. L. (2001). Direct and indirect measures of oxygen extraction, tidal lung volumes and respiratory rates in a rehabilitating gray whale calf. Aquat. Mamm. 27, 279–283.
Sumich, J. L., and May, M. A. (2009). Scaling and remote monitoring of tidal lung volumes of young gray whales, Eschrichtius robustus. Mar. Mamm. Sci. 25, 221–228. doi: 10.1111/j.1748-7692.2008.00272.x
Wahrenbrock, E. A., Maruscha, G. F., Elsner, R., and Kenney, D. W. (1974). Respiration and metabolism in 2 baleen whale calves. Mar. Fish. Rev. 36, 3–9.
Wells, R. S., Rhinehart, H. L., Hansen, L. J., Sweeney, J. C., Townsend, F. I., Stone, R., et al. (2004). Bottlenose dolphins as marine ecosystem sentinels: developing a health monitoring system. EcoHealth 1, 246–254. doi: 10.1007/s10393-004-0094-6
Wells, R. S., and Scott, M. D. (2018). “Bottlenose dolphin: common bottlenose dolphin (Tursiops truncatus),” in Encyclopedia of Marine Mammals, eds B. Würsig, J. Thewissen, and K. Kovacs (San Diego, CA: Academic Press/Elsevier).
West, J. B. (1962). Regional differences in gas exchange in the lung of erect man. J. Appl. Physiol. 17, 893–898. doi: 10.1152/jappl.1962.17.6.893
Williams, T. M. (2001). Intermittent swimming by mammals: a strategy for increased energetic efficiency during diving. Am. Zool. 41, 166–176.
van der Hoop, J. M., Fahlman, A., Jensen, F. H., Johnson, M., Brodsky, M., Hurst, T., et al. (2014). “Acoustic parameters as indicators of metabolic rate in Bottlenose Dolphins,” in Proceedings of the 5th Biologging Science Symposium, Strasbourg.
Yazdi, P., Kilian, A., and Culik, B. M. (1999). Energy expenditure of swimming bottlenose dolphins (Tursiops truncatus). Mar. Biol. 134, 601–607. doi: 10.1007/s002270050575
Keywords: lung mechanics, total lung capacity, field metabolic rate, energetics, minimum air volume, diving physiology, marine mammals, spirometry
Citation: Fahlman A, McHugh K, Allen J, Barleycorn A, Allen A, Sweeney J, Stone R, Faulkner Trainor R, Bedford G, Moore MJ, Jensen FH and Wells R (2018) Resting Metabolic Rate and Lung Function in Wild Offshore Common Bottlenose Dolphins, Tursiops truncatus, Near Bermuda. Front. Physiol. 9:886. doi: 10.3389/fphys.2018.00886
Received: 05 May 2018; Accepted: 19 June 2018;
Published: 17 July 2018.
Edited by:
Jose Pablo Vazquez-Medina, University of California, Berkeley, United StatesReviewed by:
Birgitte I. McDonald, Moss Landing Marine Laboratories, United StatesTania Zenteno-Savin, Centro de Investigación Biológica del Noreste (CIBNOR), Mexico
Copyright © 2018 Fahlman, McHugh, Allen, Barleycorn, Allen, Sweeney, Stone, Faulkner Trainor, Bedford, Moore, Jensen and Wells. This is an open-access article distributed under the terms of the Creative Commons Attribution License (CC BY). The use, distribution or reproduction in other forums is permitted, provided the original author(s) and the copyright owner(s) are credited and that the original publication in this journal is cited, in accordance with accepted academic practice. No use, distribution or reproduction is permitted which does not comply with these terms.
*Correspondence: Andreas Fahlman, YWZhaGxtYW5Ad2hvaS5lZHU=