- 1Departamento de Biotecnologia, Centro de Biotecnologia, Universidade Federal da Paraíba, João Pessoa, Brazil
- 2Departamento de Fisiologia e Patologia, Centro de Ciências da Saúde, Universidade Federal da Paraíba, João Pessoa, Brazil
- 3Departamento de Fisiologia, Faculdade de Medicina de Ribeirão Preto, Universidade de São Paulo, Ribeirão Preto, Brazil
- 4Departamento de Ciências Fisiológicas, Instituto de Ciências Biomédicas, Universidade Federal de Alfenas, Alfenas, Brazil
It is known that circulating angiotensin II (ANG-II) acts on the circumventricular organs (CVOs), which partially lack a normal blood-brain barrier, to stimulate pressor responses, vasopressin (AVP), and oxytocin (OT) secretion, as well as sodium and water intake. Although ANG-II type 1 receptors (AT1R) are expressed in neurons and astrocytes, the involvement of CVOs glial cells in the neuroendocrine, cardiovascular and behavioral responses induced by central ANG II remains to be further elucidated. To address this question, we performed a set of experiments combining in vitro studies in primary hypothalamic astrocyte cells (HACc) and in vivo intracerebroventricular (icv) microinjections into the lateral ventricle of awake rats. Our results showed that ANG-II decreased glutamate uptake in HACc. In addition, in vivo studies showed that fluorocitrate (FCt), a reversible glial inhibitor, increased OT secretion and mean arterial pressure (MAP) and decreased breathing at rest. Furthermore, previous FCt decreased AVP secretion and sodium intake induced by central ANG-II. Together, our findings support that CVOs glial cells are important in mediating neuroendocrine and cardiorespiratory functions, as well as central ANG-II-induced AVP release and salt-intake behavior in awake rats. In the light of our in vitro studies, we propose that these mechanisms are, at least in part, by ANG-II-induced astrocyte mediate reduction in glutamate extracellular clearance.
Introduction
Hypovolemia produced by dehydration, salt loading or water deprivation induces a marked increase in circulating ANG-II, which modulates body fluid and cardiorespiratory homeostasis through circumventricular organs (CVOs). CVOs are rich in fenestrated capillaries and partially lack the blood brain barrier (Fitzsimons, 1980; Gross et al., 1987; Beresford and Fitzsimons, 1992). Intracerebroventricular microinjections of ANG-II induce: (i) AVP and OT release (Lang et al., 1981; Beresford and Fitzsimons, 1992; Reis et al., 2007, 2010; Sakai et al., 2007), (ii) water and sodium intake (Fitzsimons, 1980; Beresford and Fitzsimons, 1992; Sakai et al., 2007; Reis et al., 2010; Matsuda et al., 2016; Roncari et al., 2017), and (iii) cardiorespiratory responses (Potter and McCloskey, 1979; Alexander and Lumbers, 1981; Bains et al., 1992; Ohtake and Jennings, 1993; Saad et al., 2004; Reis et al., 2010). There is a high density of angiotensinergic receptors (AT1R and AT2R) in neurons but also in CVOs astrocytes, mainly from subfornical organ (SFO) and organum vasculosum of the lamina terminalis (OVLT; Gebke et al., 1998). In vitro studies have shown that ANG-II in isolated SFO, OVLT neurons or astrocytes induce calcium transients waves (Li and Ferguson, 1993; Gebke et al., 1998), suggesting that these cells could be important intermediates involved in the hydromineral and cardiorespiratory homeostasis induced by central ANG-II. It is known that circulating ANG-II induces new ANG-II synthesis in SFO neurons projecting to pre-autonomic and neuroendocrine portions of the paraventricular nuclei (PVN; Bains et al., 1992; Li and Ferguson, 1993; Sakai et al., 2007; Burmeister et al., 2011; Stern et al., 2016). The PVN has been described as a key angiotensin-sensitive hypothalamic nucleus; integrating neuroendocrine, behavioral and cardiorespiratory responses to hydromineral imbalance (Ferguson, 1988; Bains et al., 1992; Yeh et al., 1997; Schlenker et al., 2001; Stern et al., 2016). Hypothalamic astrocytes appear to be functionally critical for ANG II-induced fluid balance and hemodynamic homeostasis, since an elegant study by Stern et al. (2016), combining in vitro patch-clamp and in vivo approaches showed that ANG-II in the PVN inhibited astrocyte-specific glutamate transporter (GLT-1) activity, inducing increased extracellular glutamate levels, which contributed to increased pre-sympathetic neuronal activity, sympathoexcitatory outflow, and blood pressure. We hypothesize that neuroendocrine, behavioral and cardiorespiratory responses, induced by central ANG-II, could be mediated by CVOs glial cells. To address this issue, we performed in vitro experiments to evaluate ANG-II effects in HACc and in vivo experiments analyzing the effects of glial inhibition on the ANG-II-induced neuroendocrine, behavioral, and cardiovascular responses in awake rats.
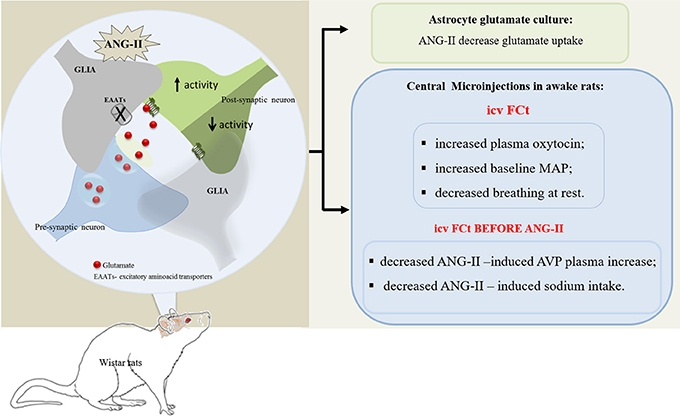
Graphical Abstract. In vitro studies showed that ANG-II decreased astrocyte glutamate uptake, in turn, contributing to increase extracellular glutamate bioavailability. In addition, in vivo studies showed that CVOs glial cells are important to modulate neuroendocrine and cardiorespiratory homeostasis, including ANG-II-induced AVP release and sodium intake in awake rats.
Materials and Methods
Ethical Approval
All experimental procedures used in this study were approved by Ethics Committee on the Use of Animals of the Biotechnology Center of the Federal University of Paraiba [CEUA-CBIOTEC/UFPB (ref. numbers CEUA no° 0606/13 and CEUA no° 023/15)] or by the Ethics Committee on Animal Use of the School of Medicine of Ribeirao Preto of the University of Sao Paulo [CEUA-FMRP/USP (ref. number n° 95/2011)] and performed according to the guidelines of the Brazilian code of practice for care and use of animals for scientific purposes (Brazilian College of Animal Experimentation-COBEA).
Primary hypothalamic astrocyte cultures were generated from newborn (2–3 days old) male Wistar rats (Rattus norvegicus), obtained from the Animal Facility of the School of Medicine of Ribeirao Preto—University of Sao Paulo (FMRP-USP). The rats pups were swiftly decapitated, the brains were dissected and placed in an isotonic salt solution. The medial basal hypothalamus was rostrally delimited by the optic chiasm and mammillary bodies, laterally by the tuber cinereum and by the top of third ventricle, dissected and isolated from the brain. Connective tissues were chemically digested with trypsin (0.05%) and mechanically dissociated (fire-polished glass Pasteur pipette). Cells were grown 7–10 days in a CO2 incubator at 37°C in Dulbecco's modified Eagle's medium (DMEM)/F12 (Sigma) containing 10% bovine fetal serum (LGC Biotechnology) and 1% penicillin-streptomycin (Mediatech). Upon reaching confluence, cell cultures were shaken at 200 rpm for 30 min, according to McCarthy and De Vellis (1980). Afterwards, the astrocyte cultures were transferred to 12- or 6-well sterile culture plates (TPP®) with fresh medium.
Tritiated [3H] Aspartate Uptake Assay
[3H]aspartate uptake modulated by ANG II was evaluated in HACs. Considering that excitatory amino acid transporter (EAAT) mediate aspartic acid and glutamic transport (Schousboe et al., 2004; Kavanaugh, 2009). Here, [3H] aspartate was used as tracer of glutamate to measure GLAST and GLT-1 (EEATs) activity in HACs. Thus, assays were performed with (3H)-aspartate [0.1 mCi/mL (PerkinElmer Life and Analytical Sciences)] to evaluate aspartate astrocyte uptake. On the day of the experiment, culture medium was removed and incubated at 37°C for a variable period of time (according to the experimental protocol) with (1) isotonic Hank solution (~290 mOsm/kg H2O, 128 mM NaCl, pH 7.4) + 10 μL (0.1 μCi) of tritiated (3H)-, assigned as control group; (2) isotonic Hank solution containing ANG II (1, 10, or 100 nM, Peninsula Laboratories) + 10 μL (0.1 μCi) of tritiated (3H)-aspartate. For each ANG II concentration we performed a different set of experiments, stimulating HACCs during 5, 10, 15, or 30 min. Thus, each amount of [3H]-aspartate taken up by astrocytes was quantified by liquid scintillation counting (Cristovao-Ferreira et al., 2011; Pérez-Domínguez et al., 2014). Afterwards, Hank solution was removed and adhered cells were lysed with cold deionized sterile water (for 2 min) and, with the aid of a cell scraper, were collected into vials containing scintillation liquid (Scintisafetm econo 1, Fisher Scientific sx20-5). Due to the adsorption of plastic surfaces shown by aspartate, a blank well (without cells) was utilized as a measure of background signal. Samples of medium and astrocyte lysates were submitted to a β-radiation counting (LS 6500—Beckman, Beckmann Instruments Inc., Fullerton, CA, USA).
Immunofluorescence for GFAP and GLT-1
For immunofluorescence studies, after the separation of astrocytes by agitation, the cells were transferred to 12-well sterile culture plates (TPP®) containing glass coverslips pretreated with poly-L-lysine (5 mg/50 mL, Sigma). Once confluent, astrocyte cells were then fixed with methanol for 3 min. Coverslips containing the fixed cells were incubated for 1 h with 10% Normal Horse Serum (Vector Laboratories), followed by primary antibodies to astrocyte specific markers; rabbit anti-glial fibrillary acidic protein (anti-GFAP, 1:500, Sigma-Aldrich #G9269) and mouse anti-SLC 1A2 [GLT-1 subtype (mouse 1:500, Sigma-Aldrich #WH0006506M10)] or HACCs were incubated with rabbit anti-GFAP in combination with primary antibody to neurons specific marker; mouse anti-NeuN (1:750, Millipore #MAB 377) antibodies, overnight. After incubation, the coverslips were rinsed in 0.01 M PBS and incubated with Cy3-conjugated donkey anti-mouse and FITC-conjugated donkey anti-rabbit secondary antibody (1:250, Jackson Immunoresearch) for 2 h, washed and to finish the cells were incubated with DAPI (1 μg/ml Thermo Scientific #28718-90-3) for 5 min. After washes, cells were fixed with mounting medium (Fluromount) and visualized under fluorescence light. Immunofluorescence to GFAP, GLT-1, and NeuN were qualitatively performed to assess astrocyte cells primary cultures homogenity.
Stereotaxia and Central Microinjections
The rats were anesthetized with ketamine (75 mg/Kg body weight, i.p.) and xylazine (10 mg/Kg body weight, i.p.) using a stereotaxic apparatus (David-Kopf, Tujunga, CA, EUA). Unilateral stain- less steel guide cannulas (12-mm-long-cannula- 22 gauge Small Parts, Miami Lakes, FL, USA) were implanted in the left lateral cerebral ventricle, according to brain atlas of Paxinos and Watson (1997), as follows; −0.5 mm rostral to the interaural line, left +1.4 mm from the medial suture and −3.9 mm deep from the skull. The cannula was fixed in the skull using dental acrylic resin and two metal screws. A tight-fitting mandril was kept inside the guide cannula to avoid occlusion. After surgery, the rats received a prophylactic injection of penicillin and streptomycin (0.1 mL/100 g 1.200.000 IU, Fort Dodge, Campinas, SP, Brazil). The drugs were microinjected icv into the lateral ventricle using a Hamilton microsyringe (Hamilton, Reno, NV, USA) connected by a PE-10 polyethylene tubing to a dental needle. At the time of the experiment, the mandril was carefully removed and the dental needle was inserted into the guide cannula. The final volume microinjected was 0.5 μL.
Catheterization
One day before the cardiorespiratory experiment and 5–6 days after stereotaxic surgery, the rats were anesthetized with ketamine and xylazine (75 and 10 mg/Kg, respectively, i.p). A polyethylene catheter (PE-10 connected to PE-50; Clay Adams, Parsippany, NJ, USA) was inserted into the abdominal aorta through the femoral artery for cardiovascular recording. The catheters were tunneled subcutaneously and exteriorized in the back of the neck. After catheterization surgery, animals were housed in individual cages.
Cardiovascular and Respiratory Recordings in Awake Rats
On the day of the experiment (24 h after artery catheterization), the rats were placed in Plexiglas chambers (5 l) for respiratory measurements. The femoral artery catheter was then flushed with heparinized saline to prevent clotting and connected to a pressure transducer (BRPL2, WPI, Sarasota, FL, USA) coupled to an amplifier and an acquisition system (PowerLab, ADInstruments, Bella Vista, NSW, Australia) running LabChart 5.0 (ADInstruments, Bella Vista, NSW, Australia). MAP (mmHg) and HR (bpm) were derived from the pulsatile arterial pressure (PAP, mmHg). Baseline BP and HR were recorded for 60 min. After establishing baseline cardiovascular parameters, ventilatory activity was measured using the whole-body plethysmographic method according to (Malan, 1973). After each icv microinjection, the plexiglas chamber was closed and air flow was suspended for short periods (3 min). Before breath recordings, we performed a volume calibration via injecting 1 ml of air into the chamber and subsequently captured air displacement by the respiratory cycles via a pressure differential transducer connected to a signal amplifier (ML141 Spirometer, 196 PowerLab; ADInstruments). Tidal volume (VT, mL) and minute volume (VM, mL) were derived from the breath according to the method reported by Bartlett and Tenney (1970).
Animals and in Vivo Experimental Protocols
Male wistar rats (R. norvegicus) weighing 260–280 g (total 64 animals) were obtained from the Biotechnology Center of Federal University of Paraiba and from the School of Medicine of Ribeirao Preto, University of São Paulo, Brazil. The animals were maintained in individual cages at controlled room temperature (21 ± 2°C), 12-h light-dark cycle (lights were on from 6:00 a.m. to 6:00 p.m.) and with tap water and food (Labina®, Purina, SP, Brazil) ad libitum.
Experimental Protocols
All studies were performed in awake and freely moving rats. The animals were assigned to one of three experimental protocols: neuroendocrine, drinking, and cardiorespiratory responses. In the neuroendocrine and drinking protocols the rats were divided in four groups, according to treatment; (1) saline (0.15 M NaCl); (2) ANG-II (0.1 M); (3) FCt (50 mM) and (4) FCt (50 mM) + ANG-II (0.1 M). The neuroendocrine protocol was designed to measure AVP and OT plasma release. Five-to-six days after stereotaxic surgery and 24 h before the experiment the rats were placed in the experimental room. On the experimental day, microinjections were performed in 5 min intervals. Five minutes after the second icv microinjection, the animals were decapitated and trunk blood was collected for radioimmunoassay analyses. The drinking protocol was designed to measure cumulative water and sodium (1.5% NaCl) intake. Two days after stereotaxic surgery, and 72 h before the experiments, the animals were placed in an individual metabolic cages provided with water and sodium solution (1.5% NaCl) burettes. During the experiment, food was removed, and cumulative water and sodium intake were measured at 5, 15, 30, 60, 120, 240 min after the last microinjection. In the cardiorespiratory protocol, 5 days after stereotaxic surgery the animals were anesthetized for femoral artery catheterization. The cardiorespiratory protocol was designed to measure mean arterial pressure (MAP, mmHg), heart rate (HR, bpm), respiratory frequency (fR, beats/min), VT (mL), and VM (mL). Twenty-four hours after catheterization, the animals were placed in a plethysmographic chamber to record ventilatory parameters and the femoral artery catheter was connected to a pressure transducer to record cardiovascular parameters. Before beginning the cardiorespiratory recording, at least 60 min period was allowed for stabilization of parameters and animal adaptation. We performed icv microinjection of ANG-II (0.1 M) before (as control) and 5, 10, 15, and 30 min after icv saline (0.15 M NaCl) or FCt (50 mM) pretreatment.
ANG-II and Fluorocitrate (FCt) Dilution
ANG-II (Millipore #CAS 447-91-3) was dissolved in sterile saline (0.15 M) at a final concentration of 0.1 M (Reis et al., 2010). Fluorocitrate (Sigma-Aldrich #F9634) solutions were prepared according to a protocol based on the study by Costa et al. (2013) and Paulsen et al. (1987). In brief, 2 mg of barium fluorocitrate was dissolved in 10 μL of HCl 1 M until it formed a homogeneous solution and Na2SO4 0.1 mM was added dropwise in order to precipitate Ba++ ions. Next, 20 μl of Na2HPO4 0.1 M was added and the solution was centrifuged at 3,000G for 10 min. The supernatant was isolated and diluted in saline solution (0.15 M NaCl) to a final concentration of 50 mM. The pH of the final FCt solution was adjusted to 7.4 with sodium bicarbonate (Reagen, Rio de Janeiro, Brazil).
The toxic effect of FCt seems to depend not only for the dose but also on the volume and central area microinjected (Paulsen et al., 1987, 1988a,b; Hassel et al., 1992). Studies by Paulsen et al. (1987) suggested that 1 nmol/1 μL of FCt microinjected intrastriatally is not accompanied by seizure and acts as a selective, reversible glial cell inhibitor. On the other hand, studies by Mirsattari et al. (2008) observed that FCt 0.84 nmol/0.125 μL microinjected intracortically, induces seizure. Additionally, intratriatal microinjection of FCt, at the same dose (1 nmol) as Paulsen et al. (1987) but in a larger volume (i.e., 1 μL), induce seizures (Hassel et al., 1992). In our study the FCt dose (50 mM/500 nL) was chosen by considering the drug dilution in the total estimated (400–550 μL) cerebrospinal fluid volume (i.e., adult rats ~300 g; Frankmann, 1986; Pegg et al., 2010). It is important to note that in our study icv microinjection of FCt was not accompanied by seizures or death over 4 h of observation (i.e., drinking behavioral protocol) and the physiological changes induced by this glial cell inhibitor were reversible a few minutes after microinjection, as we observed in the cardiorespiratory protocol.
Determination of Plasma Hormone Levels
After decapitation, trunk blood was collected into cold plastic tubes containing heparin (10 μL per mL of blood) to measure the plasma OT and AVP levels. Plasma was obtained after centrifugation at 3,000 rpm for 25 min at 4°C and stored at −70°C until specific extraction and immunoassay procedures were performed. AVP and OT were extracted from 1 ml of plasma with acetone and petroleum ether. All measurements were performed in duplicate. The inter- and intra-assay variations were 12.6 and 7% for OT, and 17.5 and 3.3% for AVP, respectively. AVP and OT plasma measurements were performed using specific radioimmunoassay techniques, as previously described (Haanwinckel et al., 1995; Elias et al., 1997).
Histological Procedure
Histological examination of the microinjection was performed after completion of all experiments. The rats were anesthetized via ip injection of ketamine and xylazine (75 and 10 mg/Kg, respectively), and 0.5 μl of filtered Evan's Blue 2% (Vetec, Química Fina Ltda, Rio de Janeiro, RJ, Brazil) dye was microinjected in the lateral ventricle as a marker for the microinjection site. Animals were transcardially perfused with 10% formaldehyde and the brains were removed and kept in sucrose (30%) solution for 24 h. Sections (60 μm) from hypothalamus were cut on a cryostat (Leica, Wetzlar, Germany). Histological sections were analyzed using Paxinos and Watson (1997) atlas as reference and considered positive when guide cannula tracing were visualized in bright field microscopy.
Results
Effects of ANGII on Hypothalamic Astrocyte Culture Cells-Induced Glutamate Uptake
As represented by Figures 1A–G, HACs primary culture homogeneity were qualitatively determined, as showed in the colocalization between GFAP and DAPi (Figure 1D) and GFAP and GLT-1 (Figure 1G) and confirmed by no immunoreactivity for NeuN (Figure 1B). HACc were treated for 5 min with ANG-II at 1, 10, and 100 nM as shown in Figure 1H. ANG-II stimulation induced a decrease in the 3H-aspartate uptake in primary hypothalamic astrocytes [F(3, 37) = 6.7, P = 0.001]; similar results were obtained 5, 15, and 30 min after ANG-II stimulation at 100 nM [F(3, 105) = 10.4, P < 0.0001]. It is important to note that 5 min (53 ± 5% control) ANG-II stimulation was more efficient in reducing 3H-aspartate uptake than 10 (86 ± 7.5% control) or 15 min (83 ± 5.6% control; Figure 1I).
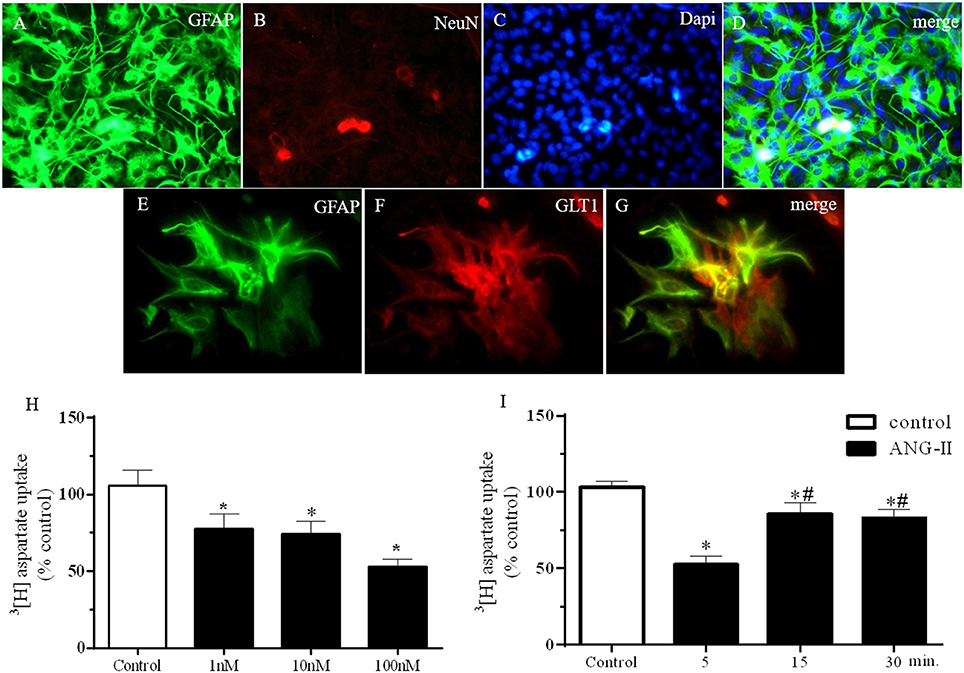
Figure 1. ANG-II decrease 3[H]-Aspartate uptake in hypothalamic astrocyte primary cultures cells. (A–G) Immunofluorescence showing GFAP (A,E), NeuN (B), DAPi (C), and GLT1 (F) in primary astrocyte culture. Panel (G) merge of panels (A–C). (H) 3[H] Aspartate uptake after ANG-II stimulation at 1, 10, or 100 nM during 5 min. (I) 3[H] Aspartate uptake after ANG-II stimulation at 100 nM during 5 (n = 11), 15 (n = 18), or 30 (n = 26) min. Differences among groups were calculated by One-Way ANOVA followed by the Newman-Keuls post test. Values were expressed as % of control. *p < 0.05 vs. control; #p< 0.05 vs. 15 and 30 min.
Effects of FCt Pretreatment on Central ANG-II-Induced AVP and OT Secretion
Figure 2 shows plasma concentration of AVP (Figure 2A) and OT (Figure 2B) induced by icv saline or ANG-II in animals pretreated with saline or FCt. For AVP secretion (Figure 2A), we found a statistically significant effect of treatment [F(3, 19) = 5.7, p = 0.006]. As expected, central ANG-II induced an increase in AVP secretion [2.3 ± 0.4 vs. 1.3 ± 0.1 pg/mL]. Microinjection of FCt alone did not change AVP plasma levels (1 ± 0.1 vs. 1.3 ± 0.1 pg/mL), however FCt pretreatment attenuated ANG-II-induced AVP plasma increase (1.3 ± 0.2 vs 2.3 ± 0.4 pg/mL). For OT secretion (Figure 2B) we again found a significant effect of treatment [F(3, 18) = 3.4, p = 0.04]. Central ANG-II induced an increase in OT plasma levels [4.0 ± 0.8 vs. 1.4 ± 0.2 pg/mL]. In addition, FCt alone increased OT plasma levels (3.4 ± 0.7 vs. 1.4 ± 0.2 pg/mL), however it did not change the ANG-II-induced effect on OT release (3.2 ± 0.4 vs. 4.0 ± 0.8 pg/mL).
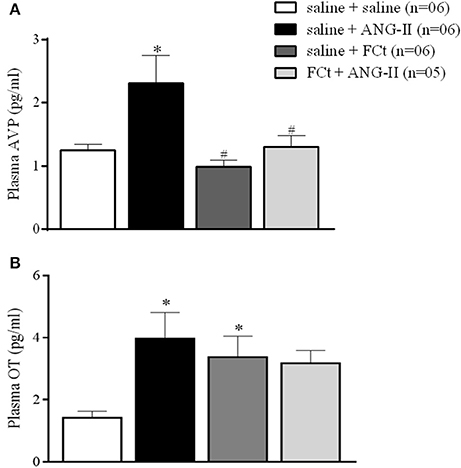
Figure 2. Effect of icv saline, ANG-II or FCt microinjection on vasopressin [AVP (A)] and Oxytocin [OT (B)] plasma release after local saline or FCt pretreatment in awake rats. Differences among groups were calculated by One-Way ANOVA followed by the Newman–Keuls post test. Values were expressed as means ± SEM. *p < 0.05 vs. control group (saline + saline). #p < 0.05 vs. saline + ANG-II group.
Effects of FCt Pretreatment on Central ANG-II-Induced Water and Sodium Intake
The results summarized in Figure 3 show cumulative water and sodium intakes induced by central ANG-II (icv) in saline or FCt pretreated animals before and 5, 15, 30, 60, 120, and 240 min after ANG-II injection. Data analysis indicated a significant increase in the water intake as function of treatment [F(3, 120) = 4.3, P = 0.0001], but this parameter did not change as a function of time [F(5, 120) = 1.2, P = 1] or interaction between factors [F(5, 120) = 0.3, P = 1]. As indicated in Figure 3A, central ANG-II significantly increased cumulative water intake (7 ± 1 vs. 0.25 ± 0.2 mL/4 h), being this effect was not changed by previous FCt administration (7 ± 1 vs. 6 ± 0.6 mL/4 h). The results also showed a significant increase in sodium intake as function of treatment [F(3, 120) = 240, P < 0.0001] and time [F(5, 120) = 13, P < 0.0001], as well as an interaction between factors [F(15, 120) = 4.4 P < 0.0001]. Furthermore, central ANG-II-induced a marked increase in sodium intake (16 ± 1 vs. 2.5 ± 0.7 mL/4 h), which was blocked by previous FCt microinjection (2.7 ± 0.3 vs. 16 ± 1 ml/4 h) (Figure 3B). Nevertheless, FCt alone did not change cumulative water (0.25 ± 0.2 vs. 0.7 ± 0.1 mL/4 h; Figure 3A) or sodium intake (0.8 ± 0.3 vs. 2.5 ± 0.7 mL/4 h; Figure 3B).
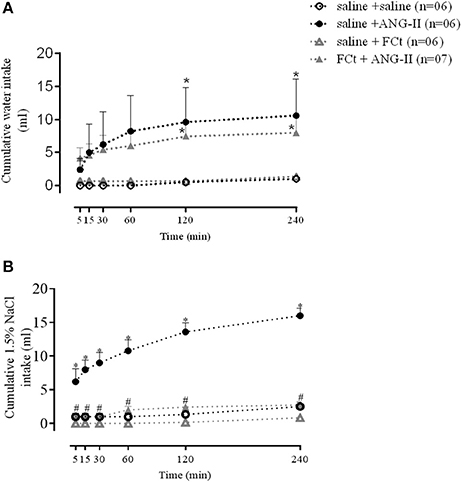
Figure 3. Effect of icv saline, ANG-II or FCt microinjections on water (A) and sodium [1.5% NaCl (B) intake] 5, 15, 30, 60, 120, and 240 min after saline or FCt pretreatment in awake rats. Differences among groups were calculated by Two-Way ANOVA followed by the Newman–Keuls post test. Values were expressed as means ± SEM. *p < 0.05 vs. control group (saline + saline). #p < 0.05 vs. saline + ANG-II group.
Effects of FCt Pretreatment on Central ANG II-Induced Cardiorespiratory Responses
The representative tracings in Figure 4A show changes in PAP, MAP, and HR in response to central ANG-II microinjection in awake rats in the presence or absence of FCt. One-way ANOVA analysis revealed a significant difference in baseline MAP as a function of treatment [F(2, 18) = 11.5, p = 0.0006]. Microinjection of ANG-II (135 ± 2.8 vs. 116 ± 2 mmHg) or FCt (129 ± 3.6 vs. 116 ± 2 mmHg) increased baseline MAP, but no significant changes were observed in baseline HR (Figures 4A–C). Results showed that FCt pretreatment did not alter the central ANG-II-induced pressor response [F(4, 25) = 1, p = 0.4; Figures 4A,D] or baseline HR [F(4, 25) = 1.2, p = 0.35; Figure 4E]. As observed in Table 1, saline (0.15 M) microinjection did not change baseline PAP, MAP, or HR, neither cardiovascular changes were induced by central ANG-II.
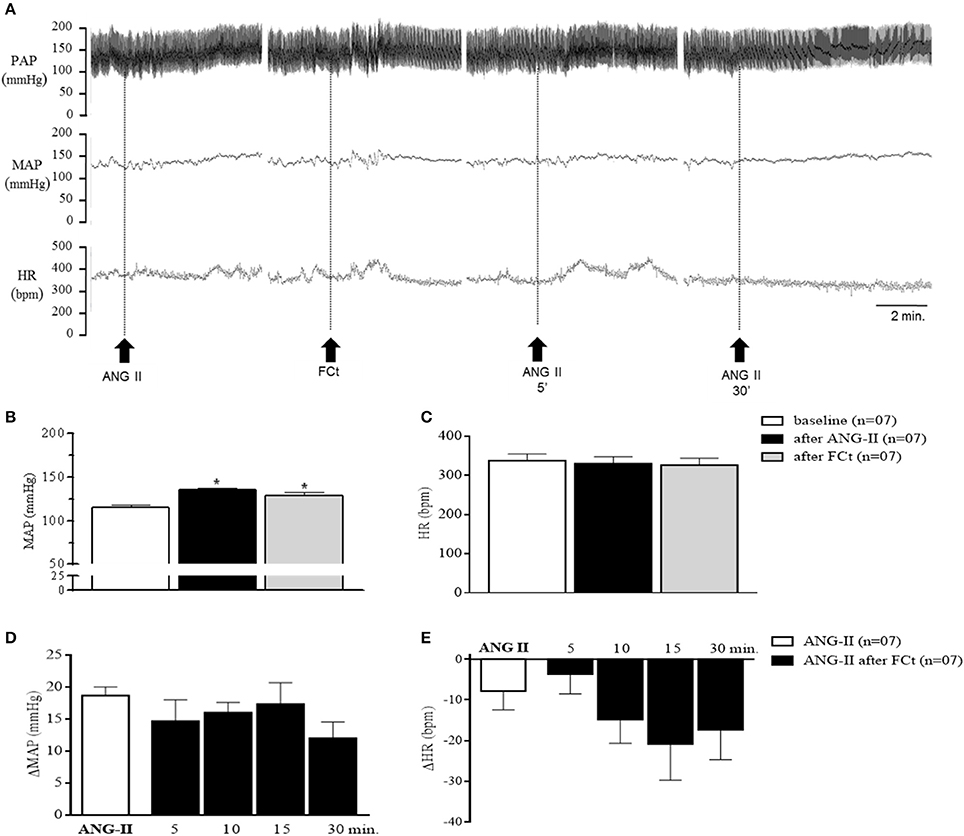
Figure 4. Effect of icv ANG-II or FCt microinjection on baseline cardiovascular parameters. (A) Representative tracings of one rat showing changes in pulsatile arterial pressure (PAP, mmHg), mean arterial pressure (MAP, mmHg), and baseline heart rate (HR, bpm) induced by icv ANG-II before and 5 and 30 min after FCt pretreatment. Arrows indicate icv ANG-II or FCt microinjections. (B,C) Changes in baseline MAP and (B) heart rate (C) before and after icv microinjection of ANG-II or FCt. (D,E) changes in MAP (D) and HR (E) to icv microinjection of ANG-II before and 5, 10, 15, and 30 min after local FCt pretreatment in awake rats. Differences among groups were calculated by One-Way ANOVA followed by the Newman–Keuls post test. Values were expressed as means ± SEM. *p < 0.05 vs. control.
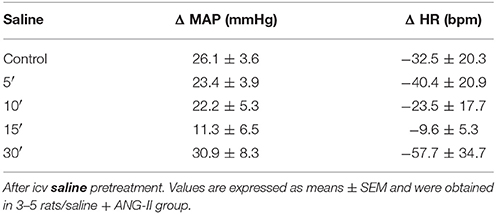
Table 1. Changes in baseline MAP (Δ, mmHg) and HR (Δ, bpm) induced by icv ANG-II microinjections before (control) and 5, 10, 15, and 30 min.
Figure 5A show typical respiratory recordings of one representative rat. Central ANG-II did not change baseline fR, VT, or VM (Figures 5B–D and Table 2). Interestingly, central FCt microinjection decreased breathing at rest [77.7 ± 2.9 vs. 109. ± 4.6 breaths/min; F(2, 22) = 13, P = 0.0002; Figures 5A,B], but did not change VT [9.7 ± 0.5 vs. 8.9 ± 0.9 mL.Kg−1; F(2, 22) = 0.35, P = 0.7; Figure 5C] or VM [827.2 ± 47 vs. 1002 ± 83 mL.Kg−11 (F(2, 22) = 1.2, P = 0.3) (Figure 5D). In addition, icv microinjection of saline + ANG-II or FCt + ANG-II did not change respiratory fR, VT, or VM at rest (Table 2).
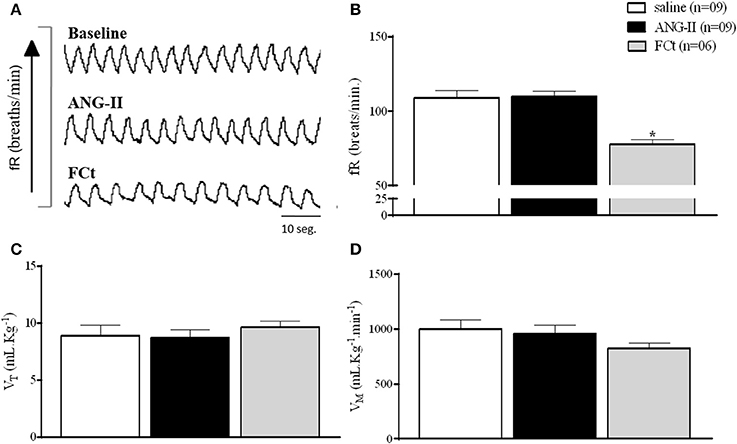
Figure 5. Effect of icv ANG-II or FCt microinjection on baseline respiratory parameters. (A) Representative tracings of one rat showing the baseline and changes in the fR (breaths/min) induced by icv microinjection of ANG-II and FCt. Arrows indicate icv ANG-II or FCt microinjections. (B–D) Respiratory frequency [fR, breaths/min (B)]; Tidal Volume [VT mL.Kg−1 (C)] and Volume Minute [VM mL.Kg-1.min−1 (D)] after microinjection icv of saline, ANG-II, or FCt. One-Way ANOVA followed by the Newman–Keuls post test. Values were expressed as means ± SEM. *p < 0.05 vs. saline.
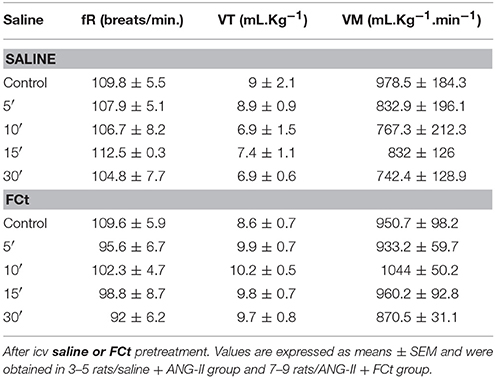
Table 2. Changes in baseline fR, VT, and VM induced by icv ANG-II microinjections before (control) and 5, 10, 15, and 30 min.
Histology
Figure 6 shows a photomicrograph of a coronal brain section illustrating the unilateral track of the microinjection site in the left lateral ventricle of one representative animal. For all experimental protocols, only rats with positive histological verification of the cannula site were included in the analyses.
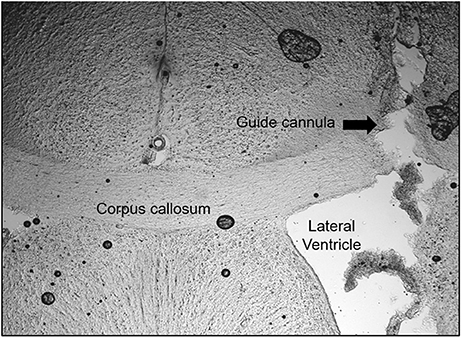
Figure 6. Sites of the unilateral microinjections into the lateral ventricle. Photomicrograph of a coronal section (60 μm) of the brain of one rat, representative of the group, showing microinjections tracing in the lateral ventricle. Arrows show the tract of the cannula.
Discussion
The present set of in vitro data suggest that ANG-II stimulates hypothalamic astrocytes, decreasing extracellular glutamate clearance. Furthermore, in vivo studies showed that central acute FCt-mediated glial inhibition increased OT secretion; decreased salt intake and interestingly increased pressure and decreased respiratory frequency at rest. In addition, our data showed that central acute inhibition of glial cells decreased AVP secretion and salt intake induced by central ANG-II. Taken together, our results suggest that CVOs glial cells are important in integrating signaling which acts to modulate body fluid and cardiorespiratory homeostasis, including central effects induced by central ANG-II. A summary of our results are illustrated in the Graphical Abstract.
ANG II Effects in the Hypothalamic Astrocyte Culture Cells
Our in vitro findings indicated that ANG II decreased glutamate uptake in HACc, as indirectly assessed in the present study by [3H]-aspartate transport across the membrane. Studies by Boudaba et al. (2003) observed that dehydration increased SON postsynaptic excitatory currents (mEPSCs), which is mediated by a diminished astrocyte glutamate uptake. In addition, recent studies by Stern et al. (2016) showed that ANG II decreased PVN astrocyte glutamate currents. Taken together, these studies suggest that dehydration and ANG II decrease astrocyte glutamate transport, inducing an increase in the extracellular glutamate bioavailability, which in turn, contributes to an increase in neuronal activity (see Graphical Abstract).
Role of Circumventricular Glial Cells in the Neuroendocrine Responses Induced by Central ANG-II
The present data showed that FCt alone did not change AVP secretion. However, FCt pretreatment decreased central ANG-II-induced AVP release, suggesting that CVOs glial cells modulate AVP release driven by central ANG-II. This mechanism could be mediated by an ANG-II-induced decrease in astrocyte glutamate transport, leading to an increased glutamatergic neurotransmission, as suggested by Stern et al. (2016) and supported by our in vitro studies. Thus, here we suggest, as illustrated in the Graphical Abstract, that ANG-II induced AVP secretion is modulated by astrocyte-mediated extracellular glutamate clearance, which is impaired by FCt, an inhibitor of glial cell metabolism, leading to a decreased participation of these cells in glutamine-glutamate cycle (Paulsen et al., 1987, 1988a,b; Hassel et al., 1992). Next, we reported that central FCt microinjection induced alone a significant increase in OT secretion, suggesting that baseline activity of oxytocinergic neurons is under CVOs glial cells modulation. In agreement, studies by Di et al. (2013) showed that FCt promotes a significant reduction in post-synaptic inhibitory currents evoked in SON magnocellular neurons. Furthermore, it is known that OT neurons, under basal conditions, are inhibited by GABAergic inputs (Haam et al., 2012; Morton et al., 2014), whose post-synaptic activity is modulated by surrounding astrocytes (Potapenko et al., 2013). In light of exciting evidence, we hypothesized that under basal conditions, CVOs glial cells act to inhibit activity of hypothalamic oxytocinergic neurons. A number of studies have suggested that acute stimuli elucidate different, opposing mechanisms to modulate magnocellular vasopressinergic and oxytocinergic neurons (Haam et al., 2012; Di et al., 2013; Morton et al., 2014; Stachniak et al., 2014). Accordingly, central acute FCt attenuated ANG-II induced AVP secretion, as discussed above, but did not change ANG-II induced OT secretion, suggesting CVOs glial cells are important to modulate ANG-II-induced AVP secretion, but are not critical to modulate ANG-II-induced OT secretion.
Role of Circumventricular Glial Cells in the Behavioral Responses Induced by Central ANG-II
The present study provides additional evidence that central ANG-II promotes a significant increase in water and sodium intake in awake rats (Fitzsimons, 1980; Beresford and Fitzsimons, 1992; Saad et al., 2004; Reis et al., 2007, 2010; Matsuda et al., 2016). ANG-II receptors are strongly expressed in CVOs (Lenkei et al., 1997; Gebke et al., 1998), especially in the SFO, the main site involved in the control of sodium and water intake.
Despite the abundance of glial cells processes surrounding SFO neurons (Watanabe et al., 2006), the relationship between these glial cells in modulating drinking behavior is currently poorly understood. Our results suggest that CVOs glial cells are not involved in modulating water intake, but seem to be involved in ANG-II-induced sodium intake. Supported by our in vitro findings, and accordingly to the report of Stern et al. (2016), we suggest that central ANG-II-induced sodium intake could be modulated by astrocyte-mediated extracellular glutamate clearance (see Graphical Abstract). Another explanation was postulated by Noda's group; in a set of elegant studies, they showed that SFO glial cells, through Na+ sensitive channels (Nax), are involved in sodium intake (Watanabe et al., 2006; Shimizu et al., 2007; Matsuda et al., 2016). However, it appears that CVOs glial cells are associated with an inhibitory mechanism, which acts to modulate sodium intake, since elevated extracellular Na+ concentration increased firing of GABAergic interneurons in the SFO of wild-type, but not of Nax-Knockout mice (Shimizu et al., 2007; Matsuda et al., 2016). Additionally, in a different study, the same group showed that lactate released by sodium-sensitive astrocytes (Nax-positive) increased local GABAergic interneuron activity, which seems to be involved in the inhibitory modulatory pathway driving sodium intake in dehydrated mice (Watanabe et al., 2006; Shimizu et al., 2007; Matsuda et al., 2016). As previously described, Zielke et al. (2007) reported that FCt microdialysis into the hippocampus of freely moving rats considerably increased interstitial lactate levels. In that regard, we also hypothesize that sodium intake induced by central ANG-II could be impaired by FCt-induced increased interstitial lactate. However, interaction of ANG-II, lactate and CVOs glial cells in modulating drinking behavior needs to be further investigated.
Role of Circumventricular Glial Cells in the Cardiorespiratory Responses Induced by ANG-II
Our study provides additional evidence that central ANG-II induces the pressor responses in awake rats, as previously described (Saad et al., 2004; Reis et al., 2010). In addition, pressor response induced by central ANG-II seems to be modulated by SFO angiotensinergic neurons projecting to pre-sympathetic PVN neurons (Bains et al., 1992; Bains and Ferguson, 1994). Bains et al. (1992) observed that PVN AT1R antagonism attenuated a pressor response induced by SFO electrical stimulation. In addition, our results show that CVOs glial inhibition induces a pressor response in awake rats. In that regard, blockade of PVN astrocyte glutamate transporter increased renal sympathetic outflow and induced a pressor response in anesthetized rats (Bardgett et al., 2014; Stern et al., 2016). Furthermore, our results showed that central FCt did not change the ANG-II induced pressor response in awake rats, suggesting that CVOs glial cells are not critical to modulate central ANG-II induced pressor response. On the other hand, studies by Stern et al. (2016), focused on PVN pre-sympathetic neurons, indicated that ANG-II-induced sympathoexcitation and pressor responses are mediated by astrocyte-neuron interactions in the PVN. Thus, although our studies have shown that CVOs glial cells are not critical to modulate central ANG-II pressor response, those cells appear to play a critical role in mediating cardiovascular homeostasis.
Next, we observed that central ANG-II did not promote significant changes in respiratory frequency rate, tidal volume or ventilation in freely-moving awake rats. Studies by Zubcevic et al. (2013) have shown that icv ANG-II infusion (for 7 days) changed the pattern of phrenic nerve activity during the post-inspiration phase (PI or stage I expiration), typical of sympathetic-respiratory coupling, demonstrating that chronic activation of angiotensinergic receptors in CVOs cells seems to be involved in the sympathetic-respiratory coupling which promotes changes in the respiratory pattern. It is important to note that chronic ANG-II activation in these hypothalamic regions increases the expression of AT1R, thereby increasing plasma ANG-II sensitivity (Wei et al., 2009). Concerning that, central ANG-II-induced ventilatory changes could be observed in pathological conditions. Supporting our finding, studies by Walker and Jennings (1998) did not observe changes in ventilation promoted by intravenous ANG-II in awake rats. Considering that ANG-II appears to exert ventilatory effects via different mechanisms, our results suggest that central ANG-II is not involved in baseline respiratory outflow in awake rats. Our data showed that FCt-inhibited CVOs glial cells attenuate respiratory frequency at rest, which could be mediated by a decreased glutamine-glutamate cycle (Paulsen et al., 1987, 1988a,b; Hassel et al., 1992). Indeed, many other studies have suggested that brainstem astrocytes are involved in the respiratory network (Gourine et al., 2005; Erlichman et al., 2010; Sobrinho et al., 2017). Our findings provide new evidence supporting that CVOs glial cells are involved in the maintenance of breathing control at rest, in awake rats. However, the interaction between CVOs glial cells and the respiratory brainstem nucleus remains to be fully evaluated.
In summary, our in vitro findings showed that ANG-II decreased glutamate uptake in HACc. In addition, our in vivo findings suggest that CVOs glial cells modulate OT secretion, blood pressure and breathing at rest. Furthermore, CVOs glial cells are important in mediating ANG-II induced salt intake behavior and AVP secretion, which we propose is modulated by ANG-II inhibited glutamate uptake by surrounding CVOs astrocytes.
Author Contributions
AF, JC: designed experiments; AF, JC, SR: performed and analyzed experiments; AF, JC: wrote the manuscript; JdBA, CB, MF-S, LE, SR, JA-R, and VB: review the manuscript.
Funding
This work was supported by Conselho Nacional de Desenvolvimento Científico e Tecnológico (CNPq 447690/2014-0) and Coordenação de Aperfeiçoamento de Pessoal de Nível Superior (CAPES 8888.1.062209/2014.1).
Conflict of Interest Statement
The authors declare that the research was conducted in the absence of any commercial or financial relationships that could be construed as a potential conflict of interest.
Acknowledgments
The authors thank Clênia de Oliveira Cavalcanti, Sara Alves Luceno Madeiro, Milene Mantovani, and Maria Valci Santos for their technical assistance.
Abbreviations
ANG-II, Angiotensin II; HACc, Astrocyte culture cells; CVOs, Circumventricular organs; EAAT, Excitatory amino acid transporter; FCt, Fluorocitrate; GLT1, Glial-specific glutamate transporters; GLAST, Glutamate aspartate transporter; HR, Heart rate; icv, Intracerebroventricular; MAP, Mean arterial pressure; VM, Minute Volume; OVLT, Organum vasculosum of the lamina terminalis; OT, Oxytocin; PVN, Paraventricular nuclei; PAP, Pulsatile arterial pressure; fR, Respiratory frequency; SFO, Subfornical organ; VT, Tidal volume; AVP, Vasopressin.
References
Alexander, I. E., and Lumbers, E. R. (1981). The effects of angiotensin on respiratory patterns of anaesthetized dogs. Respir. Physiol. 46, 261–270. doi: 10.1016/0034-5687(81)90126-2
Bains, J. S., and Ferguson, A. V. (1994). Angiotensin II neurotransmitter actions in paraventricular nucleus are potentiated by a nitric oxide synthase inhibitor. Regul. Pept. 50, 52–59. doi: 10.1016/0167-0115(94)90191-0
Bains, J. S., Potyok, A., and Ferguson, A. V. (1992). Angiotensin II actions is paraventricular nucleus: functional evidence for neurotransmitter role in efferents originating in subfornical organ. Brain Res. 599, 223–229. doi: 10.1016/0006-8993(92)90395-P
Bardgett, M. E., Chen, Q.-H., Guo, Q., Calderon, A. S., Andrade, M. A., and Toney, G. M. (2014). Coping with dehydration: sympathetic activation and regulation of glutamatergic transmission in the hypothalamic PVN. Am. J. Physiol. Regul. Integr. Comp. Physiol. 306, R804–R813. doi: 10.1152/ajpregu.00074.2014
Bartlett, D., and Tenney, S. M. (1970). Control of breathing in experimental anemia. Respir. Physiol. 10, 384–395. doi: 10.1016/0034-5687(70)90056-3
Beresford, M. J., and Fitzsimons, J. T. (1992). Intracerebroventricular angiotensin II-induced thirst and sodium appetite in rat are blocked by the AT1 receptor antagonist, Losartan (DuP 753), but not by the AT2 antagonist, CGP 42112B. Exp. Physiol. 77, 761–764. doi: 10.1113/expphysiol.1992.sp003643
Boudaba, C., Linn, D. M., Halmos, K. C., and Tasker, J. G. (2003). Increased tonic activation of presynaptic metabotropic glutamate receptors in the rat supraoptic nucleus following chronic dehydration. J. Physiol. 551, 815–823. doi: 10.1113/jphysiol.2003.042739
Burmeister, M. A., Young, C. N., Braga, V. A., Butler, S. D., Sharma, R. V., and Davisson, R. L. (2011). In vivo bioluminescence imaging reveals redox-regulated activator protein-1 activation in paraventricular nucleus of mice with renovascular hypertension. Hypertension 57, 289–297. doi: 10.1161/HYPERTENSIONAHA.110.160564
Costa, K. M., Moraes, D. J. A., and MacHado, B. H. (2013). Acute inhibition of glial cells in the NTS does not affect respiratory and sympathetic activities in rats exposed to chronic intermittent hypoxia. Brain Res. 1496, 36–48. doi: 10.1016/j.brainres.2012.12.003
Cristovao-Ferreira, S., Navarro, G., Brugarolas, M., Perez-Capote, K., Vaz, S. H., Fattorini, G., et al. (2011). Modulation of GABA transport by adenosine A1R-A2AR heteromers, which are coupled to both Gs- and Gi/o-proteins. J. Neurosci. 31, 15629–15639. doi: 10.1523/JNEUROSCI.2526-11.2011
Di, S., Popescu, I. R., and Tasker, J. G. (2013). Glial control of endocannabinoid heterosynaptic modulation in hypothalamic magnocellular neuroendocrine cells. J. Neurosci. 33, 18331–18342. doi: 10.1523/JNEUROSCI.2971-12.2013
Elias, L. L. K., Antunes-Rodrigues, J., and Moreira, A. C. (1997). Release of plasma atrial natriuretic peptide after volume expansion is not related to pituitary-adrenal axis diurnal variation in normal subjects. Braz. J. Med. Biol. Res. 30, 621–625. doi: 10.1590/S0100-879X1997000500008
Erlichman, J. S., Leiter, J. C., and Gourine, A. V. (2010). ATP, glia and central respiratory control. Respir. Physiol. Neurobiol. 173, 305–311. doi: 10.1016/j.resp.2010.06.009
Ferguson, A. V. (1988). Paraventricular nucleus neurons projecting to the dorsomedial medulla are influenced by systemic angiotensin. Brain Res. Bull. 20, 197–201. doi: 10.1016/0361-9230(88)90179-7
Fitzsimons, J. T. (1980). Thirst and sodium appetite. Endeavour 4, 97–101. doi: 10.1016/0160-9327(80)90055-1
Frankmann, S. P. (1986). A technique for repeated sampling of CSF from the anesthetized rat. Physiol. Behav. 37, 489–493. doi: 10.1016/0031-9384(86)90211-8
Gebke, E., Müller, A. R., Jurzak, M., and Gerstberger, R. (1998). Angiotensin II-induced calcium signalling in neurons and astrocytes of rat circumventricular organs. Neuroscience 85, 509–520. doi: 10.1016/S0306-4522(97)00601-5
Gourine, A. V., Llaudet, E., Dale, N., and Spyer, K. M. (2005). Release of ATP in the ventral medulla during hypoxia in rats: role in hypoxic ventilatory response. J. Neurosci. 25, 1211–1218. doi: 10.1523/JNEUROSCI.3763-04.2005
Gross, P. M., Blasberg, R. G., Fenstermacher, J. D., and Patlak, C. S. (1987). The microcirculation of rat circumventricular organs and pituitary gland. Brain Res. Bull. 18, 73–85. doi: 10.1016/0361-9230(87)90035-9
Haam, J., Popescu, I. R., Morton, L. A., Halmos, K. C., Teruyama, R., Ueta, Y., et al. (2012). GABA is excitatory in adult vasopressinergic neuroendocrine cells. J. Neurosci. 32, 572–582. doi: 10.1523/JNEUROSCI.3826-11.2012
Haanwinckel, M. A., Eliast, L. K., Favaretrot, A. L. V., Gutkowskat, J., McCann, S. M., and Antunes-Rodrigues, J. (1995). Oxytocin mediates atrial natriuretic peptide release and natriuresis after volume expansion in the rat. Proc. Natl. Acad. Sci. U.S.A. 92, 7902–7906. doi: 10.1073/pnas.92.17.7902
Hassel, B., Paulsen, R. E., Johnsen, A., and Fonnum, F. (1992). Selective inhibition of glial cell metabolism in vivo by fluorocitrate. Brain Res. 576, 120–124. doi: 10.1016/0006-8993(92)90616-H
Kavanaugh, M. P. (2009). “Glial glutamate transporters: electrophysiology,” in Encyclopedia of Neuroscience, ed L. R. Squire (Academic Press), 805–809. doi: 10.1016/B978-008045046-9.01716-2
Lang, R. E., Rascher, W., Heil, J., Unger, T., Wiedemann, G., and Ganten, D. (1981). Angiotensin stimulates oxytocin release. Life Sci. 29, 1425–1428. doi: 10.1016/0024-3205(81)90005-9
Lenkei, Z., Palkovits, M., Corvol, P., and Llorens-Cortès, C. (1997). Expression of angiotensin type-1 (AT1) and type-2 (AT2) receptor mRNAs in the adult rat brain: a functional neuroanatomical review. Front. Neuroendocrinol. 18, 383–439. doi: 10.1006/frne.1997.0155
Li, Z., and Ferguson, A. V. (1993). Subfornical organ efferents to paraventricular nucleus utilize angiotensin as a neurotransmitter. Am. J. Physiol. 265, R302–R309. doi: 10.1152/ajpregu.1993.265.2.R302
Malan, A. (1973). Ventilation measured by body plethysmography in hibernating mammals and in poikilotherms. Respir. Physiol. 17, 32–44. doi: 10.1016/0034-5687(73)90108-4
Matsuda, T., Hiyama, T. Y., Niimura, F., Matsusaka, T., Fukamizu, A., Kobayashi, K., et al. (2016). Distinct neural mechanisms for the control of thirst and salt appetite in the subfornical organ. Nat. Neurosci. 20, 230–241. doi: 10.1038/nn.4463
McCarthy, K. D., and De Vellis, J. (1980). Preparation of separate astroglial and oligodendroglial cell cultures from rat cerebral tissue. J. Cell Biol. 85, 890–902. doi: 10.1083/jcb.85.3.890
Mirsattari, S. M., Shen, B., Leung, L. S., and Rajakumar, N. (2008). A gliotoxin model of occipital seizures in rats. Seizure 17, 483–489. doi: 10.1016/j.seizure.2007.12.008
Morton, L. A., Popescu, I. R., Haam, J., and Tasker, J. G. (2014). Short-term potentiation of GABAergic synaptic inputs to vasopressin and oxytocin neurones. J. Physiol. 19, 4221–4233. doi: 10.1113/jphysiol.2014.277293
Ohtake, P., and Jennings, D. (1993). Angiotensin II stimulates respiration in awake dogs and antagonizes baroreceptor inhibition. Respir. Physiol. 91, 335–351. doi: 10.1016/0034-5687(93)90110-V
Paulsen, R. E., Contestabile, A., Villani, L., and Fonnum, F. (1987). An in vivo model for studying function of brain tissue temporarily devoid of glial cell metabolism: the use of fluorocitrate. J. Neurochem. 48, 1377–1385. doi: 10.1111/j.1471-4159.1987.tb05674.x
Paulsen, R. E., Contestabile, A., Villani, L., and Fonnum, F. (1988a). The effect of fluorocitrate on transmitter amino acid release from rat striatal slices. Neurochem. Res. 13, 637–641. doi: 10.1007/BF00973281
Paulsen, R. E., Odden, E., and Fonnum, F. (1988b). Importance of glutamine for gamma-aminobutyric acid synthesis in rat neostriatum in vivo. J. Neurochem. 51, 1294–1299. doi: 10.1111/j.1471-4159.1988.tb03099.x
Paxinos, G., and Watson, C. (1997). The Rat Brain in Stereotaxic Coordinates, 3rd Edition. San Diego, CA: Academic Press.
Pegg, C. C., He, C., Stroink, A. R., Kattner, K. A., and Wang, C. X. (2010). Technique for collection of cerebrospinal fluid from the cisterna magna in rat. J. Neurosci. Methods 187, 8–12. doi: 10.1016/j.jneumeth.2009.12.002
Pérez-Domínguez, M., Hernández-Benítez, R., Peña Segura, C., and Pasantes-Morales, H. (2014). Thrombin-facilitated efflux of d-[3H]-aspartate from cultured astrocytes and neurons under hyponatremia and chemical ischemia. Neurochem. Res. 39, 1219–1231. doi: 10.1007/s11064-014-1300-8
Potapenko, E. S., Biancardi, V. C., Zhou, Y., and Stern, J. E. (2013). Astrocytes modulate a postsynaptic NMDA-GABAA-receptor crosstalk in hypothalamic neurosecretory neurons. J. Neurosci. 33, 631–640. doi: 10.1523/JNEUROSCI.3936-12.2013
Potter, E. K., and McCloskey, D. I. (1979). Respiratory stimulation by angiotensin II. Respir. Physiol. 36, 367–373. doi: 10.1016/0034-5687(79)90048-3
Reis, W. L., Giusti-Paiva, A., Ventura, R. R., Margatho, L. O., Gomes, D. A., Elias, L. L. K., et al. (2007). Central nitric oxide blocks vasopressin, oxytocin and atrial natriuretic peptide release and antidiuretic and natriuretic responses induced by central angiotensin II in conscious rats. Exp. Physiol. 92, 903–911. doi: 10.1113/expphysiol.2007.037911
Reis, W. L., Saad, W. A., Camargo, L. A., Elias, L. L., and Antunes-Rodrigues, J. (2010). Central nitrergic system regulation of neuroendocrine secretion, fluid intake and blood pressure induced by angiotensin-II. Behav. Brain Funct. 6:64. doi: 10.1186/1744-9081-6-64
Zielke, H. R., Zielke, C. L., Baab, P. J., and Tyson Tildon, J. (2007). Effect of fluorocitrate on cerebral oxidation of lactate and glucose in freely moving rats. J. Neurochem. 101, 9–16. doi: 10.1111/j.1471-4159.2006.04335.x
Roncari, C. F., David, R. B., De Paula, P. M., Colombari, D. S. A., De Luca, L. A., Colombari, E., et al. (2017). The lateral parabrachial nucleus and central angiotensinergic mechanisms in the control of sodium intake induced by different stimuli. Behav. Brain Res. 333, 17–26. doi: 10.1016/j.bbr.2017.06.020
Saad, W. A., de Arruda Camargo, L. A., Guarda, I. F. M. S., Dos Santos, T. A., Guarda, R. S., Saad, W. A., et al. (2004). Interaction between supraoptic nucleus and septal area in the control of water, sodium intake and arterial blood pressure induced by injection of angiotensin II. Pharmacol. Biochem. Behav. 77, 667–674. doi: 10.1016/j.pbb.2004.01.013
Sakai, K., Agassandian, K., Morimoto, S., Sinnayah, P., Cassell, M. D., Davisson, R. L., et al. (2007). Local production of angiotensin II in the subfornical organ causes elevated drinking. J. Clin. Invest. 117, 1088–1095. doi: 10.1172/JCI31242
Schlenker, E., Barnes, L., Hansen, S., and Martin, D. (2001). Cardiorespiratory and metabolic responses to injection of bicuculline into the hypothalamic paraventricular nucleus (PVN) of conscious rats. Brain Res. 895, 33–40. doi: 10.1016/S0006-8993(01)02011-X
Schousboe, A., Sarup, A., Bak, L. K., Waagepetersen, H. S., and Larsson, O. M. (2004). Role of astrocytic transport processes in glutamatergic and GABAergic neurotransmission. Neurochem. Int. 45, 521–527. doi: 10.1016/j.neuint.2003.11.001
Shimizu, H., Watanabe, E., Hiyama, T. Y., Nagakura, A., Fujikawa, A., Okado, H., et al. (2007). Glial nax channels control lactate signaling to neurons for brain [Na+] sensing. Neuron 54, 59–72. doi: 10.1016/j.neuron.2007.03.014
Sobrinho, C., Gonçalves, C. M., Takakura, A. C., Mulkey, D. K., and Moreira, T. S. (2017). Fluorocitrate-mediated depolarization of astrocytes in the retrotrapezoid nucleus stimulates breathing. J. Neurophysiol. 118, 1690–1697. doi: 10.1152/jn.00032.2017
Stachniak, T. J., Trudel, E., and Bourque, C. W. (2014). Cell-specific retrograde signals mediate antiparallel effects of angiotensin II on osmoreceptor afferents to vasopressin and oxytocin neurons. Cell Rep. 8, 355–362. doi: 10.1016/j.celrep.2014.06.029
Stern, J. E., Son, S., Biancardi, V. C., Zheng, H., Sharma, N., and Patel, K. P. (2016). Astrocytes contribute to angiotensin II stimulation of hypothalamic neuronal activity and sympathetic outflow. Hypertension 68, 1483–1493. doi: 10.1161/HYPERTENSIONAHA.116.07747
Walker, J. K., and Jennings, D. B. (1998). Ventilatory and metabolic effects of hypercapnia in conscious rats: AVP V1 receptor block. Can. J. Physiol. Pharmacol. 76, 361–366. doi: 10.1139/y98-039
Watanabe, E., Hiyama, T. Y., Shimizu, H., Kodama, R., Hayashi, N., Miyata, S., et al. (2006). Sodium-level-sensitive sodium channel Na(x) is expressed in glial laminate processes in the sensory circumventricular organs. Am. J. Physiol. Regul. Integr. Comp. Physiol. 290, R568–R576. doi: 10.1152/ajpregu.00618.2005
Wei, S.-G., Yu, Y., Zhang, Z.-H., and Felder, R. B. (2009). Angiotensin II upregulates hypothalamic AT1 receptor expression in rats via the mitogen-activated protein kinase pathway. Am. J. Physiol. Heart Circ. Physiol. 296, H1425–H1433. doi: 10.1152/ajpheart.00942.2008
Keywords: central ANG-II, glial cells, pressor response, neuroendocrine response, drinking behavior
Citation: Flôr AFL, de Brito Alves JL, França-Silva MS, Balarini CM, Elias LLK, Ruginsk SG, Antunes-Rodrigues J, Braga VA and Cruz JC (2018) Glial Cells Are Involved in ANG-II-Induced Vasopressin Release and Sodium Intake in Awake Rats. Front. Physiol. 9:430. doi: 10.3389/fphys.2018.00430
Received: 09 February 2018; Accepted: 06 April 2018;
Published: 01 May 2018.
Edited by:
Joaquin Garcia-Estañ, Universidad de Murcia, SpainReviewed by:
Vicente Lahera, Complutense University of Madrid, SpainKathleen S. Curtis, Oklahoma State University Center for Health Sciences, United States
Copyright © 2018 Flôr, de Brito Alves, França-Silva, Balarini, Elias, Ruginsk, Antunes-Rodrigues, Braga and Cruz. This is an open-access article distributed under the terms of the Creative Commons Attribution License (CC BY). The use, distribution or reproduction in other forums is permitted, provided the original author(s) and the copyright owner are credited and that the original publication in this journal is cited, in accordance with accepted academic practice. No use, distribution or reproduction is permitted which does not comply with these terms.
*Correspondence: Josiane C. Cruz, am9zaWFuZWNydXpAY2Jpb3RlYy51ZnBiLmJy