- Department of Biology, Ecology and Earth Sciences, University of Calabria, Rende, Italy
Changes in environmental oxygen (O2) are naturally occurring phenomena which ectotherms have to face on. Many species exhibit a striking capacity to survive and remain active for long periods under hypoxia, even tolerating anoxia. Some fundamental adaptations contribute to this capacity: metabolic suppression, tolerance of pH and ionic unbalance, avoidance and/or repair of free-radical-induced cell injury during reoxygenation. A remarkable feature of these species is their ability to preserve a normal cardiovascular performance during hypoxia/anoxia to match peripheral (tissue pO2) requirements. In this review, we will refer to paradigms of hypoxia- and anoxia-tolerant teleost fish to illustrate cardiac physiological strategies that, by involving nitric oxide and its metabolites, play a critical role in the adaptive responses to O2 limitation. The information here reported may contribute to clarify the molecular and cellular mechanisms underlying heart vulnerability vs. resistance in relation to O2 availability.
Introduction
Molecular oxygen (O2) is essential for life. A limited O2 supply leads to tissue hypoxia which often results in organ damage.
When compared to terrestrial animals, water-breathing organisms are more likely to be exposed to wider temporal and spatial variations of O2 supply. This is largely due to the inherent properties of the water and to the rapid fluctuations in the pattern of O2 production and consumption (Nikinmaa et al., 2011). Several animal species are adapted to tolerate regular and often severe hypoxia. This is the case of various fish, frog, and turtle species that tolerate anoxia, and some snakes and insects that can endure severe hypoxia (Hermes-Lima and Zenteno-Savín, 2002). Teleost fish exhibit a very large spectrum of O2 sensitivity, moving from species showing an extraordinary ability to tolerate hypoxia and anoxia to species that dramatically suffer O2 deprivation. Among teleost fish, examples of hypoxia/anoxia resistance are the members of the cyprinid genus Carassius, such as the goldfish (Carassius auratus), and the crucian carp (Carassius carassius), which exhibit a striking capacity to survive and remain active for long periods under low O2, even tolerating anoxia (Bickler and Buck, 2007). This capacity is correlated with the ability to generate ethanol as anaerobic end-product, which is acid–base neutral, in contrast with the normal glycolytic end-product lactic acid. Among cyprinids, the zebrafish (Danio rerio) is characterized by a growth-dependent transition from hypoxia tolerance to sensitivity (Padilla and Roth, 2001). Its genome is fully sequenced and this is of benefit for studying the components of hypoxia-resistance pathways in fish.
Despite the different abilities of vertebrates to tolerate a limited O2 availability in the environment or in internal tissues, many studies in mammalian and non-mammalian models (see Fago and Jensen, 2015, for references) suggest a common set of concerted physiological responses. They basically include depression of O2 consumption rates, protection against oxidative damage, and, at least in air-breathing species, redistribution of blood flow into the circulation. All these responses require the activation of a complex network of intracellular cascades, such as those related to nitric oxide (NO) and its metabolites, nitrite and nitrate, that represent common signaling molecules able to control and coordinate the molecular circuits that sustain adaptive hypoxia-dependent physiological responses (Fago and Jensen, 2015).
The heart is a major target of hypoxia. As largely documented in mammals, O2 deprivation is accompanied by changes in cardiac muscle metabolism, reactivation of fetal gene programs and hypertrophy responses, modifications of the extracellular matrix composition, mitochondrial biogenesis and arrangement, as well as of the expression of intracellular effectors [i.e., the NO pathway, hypoxamiRs, Hypoxia Inducible Factor (HIF), etc.] (Fago and Jensen, 2015). A large number of studies on mammals have recognized the critical role of NO and its metabolites, in particular nitrite and S-nitrosothiols (SNO; formed when Cys thiols react with NO+), in the mechanisms which control the cardiac response to low O2 availability. This role has been recently extended to non-mammalian vertebrates, including fish, in which a growing body of evidence has documented the cardioprotective role of NO and its related nitrosative signals, under hypoxic challenges.
In this review we will illustrate the cardiac role of the nitric oxide synthase (NOS)/NO/nitrite system with emphasis to hypoxia-activated cardio-protective effects of nitrite, a major source of NO under low O2. We will mainly provide information on the cardiac nitrosative signaling of teleost species characterized by a high ability to tolerate hypoxia/anoxia. This feature makes them valuable models to whom deserve a renewed attention to explore the mechanisms that contribute to survive low O2, also in a translational perspective for human cardioprotection. For the reader who is unfamiliar with the adaptive physiology of fish, we will outline their phenotypic plasticity in relation to anoxia and hypoxia, and to the different mechanisms that allow O2 detection. In the subsequent adaptive response, the heart, as a major effector, is modulated to properly sustain organism requirements.
Environmental Stress Response in Vertebrates
Many vertebrate species are able to face a wide range of environmental changes in abiotic parameters that include O2 availability, temperature, pH, and salinity. A common universal strategy characterizes the homeostatic response to different environmental stresses. This strategy requires important modifications from molecular to organismal level. An example is the response to hypoxia/anoxia that, as in case of the thermal stress, is characterized by a decrement in bioenergetic demand/production whose consequences are energy conservation, osmotic balance, and substrate economy (Boutilier, 2001 and references therein). The universal nature of the stress response is confirmed by genomic and post-genomic studies showing that the phenotypic flexibility of many species in response to hypoxia (as well as to hypothermia) involves the same pattern of genes that influence ATP and protein turnover, energy conservation, and stress factors release (Hochachka and Somero, 2002).
Two physiological adaptations confer the ability to cope with environmental stresses: “capacity adaptations” (i.e., the condition in which organisms preserve normal levels of both activity and homeostasis, enabling them “to grow and reproduce under the harsh conditions”) and “resistance adaptations” (i.e., the condition in which organisms enhance their resistance even losing homeostasis, enabling them “to avoid or survive the stress until conditions become favorable again”) (Cossins and Bowler, 1987). The reaction to stressors varies between individual members of a given species and represents a combination of factors, i.e., the appraisal of the environmental change and the ability to cope (Broom and Johnson, 1993; Koolhaas et al., 1999). As stated by Wilson et al. (1994), phenotypes within one species have a differential fitness and this differentiation represents adaptive individual differences in resource use and response to risk.
The different responses to stress can be clustered in two different phenotypes within the same species: proactive and reactive (Wilson et al., 1994). Proactive animals are characterized by high sympathetic and locomotor activity, while reactive individuals show low mobility and sympathetic activity (Van Raaij et al., 1996). Proactive individuals are usually audacious and more aggressive with respect to their reactive counterparts; these different activity levels between the two phenotypes have been observed both in wild (Montiglio et al., 2010) and laboratory environments (Tran and Gerlai, 2013). As observed in the teleost rainbow trout (Oncorhynchus mykiss) exposed to hypoxia, the proactive phenotype corresponds to an escaping and “non-surviving fish,” showing a strong avoidance behavior with a consequent high energy expenditure and instantaneous beginning of anaerobic metabolism. In contrast, the reactive fish remains quiet and survives thanks to the delaying activation of anaerobic mechanisms (Schjolden et al., 2005 and references therein).
Another trait of the adaptive response to low O2 is its relationship with animal development and growth. In general, it can be assumed that adults are less hypoxia-tolerant than neonatal and embryonic vertebrates (Crowder et al., 1998). If exposure to hypoxia occurs during juvenile or adult life, the effect is reversible. In contrast, if the stress takes place during the development, its influence persists throughout the life (Padilla and Roth, 2001).
Anoxia and Hypoxia Tolerance of the Fish Heart
Fish, as well as amphibians and reptiles, are characterized by an impressive ability to survive long period of partial and/or complete O2 deprivation. Three major adaptations allow these animals to face anoxia: deep metabolic depression, tolerance of acidosis and osmotic stress, prevention and/or restoration of cell damage induced by the huge radical production during re-oxygenation (see for review Driedzic and Gesser, 1994; Bickler and Buck, 2007). Moreover, long-term survival to anoxic stress requires massive accumulations of glycogen in critical tissues, and an extreme metabolic depression in specific body districts, and this allows the extension of anoxia tolerance to the whole organism (Hochachka, 1986).
Fish show a very high variety of phenotypes characterized by different abilities to cope with O2 fluctuations. At the basis of this plasticity is the expression of specific genes. Many investigations have recently attempted to identify these genes, and this resulted in the development of a database of Fish Hypoxia Responsive Genes (HRGFish) which currently covers 818 gene sequences and 35 genes (including HIF, myoglobin, and glucose transporters) from 38 fishes (Rashid et al., 2017).
The formidable adaptations shown by teleost fish to face low and very low O2 availability is typically related to the anaerobic capacity of the animal. This is exemplified by an early study by Mathur (1967) on the Indian cyprinid Rasbora daniconius showing that, if placed in a hermetically sealed glass jar, this teleost survives for more than 100 days. This resistance to protracted anoxia is supported by a notable anaerobic potential and the ability to escape from acidosis caused by the anaerobic waste-product increase (e.g., lactate).
Because of their very high resistance to minimum rates of water O2 saturation and a wide range of temperatures (from <4°C up to >38°C), Cyprinids, such as the common carp Cyprinus carpio, and its related specie, the crucian carp C. carassius, are largely recognized as major experimental models in which analyze morpho-functional adaptation to environmental or laboratory hypoxia. In these species, a significant metabolic down-regulation (down to 30%), which allows glycogen storing, is fundamental to surviving anoxia (Nilsson, 2001; Lutz and Nilsson, 2004).
Information on these fascinating models of hypoxia/anoxia resistance mainly comes from studies aimed to explore the mechanisms of defense of their hearts. Remarkable cardiac interspecific differences have emerged. In fact, while in the common carp, a critical decrease in heart function occurs during 24 h of severe hypoxia, the crucian carp conserves normal cardiac activity and autonomic cardiovascular control up to 5 days of anoxia at 8°C (Stecyk et al., 2004). This preserved cardiovascular function allows the crucian carp to effectively perfuse with blood both gills and liver. The appropriate perfusion mobilizes huge amount of glucose from the large hepatic glycogen store to all tissues, allowing, at the same time, lactate transport to the muscle where it is converted to ethanol (Nilsson, 2001). This, being less harmful than lactate, prevents acidosis. Additionally, the easily diffusible ethanol is quickly removed by the branchial epithelium thanks to the very effective blood perfusion. Accordingly, a preserved heart performance is the basis for improving anoxia resistance of the whole piscine organism thanks to the improved metabolic and functional cooperation among single organs (Figure 1). In this context, also the cooperation between different cardiac regions contributes to the response to low O2. In the cardiac ventricle of the bluefin tuna (Thunnus thynnus), the subendocardial trabeculae (spongiosa), essentially perfused by venous blood, are capable to metabolize lactate more than the compact subepicardial myocardium, which is completely perfused by oxygenated blood through the coronary vessels coming from the gills (Figure 2); this allows the spongiosa to face a reduced O2 availability (reviewed in Tota et al., 2011).
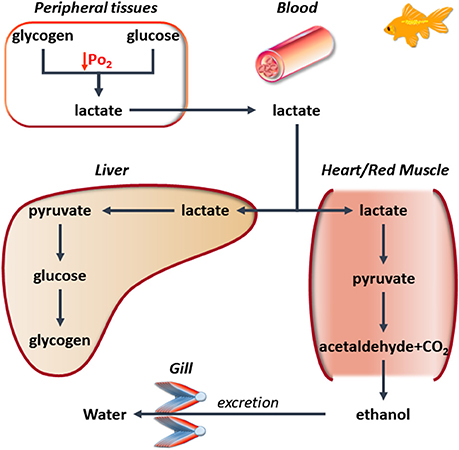
Figure 1. A simplified scheme illustrating the metabolic cooperation among different tissues in hypoxia-tolerant Cyprinid in the presence of low O2. Under these conditions, the muscle is able to activate the conversion of lactate (also produced by other organs) to ethanol. This is excreted by the gills, thus preventing lactate accumulation.
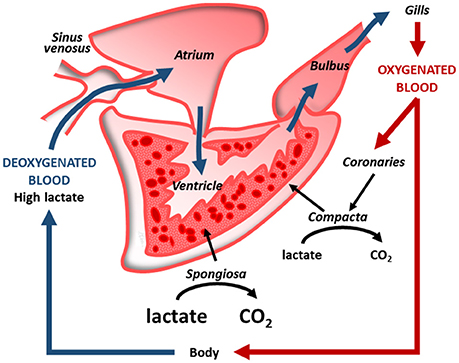
Figure 2. Intracardiac cooperation in a coronarized teleost heart (e.g., tuna). With respect to the compact layer, supplied with coronary oxygenated blood, the spongy layer, supplied by venous blood, has a greater capability to oxidize lactate to CO2 (modified from Tota et al., 2011 with permission).
The role of fuel substrates, in relation to the response to low O2 availability, has been also analyzed. Studies on the isolated and perfused eel heart show that glucose supply is essential for maintaining the cardiac performance during acute anoxic stress (see references in Imbrogno, 2013). In Anguilla rostrata, characterized by a marked anoxic endurance, if oxidative phosphorylation is blocked by perfusion with NaCN, endogenous glycogen stores are consumed regardless of the presence or absence of glucose in the medium (Bailey et al., 2000). Of note, down-regulation of oxidative phosphorylation is critical for heart endurance under protracted anoxia. As shown in the Carassius species, a strong metabolic depression is essential for the conservation of glycogen stores allowing fish to resist very long period of anoxia (Bickler and Buck, 2007; Vornanen and Haverinen, 2016).
During anoxia exposure, anoxia tolerant hearts show an in vivo cardiac ATP demand lower than their cardiac maximum glycolytic potential (i.e., the maximum ATP production solely from glycolysis) (Farrell and Stecyk, 2007). Two strategies, for achieving this have been proposed: a naturally low routine cardiac ATP demand that can be sustained by anaerobic glycolysis (as in the case of crucian carp, C. carassius), or a considerably down-regulation of cardiac ATP demand to a level that can be sustained by glycolytic ATP production (e.g., in C. carpio). In the case of the C. carpio, hypoxic bradycardia may represent a strategy to protect the heart since it allows the depression of cardiac power output and thus ATP requirement, reducing the need of oxygen for several hours (Farrell, 2007). In the case of C. carassius, during anoxia, anaerobic ATP production is sufficient to power the heart to pump, thus it is not necessary to activate bradycardia (Stecyk et al., 2004).
Oxygen-Sensing and Hypoxia/Anoxia Responses
Detection of environmental, hematic, or tissue O2 is a crucial task of the homeostatic response of vertebrates to hypoxia/anoxia. Complex and integrated systems and effectors are present in the different classes, phyla, and species, and even within the same species. A critical step is the activation of sensors which detect O2 during the initial, acute phase of hypoxia. As a consequence, catecholamines are secreted and the cardiovascular activity is modulated so that vascular tissue perfusion is adjusted to balance O2 supply and demand (Buckler, 2007; Milsom and Burleson, 2007). If the hypoxia persists, these mechanisms activate long-term responses that require the regulation of gene expression [i.e., the hypoxia inducible factor (HIF) transcription factor family] and this sustains and potentiates the initial response.
Detection of environmental and blood hypoxia relies on the activation of extremely specialized chemoreceptors. They are characterized by a very high sensitivity to small changes in PO2 in either the external environment, or the internal micro environment, depending on their position. They are strategically located in regions exposed to O2 fluctuations, as in the case of the neuroepithelial cells (NECs) of the gills, the neuroepithelial bodies of the airways, and the carotid bodies in the vasculature.
In fish, regardless their phyletic position, a major role in O2-sensing is attributed to branchial NECs whose location and orientation is not uniform across species. They can be distributed either across the gill arches, or outside the gills in the oro-branchial cavity (see Milsom, 2012 for references; Gilmour and Perry, 2007; Milsom and Burleson, 2007). In addition, NECs may be oriented externally or internally or both, and this provides the animals with a selective ability to detect PO2 variations in water, and blood, or in both environments (reviewed by Milsom, 2012). It is generally assumed that hypoxia-tolerant species responds primarily to arterial hypoxaemia, while less tolerant fish respond more immediately to aquatic hypoxia (Sundin et al., 2000).
Externally-oriented chemoreceptors represent a primitive O2 sensing mechanism that disappeared early in vertebrate evolution, since they are absent in the obligate air-breathing South American lungfish, Lepidosiren paradoxa (Sanchez et al., 2001). In this fish, only blood hypoxia, but not water hypoxia, induces a rapid ventilator response, suggesting a prevalent role of internal rather than of branchial receptors.
Branchial NECs are differently sensitive to low PO2 and this correlates with the degree of hypoxia tolerance/intolerance of the various species. For example, in the hypoxia tolerant goldfish, isolated NECs do not respond to low PO2 until severe hypoxic or even anoxic levels. An increased ventilation is observed only if goldfish are exposed at water PO2 that are lower (25 mmHg: Tzaneva et al., 2011) than those (110 mmHg) eliciting the ventilatory response in the hypoxia sensitive zebrafish (Vulesevic et al., 2006). This may be advantageous during prolonged environmental anoxia to avoid continuous stimulation of stimulation of chemoreceptors without the possibility of increasing O2 uptake.
An interesting aspect of fish O2-sensitivity is the relationship with development. As observed in zebrafish, animals may change from hypoxia/anoxia tolerance to hypoxia sensitivity during growth. The zebrafish embryo is initially anoxia-tolerant but becomes hypoxia-sensitive between 2 and 3 days post-fertilization (dpf), when it begins to show hyperventilation under hypoxia (Padilla and Roth, 2001; Mendelsohn et al., 2008). This suggests that the chemoreflex induced by hypoxia appears before gill NECs are fully functional, and is presumably mediated by extrabranchial chemoreceptors (Jonz et al., 2015).
Three Hypotheses for Oxygen-Sensing and Downstream Responses
Studies in mammals have proposed three hypotheses for O2 sensing: a “membrane hypothesis,” a “mitochondrial/metabolic hypothesis,” and a more recent recent “gasotransmitter hypothesis” (reviewed by Prabhakar and Peers, 2014; Dzal et al., 2015; López-Barneo et al., 2016). Once activated, all these mechanisms converge on K+ currents of chemoreceptors cells with consequent neurotransmitter release and activation of the physiological modulation of ventilation and perfusion to maintain homeostasis.
Membrane Hypothesis
In mammals, chemoreceptor-dependent O2 sensing relies on the modulation of plasmalemmal K+ channels. Different types of K+ channels are involved, including background K+ (KB) channels (Buckler, 1999), large conductance Ca2+-activated K+ currents (KCa) (Peers, 1990; Wyatt and Peers, 1995), TASK-like background K+ channels (Buckler, 2007), and kv3 and kv4 channels (Sanchez et al., 2002; Pérez-García et al., 2004). Modulation of these channels regulates membrane permeability and cell excitability, according to a common scheme for chemotransduction. Inhibition of the resting K+ current allows membrane depolarization, opening of voltage-dependent Ca2+ channels, neurotransmitter secretion and excitation of afferent neurons to cardio-respiratory centers (Nurse, 2005).
Available data suggest that also in fish, hypoxic chemotransduction involves O2-sensitive K+ currents, as supported by evidence in gill NECs of zebrafish (Jonz et al., 2004; Qin et al., 2010) and channel catfish (Ictalurus punctatus) (Burleson et al., 2006). While in zebrafish, the cell current appears to be mediated by background K+ (KB) channels (Jonz et al., 2004; Qin et al., 2010), similar to TASK-like background K+ channels of the mammalian carotid body (Buckler, 2007), in catfish the hypoxic response seems to be mediated by O2-sensitive voltage-dependent outward K+ (Kv) current (Burleson et al., 2006).
Variations in membrane Ca2+ fluxes and intracellular Ca2+ concentrations, with consequent stimulation of neurotransmitter release, are presumably also involved in fish O2 chemoreception (Jonz, 2014). However, while in both zebrafish and catfish, gill NECs express a small number of Ca2+-activated K+ channels (KCa) (Jonz et al., 2004; Burleson et al., 2006; Qin et al., 2010), the goldfish gill NECs express predominantly KCa channels, with a minor contribution of KB and Kv (Zachar and Jonz, 2012) and L-type Ca2+ channels (Zachar et al., 2017). These data suggest a species-specific expression of ion channels that, modulated as a consequence of PO2 changes, participate to O2 sensing.
Mitochondrial/Metabolic Hypothesis
Under hypoxia, the mitochondrial ATP generation is reduced and this affects many intracellular effectors, and thus a large spectrum of cell functions. Two major cellular events are proposed downstream the hypoxia-dependent ATP decrease. One is the inhibition of K+ TASK-like background channels. This may result in membrane depolarization and initiation of the electric activity and of voltage-dependent Ca2+ entry (Varas et al., 2007). The second is the increased cytosolic AMP/ATP ratio followed by the activation of AMP-activated protein kinase (AMPK). This, by inhibiting O2 sensitive K+ channels (KB and KCa) leads to chemoreceptors depolarization (Evans et al., 2005, 2009; Wyatt and Evans, 2007; Wyatt et al., 2007). In fish, no data are available concerning the involvement of mitochondria in O2 sensing. Only few data suggest a role for AMPK in the downstream response to anoxia of tolerant species. As observed in heart and brain of the tolerant crucian carp, AMPK induces metabolic depression and ethanol secretion only under anoxia, but not under hypoxia (Stensløkken et al., 2008), consistent with a quiescent kinase until complete anoxia is achieved (Pamenter, 2014). This may be an advantage for hypoxia-tolerant species since may allow to increase the depth and/or duration of hypoxia they can tolerate before the activation of AMPK-mediated metabolic adjustments becomes a necessity. In this way, protein synthesis, and other AMPK downregulated anabolic pathways, continue to function under hypoxia along with the ability to preferentially shunt blood flow to at-risk organs (e.g., brain and heart) (Pamenter, 2014).
Gasotransmitters Hypothesis
NO, Carbon Monoxide (CO), and Hydrogen sulfide (H2S), are mediators of O2 sensing in chemoreceptors. As shown in the mammalian carotid body, under normoxia, NO and CO are inhibitory, while H2S is excitatory. Regardless the type of response, their effects occur via ion channels modulation: NO causes glomus cell hyperpolarization by inhibiting Ca2+ channels and activating K+ channels; CO also activates these latter channels, preventing cell depolarization, while H2S inhibit them (for review see Prabhakar and Peers, 2014). Under hypoxia, both NO and CO production decrease, as their enzymatic activity requires molecular O2, leading to a reduced inhibition of L-type Ca2+ channels and to the closure of K+ channels. In contrast, H2S generation increases under hypoxia. This condition is associated with the inhibition of maxiK and TASK-like channels (see Prabhakar and Peers, 2014). All the above effects result in membrane potential depolarization, neurotransmitter release, and afferent neurons activation.
In fish, few data suggest a role for NO and CO in O2 chemoreception and in the control of breathing, while more robust information indicates that H2S is directly involved in O2 sensing and in the hypoxic response (Olson et al., 2008).
A NO-dependent O2-sensing mechanism has been proposed based on the identification of nNOS in neuroepithelial cells of adult and larvae of the zebrafish (Porteus et al., 2015). According to Perry and Tzaneva (2016), like in mammalian glomus cells (Summers et al., 1999), NO may inhibit ion channels involved in neurosecretion. This is supported by the NO-dependent regulation of intracellular Ca2+ observed in the melanophore of the Indian snakehead Channa punctatus (Biswas et al., 2011) and in the growth hormone release of goldfish (Chang et al., 2014). Apart from its putative involvement in O2 chemoreception, in teleosts, as well as in mammals, NO may play a role in the hypoxia-elicited response via a modulation of the ventilatory performance. This is supported by evidence in zebrafish that NO produced by nNOS expressed in branchial NECs, modulates the response to hypoxic stimuli by inhibiting and stimulating ventilation in adult and larvae, respectively (Porteus et al., 2015). As hereafter discussed, NO and its metabolites play a crucial role in the responses to O2 limitation, representing signaling molecules able to control and coordinate the molecular circuits that sustain adaptive hypoxia-dependent physiological responses.
In fish, as in mammals, O2 sensing involves the HO-dependent generation of CO. As observed in the goldfish, HO-1 (the hypoxia inducible isoform) is present in branchial NECs and its inhibitory effect on ventilation in goldfish acclimated to 7°C may reflect the HO-1-mediated production of CO within NECs (Tzaneva and Perry, 2014). The involvement of CO in the control of the ventilatory response has been also proposed by data in zebrafish, in which HO-1 expression in both larvae (skin NECs) and adult (gill NECs), is indicative of an endogenous CO production (Tzaneva and Perry, 2016). Once produced in branchial peripheral chemoreceptors, CO may affect the piscine ventilatory response via a modulation of intracellular Ca2+ and neurotransmitter release (Tzaneva and Perry, 2014), as in mammalian glomus cells (Overholt and Prabhakar, 1997; Prabhakar, 1999). This view is supported by the presence of both L-type Ca2+ and KCa channels in gill NECs of the goldfish which respond to hypoxia by increasing intracellular Ca2+ and synaptic vesicle activity (Zachar et al., 2017).
Several evidence in mammals indicate that H2S acts as an O2 sensor (Olson, 2011) and a mediator of hypoxic signaling (Peng et al., 2010). H2S generation, by constitutive cystathionine γ-lyase (CSE), cystathionine β-synthase (CBS), and 3-mercaptopyruvate sulfurtransferase (3-MST)/cysteine aminotransferase (CAT) (Kimura, 2011), is linked to O2 availability. In fact, it is present at low levels under normoxia, because of its oxidation by the mitochondrial electron transport chain enzymes, but increases under hypoxia, when the activity of the mitochondrial electron transport chain is reduced (Olson, 2011). In the carotid body, the mechanism involves a reduced activity of HO-2 which in turn increases CSE activity and thus H2S production; however, the role of CSE and H2S in the hypoxia sensing is not universally accepted. Recently, Wang et al. (2017) reported that in glomus cells from CSE−/− mice, hypoxia-dependent effects on TASK-like channels, intracellular calcium, and ventilation were not modified. Even if the study does not provide a role for CSE in acute hypoxia sensing, this cannot be excluded (Wang et al., 2017), also considering that CSE inhibition affects the hypoxia response in chronic pathological states (Yuan et al., 2016).
H2S plays a role in O2 sensing also in fish. The first evidence was obtained in rainbow trout and zebrafish where, under hypoxia, the gas initiates the cardiorespiratory response by promoting membrane depolarization of chemoreceptive NECs (Olson et al., 2008; Porteus et al., 2014). This is also supported by the identification of CBS and CSE in the gills of rainbow trout and zebrafish whose inhibition, or gene knockdown (in zebrafish larvae), abolishes or attenuates the hyperventilatory response to hypoxia (Porteus et al., 2014). In addition, under normoxia, Na2S (H2S donor) induces membrane depolarization of trout branchial NECs (Olson et al., 2008), and increases intracellular Ca2+ in zebrafish (Porteus et al., 2014). Also for this gasotransmitter, the mechanism appears similar to that described in mammals.
Cardiac Nitrosative Signals
Generation of NO and its Metabolites
In almost all animal tissues, NO is generated by the family of NOS isoenzymes [i.e., the constitutive endothelial (eNOS) and neuronal (nNOS), and the inducible (iNOS), isoforms], which convert L-arginine into L-citrulline and NO, in the presence of O2 and NADPH as essential cofactors. This reaction, because of the obligatory requirement for molecular O2, is vulnerable to hypoxia (Moncada and Higgs, 1993; Bryan, 2006; Lundberg et al., 2008).
NOS enzymes are the products of different genes, and show different localization, regulation, catalytic properties and inhibitor sensitivity (Pautz et al., 2010; Imbrogno et al., 2011). The constitutive NOSs produce, in the steady-state, nanomolar concentrations of NO. In contrast iNOS, if induced by immunologic and inflammatory stimuli, generates micromolar cytotoxic amounts of the gas (Vallance et al., 2000).
NO exerts its physiological effects by reversible binding and/or reacting with hemes, thiols or amines, forming iron-nitrosyl (FeNO), S-nitroso (SNO) and N-nitroso (NNO) compounds (Hill et al., 2010). NO has a very short half-life. It is rapidly metabolized to nitrite in reaction with O2 (Lundberg et al., 2008), and is inactivated by oxidation to nitrate in reaction with oxygenated hemoglobin (Hb) and myoglobin (Mb). NO can also react with O2, yielding peroxynitrite (ONOO−) (Ronson et al., 1999), and this depletes the bioactivity of the gas (Guzik et al., 2002). ONOO− itself is not only a signaling molecule, but also a highly reactive species (Pacher et al., 2007), being able to form additional types of reactive nitrogen species, including nitrogen dioxide (NO2) and dinitrogen trioxide (N2O3). All reactive nitrogen species are responsible for protein post-translational modifications because of either ability to induce either S-nitrosation [the formation of a covalent bond between an NO+ equivalent and a nucleophilic center (amine or thiol)], or S-nitrosylation [the addition of NO without changing the formal charge of the substrate (metal center or radical species)] (Heinrich et al., 2013). Often, S-nitrosation and S-nitrosylation are used interchangeably to refer to the same substrate modification, i.e., the process leading to S-nitrosothiols (SNO) formation within proteins (for specific chemical terminology, see Heinrich et al., 2013). Likely, an uncontrolled nitrosation/nitrosylation of cysteine residues may induce nitrosative stress, with important effects on proteins activity, stability, conformation and/or ability to interact with other molecules (Foster et al., 2009).
In the presence of a reduced O2 availability, when the conversion of L-arginine in L-citrulline and NO is compromised, nitrite can be reduced back to NO, providing an alternative pathway for gas generation (Gladwin et al., 2005; Lundberg et al., 2009; van Faassen et al., 2009). This NO regeneration occurs through acidic disproportionation (Zweier et al., 1999) and enzymatic reduction via xanthine oxidoreductase (Millar et al., 1998), mitochondrial enzymes (Kozlov et al., 1999; Castello et al., 2006), or deoxygenated Hb (Cosby et al., 2003; Nagababu et al., 2003), Mb (Shiva et al., 2007a) and neuroglobin (Petersen et al., 2008). Under anoxia, also eNOS is capable of reducing nitrite to NO (Gautier et al., 2006). Thus, in the presence of low O2, NO production is gradually taken over by nitrite reduction, nitrite functioning as a pool of NO availability (Lundberg et al., 2008; van Faassen et al., 2009; Angelone et al., 2012).
Also nitrate contributes to NO homeostasis since it can be slowly reduced to nitrite by the ubiquitous enzyme xanthine oxidoreductase (Jansson et al., 2008). The nitrate-nitrite-NO pathway may be considered complementary to the classical L-arginine-NOS pathway. All these pathways partly work in parallel, but when O2 availability is reduced and NOS activity is decreased, nitrite reduction to NO becomes more pronounced. Thus, according to the general concept of the NO cycle in mammals, first proposed by Reutov (Reutov, 2002), and ions are formed as a result of non-enzymatic/enzymatic NO oxidation: L-Arg => NO =>/; the reduction of ions to NO: + e- => NO takes place through the nitrite reductase reaction (reviewed in Tota et al., 2010). The NOS and nitrite-reductase component of the NO cycle are schematically reported in Figure 3.
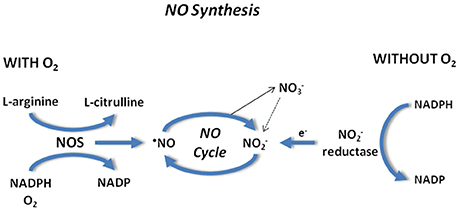
Figure 3. NO synthesis in the presence (Left) and absence (Right) of O2. Note NOS and nitrite reductase components involved in the NO formation.
The Cardiac NO Signaling in the Response to Hypoxia
Mammals
In mammals, the cardiac physiological role of NO in relation to O2 availability is well established. A hypoxia-dependent increase of NO helps mammalian myocardial cells to limit cardiac injury caused by low O2 supply. For example NO, by down-regulating O2 consumption rate, both competitively and uncompetitively inhibits O2 binding to mitochondrial cytochrome c oxidase (Mason et al., 2006; Erusalimsky and Moncada, 2007; Cooper et al., 2008). NO inhibition of cellular respiration mainly occurs at low O2 tensions; thus, particularly under hypoxia, NO may protect cellular functions by extending O2 availability (Hagen et al., 2003; Misfeldt et al., 2009). In oxygenated heart muscle from guinea pig, NO has been found to increase metabolic efficiency, determined by the coupling between myocardial O2 consumption and cardiac performance and the coupling between myocardial O2 consumption and ATP synthesis (Shen et al., 2001).
Of note, in the presence of ischemia, the NO-dependent suppression of the electron-transport chain, by reducing mitochondrial energy production, limits the cardiac damages induced by ischemia/reperfusion (IR) (Shiva et al., 2007b).
Fish
In fish, the role of NO as a major organizer of complex cardiac transduction signals has been widely assessed, and to date many data are available about its wide cardiac repertoire of actions (see for example Garofalo et al., 2009a, 2012; Imbrogno et al., 2010, 2013, 2017, 2018; Filice et al., 2017; Imbrogno and Cerra, 2017). Very recent studies extended the large variety of NO functions in fish to the mechanisms which allow to maintain the cardiovascular status and control the response to low O2. It is well established in fish that, if NOS activity is compromised by limited O2, an increased NOS expression or, alternatively, a nitrite reduction to NO, stabilize NO levels, and this contributes to protect the hypoxic myocardium (Hansen and Jensen, 2010; Sandvik et al., 2012; Imbrogno et al., 2014). A similar NOS enhanced expression can be also observed in the vasculature of the trout in response to hypoxia (McNeill and Perry, 2006).
Experimental evidences indicating the NO involvement in cardiac homeostasis of teleosts fish under hypoxic/anoxic conditions mainly derive from studies on the goldfish C. auratus, a champion of hypoxia tolerance. In the goldfish heart, NO inhibits mitochondrial respiration without affecting contractility (Pedersen et al., 2010). This increases myocardial efficiency (i.e., the force generated per O2 consumed), thus importantly contributing to maintain fish myocardial function in the presence of hypoxia or anoxia (Stecyk et al., 2004). In line with these observations, Imbrogno et al. (2014) showed that, during acute hypoxia, the goldfish heart enhances its basal performance, as well as the sensitivity to heterometric (i.e., Frank-Starling) regulation. This has been considered an important mechanism for maintaining functional and metabolic interactions between organs and tissues, required for the hypoxia tolerance of the organism. Interestingly, in the goldfish, exposure to hypoxia is accompanied by an increased myocardial NOS expression, pointing to NO generation as a crucial step for adjusting the cardiac function of the goldfish during hypoxic challenges (Imbrogno et al., 2014).
It has been also reported that the hypoxia-induced increase in NO production in goldfish heart could activate sarcolemmal KATP channels, a response that may enhance tolerance of hypoxia in this species (Cameron et al., 2003). This mechanism represents a point of convergence with the mammalian preconditioning protection of ischemic myocardium in which the opening of ATP-sensitive K+ channels is a crucial event (Noma, 1983).
Of note, in the hypoxic goldfish heart, the increased NOS expression is accompanied by an enhanced expression of HIF-1α. Such hypoxia-dependent cross-talk between NOS and HIF-1α represents an evolutionary conserved trait of the vertebrate response to low O2. In fact, in the mammalian heart, under hypoxic stress (i.e., during ischemia), HIF-1α activates a number of critical genes (Hochachka and Lutz, 2001; Liu and Simon, 2004; Semenza, 2007), including NOS (Jugdutt, 2002), and this contributes to cell survival. At the same time, high NO concentrations (>1 μM) stabilize HIF-1α (Mateo et al., 2003), thus increasing the dimeric form of the protein that, via binding to HIF responsive elements (HREs), promotes NOS expression (Mateo et al., 2003). Interestingly, in hypoxia-resistant fish, as in the case of the crucian carp, HIF-1α stabilization occurs even under normoxic conditions, suggesting a high basal expression of hypoxic-regulated genes, including NOS (Sollid et al., 2006).
As illustrated above, under hypoxic conditions, a significant source of NO is represented by nitrite. Compared to terrestrial animals, fish are exposed to an additional direct uptake of exogenous nitrite from the environmental water across the respiratory surfaces (Jensen, 2009). This external nitrite supply is an important source for the internal NO generation during severe hypoxia. An example is the crucian carp that, when exposed to deep hypoxia, takes up ambient nitrite across the gills and directs it to tissues, including the heart (Hansen et al., 2016).
In hypoxia-tolerant fish, such as the goldfish and the crucian carp, basal plasma nitrite levels are around 0.75–1.75 μM. These values are higher than those observed not only in mammals, but also in fish that are hypoxia intolerant (e.g., flounder, eelpout, oyster toadfish, brown trout) (about 0.2 μM) (Jensen, 2009; Hansen and Jensen, 2010; Sandvik et al., 2012). The reason for these high nitrite plasma levels in hypoxia-tolerant species is unclear. Presumably, this depends on the high overall NOS activity/expression under normoxia (Kleinbongard et al., 2003). Of note, fish living in nitrite-contaminated environments, have significantly high plasma nitrite, concentrations reaching the millimolar range (Bath and Eddy, 1980). At high concentrations, nitrite is toxic and can influence ion, respiratory and circulatory homeostasis (Jensen, 2009). As shown in the zebrafish, exposure to high nitrite is accompanied by very high levels of HbNO, a biomarker of NO generation from nitrate (Jensen, 2007). The consequent high nitrite-derived NO could perturb physiological processes, and may induce tissues nitrosative stress, resulting in high levels of S-nitrosylated proteins and cell damage (Jensen, 2009). For these reasons, fish need to balance the advantages of a rich ambient pool of nitrite for internal NO production with the potentially dangerous effects of nitrite-polluted habitats (Jensen and Hansen, 2011). At the same time, the possibility to maintain internal nitrite levels is particularly important for securing a source for NO production during hypoxia and anoxia, where NOS enzymes are unable to produce NO. At this purpose, the goldfish and the crucian carp possess an intrinsic ability to increase intracellular nitrite concentration and nitrosylated compounds during deep hypoxia and anoxia in tissues with high myoglobin and mitochondria content, such as the heart (Sandvik et al., 2012; Jensen et al., 2014; Hansen et al., 2016). This occurs at the expenses of extracellular nitrite. The extracellular-intracellular transfer of nitrite is facilitated by nitrite binding to intracellular proteins that, by keeping low the cytosolic concentration of free nitrite, allows inward diffusion (Hansen and Jensen, 2010). As shown in the crucian carp, anoxia increases tissue nitrite in the heart, but not in the white muscle. This is indicative of a role for Mb nitrite reductase activity, which is present at high levels in the heart and in the red musculature (Jensen et al., 2014).
Although no clear evidence is available, in fish, mitochondria may play a role in elevating intracellular nitrite during hypoxia and anoxia. Indeed, the cytoprotective effects of nitrite under low O2 are largely directed at the mitochondria (Walters et al., 2012; de Lima Portella et al., 2015). In mammalian mitochondria, nitrite S-nitrosates complex I, attenuating ROS generation during early reperfusion (Dezfulian et al., 2009; Chouchani et al., 2013), and nitrosylates complex IV, which inhibits O2 consumption rates (Hendgen-Cotta et al., 2008). Unlike mammals, in hypoxia-tolerant ectotherms, as presumably in all ectotherms, reoxygenation does not affect mitochondria, which maintain their capacity to produce energy. For example, reoxygenation does not result in mitochondria Ca2+ overload and/or in reversing ATP-synthase into an ATPase (Galli and Richards, 2014 for references). At the same time, mitochondrial proton leak is kept low (Galli and Richards, 2014 for references). With respect to hypoxia-intolerant, in hypoxia-tolerant species, mitochondria respiration appears more resistant to hypoxic stresses. For example, among elasmobranchs, cardiac mitochondria from the hypoxia-tolerant epaulet shark (Hemiscyllium ocellatum) produce less reactive oxidative species than the hypoxia sensitive shovelnose ray (Aptychotrema rostrata) (Hickey et al., 2012). For this purpose, the dynamic organization of respiratory chain complexes and ATP synthase (Cogliati et al., 2016) results crucial for mitochondrial respiration under hypoxia.
Unique natural animal models to analyse the role of NO, nitrite and Mb in the response to hypoxia are Antarctic teleosts. Some of them are example of disaptation, being naturally deprived of Hb, and/or of cardiac Mb, as in the case of the icefish Chionodraco hamatus (Hb−/Mb+), Chenocephalus aceratus (Hb−/Mb−), and their red-blooded counterparts Trematomus bernacchii (Hb+/Mb+) (Garofalo et al., 2009b). This condition makes these teleosts well suited for comparatively studying evolutionary and mechanistic aspects of the NO-nitrite system in relation to cardiac homeostasis and adaptation, including the response to varying O2 levels (see for references Garofalo et al., 2009b). This aspect is crucial in the stably ice Antarctic waters, which are highly oxygenated but, at the same time, exposed to changes in O2 content. At the moment, no data are available in Antarctic teleost on the putative role of the NO/nitrite equilibrium in relation to the response to low O2. In the heart of C. hamatus and T. bernacchii, under normoxia, nitrite influences cardiac performance by inducing a concentration-dependent increase of contractility (Garofalo et al., 2015). In the Antarctic Hb- and Mb-less (Hb−/Mb−) icefish C. aceratus, intracardiac NOS expression is lower than in its Mb+ counterpart, the Hb−/Mb+ C. hamatus (Amelio et al., 2006). Compared to the Mb expressing C. hamatus, in C. aceratus the heart is more sensitive to NOS stimulation by L-arginine (Cerra et al., 2009). Since the nitrite reductase activity of cardiac Mb is absent and NOS is poorly expressed, other mechanisms have been proposed to contribute to local NO production. For example, in the absence of the Mb-mediated scavenging effect, NO half-life is increased; the consequent larger availability of free NO may compensate for the reduced NOS expression. Contrarily, in C. hamatus, cardiac Mb may contribute to local NO generation and this maintains the nitrergic homeostasis. Interestingly, in C. hamatus, very low concentrations of exogenous nitrite (0.1 μmol/l) increase cardiac contractility, an effect similar to that elicited by NO (Cerra et al., 2009). At the same time, as in mammals (Vanin et al., 2007), the largely expressed NOS equipment might represent a rich source of NO from nitrite. Of note, in Hb−/Mb− icefish, the high NO levels occurring in the absence of both respiratory proteins correlate with major cardiovascular and subcellular compensations, including mitochondrial enlargement within myocardiocytes (Urschel and O'Brien, 2008). This contributes to myocardial oxidative equilibrium and hence to heart protection under hypoxia.
Conclusions
This review emphasizes the amazing flexibility of teleost fish in relation to their peculiar ability to cope with low O2. Teleosts possess complex equipments for sensing O2 that activate intricate downstream molecular signal-transduction networks crucial to balance tissue O2 supply and demand. Although much still remains to be investigated, the available information indicate the critical role played by NO and its metabolites in the physiological strategies that in teleost allow cardiac adaptive responses to O2 limitation, also contributing to better understand the extraordinary morphofunctional plasticity and adaptation that determined their evolutionary success.
Author Contributions
AG and FG participated in drafting, writing, and editing the manuscript. MCC and SI participated in writing and editing the manuscript. All Authors approved it for publication.
Funding
This work was supported by the MIUR of Italy (ex 60%) and the Dottorato di Ricerca in Scienze della Vita.
Conflict of Interest Statement
The authors declare that the research was conducted in the absence of any commercial or financial relationships that could be construed as a potential conflict of interest.
References
Amelio, D., Garofalo, F., Pellegrino, D., Giordano, F., Tota, B., and Cerra, M. C. (2006). Cardiac expression and distribution of nitric oxide synthases in the ventricle of the cold-adapted Antarctic teleosts, the hemoglobinless Chionodraco hamatus and the red-blooded Trematomus bernacchii. Nitric Oxide 15, 190–198. doi: 10.1016/j.niox.2005.12.007
Angelone, T., Gattuso, A., Imbrogno, S., Mazza, R., and Tota, B. (2012). Nitrite is a positive modulator of the Frank-Starling response in the vertebrate heart. Am. J. Physiol. 302, R1271–R1281. doi: 10.1152/ajpregu.00616.2011
Bailey, J. R., Rodnick, K. J., MacDougall, R., Clowe, S., and Driedzic, W. R. (2000). Anoxic performance of the American eel (Anguilla rostrata L.) heart requires extracellular glucose. J. Exp. Zool. 286, 699–706. doi: 10.1002/(SICI)1097-010X(20000601)286:7<699::AID-JEZ4>3.0.CO;2-G
Bath, R. N., and Eddy, F. B. (1980). Transport of nitrite across fish gills, J. Exp. Zool. 214, 119–121. doi: 10.1002/jez.1402140115
Bickler, P. E., and Buck, L. T. (2007). Hypoxia tolerance in reptiles, amphibians, and fishes: life with variable oxygen availability. Annu. Rev. Physiol. 69, 5.1–5.26. doi: 10.1146/annurev.physiol.69.031905.162529
Biswas, S. P., Palande, N. V., and Jadhao, A. G. (2011). Nitric oxide inhibited the melanophore aggregation induced by extracellular calcium concentration in snakehead fish, Channa punctatus. Fish Physiol. Biochem. 37, 919–927. doi: 10.1007/s10695-011-9489-9
Boutilier, R. G. (2001). Mechanisms of cell survival in hypoxia and hypothermia. J. Exp. Biol. 204(Pt 18), 3171–3181.
Bryan, N. S. (2006). Nitrite in nitric oxide biology: cause or consequence? A systems-based review. Free Radic. Biol. Med. 41, 691–701. doi: 10.1016/j.freeradbiomed.2006.05.019
Buckler, K. J. (1999). Background leak K+-currents and oxygen sensing in carotid body type 1 cells. Respir. Physiol. 115, 179–187. doi: 10.1016/S0034-5687(99)00015-8
Buckler, K. J. (2007). TASK-like potassium channels and oxygen sensing in the carotid body. Respir. Physiol. Neurobiol. 157, 55–64. doi: 10.1016/j.resp.2007.02.013
Burleson, M. L., Mercer, S. E., and Wilk-Blaszczak, M. A. (2006). Isolation and characterization of putative O2 chemoreceptor cells from the gills of channel catfish (Ictalurus punctatus). Brain Res. 1092, 100–107. doi: 10.1016/j.brainres.2006.03.085
Cameron, J. S., Hoffmann, K. E., Zia, C., Hemmett, H. M., Kronsteiner, A., and Lee, C. M. (2003). A role for nitric oxide in hypoxia-induced activation of cardiac KATP channels in goldfish (Carassius auratus). J. Exp. Biol. 206, 4057–4065. doi: 10.1242/jeb.00655
Castello, P. R., David, P. S., McClure, T., Crook, Z., and Poyton, R. O. (2006). Mitochondrial cytochrome oxidase produces nitric oxide under hypoxic conditions: implications for oxygen sensing and hypoxic signaling in eukaryotes. Cell Metab. 3, 277–287. doi: 10.1016/j.cmet.2006.02.011
Cerra, M. C., Angelone, T., Parisella, M. L., Pellegrino, D., and Tota, B. (2009). Nitrite modulates contractility of teleost (Anguilla anguilla and Chionodraco hamatus, i.e. the Antarctic hemoglobinless icefish) and frog (Rana esculenta) hearts. Biochim. Biophys. Acta 1787, 849–855. doi: 10.1016/j.bbabio.2009.03.008
Chang, J. P., Sawisky, G. R., Davis, P. J., Pemberton, J. G., Rieger, A. M., and Barreda, D. R. (2014). Relationship between nitric oxide- and calcium-dependent signal transduction pathways in growth hormone release from dispersed goldfish pituitary cells. Gen. Comp. Endocrinol. 206, 118–129. doi: 10.1016/j.ygcen.2014.07.006
Chouchani, E. T., Methner, C., Nadtochiy, S. M., Logan, A., Pell, V. R., Ding, S., et al. (2013). Cardioprotection by S-nitrosation of a cysteine switch on mitochondrial complex I. Nat. Med. 19, 753–759. doi: 10.1038/nm.3212
Cogliati, S., Enriquez, J. A., and Scorrano, L. (2016). Mitochondrial Cristae: where beauty meets functionality. Trends Biochem. Sci. 41, 261–273. doi: 10.1016/j.tibs.2016.01.001
Cooper, C. E., Mason, M. G., and Nicholls, P. (2008). A dynamic model of nitric oxide inhibition of mitochondrial cytochrome c oxidase. Biochim. Biophys. Acta 1777, 867–876. doi: 10.1016/j.bbabio.2008.03.021
Cosby, K., Partovi, K. S., Crawford, J. H., Patel, R. P., Reiter, C. D., Martyr, S., et al. (2003). Nitrite reduction to nitric oxide by deoxyhemoglobin vasodilates the human circulation. Nat. Med. 9, 1498–1505. doi: 10.1038/nm954
Crowder, W. C., Nie, M., and Ultsch, G. R. (1998). Oxygen uptake in bullfrog tadpoles (Rana catesbeiana). J. Exp. Zool. 280, 121–134. doi: 10.1002/(SICI)1097-010X(19980201)280:2<121::AID-JEZ3>3.0.CO;2-Q
de Lima Portella, R., Bickta, J., and Shiva, S. (2015). Nitrite confers preconditioning and cytoprotection after Ischemia/reperfusion injury through the modulation of mitochondrial function. Antioxid. Redox Signal. 23, 307–327. doi: 10.1089/ars.2015.6260
Dezfulian, C., Shiva, S., Alekseyenko, A., Pendyal, A., Beiser, D. G., Munasinghe, J., et al. (2009). Nitrite therapy after cardiac arrest reduces reactive oxygen species generation, improves cardiac and neurological function, and enhances survival via reversible inhibition of mitochondrial complex I. Circulation 120, 897–905. doi: 10.1161/CIRCULATIONAHA.109.853267
Driedzic, W. R., and Gesser, H. (1994). Energy metabolism and contractility in ectothermic vertebrate hearts: hypoxia, acidosis, and low temperature. Physiol. Rev. 74, 221–258. doi: 10.1152/physrev.1994.74.1.221
Dzal, Y. A., Jenkin, S. E., Lague, S. L., Reichert, M. N., York, J. M., and Pamenter, M. E. (2015). Oxygen in demand: How oxygen has shaped vertebrate physiology. Comp. Biochem. Physiol. A Mol. Integr. Physiol. 186, 4–26. doi: 10.1016/j.cbpa.2014.10.029
Erusalimsky, J. D., and Moncada, S. (2007). Nitric oxide and mitochondrial signaling: from physiology to pathophysiology. Arterioscler. Thromb. Vasc. Biol. 27, 2524–2531. doi: 10.1161/ATVBAHA.107.151167
Evans, A. M., Hardie, D. G., Peers, C., Wyatt, C. N., Viollet, B., Kumar, P., et al. (2009). Ion channel regulation by AMPK: the route of hypoxia-response coupling in thecarotid body and pulmonary artery. Ann. N. Y. Acad. Sci. 1177, 89–100. doi: 10.1111/j.1749-6632.2009.05041.x
Evans, A. M., Mustard, K. J., Wyatt, C. N., Peers, C., Dipp, M., Kumar, P., et al. (2005). Does AMP-activated protein kinase couple inhibition of mitochondrial oxidative phosphorylation by hypoxia to calcium signaling in O2-sensing cells? J. Biol. Chem. 280, 41504–41511. doi: 10.1074/jbc.M510040200
Fago, A., and Jensen, F. B. (2015). Hypoxia tolerance, nitric oxide, and nitrite: lessons from extreme animals. Physiology (Bethesda). 30, 116–126. doi: 10.1152/physiol.00051.2014
Farrell, A. P. (2007). Tribute to P. L. Lutz: a message from the heart – why hypoxic bradycardia in fishes? J. Exp. Biol. 210, 1715–1725. doi: 10.1242/jeb.02781
Farrell, A. P., and Stecyk, J. A. (2007). The heart as a working model to explore themes and strategies for anoxic survival in ectothermic vertebrates. Comp. Biochem. Physiol. A 147, 300–312. doi: 10.1016/j.cbpa.2007.01.021
Filice, M., Amelio, D., Garofalo, F., David, S., Fucarino, A., Jensen, F. B., et al. (2017). Angiotensin II dependent cardiac remodeling in the eel Anguilla anguilla involves the NOS/NO system. Nitric Oxide 65, 50–59. doi: 10.1016/j.niox.2017.02.007
Foster, M. W., Hess, D. T., and Stamler, J. S. (2009). Protein S-nitrosylation in health and disease: a current perspective. Trends Mol. Med. 15, 391–404. doi: 10.1016/j.molmed.2009.06.007
Galli, G. L., and Richards, J. G. (2014). Mitochondria from anoxia-tolerant animals reveal common strategies to survive without oxygen. J. Comp. Physiol. B. 184, 285–302. doi: 10.1007/s00360-014-0806-3
Garofalo, F., Amelio, D., Gattuso, A., Cerra, M. C., and Pellegrino, D. (2015). Cardiac contractility in Antarctic teleost is modulated by nitrite through xanthine oxidase and cytochrome p-450 nitrite reductase. Nitric Oxide 49, 1–7. doi: 10.1016/j.niox.2015.05.002
Garofalo, F., Imbrogno, S., Tota, B., and Amelio, D. (2012). Morpho-functional characterization of the goldfish (Carassius auratus L.) heart. Comp. Biochem. Physiol. 163, 215–222. doi: 10.1016/j.cbpa.2012.05.206
Garofalo, F., Parisella, M. L., Amelio, D., Tota, B., and Imbrogno, S. (2009a). Phospholamban S-nitrosylation modulates starling response in fish heart. Proc. R. Soc. B: Biol. Sci. 276, 4043–4052. doi: 10.1098/rspb.2009.1189
Garofalo, F., Pellegrino, D., Amelio, D., and Tota, B. (2009b). The Antarctic hemoglobinless icefish, fifty five years later: a unique cardiocirculatory interplay of disaptation and phenotypic plasticity. Comp. Biochem. Physiol. A Mol. Integr. Physiol. 154, 10–28. doi: 10.1016/j.cbpa.2009.04.621
Gautier, C., van Faassen, E., Mikula, I., Martasek, P., and Slama-Schwok, A. (2006). Endothelial nitric oxide synthase reduces nitrite anions to NO under anoxia, Biochem. Biophys. Res. Commun. 341, 816–821. doi: 10.1016/j.bbrc.2006.01.031
Gilmour, K. M., and Perry, S. F. (2007). “Branchial chemoreceptor regulation of cardiorespiratory function,” in Fish Physiology v25 Sensory Systems Neuroscience, eds T. J. Hara and B. Zielinski (San Diego, CA: Academic Press), 97–151.
Gladwin, M. T., Schechter, A. N., Kim-Shapiro, D. B., Patel, R. P., Hogg, N., Shiva, S., et al. (2005). The emerging biology of the nitrite anion. Nat. Chem. Biol. 1, 308–314. doi: 10.1038/nchembio1105-308
Guzik, T. J., West, N. E., Pillai, R., Taggart, D. P., and Channon, K. M. (2002). Nitric oxide modulates superoxide release and peroxynitrite formation in human blood vessels. Hypertension 39, 1088–1094. doi: 10.1161/01.HYP.0000018041.48432.B5
Hagen, T., Taylor, C. T., Lam, F., and Moncada, S. (2003). Redistribution of intracellular oxygen in hypoxia by nitric oxide: effect on HIF1alpha. Science 302, 1975–1978. doi: 10.1126/science.1088805
Hansen, M. N., Gerber, L., and Jensen, F. B. (2016). Nitric oxide availability in deeply hypoxic crucian carp: acute and chronic changes and utilization of ambient nitrite reservoirs. Am. J. Physiol. Regul. Integr. Comp. Physiol. 310, R532–R540. doi: 10.1152/ajpregu.00515.2015
Hansen, M. N., and Jensen, F. B. (2010). Nitric oxide metabolites in goldfish under normoxic and hypoxic conditions. J. Exp. Biol. 213, 3593–3602. doi: 10.1242/jeb.048140
Heinrich, T. A., da Silva, R. S., Miranda, K. M., Switzer, C. H., Wink, D. A., and Fukuto, J. M. (2013). Biological nitric oxide signalling: chemistry and terminology. Br. J. Pharmacol. 169, 1417–1429. doi: 10.1111/bph.12217
Hendgen-Cotta, U. B., Merx, M. W., Shiva, S., Schmitz, J., Becher, S., Klare, J. P., et al. (2008). Nitrite reductase activity of myoglobin regulates respiration and cellular viability in myocardial ischemia-reperfusion injury. Proc. Natl. Acad. Sci. U.S.A. 105, 10256–10261. doi: 10.1073/pnas.0801336105
Hermes-Lima, M., and Zenteno-Savín, T. (2002). Animal response to drastic changes in oxygen availability and physiological oxidative stress. Comp. Biochem. Physiol. C Toxicol. Pharmacol. 133, 537–556.
Hickey, A. J., Renshaw, G. M., Speers-Roesch, B., Richards, J. G., Wang, Y., Farrell, A. P., et al. (2012). A radical approach to beating hypoxia: depressed free radical release from heart fibres of the hypoxia-tolerant epaulette shark (Hemiscyllum ocellatum). J. Comp. Physiol. B 182, 91–100. doi: 10.1007/s00360-011-0599-6
Hill, B. G., Dranka, B. P., Bailey, S. M., Lancaster, J. R. Jr., and Darley-Usmar, V. M. (2010). What part of NO don't you understand? Some answers to the cardinal questions in nitric oxide biology. J. Biol. Chem. 285, 19699–19704. doi: 10.1074/jbc.R110.101618
Hochachka, P. W. (1986). Defense strategies against hypoxia and hypothermia. Science 231, 234–241. doi: 10.1126/science.2417316
Hochachka, P. W., and Lutz, P. L. (2001). Mechanism, origin, and evolution of anoxia tolerance in animals. Comp. Biochem. Physiol. B Biochem. Mol. Biol. 130, 435–459. doi: 10.1016/S1096-4959(01)00408-0
Hochachka, P. W., and Somero, G. N. (2002). Biochemical Adaptation: Mechanism and Process in Physiological Evolution. New York, NY: Oxford University Press.
Imbrogno, S. (2013). The eel heart: multilevel insights into functional organ plasticity. J. Exp. Biol. 216, 3575–3586. doi: 10.1242/jeb.089292
Imbrogno, S., Capria, C., Tota, B., and Jensen, F. B. (2014). Nitric oxide improves the hemodynamic performance of the hypoxic goldfish (Carassius auratus) heart. Nitric Oxide 42, 24–31. doi: 10.1016/j.niox.2014.08.012
Imbrogno, S., and Cerra, M. C. (2017). “Hormonal and autacoid control of cardiac function,” in Fish Physiology: The Cardiovascular System: Design, Control and Function, 1st Edn., Vol. 36, eds A. K. Gamperl, A. P. Farrell, and C. J. Brauner (Oxford: Academic Press), 265–315.
Imbrogno, S., Filice, M., Cerra, M. C., and Gattuso, A. (2018). NO, CO, and H2S: what about gasotransmitters in fish and amphibian hearts? Acta Physiologica (Oxf). doi: 10.1111/apha.13035. [Epub ahead of print].
Imbrogno, S., Garofalo, F., Amelio, D., Capria, C., and Cerra, M. C. (2013). Humoral control of cardiac remodeling in fish: role of Angiotensin, II. Gen. Comp. Endocrinol. 194, 189–197. doi: 10.1016/j.ygcen.2013.09.009
Imbrogno, S., Garofalo, F., Cerra, M. C., Mahata, S. K., and Tota, B. (2010). The catecholamine release-inhibitory peptide catestatin (chromogranin A344-363) modulates myocardial function in fish. J. Exp. Biol. 213, 3636–3643. doi: 10.1242/jeb.045567
Imbrogno, S., Mazza, R., Pugliese, C., Filice, M., Angelone, T., Loh, Y. P., et al. (2017). The Chromogranin A-derived sympathomimetic serpinin depresses myocardial performance in teleost and amphibian hearts. Gen. Comp. Endocrinol. 240, 1–9. doi: 10.1016/j.ygcen.2016.09.004
Imbrogno, S., Tota, B., and Gattuso, A. (2011). The evolutionary functions of cardiac NOS/NO in vertebrates tracked by fish and amphibian paradigms. Nitric Oxide 25, 1–10. doi: 10.1016/j.niox.2011.05.001
Jansson, E. A., Huang, L., Malkey, R., Govoni, M., Nihlén, C., Olsson, A., et al. (2008). A mammalian functional nitrate reductase that regulates nitrite and nitric oxide homeostasis. Nat. Chem. Biol. 4, 411–417. doi: 10.1038/nchembio.92
Jensen, F. B. (2007). Nitric oxide formation from nitrite in zebrafish J. Exp. Biol. 210, 3387–3394. doi: 10.1242/jeb.008748
Jensen, F. B. (2009). The role of nitrite in nitric oxide homeostasis: a comparative perspective. Biochim. Biophys. Acta 1787, 841–848. doi: 10.1016/j.bbabio.2009.02.010
Jensen, F. B., and Hansen, M. N. (2011). Differential uptake and metabolism of nitrite in normoxic and hypoxic goldfish. Aquat. Toxicol. 101, 318–325. doi: 10.1016/j.aquatox.2010.11.007
Jensen, F. B., Hansen, M. N., Montesanti, G., and Wang, T. (2014). Nitric oxide metabolites during anoxia and reoxygenation in the anoxia-tolerant vertebrate Trachemys scripta. J. Exp. Biol. 217, 423–431. doi: 10.1242/jeb.093179
Jonz, M. G. (2014). “Oxygen sensing,” in The Physiology of Fishes 4th Edn., eds D. H. Evans, J. B. Claiborne, and S. Currie (Boca Raton, FL: CRC Press), 149–174.
Jonz, M. G., Fearon, I. M., and Nurse, C. A. (2004). Neuroepithelial oxygen chemoreceptors of the zebrafish gill. J. Physiol. 560, 737–752. doi: 10.1113/jphysiol.2004.069294
Jonz, M. G., Zachar, P. C., Da Fonte, D. F., and Mierzwa, A. S. (2015). Peripheral chemoreceptors in fish: a brief history and a look ahead. Comp. Biochem. Physiol. A Mol. Integr. Physiol. 186, 27–38. doi: 10.1016/j.cbpa.2014.09.002
Jugdutt, B. I. (2002). Nitric oxide and cardioprotection during ischemia-reperfusion. Heart Fail. Rev. 7, 391–405.
Kimura, H. (2011). Hydrogen sulfide: its production, release and functions. Amino Acids, 41, 113–121. doi: 10.1007/s00726-010-0510-x
Kleinbongard, P., Dejam, A., Lauer, T., Rassaf, T., Schindler, A., Picker, O., et al. (2003). Plasma nitrite reflects constitutive nitric oxide synthase activity in mammals. Free Radic. Biol. Med. 35, 790–796. doi: 10.1016/S0891-5849(03)00406-4
Koolhaas, J. M., Korte, S. M., De Boer, S. F., Van Der Vegt, B. J., Van Reenen, C. G., Hopster, H., et al. (1999). Coping styles in animals: current status in behavior and stress-physiology. Neurosci. Biobehav. Rev. 23, 925–935. doi: 10.1016/S0149-7634(99)00026-3
Kozlov, A. V., Staniek, K., and Nohl, H. (1999). Nitrite reductase activity is a novel function of mammalian mitochondria. FEBS Lett. 454, 127–130. doi: 10.1016/S0014-5793(99)00788-7
Liu, L., and Simon, M. C. (2004). Regulation of transcription and translation by hypoxia. Cancer Biol. Ther. 3, 492–497. doi: 10.4161/cbt.3.6.1010
López-Barneo, J., González-Rodríguez, P., Gao, L., Fernández-Agüera, M. C., Pardal, R., and Ortega-Sáenz, P. (2016). Oxygen sensing by the carotid body: mechanisms and role in adaptation to hypoxia. Am. J. Physiol. Cell. Physiol. 310, C629–C642. doi: 10.1152/ajpcell.00265.2015
Lundberg, J. O., Gladwin, M. T., Ahluwalia, A., Benjamin, N., Bryan, N. S., Butler, A., et al. (2009). Nitrate and nitrite in biology, nutrition and therapeutics. Nat. Chem. Biol. 5, 865–869. doi: 10.1038/nchembio.260
Lundberg, J. O., Weitzberg, E., and Gladwin, M. T. (2008). The nitrate-nitrite-nitric oxide pathway in physiology and therapeutics. Nat. Rev. Drug Discov. 7, 156–167. doi: 10.1038/nrd2466
Lutz, P. L., and Nilsson, G. E. (2004). Vertebrate brains at the pilot light. Respir. Physiol. Neurobiol. 141, 285–296. doi: 10.1016/j.resp.2004.03.013
Mason, M. G., Nicholls, P., Wilson, M. T., and Cooper, C. E. (2006). Nitric oxide inhibition of respiration involves both competitive (heme) and noncompetitive (copper) binding to cytochrome c oxidase. Proc. Natl. Acad. Sci. U.S.A. 103, 708–713. doi: 10.1073/pnas.0506562103
Mateo, J., García-Lecea, M., Cadenas, S., Hernández, C., and Moncada, S. (2003). Regulation of hypoxia-inducible factor-1alpha by nitric oxide through mitochondria-dependent and -independent pathways. Biochem. J. 376, 537–544. doi: 10.1042/bj20031155
Mathur, G. B. (1967). Anaerobic respiration in a cyprinoid fish Rasbora daniconius (Ham). Nature 214, 318–319. doi: 10.1038/214318b0
McNeill, B., and Perry, S. F. (2006). The interactive effects of hypoxia and nitric oxide on catecholamine secretion in rainbow trout (Oncorhynchus mykiss). J. Exp. Biol. 209, 4214–4223. doi: 10.1242/jeb.02519
Mendelsohn, B. A., Kassebaum, B. L., and Gitlin, J. D. (2008). The zebrafish embryo as a dynamic model of anoxia tolerance. Dev. Dyn. 237, 1780–1788. doi: 10.1002/dvdy.21581
Millar, T. M., Stevens, C. R., Benjamin, N., Eisenthal, R., Harrison, R., and Blake, D. R. (1998). Xanthine oxidoreductase catalyses the reduction of nitrates and nitrite to nitric oxide under hypoxic conditions. FEBS Lett. 427, 225–228. doi: 10.1016/S0014-5793(98)00430-X
Milsom, W. K. (2012). New insights into gill chemoreception: receptor distribution and roles in water and air breathing fish. Respir. Physiol. Neurobiol. 184, 326–339. doi: 10.1016/j.resp.2012.07.013
Milsom, W. K., and Burleson, M. L. (2007). Peripheral arterial chemoreceptors and the evolution of the carotid body. Respir. Physiol. Neurobiol. 157, 4–11. doi: 10.1016/j.resp.2007.02.007
Misfeldt, M., Fago, A., and Gesser, H. (2009). Nitric oxide increases myocardial efficiency in the hypoxia-tolerant turtle Trachemys scripta. J. Exp. Biol. 212, 954–960. doi: 10.1242/jeb.025171
Moncada, S., and Higgs, A. (1993). The L-arginine-nitric oxide pathway. N. Engl. J. Med. 329, 2002–2012. doi: 10.1056/NEJM199312303292706
Montiglio, P. O., Garant, D., Thomas, D., and Re'ale, D. (2010). Individual variation in temporal activity patterns in open-field tests. Anim. Behav. 80, 905–912. doi: 10.1016/j.anbehav.2010.08.014
Nagababu, E., Ramasamy, S., Abernethy, D. R., and Rifkind, J. M. (2003). Active nitric oxide produced in the red cell under hypoxic conditions by deoxyhemoglobin-mediated nitrite reduction, J. Biol. Chem. 278, 46349–46356. doi: 10.1074/jbc.M307572200
Nikinmaa, M., Gassmann, M., and Bogdanova, A. (2011). “Oxygen sensing: the role of reactive oxygen species,” in Oxidative Stress in Aquatic Ecosystems, eds D. Abele, J. P. Vàzquez- Medina, and T. Zenteno-Savin (Chichester: John Wiley & Sons Ltd), 165–177.
Nilsson, G. E. (2001). Surviving anoxia with the brain turned on. News Physiol. Sci. 16, 218–221. doi: 10.1152/physiologyonline.2001.16.5.217
Noma, A. (1983). ATP-regulated K+ channels in cardiac muscle. Nature 305, 147–158. doi: 10.1038/305147a0
Nurse, C. A. (2005). Neurotransmission and neuromodulation in the chemosensory carotid body. Auton. Neurosci. 120, 1–9. doi: 10.1016/j.autneu.2005.04.008
Olson, K. R. (2011). Hydrogen sulfide is an oxygen sensor in the carotid body. Respir. Physiol. Neurobiol. 179, 103–110. doi: 10.1016/j.resp.2011.09.010
Olson, K. R., Healy, M. J., Qin, Z., Skovgaard, N., Vulesevic, B., Duff, D. W., et al. (2008). Hydrogen sulfide as an oxygen sensor in trout gill chemoreceptors. Am. J. Physiol. Regul. Integr. Comp. Physiol. 295, R669–R680. doi: 10.1152/ajpregu.00807.2007
Overholt, J. L., and Prabhakar, N. R. (1997). Ca2+ current in rabbit carotid body glomus cells is conducted by multiple types of high-voltage-activated Ca2+ channels. J. Neurophysiol. 78, 2467–2474. doi: 10.1152/jn.1997.78.5.2467
Pacher, P., Beckman, J. S., and Liaudet, L. (2007). Nitric oxide and peroxynitrite in health and disease. Physiol. Rev. 87, 315–424. doi: 10.1152/physrev.00029.2006
Padilla, P. A., and Roth, M. B. (2001). Oxygen deprivation causes suspended animation in the zebrafish embryo. Proc. Natl. Acad. Sci. U.S.A. 98, 7331–7335. doi: 10.1073/pnas.131213198
Pamenter, M. E. (2014). Mitochondria: a multimodal hub of hypoxia tolerance. Can. J. Zool. 92, 569–589. doi: 10.1139/cjz-2013-0247
Pautz, A., Art, J., Hahn, S., Nowag, S., Voss, C., and Kleinert, H. (2010). Regulation of the expression of inducible nitric oxide synthase. Nitric Oxide 23, 75–93. doi: 10.1016/j.niox.2010.04.007
Pedersen, C. L., Faggiano, S., Helbo, S., Gesser, H., and Fago, A. (2010). Roles of nitric oxide, nitrite and myoglobin on myocardial efficiency in trout (Oncorhynchus mykiss) and goldfish (Carassius auratus): implications for hypoxia tolerance. J. Exp. Biol. 213, 2755–2762. doi: 10.1242/jeb.041624
Peers, C. (1990). Hypoxic suppression of K+ currents in type I carotid body cells: selective effect on the Ca2+-activated K+ current. Neurosci. Lett. 2, 253–256. doi: 10.1016/0304-3940(90)90846-2
Peng, Y. J., Nanduri, J., Raghuraman, G., Souvannakitti, D., Gadalla, M. M., Kumar, G. K., et al. (2010). H2S mediates O2 sensing in the carotid body. Proc. Natl. Acad. Sci. U.S.A. 107, 10719–10724. doi: 10.1073/pnas.1005866107
Pérez-García, M. T., Colinas, O., Miguel-Velado, E., Moreno-Domínguez, A., and López-López, J. R. (2004). Characterization of the Kv channels of mouse carotid body chemoreceptor cells and their role in oxygen sensing. J. Physiol. 557, 457–471. doi: 10.1113/jphysiol.2004.062281
Perry, S. F., and Tzaneva, V. (2016). The sensing of respiratory gases in fish: Mechanisms and signalling pathways. Respir. Physiol. Neurobiol. 224, 71–79. doi: 10.1016/j.resp.2015.06.007
Petersen, M. G., Dewilde, S., and Fago, A. (2008). Reactions of ferrous neuroglobin and cytoglobin with nitrite under anaerobic conditions J. Inorg. Biochem. 102, 1777–1782. doi: 10.1016/j.jinorgbio.2008.05.008
Porteus, C. S., Abdallah, S. J., Pollack, J., Kumai, Y., Kwong, R. W., Yew, H. M., et al. (2014). The role of hydrogen sulphide in the control of breathing in hypoxic zebrafish (Danio rerio). J. Physiol. 592, 3075–3088. doi: 10.1113/jphysiol.2014.271098
Porteus, C. S., Pollack, J., Tzaneva, V., Kwong, R. W., Kumai, Y., Abdallah, S. J., et al. (2015). A role for nitric oxide in the control of breathing in zebrafish (Danio rerio). J. Exp. Biol. 218, 3746–3753. doi: 10.1242/jeb.127795
Prabhakar, N. R. (1999). NO and CO as second messengers in oxygen sensing in the carotid body. Respir. Physiol. 115, 161–168. doi: 10.1016/S0034-5687(99)00019-5
Prabhakar, N. R., and Peers, C. (2014). Gasotransmitter regulation of ion channels: a key step in O2 sensing by the carotid body. Physiology (Bethesda) 29, 49–57. doi: 10.1152/physiol.00034.2013
Qin, Z., Lewis, J. E., and Perry, S. F. (2010). Zebrafish (Danio rerio) gill neuroepithelial cells are sensitive chemoreceptors for environmental CO2. J. Physiol. 588, 861–872. doi: 10.1113/jphysiol.2009.184739
Rashid, I., Nagpure, N. S., Srivastava, P., Kumar, R., Pathak, A. K., Singh, M., et al. (2017). HRGFish: a database of hypoxia responsive genes in fishes. Sci. Rep. 7:42346. doi: 10.1038/srep42346
Reutov, V. P. (2002). Nitric oxide cycle in mammals and the cyclicity principle. Biochemistry (Mosc) 67, 293–311. doi: 10.1023/A:1014832416073
Ronson, R. S., Nakamura, M., and Vinten-Johansen, J. (1999). The cardiovascular effects and implications of peroxynitrite. Cardiovasc. Res. 44, 47–59. doi: 10.1016/S0008-6363(99)00184-4
Sanchez, A., Soncini, R., Wang, T., Koldkjaer, P., Taylor, E. W., and Glass, M. L. (2001). The differential cardio-respiratory responses to ambient hypoxia and systemic hypoxaemia in the South American lungfish, Lepidosiren paradoxa. Comp. Biochem. Physiol. Part A Mol. Integr. Physiol. 130, 677–687. doi: 10.1016/S1095-6433(01)00395-6
Sanchez, D., López-López, J. R., Pérez-García, M. T., Sanz-Alfayate, G., Obeso, A., Ganfornina, M. D., et al. (2002). Molecular identification of Kvalpha subunits that contribute to the oxygen-sensitive K+ current of chemoreceptor cells of the rabbit carotid body. J. Physiol. 542, 369–382. doi: 10.1113/jphysiol.2002.018382
Sandvik, G. K., Nilsson, G. E., and Jensen, F. B. (2012). Dramatic increase of nitrite levels in hearts of anoxia-exposed crucian carp supporting a role in cardioprotection. Am. J. Physiol. Regul. Integr. Comp. Physiol. 302, R468–R477. doi: 10.1152/ajpregu.00538.2011
Schjolden, J., Stoskhus, A., and Winberg, S. (2005). Does individual variation in stress responses and agonistic behavior reflect divergent stress coping strategies in juvenile rainbow trout? Physiol. Biochem. Zool. 78, 715–723. doi: 10.1086/432153
Shen, W., Tian, R., Saupe, W. K., Spindler, M., and Ingwall, S. J. (2001). Endogenous nitric oxide enhances coupling between O2 consumption and ATP synthesis in guinea pig hearts. Am. J. Physiol. Heart Circ. Physiol. 281, 838–846. doi: 10.1152/ajpheart.2001.281.2.H838
Shiva, S., Huang, Z., Grubina, R., Sun, J., Ringwood, L. A., et al. (2007a). Deoxymyoglobin is a nitrite reductase that generates nitric oxide and regulates mitochondrial respiration. Circ. Res. 100, 654–661. doi: 10.1161/01.RES.0000260171.52224.6b
Shiva, S., Sack, M. N., Greer, J. J., Duranski, M., Ringwood, L. A., Burwell, L., et al. (2007b). Nitrite augments tolerance to ischemia/reperfusion injury via the modulation of mitochondrial electron transfer. J. Exp. Med. 204, 2089–2102. doi: 10.1084/jem.20070198
Clark, A. B., Coleman, K., and Dearstyne, T. (1994). Shyness and boldness in humans and other animals. Trends Ecol. Evol. 9, 442–446. doi: 10.1016/0169-5347(94)90134-1
Sollid, J., Rissanen, E., Tranberg, H. K., Thorstensen, T., Vuori, K. A., Nikinmaa, M., et al. (2006). HIF-1alpha and iNOS levels in crucian carp gills during hypoxia-induced transformation. J. Comp. Physiol. B. 176, 359–369. doi: 10.1007/s00360-005-0059-2
Stecyk, J. A., Stenløcken, K. O., Farrell, A. P., and Nilsson, G. E. (2004). Maintained cardiac pumping in anoxic crucian carp. Science 306:77. doi: 10.1126/science.1100763
Stensløkken, K. O., Ellefsen, S., Stecyk, J. A., Dahl, M. B., Nilsson, G. E., and Vaage, J. (2008). Differential regulation of AMP-activated kinase and AKT kinase in response to oxygen availability in crucian carp (Carassius carassius). Am. J. Physiol. Regul. Integr. Comp. Physiol. 295, R1803–R1814. doi: 10.1152/ajpregu.90590.2008
Summers, B. A., Overholt, J. L., and Prabhakar, N. R. (1999). Nitric oxide inhibits L-type Ca2+ current in glomus cells of the rabbit carotid body via a cGMP-independent mechanism. J. Neurophysiol. 81, 1449–1457. doi: 10.1152/jn.1999.81.4.1449
Sundin, L., Reid, S. G., Rantin, F. T., and Milsom, W. K. (2000). Branchial receptors and cardiorespiratory reflexes in a neotropical fish, the tambaqui (Colossoma macropomum). J. Exp. Biol. 203(Pt 7), 1225–1239.
Tota, B., Angelone, T., Mancardi, D., and Cerra, M. C. (2011). Hypoxia and anoxia tolerance of vertebrate hearts: an evolutionary perspective. Antioxid. Redox Signal. 14, 851–862. doi: 10.1089/ars.2010.3310
Tota, B., Quintieri, A. M., and Angelone, T. (2010). The emerging role of nitrite as an endogenous modulator and therapeutic agent of cardiovascular function. Curr. Med. Chem. 17, 1915–1925. doi: 10.2174/092986710791163948
Tran, S., and Gerlai, R. (2013). Individual differences in activity levels in zebrafish (Danio rerio). Behav. Brain Res. 257, 224–229. doi: 10.1016/j.bbr.2013.09.040
Tzaneva, V., Bailey, S., and Perry, S. F. (2011). The interactive effects of hypoxemia, hyperoxia, and temperature on the gill morphology of goldfish (Carassius auratus). Am. J. Physiol. Regul. Integr. Comp. Physiol. 300, R1344–R1351. doi: 10.1152/ajpregu.00530.2010
Tzaneva, V., and Perry, S. F. (2014). Heme oxygenase-1 (HO-1) mediated respiratory responses to hypoxia in the goldfish, Carassius auratus. Respir. Physiol. Neurobiol. 199, 1–8. doi: 10.1016/j.resp.2014.04.006
Tzaneva, V., and Perry, S. F. (2016). Role of endogenous carbon monoxide in the control of breathing in zebrafish (Danio rerio). Am. J. Physiol. Regul. Integr. Comp. Physiol. 311, R1262–R1270. doi: 10.1152/ajpregu.00094.2016
Urschel, M. R., and O'Brien, K. M. (2008). High mitochondrial densities in the hearts of Antarctic icefishes are maintained by an increase in mitochondrial size rather than mitochondrial biogenesis. J. Exp. Biol. 211, 2638–2646. doi: 10.1242/jeb.018598
Vallance, P., Rees, D., and Moncada, S. (2000). “Therapeutic potential of NOS inhibitors in septic shock,” in Handbook of Experimental Pharmacology, Vol. 143, ed B. Mayer (Berlin: Springer-Verlag), 385–397.
van Faassen, E. E., Bahrami, S., Feelisch, M., Hogg, N., Kelm, M., Kim-Shapiro, D. B., et al. (2009). Nitrite as regulator of hypoxic signaling in mammalian physiology. Med. Res. Rev. 29, 683–741. doi: 10.1002/med.20151
Vanin, A. F., Bevers, L. M., Slama-Schwok, A., and van Faassen, E. E. (2007). Nitric oxide synthase reduces nitrite to NO under anoxia. Cell. Mol. Life Sci. 64, 96–103. doi: 10.1007/s00018-006-6374-2
Van Raaij, M., Van den Thillart, G., Vianen, G. J., Pit, D. S., Balm, P. H., and Steffens, A. B. (1996). Substrate mobilization and hormonal changes in rainbow trout (Oncorhynchus mykiss, L.) and common carp (Cyprinus carpio, L.) during deep hypoxia and subsequent recovery. J. Comp. Physiol. 166, 443–452. doi: 10.1007/BF02337889
Varas, R., Wyatt, C. N., and Buckler, K. J. (2007). Modulation of TASK-like background potassium channels in rat arterial chemoreceptor cells by intracellular ATP and other nucleotides. J. Physiol. 583, 521–536. doi: 10.1113/jphysiol.2007.135657
Vornanen, M., and Haverinen, J. (2016). Glycogen dynamics of crucian carp (Carassius carassius) in prolonged anoxia. J. Comp. Physiol. B. 186, 999–1007. doi: 10.1007/s00360-016-1007-z
Vulesevic, B., McNeill, B., and Perry, S. F. (2006). Chemoreceptor plasticity and respiratory acclimation in the zebrafish Danio rerio. J. Exp. Biol. 209(Pt 7), 1261–1273. doi: 10.1242/jeb.02058
Walters, A. M., Porter, G. A., and Brookes, P. S. (2012). Mitochondria as a drug target in Ischemic heart disease and cardiomyopathy. Circ. Res. 111, 1222–1236. doi: 10.1161/CIRCRESAHA.112.265660
Wang, J., Hogan, J. O., Wang, R., White, C., and Kim, D. (2017). Role of cystathionine-γ-lyase in hypoxia-induced changes in TASK activity, intracellular [Ca2+] and ventilation in mice. Respir. Physiol. Neurobiol. 246, 98–106. doi: 10.1016/j.resp.2017.08.009
Wyatt, C. N., and Evans, A. M. (2007). AMP-activated protein kinase and chemotransduction in the carotid body. Respir. Physiol. Neurobiol. 157, 22–29. doi: 10.1016/j.resp.2007.01.021
Wyatt, C. N., Mustard, K. J., Pearson, S. A., Dallas, M. L., Atkinson, L., Kumar, P., et al. (2007). AMP-activated protein kinase mediates carotid body excitation by hypoxia. J. Biol. Chem. 282, 8092–8098. doi: 10.1074/jbc.M608742200
Wyatt, C. N., and Peers, C. (1995). Ca2+−activated K+ channels in isolated type I cells of the neonatal rat carotid body. J. Physiol. 483, 559–565. doi: 10.1113/jphysiol.1995.sp020606
Yuan, G., Peng, Y. J., Khan, S. A., Nanduri, J., Singh, A., Vasavda, C., et al. (2016). H2S production by reactive oxygen species in the carotid body triggers hypertension in a rodent model of sleep apnea. Sci. Signal. 9:ra80. doi: 10.1126/scisignal.aaf3204
Zachar, P. C., and Jonz, M. G. (2012). Oxygen sensitivity of gill neuroepithelial cells in the anoxia-tolerant goldfish. Adv. Exp. Med. Biol. 758, 167–172. doi: 10.1007/978-94-007-4584-1_23
Zachar, P. C., Pan, W., and Jonz, M. G. (2017). Characterization of ion channels and O2 sensitivity in gill neuroepithelial cells of the anoxia-tolerant goldfish (Carassius auratus). J. Neurophysiol. 118, 3014–3023. doi: 10.1152/jn.00237.2017
Keywords: heart, hypoxia, nitric oxide, nitrite, teleosts
Citation: Gattuso A, Garofalo F, Cerra MC and Imbrogno S (2018) Hypoxia Tolerance in Teleosts: Implications of Cardiac Nitrosative Signals. Front. Physiol. 9:366. doi: 10.3389/fphys.2018.00366
Received: 30 January 2018; Accepted: 26 March 2018;
Published: 12 April 2018.
Edited by:
Mariarosaria Santillo, University of Naples Federico II, ItalyReviewed by:
Philip Aaronson, King's College London, United KingdomGiovanna Zoccoli, Università degli Studi di Bologna, Italy
Copyright © 2018 Gattuso, Garofalo, Cerra and Imbrogno. This is an open-access article distributed under the terms of the Creative Commons Attribution License (CC BY). The use, distribution or reproduction in other forums is permitted, provided the original author(s) and the copyright owner are credited and that the original publication in this journal is cited, in accordance with accepted academic practice. No use, distribution or reproduction is permitted which does not comply with these terms.
*Correspondence: Alfonsina Gattuso, YWxmb25zaW5hLmdhdHR1c29AdW5pY2FsLml0
Maria C. Cerra, bWFyaWFfY2FybWVsYS5jZXJyYUB1bmljYWwuaXQ=
†These authors have contributed equally to this work.