- 1Department of Biomedical Imaging and Image-Guided Therapy, High-Field MR Center, Medical University of Vienna, Vienna, Austria
- 2Christian Doppler Laboratory for Clinical Molecular MR Imaging, MOLIMA, Vienna, Austria
- 3Oxford Centre for Clinical Magnetic Resonance Research, BHF Centre of Research Excellence, University of Oxford, Oxford, United Kingdom
- 4Department of Imaging Methods, Institute of Measurements Science, Slovak Academy of Sciences, Bratislava, Slovakia
- 5Centre of Sport Science and University Sport, University of Vienna, Vienna, Austria
- 6Division of Endocrinology and Metabolism, Department of Internal Medicine III, Medical University of Vienna, Vienna, Austria
Purpose: The influence of endurance training on skeletal muscle metabolism can currently be studied only by invasive sampling or through a few related parameters that are investigated by either proton (1H) or phosphorus (31P) magnetic resonance spectroscopy (MRS). The aim of this study was to compare the metabolic differences between endurance-trained triathletes and healthy volunteers using multi-parametric data acquired by both, 31P- and 1H-MRS, at ultra-high field (7T) in a single experimental protocol. This study also aimed to determine the interrelations between these MRS-derived metabolic parameters.
Methods: Thirteen male triathletes and ten active male volunteers participated in the study. Proton MRS data from the vastus lateralis yielded concentrations of acetylcarnitine, carnosine, and intramyocellular lipids (IMCL). For the measurement of phosphodiesters (PDEs), inorganic phosphate (Pi), phosphocreatine (PCr), and maximal oxidative capacity (Qmax) phosphorus MRS data were acquired at rest, during 6 min of submaximal exercise and following immediate recovery.
Results: The triathletes exhibited significantly higher IMCL levels, higher initial rate of PCr resynthesis (VPCr) during the recovery period, a shorter PCr recovery time constant (τPCr), and higher Qmax. Multivariate stepwise regression analysis identified PDE as the strongest independent predictor of whole-body maximal oxygen uptake (VO2max).
Conclusion: In conclusion, we cannot suggest a single MRS-based parameter as an exclusive biomarker of muscular fitness and training status. There is, rather, a combination of different parameters, assessable during a single multi-nuclear MRS session that could be useful for further cross-sectional and/or focused interventional studies on skeletal muscle fitness and training effects.
Introduction
The main tissue responsible for whole-body energy metabolism, glycogen storage, and glucose uptake, is skeletal muscle (Defronzo et al., 1981; DeFronzo and Tripathy, 2009). It is well-known that endurance training alters functional demands in skeletal muscle (Fujimoto et al., 2003). It has been shown that athletes with a background of endurance training have better oxidative metabolism (Pette, 1998), improved glucose uptake (Fujimoto et al., 2003), as well as enhanced capillarity and increased mitochondrial density (Pette, 1998). Moreover, the potential of exercise to adapt oxidative potential of skeletal muscles has been demonstrated by increased succinate dehydrogenase activity mainly in actively involved muscle fibers, i.e., slow-twitch muscle fibers which are primarily effected by endurance training (Saltin et al., 1977). Endurance training and long lasting low-load resistance exercise also generate metabolic stress, that can accumulate into adaptation in mitochondrial content and function (Groennebaek and Vissing, 2017). The vast majority of these observations are the result of biopsies or ex vivo measurements, unsuitable for repetitive assessment of skeletal muscle status during the training process.
Magnetic resonance spectroscopy (MRS) is a non-invasive alternative technique bound to define the performance-related characteristics of skeletal muscle and to explore the differences between skeletal muscle of endurance-trained men and untrained participants. Especially, proton (1H) and phosphorus (31P) MRS has been used to provide information about the metabolism of a tissue.
In particular, it has been reported that the carnosine content, which is measurable by localized 1H-MRS, could potentially be a good indicator for estimating muscle fiber composition, as it is present in different concentrations in slow-twitch than in fast-twitch fibers (Baguet et al., 2011). Further, a well-studied phenomenon in the context of skeletal muscle metabolism and athletic performance, also detectable by 1H-MRS, are intramyocellular lipids (IMCL). Usually, elevated IMCL levels can be found in the sedentary population, and, in the majority of cases, suggest impaired glucose uptake, which is closely related to insulin resistance (Krššák et al., 1999) and contributes to the development of obesity and type 2 diabetes mellitus (T2DM) (Kautzky-Willer et al., 2003). However, high IMCL levels alone cannot be taken as a sign of a lipid metabolism defect, as endurance-trained athletes have shown high IMCL concentrations without metabolic impairment (Thamer et al., 2003; Dubé et al., 2008). Another metabolite assessable by muscle 1H-MRS, acetylcarnitine, is important for maintaining pyruvate dehydrogenation activity, known to control the rate of aerobic carbohydrate oxidation (Constantin-Teodosiu, 2013). Moreover, skeletal muscle acetylcarnitine concentration correlates negatively with insulin resistance, with highest fasting concentrations of acetylcarnitine observed in endurance-trained athletes and the lowest in T2DM patients (Lindeboom et al., 2014).
Furthermore, 31P-MRS allows direct measurement of energy-rich metabolites, i.e., phosphocreatine (PCr), adenosine-tri-phosphate (ATP), and can be used dynamically to investigate oxidative energy production by mitochondria during exercise and subsequent recovery (Kemp and Radda, 1994; Kemp et al., 2015; Valkovič et al., 2017). Dynamic 31P-MRS has been used to probe human muscle metabolism since the 1980's (Chance et al., 1980; Meyer and Brown, 1982) with first in vivo studies focused on assessment of steady-state capability of oxidative phosphorylation of forearm muscle using the PCr/Pi ratio and dynamic changes in intracellular pH (Chance et al., 1981; Taylor et al., 1983; Arnold et al., 1984). During the years, PCr recovery rate after exercise has taken over as a measure of mitochondrial oxidative production rate (Boska, 1991; Walter et al., 1999; Kemp et al., 2015). Endurance trained athletes have been shown to recover PCr after exercise faster than untrained (McCully et al., 1989; Layec et al., 2009) and even sprint-trained athletes (Crowther et al., 2002; Johansen and Quistorff, 2003) reflecting their superior oxidative metabolism function.
Alternatively, the content of elevated skeletal muscle phosphodiesters (PDEs), measured by 31P-MRS, in age-matched participants, was suggested as a potential marker of muscle oxidative capacity (Valkovič et al., 2016). Changes in muscle mitochondrial density in response to training can potentially be assessed through alkaline inorganic phosphate (Pi) pool (Pi2) concentrations or Pi2/Pi ratios (Kan et al., 2010; Valkovič et al., 2016). In addition, 31P-MRS saturation transfer techniques allow estimation of PCr-to-ATP and Pi-to-ATP fluxes (FCK and FATP, respectively) even in muscles at rest (Valkovič et al., 2017). These resting measurements correlate with the findings of dynamic experiments (Schmid et al., 2012; Valkovič et al., 2016) and have been related to insulin resistance (Petersen et al., 2004). Nevertheless, the absolute values of FATP measured at rest significantly overestimates pure oxidative ATP synthesis, mainly due to strong glycolytic component of FATP and close to equilibrium turnover at rest (Balaban and Koretsky, 2011; From and Ugurbil, 2011). On the other hand, if measured during steady-state aerobic exercise, the glycolytic component of FATP will be minimal and the total measured flux will represent mitochondrial ATP turnover more closely (Brindle et al., 1989).
Taken together, 31P and 1H-MRS both provide ideal non-invasive tools for the in vivo assessment of skeletal muscle metabolism, which plays an essential role in whole-body energy metabolism and is the key factor in athletic performance. However, the applicability of different MRS methods on clinical lower-field MR systems has been limited by insufficient spectral resolution and rather low spectral and experimental signal-to-noise-ratio, which resulted in relatively long acquisition times, making a comprehensive measurement unsuitable for monitoring of muscular training status, i.e., the metabolic fitness of a skeletal muscle, or for routine examinations during the training process.
MRS examinations at ultra-high field (7T) provide, generally, better spectral resolution, higher repeatability, and improvements that have led to the opportunity to measure subtle signals of parameters for energy metabolism [i.e., carnosine, acetylcarnitine, the alkaline Pi pool (Pi2), PDE, i.e., glycerol-phosphocholine (GPC), and glycerol-phosphoethanolamine (GPE)], and to assess metabolic reaction rates under steady-state exercise conditions, which have never been measured in athletes in one integrative study.
Our aim was to use multi-nuclear MRS at 7T to compare the value of suggested basal and dynamic markers of oxidative metabolism in different training status of vastus lateralis (VL) in two groups of volunteers: healthy active individuals and endurance-trained triathletes. In our measurement and analysis we included (i) the examination of IMCL content in the context of the paradox of an athlete's high IMCL levels, (ii) the assessment of acetylcarnitine concentration because of its important role in lipid metabolism, and (iii) and the measurement carnosine concentration as a possible consequence of muscle fiber-type distribution. Moreover, endurance-trained athletes should have a higher volume of mitochondrial density, and, therefore, faster oxidative metabolism and faster PCr resynthesis following submaximal exercise (Johansen and Quistorff, 2003; Bogdanis, 2012). New, recently suggested markers of oxidative muscle metabolism, e.g., alkaline Pi, PDE, and Pi-to-ATP reaction rate measured in metabolic steady state during aerobic exercise, were also compared between the groups. As a final point we aimed to perform a bivariate and multivariate analysis of the interrelations between measured metabolic parameters.
Methods
Study Population
Thirteen male triathletes (age = 28.2 ± 5.7 years, BMI = 22.3±1.4 kg.m−2) and 10 healthy active male volunteers (age = 26.2 ± 4.7 years, BMI = 22.8 ± 2.6 kg.m−2) participated in the study.
All participating athletes were in a pre-season preparation phase without any special training dedicated methods, and without special diet or nutrition supplementation. All of them have been competing in triathlon events across various distances for at least 3 years at a national level, and the performance criterion was the time they needed to cycle 20 km (maximum allowed: 39 min) and 40 km (maximum allowed: 70 min) during their past competitions.
For the group of active volunteers, the inclusion criterion was normal and regular physical activity, e.g., cycling, running, gym, and team sports up to three times per week. No special nutrition and supplementation or any physical activity, such as weightlifting or special strength training, was required as an inclusion factor.
All participants, triathletes and healthy active volunteers, refrained from any physical activity 24 h before MR examinations and had no other changes in daily routine. Written, informed consent was provided before commencing and the study protocol adhered to the local ethics committee requirements.
One week before MR examinations, all participants underwent a standardized protocol for the determination of maximal oxygen uptake (VO2max) on a bicycle ergometer (Lode Excalibur, Groningen, The Netherlands) with continuous increments, until exhaustion, Measurement of VO2max was performed via “breath-by-breath” spiroergometry (Master CPX, VIASYS Healthcare). The test protocol included a 3-min adaptation phase for the measurement of the baseline and subsequent continuous increases of 20 W/min with a constant RPM of 90 rpm up to the individual maximal exhaustion. This ensured that the total duration of the spiroergometry for all subjects (trained and untrained) was between 7 min and a maximum of 26 min.
Magnetic Resonance (MR) Measurements
The entire MR examination protocol was performed within a single visit, starting at the same time in the afternoon, approximately 1 h after a mixed meal lunch. All MR measurements were performed on a 7T whole-body MR system (Siemens Healthcare, Erlangen, Germany). A 28-channel knee coil (QED, Mayfield Village, OH, USA) was used to acquire 1H-MR spectra and a dual-tuned (31P/1H) circular surface coil (10 cm diameter, Rapid Biomedical, Rimpar, Germany) was used to acquire 31P-MR spectra from the quadriceps of the left leg.
The measurements were divided into two parts. First, volunteers were in the supine position with the left thigh muscle placed inside the 28-channel knee coil for the acquisition of the 1H-MRS data. Afterward, the volunteers turned into the prone position, for the 31P-MRS measurement, and their thigh muscle was strapped onto an MR-compatible ergometer (Quadspect, Ergospect, Innsbruck, Austria) dedicated for knee extension exercise. The exercise resistance was set to 30% of the maximal voluntary contraction (MVC) force, determined by repeated measurement of the maximum force generated against the pedal fixed in the exercise position. The 1H/31P surface coil was positioned under the thigh muscles of the left leg. The localized 31P-MRS data acquisition which is described below in detail was performed before and during exercise and recovery protocol. This consisted of 7.5 min of baseline period dedicated for measurement at rest followed by 6 min of knee extension exercise and ending with 6 min of recovery (Valkovič et al., 2014; Tušek Jelenc et al., 2016). The volunteers were instructed by an audio signal to time the contraction-relaxation periods (exercise frequency 0.5 Hz), so that the spectra were always acquired during the relaxed state of the muscle under investigation. A schematic illustration of the experimental design is shown in Figure 1.

Figure 1. Schematic representation of the measurement protocol. First, volunteers were in the supine position with the left thigh muscle placed inside the 28-channel knee coil for the acquisition of the 1H-MRS data. Afterward, the volunteers turned into the prone position, for the 31P-MRS measurement, and their thigh muscle was strapped onto an MR-compatible ergometer dedicated for knee extension exercise. The 31P-MRS protocol consisted of 7.5 min of measurement at rest (2 min of partially relaxed, long TR static data acquisition, 3.5 min of four-angle saturation transfer (FAST) and 2 min of baseline data for the dynamic experiment) followed by 6 min of knee extension exercise (including the 3.5 min long FAST measurement starting 2 min after the onset of exercise, i.e., once the steady-state is reached) and ending with 6 min of recovery.
1H-MRS
T1 weighted, multi-slice localizer images were acquired and used for volume-of-interest (VOI) positioning. Spatial selection was achieved using a STEAM localization sequence and the VOI of 21 ml (40 × 35 × 15 mm3) was carefully placed in the VL muscle. Voxel positioning was guided by images so as to properly adjust voxel geometry and orientation to fit the actual shape of subject's muscle, avoiding the boundaries of muscle and subcutaneous fat. Localized shimming was performed manually, on the adjustment volume that matched the VOI, after automatic field-map acquisition based on gradient recalled, double echo field-map acquisition (GRE-SHIM, Siemens Healthcare, Erlangen, Germany). The final linewidth of the water signal was in the range of 28–38 Hz in the magnitude mode.
Signals of acetylcarnitine and IMCL were measured with the following parameters: repetition time (TR)/echo time (TE) = 2,000/350 ms; spectral bandwidth = 3 kHz; number of averages (NA) = 128; delta frequency = −2.5 ppm relative to water resonance; number of preparation scans = 4 (Klepochová et al., 2017).
The MR signal of carnosine was measured with water signal suppression and the following parameters: TR/TE = 9,000/20 ms; spectral bandwidth = 3 kHz; NA = 64; delta frequency = 2.8 ppm relative to water resonance; number of preparation scans = 0, as described by Just Kukurová et al. (2016).
For acquisition of a concentration reference, the water signal was measured with a TR = 2,000 ms and a TE = 20 ms; NA = 1; and delta frequency = 0 ppm.
31P-MRS
Depth-resolved in vivo spectroscopy (DRESS) localization (Bottomley et al., 1984) was used for 31P-MRS signal detection at rest, as well as during exercise and recovery. In particular, a 15 mm-thick MRS selection slab representing the VOI was placed over the VL, based on localizer images, and manual shimming was performed. A 90° FA was adjusted by finding the RF transmit voltage that provided the maximum of the localized PCr signal. No decoupling or NOE was used.
During the resting baseline, the assessment of metabolite concentrations static long TR 31P MR spectra (FA = 90°, TR = 15 s, NA = 8, acquisition time = 2 min) was performed. This measurement was followed by DRESS-localized four-angle saturation transfer (FAST) measurements performed in two experiments during next 3.5 min of resting baseline. The first part applied nominal FA of 52° (β) and NA = 8, and the second part applied nominal FA of 15° (α) and NA = 24, as described previously (Tušek Jelenc et al., 2016). The FAST measurement at rest was followed by the dynamic protocol with temporal resolution of 2 s, i.e., single shot acquisition with TR = 2 s. The dynamic protocol consisted of 2 min for baseline data, 6 min of knee extension exercise and 6 min of recovery (Valkovič et al., 2014). During exercise-induced metabolic steady-state, the FAST experiment was repeated to assess the reactions of creatine kinase (CK) and the ATP synthesis/hydrolysis cycle, as described previously (Tušek Jelenc et al., 2016). During the last 30 s of exercise and during the following recovery localized acquisition of dynamic 31P MRS data was performed again. Overview about the timing of 31P MRS data acquisition is given also in the Figure 1.
At the end our comprehensive dynamic 31P MRS protocol yielded basal concentrations of phosphorus containing metabolites at rest, forward rate of oxidative ATP synthesis (FATP) and creatine kinase (FCK) at rest and during exercise, depletion of PCr during the exercise, time constant and rate of PCr resynthesis during recovery (τPCr, VPCr), maximal rate of oxidative phosphorylation, i.e., mitochondrial capacity (Qmax), and time course of intracellular pH changes in skeletal muscle.
Data Processing, Calculations, and Statistical Analysis
All measured spectra were analyzed using the jMRUI (Java-Based Magnetic Resonance User Interface; version 5) with the AMARES (Advanced Method for Accurate, Robust, and Efficient Spectral Fitting) time-domain fitting algorithm (Vanhamme et al., 1997).
1H-MRS
Spectral lines of acetylcarnitine, carnosine, IMCL, and water were fitted as a single Lorentzian. Lipid signals surrounding the acetylcarnitine peak were fitted with a constrained frequency to avoid their influence on fitted acetylcarnitine (Klepochová et al., 2017).
The C2-H peak of carnosine, resonating at 8 ppm, was fitted after removing residual peaks of water and lipids by a Hankel Lanczos Squares Singular Values Decomposition (HSVLD) algorithm, as it was shown to be the more robust of two available carnosine signals (Just Kukurová et al., 2016).
Using the water signal as an internal reference, the concentration of acetylcarnitine and carnosine were calculated according to the formula for millimolar concentrations in 1 kg of wet weight of tissue (mmol/kg ww):
where S is the signal intensities of H2O-water, m is the metabolite (e.g., acetylcarnitine or carnosine), nH2O is the number of corresponding equivalent protons in water (n = 2) and nm is for the acetylcarnitine (n = 3) or carnosine (n = 1) molecule, CF is the correction factors for T1 and T2 relaxations, CH2O = 55.56 mol/L is the concentration of the water, and wH2O is the approximate water content of skeletal muscle tissue, i.e., 0.77 L/kg wet weight of tissue.
IMCL CH2 (methylene) at 1.3 ppm was fitted, corrected for T1 and T2 relaxation effects, and expressed as a percentage of water content.
Literature relaxation times were used for T1 and T2 corrections (Bottomley et al., 1984; Ren et al., 2011; Just Kukurová et al., 2016).
31P-MRS
The resonance lines of PCr, Pi, Pi2, glycerol-phosphocholine (GPC), and glycerol-phosphoethanolamine (GPE) were fitted as single Lorentzians, whereas γ- and α-ATP were fitted as doublets and β-ATP as a triplet. The concentration of phosphodiesters (PDEs) was calculated as the sum of GPC and GPE. The frequency of the Pi2 peak was constrained with respect to the expected frequency difference between Pi2 and Pi to ~0.4 ppm (Kan et al., 2010). For the FAST experiment, the linewidths of PCr and Pi were determined from the highest signal-to-noise ratio spectra and then constrained to ±5 Hz (Tušek Jelenc et al., 2016; Valkovič et al., 2016). The apparent relaxation times () and pseudo first-order exchange rate constants of the CK reaction (kCK) and ATP synthesis reaction (kATP) were calculated according to the equations for the FAST experiment (Bottomley et al., 2002). For the quantification of metabolite concentrations, a cellular γ-ATP concentration of 8.2 mM was assumed (Taylor et al., 1986). Forward metabolic fluxes (FATP, FCK) were the product of kATP and kCK times the concentration of Pi and PCr, respectively (Valkovič et al., 2017).
The shift in resonance position between PCr and Pi signals was used to calculate intramyocellular pH (Moon and Richards, 1973) according to the modified Henderson-Hasselbalch equation. ADP concentration was calculated according to the modified method described by Kemp et al. (1993b), assuming that 15% of total creatine was not phosphorylated in the resting state.
To calculate the time constant of PCr resynthesis (τPCr), the PCr signal changes during the recovery period of the dynamic experiment were fitted to a mono-exponential function. This was used to calculate the initial PCr recovery rate (VPCr), which roughly represents ATP turnover at the end of exercise and the maximal rate of oxidative phosphorylation, i.e., mitochondrial capacity (Qmax), was calculated according to the ADP-based model of Michaelis and Menten (Kemp et al., 1993b; Valkovič et al., 2016).
Statistical Analysis
Data are presented as means ± standard deviations and were compared between the two groups by an unpaired Student's t-test, controlling for multiple comparisons using Benjamini–Hochberg procedure (Benjamini and Hochberg, 1995). The relationships between metabolic parameters determined using 1H and 31P-MRS were analyzed by linear correlations using Pearson's correlation coefficient (two-tailed probability values) to estimate the strength of the relationship. The correlation coefficient of an absolute value of 0.415, which corresponded to a 95% confidence agreement, was taken as significant. A stepwise regression analysis for the dependent variable, VO2max, was performed using the independent variables (i.e., τPCr, VPCr, Qmax, PDE, GPC, IMCL, acetylcarnitine). The results were considered statistically significant at p < 0.05 (t-test) with Benjamini–Hochberg adjusted p < 0.25.
Results
Skeletal Muscle Metabolism of Triathletes
As expected triathletes presented with a significantly higher maximal aerobic capacity (VO2max; p < 0.001) when compared to healthy active volunteers.
Typical 1H-MR spectra acquired in a triathlete and normally active volunteer, are depicted in Figure 2 and representative 31P-MR spectra acquired at rest, during the exercise-recovery experiment, and during the FAST experiment are depicted in Figure 3. Triathletes differed in several MRS-derived metabolic read-outs. In particular, the triathlete group exhibited a significantly higher IMCL (p = 0.009), higher initial rate of PCr resynthesis (VPCr) during the recovery period (p = 0.006), shorter PCr recovery time constant (τPCr) (p = 0.0007), and higher maximal oxidative flux (Qmax) (p = 0.0003). Detailed results of both participating study groups are displayed in Figure 4 and listed in Table 1.
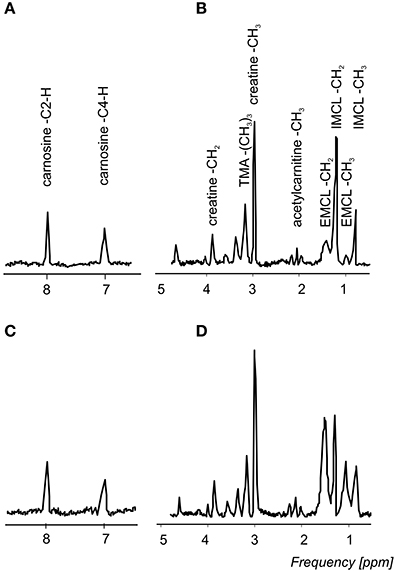
Figure 2. A representative 1H-MRS spectrum from a triathlete (A,B) and a healthy active volunteer (C,D) acquired from the vastus lateralis muscle. (A,C) present carnosine spectral lines at 7 and 8 ppm and (B,D) show the trimethyl ammonium (TMA) groups of carnitine, acetylcarnitine, and choline at 3.20 ppm, creatines at 3.03, and 3.9 ppm, acetylcarnitine at 2.13 ppm, and extramyocellular (EMCL) and intramyocellular (IMCL) lipid resonance lines at 1.5 and 1.3 ppm (CH2 group). For better visualization, a 1.5-Hz Lorentzian apodization filter was applied to reduce noise in the spectra. All spectra were normalized with respect to the water signal.
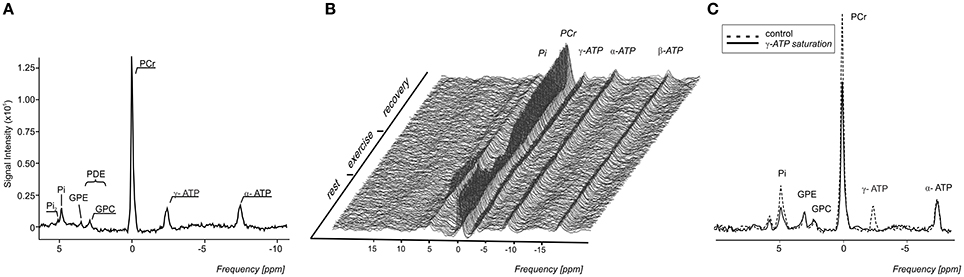
Figure 3. (A) A representative highly spectrally resolved 31P-MRS spectra. For better visualization, a 1,5-Hz Lorentzian apodization filter was applied to reduce noise in the spectra. (B) Time course of a 31P MR spectra during a knee extension exercise with depicted depletion of the PCr signal and its subsequent re-synthesis during the recovery period. (C) Saturation transfer spectra showing the effect of γ-ATP saturation, at ~-2.48 ppm (solid line) on the Pi signal compared to the control experiment with saturation at ~12.52 ppm (dashed line).
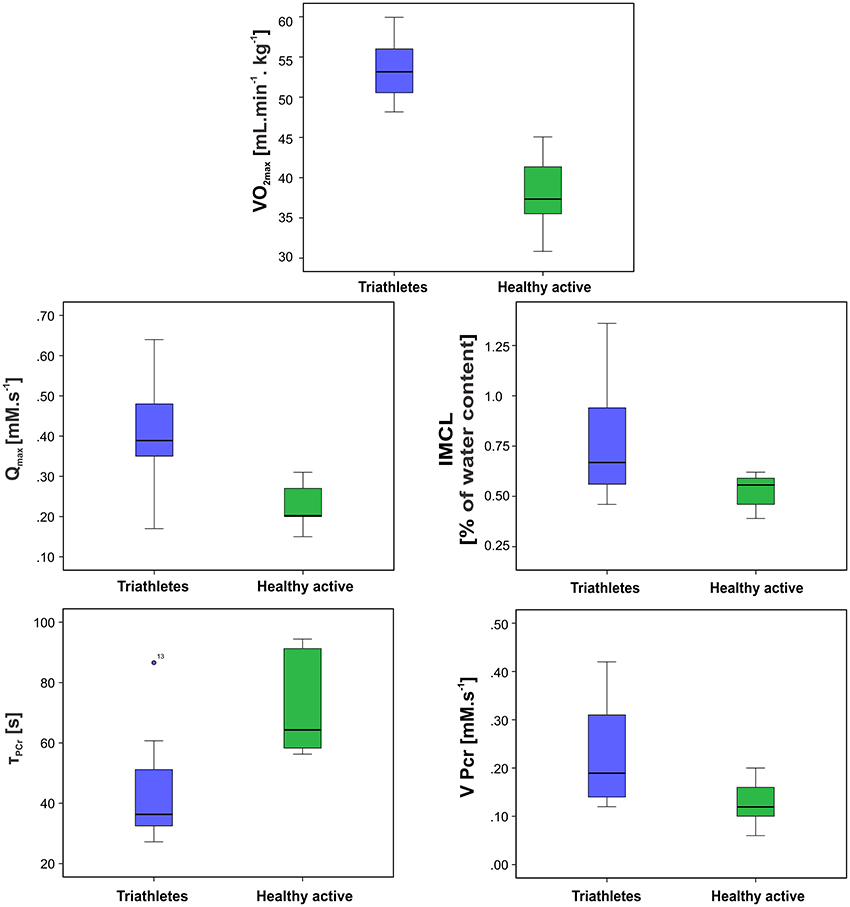
Figure 4. Box plots depicting the significantly different parameters between the two groups. The solid lines represent the median, boxes represent lower and upper quartiles, and whiskers the minimum and maximum. Outliers are denoted by circles. The outliers were also taken into account for all statistical tests.
Correlations Between the Measured Parameters
Bivariate correlation analysis of pooled data revealed, that VO2max was positively correlated with Qmax (r = 0.66, p = 0.0006), VPCr (r = 0.48, p = 0.02), and PCr concentration measured at the end of recovery (r = 0.48, p = 0.02), and negatively with τPCr (r = −0.64, p = 0.001), PDE (r = −0.57, p = 0.004), and GPC (r = −0.48, p = 0.02). GPC, and PDE were positively correlated with BMI (both, r = 0.54, p = 0.007), and, moreover, GPC was positively correlated with age (r = 0.44, p = 0.03).
Several correlations were also found between the metabolic parameters extracted from the 31P-MRS measurements and the parameters from 1H-MRS, specifically acetylcarnitine was negatively correlated with GPC and PDE (r = −0.43, p = 0.04). IMCL was negatively correlated with τPCr (r = −0.52, p = 0.01), and positively with Qmax (r = 0.55, p = 0.006) and VPCr (r = 0.46, p = 0.02). Representative bivariate correlations are depicted in Figure 5.
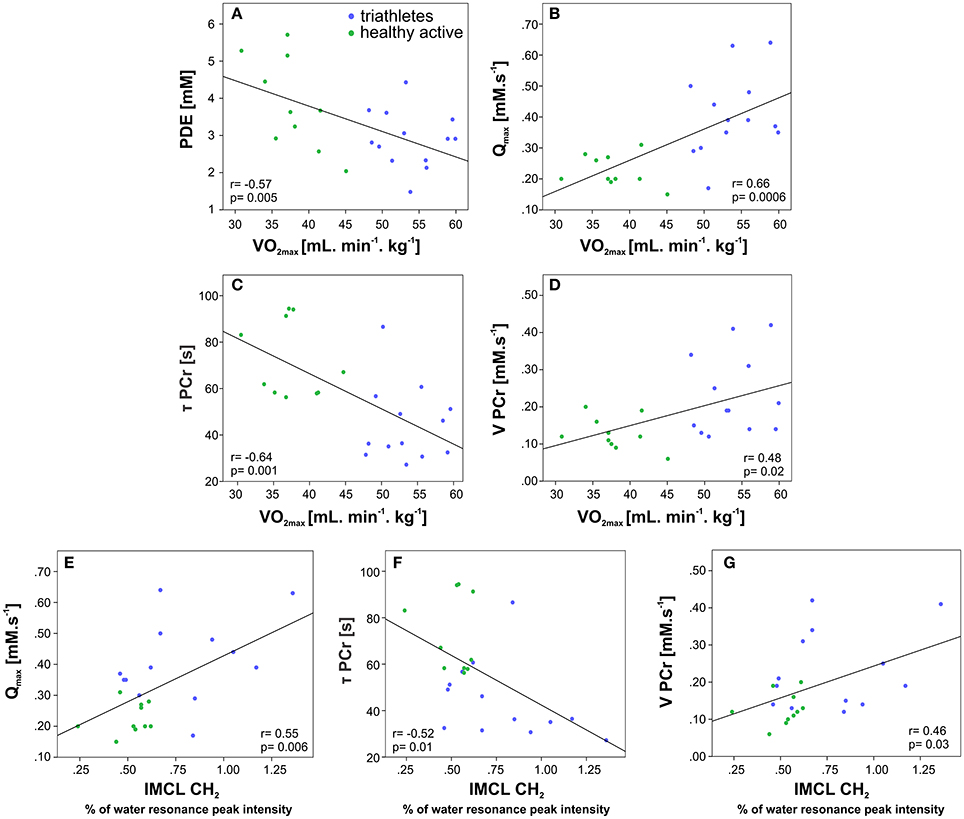
Figure 5. Plots of correlations between parameters measured by spiroergometry and 31P-MRS in triathletes and healthy active volunteers: (A) maximum oxygen uptake (VO2max) with the phosphodiesters (PDE); (B) VO2max with mitochondrial capacity (Qmax); (C) VO2max with PCr recovery rate time constant (τPCr); (D) VO2max with initial rate of PCr resynthesis (VPCr) during the recovery period; and between the parameters measured by 1H- and 31P-MRS: (E) intramyocellular lipid content (IMCL) with Qmax; (F) IMCL with τPCr and (G) IMCL with V PCr.
Multivariate stepwise regression analysis identified [PDE] (p = 0.004) as the strongest independent predictor of VO2max.
Discussion
The purpose of this study was to investigate the value of different MRS-derived read-outs of oxidative skeletal muscle metabolism for the prediction of whole body maximal oxygen uptake in endurance trained athletes and healthy active volunteers by non-invasive multi-nuclear MRS. The measurements were performed on vastus lateralis muscle, as this muscle is most representative for whole body oxygen uptake during cycling and it is mostly frequently studied region of skeletal musculature in men in the past. We present combined results and correlation analysis from localized, static 1H-MRS and static as well as dynamic 31P-MRS, measured in one experimental protocol for the first time.
The most important intergroup findings in our study were: In the triathlete group, in line with a 42% higher VO2max, we found a 50% higher IMCL content, 36% faster PCr resynthesis (τPCr), 76% higher VPCr, and a 78% higher mitochondrial capacity (Qmax) in vastus lateralis muscle. Besides, even though there was no significant difference in PDE found in the intergroup analysis, regression analysis of pooled data identified PDE as the strongest independent predictor of VO2max.
The finding of significantly higher levels of IMCL in the vastus lateralis muscle in the triathlete cohort is in good agreement with the literature. This has previously been described as the athlete's paradox, in which despite a higher amount of IMCL in the skeletal muscles of endurance trained individuals, no impairment in insulin sensitivity was observed (Thamer et al., 2003; Machann et al., 2004). In a sedentary population, increased IMCL has been associated with insulin resistance in human obesity and in T2DM, but endurance-trained athletes have an IMCL content similar to that observed in insulin-resistant obese and T2DM subjects (Dubé et al., 2008). Higher levels of IMCL in the triathlete group are potentially a consequence of the increased need for and efficiency of fatty acid oxidation during long-lasting exercise bouts (Popadic Gacesa et al., 2017).
In the context of higher amounts of IMCL in the skeletal muscle of endurance-trained athletes, together with the indication of enhanced fatty acid oxidation and its importance in maintaining insulin sensitivity, we studied muscle acetylcarnitine levels within our study population. In our study, there was no significant between-group difference. To date, only a few studies have investigated differences in concentrations of acetylcarnitine/carnitine in trained groups of volunteers. But, the link between T2DM and the concentration of acetylcarnitine has been more intensively studied (Mingrone, 2004). Still, slightly higher acetylcarnitine concentrations were observed using biopsy samples from the VL muscle in slow-twitch muscle fibers, probably due to the greater potential for fat oxidation in those types of fibers (Constantin-Teodosiu et al., 1996). This would suggest higher concentrations of acetylcarnitine in the triathlete group. Significantly higher acetylcarnitine concentrations measured by in vivo 1H-MRS in the vastus lateralis but not in the soleus muscle were recently reported in a group of moderately trained volunteers when compared to normally active population (Klepochová et al., 2017). Lindeboom et al. (2014) did not find a significant difference in acetylcarnitine concentration between endurance-trained and lean sedentary or obese sedentary volunteers and Seiler et al. (2015), in their 1H-MRS study with cohort of trained and untrained subjects investigating exercise-induced acetylcarnitine metabolism, did not find differences in acetylcarnitine concentrations in the baseline measurement before exercise, as well. Another important confounder could be the time point of acetylcarnitine measurement in the daily routine and the metabolic conditions of volunteers. A recent study showed significant differences between acetylcarnitine concentrations measured in overnight fasting conditions and metabolite concentrations after lunch (Klepochová et al., 2017). Therefore, it is difficult to compare the results of acetylcarnitine concentration measurements from other studies when the measurements were not recorded at the same time point during the day and/or under the same physical and nutritional conditions. It has already been shown that detection and quantification of acetylcarnitine is challenging (even at 7T) and our data emphasize the need for strict standardization of measurement time, dietary conditions, and also physical activity for the measurement of acetylcarnitine/carnitine. Further studies are necessary to better understand whole-body acetylcarnitine metabolism in humans.
An athletes's involvement in endurance training should stimulate mitochondrial biogenesis (Groennebaek and Vissing, 2017) as a result of training-specific adaptations to a high-volume load compared with sedentary subjects (McCully et al., 1989). This is in agreement with the higher whole body aerobic capacity (VO2max), and faster oxidative metabolism demonstrated by 31P MRS, in our study.
It is also known that untrained people possess an approximately uniformly distributed muscle fiber spectrum (Staron et al., 2000), while endurance-trained athletes show more slow-twitch fibers in muscles with high oxidative, low glycolytic capacity (Bogdanis, 2012). PCr recovery kinetics can indicate differences in functional mitochondrial performance (Meyer, 1988; Blei et al., 1993; Pesta et al., 2013) and individuals with faster PCr resynthesis typically exhibit greater fatigue-resistance during high-intensity exercise. Our finding of 36% shorter τPCr is in agreement with Pesta et al. (2013), who reported a 40% faster PCr recovery in endurance-trained athletes than in untrained individuals. Layec et al. (2010) showed faster PCr resynthesis rate and a higher Qmax in endurance-trained men, which is also in good agreement with our results, where we found a 77% higher VPCr and 78% higher Qmax in triathletes. Acidic intracellular pH at the end of exercise could significantly prolong the PCr resynthesis rate (Walter et al., 1997; van den Broek et al., 2007), but we have observed no acidification in either of the groups. The slightly higher pH of triathletes at the end of exercise is in agreement with previous reports on endurance trained subjects (Kent-Braun et al., 1990; Layec et al., 2009) and could potentially suggest faster proton efflux demonstrated earlier in quadriceps of professional road cyclists (Hug et al., 2005).
Carnosine content has been shown to be an indicator of the distribution of muscle fibers since slow-twitch fibers should contain lower concentrations of carnosine than fast-twitch fibers (Baguet et al., 2011). However, we did not find a statistically significant difference between our two groups of volunteers in carnosine content.
Next to the already established and biochemically proven role of specific metabolites (acetylcarnitine, carnosine, PCr, PCr metabolism) in the endurance training-involved adaptation of skeletal muscle metabolism and physiology, our multi-parametric analysis highlighted, again, the link between skeletal muscle PDEs and whole-body metabolism (Szendroedi et al., 2011). Similar to the findings in overweight-to-obese sedentary volunteers (Valkovič et al., 2016), lower concentrations of PDE are linked to higher whole-body and/or skeletal muscle metabolic capacity. Slower PCr recovery rate after exercise alongside increased PDE levels alongside was previously found in the elderly (Waters et al., 2003) patients with lipodystrophy (Sleigh et al., 2012), and hypothyroid patients (Rana et al., 2012). Although the role of PDEs, consisting of GPC and GPE, in the skeletal muscle energy metabolism is not totally clear yet, it is believed to be potentially acting as an indirect measure of oxidative stress on the mitochondrial membrane (Taylor et al., 1997; Schunk et al., 1999). Increased levels of skeletal muscle PDEs have been found also in the patients with Duchenne muscular dystrophy (Kemp et al., 1993a; Hooijmans et al., 2017). Moreover, in a model of membrane defect of Alzheimer's disease Faber et al. reported that an inhibition of oxidative phosphorylation causes accumulation of GPC through accelerated PC turnover (Farber et al., 2000). Rather straightforward and uncomplicated muscle-specific PDE measurement by 31P-MRS advocates further detailed and specific studies of this potentially surrogate marker of training and metabolic status. Improved spectral resolution and the observed split into single GPC and GPE signals favors the application of higher field-strength in this regard.
MVC was used in this study as a measure to set the exercise workload, as this takes into account direct power production of the muscle involved in the given exercise. However, one could argue that the endurance component of training status of our triathletes group could, therefore, be less pronounced in our dynamic examinations, as we do not consider the difference in power output connected to the lactate threshold. On the other hand, the measured intracellular pH at the end of exercise did not show substantial acidosis in either of the groups. Using a whole-body metabolism, such as VO2max, for exercise load adjustment could pronounce the endurance component in our comparison. However, the dynamic examination would be less specifically calibrated, and thus, would likely become anaerobic, which would contradict our dynamic Pi-to-ATP measurements. It also needs to be mentioned that even under aerobic steady-state exercise conditions, there is still a minor glycolytic component present, which cannot be accounted for under our experimental conditions. However, as the total amount of ATP produced through the glycolysis is negligible in comparison to ATP produced by oxidative phosphorylation, the measured 31P parameters can be considered primarily oxidative.
In conclusion, based on this non-invasive 1H and 31P-MRS study of skeletal muscle metabolic differences between endurance-trained and normally active volunteers, as well as the multivariate analysis of skeletal muscle and whole-body metabolic read-outs, we cannot suggest a single MRS-based parameter as an exclusive biomarker of skeletal muscle fitness and training status that could be, on its own directly usable in sport medicine clinics. It is, rather, a combination of different parameters, such as basal IMCL, acetylcarnitine, and PDE levels as well as measures of PCr metabolism following acute exercise challenge that can be assessed during a single, clinically acceptable multinuclear MRS session. This suggests the need for further broad population cross-sectional and/or focused interventional studies. In these further experiments, the special focus should be placed on the specific role of training regiment, metabolic status, dietary supplementation, and/or therapeutic intervention.
Ethics Statement
This study was carried out in accordance with the recommendations of Ethics Committee with written informed consent from all subjects. All subjects gave written informed consent in accordance with the Declaration of Helsinki. The protocol was approved by The Ethics Committee of the Medical University of Vienna.
Author Contributions
All persons who meet authorship criteria are listed as authors, and all authors certify that they have participated sufficiently in the work to take public responsibility for the content, including participation in the concept, design, analysis, writing, or revision of the manuscript. RK conception and design of study, acquisition of data, analysis and/or interpretation of data, drafting and revising the manuscript, final approval of the version of the manuscript to be published; LV conception and design of study, analysis and/or interpretation of data, drafting the manuscript, final approval of the version of the manuscript to be published; TH conception and design of study, acquisition of data, analysis and/or interpretation of data, final approval of the version of the manuscript to be published; CT: acquisition of data, analysis and/or interpretation of data, final approval of the version of the manuscript to be published; NB, HT, ST, and MiK drafting the manuscript, revising the manuscript critically for important intellectual content, final approval of the version of the manuscript to be published; MaK conception and design of study, analysis and/or interpretation of data, drafting and revising the manuscript critically for important intellectual content, final approval of the version of the manuscript to be published.
Funding
This study was supported by the Anniversary Fund of the Austrian National Bank (#15363 to MaK, #15455 to LV) and the Christian Doppler Society (MOLIMA to ST), by a Sir Henry Dale Fellowship from the Welcome Trust and the Royal Society (#098436/Z/12/B), by Slovak Research and Development Agency (#15-0029), and by VEGA (#2/0001/17).
Conflict of Interest Statement
The authors declare that the research was conducted in the absence of any commercial or financial relationships that could be construed as a potential conflict of interest.
Acknowledgments
The authors would like to thank all the participants for their patience and cooperation.
References
Arnold, D. L., Matthews, P. M., and Radda, G. K. (1984). Metabolic recovery after exercise and the assessment of mitochondrial function in vivo in human skeletal muscle by means of 31P NMR. Magn. Reson. Med. 1, 307–315. doi: 10.1002/mrm.1910010303
Baguet, A., Everaert, I., Hespel, P., Petrovic, M., Achten, E., and Derave, W. (2011). A new method for non-invasive estimation of human muscle fiber type composition. PLoS ONE 6:e21956. doi: 10.1371/journal.pone.0021956
Balaban, R. S., and Koretsky, A. P. (2011). Interpretation of 31P NMR saturation transfer experiments: why you can't see might confuse you. Focus on standard magnetic resonance-based measurements of the Pi to ATP rate do not index the rate of oxidative phosphorylation in cardiac and skeletal musc. Am. J. Physiol. Cell Physiol. 301, 12–15. doi: 10.1152/ajpcell.00100.2011
Benjamini, Y., and Hochberg, Y. (1995). Controlling the false discovery rate: a practical and powerful approach to multiple testing. J. R. Stat. Soc. B Methodol. 57, 289–300.
Blei, M. L., Conley, K. E., and Kushmerick, M. J. (1993). Separate measures of ATP utilization and recovery in human skeletal muscle. J. Physiol. 451, 203–222. doi: 10.1113/jphysiol.1993.sp019673
Bogdanis, G. C. (2012). Effects of physical activity and inactivity on muscle fatigue. Front. Physiol. 3:142. doi: 10.3389/fphys.2012.00142
Boska, M. (1991). Estimating the ATP cost of force production in the human gastrocnemius/soleus muscle group using 31P MRS and 1H MRI. NMR Biomed. 4, 173–181. doi: 10.1002/nbm.1940040404
Bottomley, P. A., Foster, T. B., and Darrow, R. D. (1984). Depth-Resolved Surface-Coil Spectroscopy (DRESS) for in vivo 1H, 31P, and 13C NMR. J. Magn. Reson. 59, 338–342.
Bottomley, P. A., Ouwerkerk, R., Lee, R. F., and Weiss, R. G. (2002). Four-Angle Saturation Transfer (FAST) method for measuring creatine kinase reaction rates in vivo. Magn. Reson. Med. 47, 850–863. doi: 10.1002/mrm.10130
Brindle, K. M., Blackledge, M. J., Challiss, R. A., and Radda, G. K. (1989). 31P NMR magnetization-transfer measurements of ATP turnover during steady-state isometric muscle contraction in the rat hind limb in vivo. Biochemistry 28, 4887–4893. doi: 10.1021/bi00437a054
Chance, B., Eleff, S., and Leigh, J. S. (1980). Noninvasive, nondestructive approaches to cell bioenergetics. Proc. Natl. Acad. Sci. U.S.A. 77, 7430–7434. doi: 10.1073/pnas.77.12.7430
Chance, B., Eleff, S., Leigh, J. S., Sokolow, D., and Sapega, A. (1981). Mitochondrial regulation of phosphocreatine/inorganic phosphate ratios in exercising human muscle: a gated 31P NMR study. Proc. Natl. Acad. Sci. U.S.A. 78, 6714–6718.
Constantin-Teodosiu, D. (2013). Regulation of muscle pyruvate dehydrogenase complex in insulin resistance: effects of exercise and dichloroacetate. Diabetes Metab. J. 37, 301–314. doi: 10.4093/dmj.2013.37.5.301
Constantin-Teodosiu, D., Howell, S., and Greenhaff, P. L. (1996). Carnitine metabolism in human muscle fiber types during submaximal dynamic exercise. J. Appl. Physiol. 80, 1061–1064. doi: 10.1152/jappl.1996.80.3.1061
Crowther, G. J., Jubrias, S. A., Gronka, R. K., and Conley, K. E. (2002). A ‘functional Biopsy’ of muscle properties in sprinters and distance runners. Med. Sci. Sports Exerc. 34, 1719–1724. doi: 10.1249/01.MSS.0000035047.33651.E1
Defronzo, R. A., Jacot, E., Jequier, E., Maeder, E., Wahren, J., and Felber, J. P. (1981). The effect of insulin on the disposal of intravenous glucose results from indirect calorimetry and hepatic and femoral venous catheterization. Diabetes 30, 1000–1007. doi: 10.2337/diab.30.12.1000
DeFronzo, R. A., and Tripathy, D. (2009). Skeletal muscle insulin resistance is the primary defect in type 2 diabetes. Diabetes Care 32(Suppl. 2), S157-S163. doi: 10.2337/dc09-S302
Dubé, J. J., Amati, F., Stefanovic-Racic, M., Toledo, F. G., Sauers, S. E., and Goodpaster, B. H. (2008). Exercise-induced alterations in intramyocellular lipids and insulin resistance: the athlete's paradox revisited. Am. J. Physiol. Endocrinol. Metab. 294, E882–E888. doi: 10.1152/ajpendo.00769.2007
Farber, S. A., Slack, B. E., and Blusztajn, J. K. (2000). Acceleration of phosphatidylcholine synthesis and breakdown by inhibitors of mitochondrial function in neuronal cells: a model of the membrane defect of Alzheimer's disease. FASEB J. 14, 2198–2206. doi: 10.1096/fj.99-0853
From, A. H. L., and Ugurbil, K. (2011). Standard magnetic resonance-based measurements of the Pi → ATP rate do not index the rate of oxidative phosphorylation in cardiac and skeletal muscles. Am. J. Physiol. Cell Physiol. 301, C1–C11. doi: 10.1152/ajpcell.00345.2010
Fujimoto, T., Kemppainen, J., Kalliokoski, K. K., Nuutila, P., Ito, M., and Knuuti, J. (2003). Skeletal muscle glucose uptake response to exercise in trained and untrained men. Med. Sci. Sports Exerc. 35, 777–783. doi: 10.1249/01.MSS.0000065070.49295.C0
Groennebaek, T., and Vissing, K. (2017). Impact of resistance training on skeletal muscle mitochondrial biogenesis, content, and function. Front. Physiol. 8:713. doi: 10.3389/fphys.2017.00713
Hooijmans, M. T., Niks, E. H., Burakiewicz, J., Verschuuren, J. J., Webb, A. G., and Kan, H. E. (2017). Elevated phosphodiester and T2 levels can be measured in the absence of fat infiltration in duchenne muscular dystrophy patients. NMR Biomed. 30, 1–8. doi: 10.1002/nbm.3667
Hug, F., Bendahan, D., Le Fur, Y. J., Cozzone, P., and Grélot, L. (2005). Metabolic recovery in professional road cyclists: a 31P-MRS study. Med. Sci. Sports Exerc. 37, 846–852. doi: 10.1249/01.MSS.0000162616.20085.B4
Johansen, L., and Quistorff, B. (2003). 31P-MRS characterization of sprint and endurance trained athletes. Int. J. Sports Med. 24, 183–189. doi: 10.1055/s-2003-39085
Just Kukurová, I., Valkovič, L., Ukropec, J., de Courten, B., Chmelík, M., Ukropcová, B., et al. (2016). Improved spectral resolution and high reliability of in vivo 1 H MRS at 7 T allow the characterization of the effect of acute exercise on carnosine in skeletal muscle. NMR Biomed. 29, 24–32. doi: 10.1002/nbm.3447
Kan, H. E., Klomp, D. W. J., Wong, C. S., Boer, V. O., Webb, A. G., Luijten, P. R., et al. (2010). In vivo 31P MRS detection of an alkaline inorganic phosphate pool with short T1 in human resting skeletal muscle. NMR Biomed. 23, 995–1000. doi: 10.1002/nbm.1517
Kautzky-Willer, A., Krssak, M., Winzer, C., Pacini, G., Tura, A., Farhan, S., Wagner, O., et al. (2003). Increased intramyocellular lipid concentration identifies impaired glucose metabolism in women with previous gestational diabetes. Diabetes 52, 244–251. doi: 10.2337/diabetes.52.2.244
Kemp, G. J., Ahmad, R. E., Nicolay, K., and Prompers, J. J. (2015). Quantification of skeletal muscle mitochondrial function by 31P magnetic resonance spectroscopy techniques: a quantitative review. Acta Physiol. 213, 107–144. doi: 10.1111/apha.12307
Kemp, G. J., and Radda, G. K. (1994). Quantitative interpretation of bioenergetic data from 31P and 1H magnetic resonance spectroscopic studies of skeletal muscle: an analytical review. Magn. Reson. Q. 10, 43–63.
Kemp, G. J., Taylor, D. J., Dunn, J. F., Frostick, S. P., and Radda, G. K. (1993a). Cellular energetics of dystrophic muscle. J. Neurol. Sci. 116, 201–206. doi: 10.1016/0022-510X(93)90326-T
Kemp, G. J., Taylor, D., Thompson, C. H., Hands, L. J., Rajagopalan, B., Radda, G. K., et al. (1993b). Quantitative-Analysis by P-31 magnetic-resonance spectroscopy of abnormal mitochondrial oxidation in skeletal-muscle during recovery from exercise. NMR Biomed. 6, 302–310. doi: 10.1002/nbm.1940060504
Kent-Braun, J. A., McCully, K. K., and Chance, B. (1990). Metabolic effects of training in humans: a 31P-MRS study. J. Appl. Physiol. 69, 1165–1170. doi: 10.1152/jappl.1990.69.3.1165
Klepochová, R., Valkovič, L., Gajdošík, M., Hochwartner, T., Tschan, H., Krebs, M., et al. (2017). Detection and alterations of acetylcarnitine in human skeletal muscles by 1H MRS at 7 T. Invest. Radiol. 52, 412–418. doi: 10.1097/RLI.0000000000000355
Krššák, M., Petersen, K. F., Dresner, A., DiPietro, L., Vogel, S. M., Rothman, D. L., et al. (1999). Intramyocellular lipid concentrations are correlated with insulin sensitivity in humans: a 1 H NMR spectroscopy study. Diabetologia 42, 113–116. doi: 10.1007/s001250051123
Layec, G., Bringard, A., Le Fur, Y., Vilmen, C., Micallef, J.-P., Perry, S., et al. (2010). Comparative determination of energy production rates and mitochondrial function using different 31P MRS quantitative methods in sedentary and trained subjects. NMR Biomed. 24, 425–438. doi: 10.1002/nbm.1607
Layec, G., Bringard, A., Vilmen, C., Micallef, J.-P., Le Fur, Y., Perry, S., et al. (2009). Does oxidative capacity affect energy cost? An in vivo MR investigation of skeletal muscle energetics. Eur. J. Appl. Physiol. 106, 229–242. doi: 10.1007/s00421-009-1012-y
Lindeboom, L., Nabuurs, C. I., Hoeks, J., Brouwers, B., Phielix, E., Kooi, M. E., et al. (2014). Long-echo time MR spectroscopy for skeletal muscle acetylcarnitine detection. J. Clin. Invest. 124, 4915–4925. doi: 10.1172/JCI74830
Machann, J., Häring, H., Shick, F., and Stumvoll, M. (2004). Intramyocellular lipids and insulin resistance. Diabetes Obes. Metab. 6, 239–248. doi: 10.1111/j.1462-8902.2004.00339.x
McCully, K. K., Boden, B. P., Tuchler, M., Fountain, M. R., and Chance, B. (1989). Wrist flexor muscles of elite rowers measured with magnetic resonance spectroscopy. J. Appl. Physiol. 67, 926–932. doi: 10.1152/jappl.1989.67.3.926
Meyer, R. A. (1988). A linear model of muscle respiration explains monoexponential phosphocreatine changes. Am. J. Physiol. 254, C548–C553.
Meyer, R. A., Kuchmerick, M. J., and Brown, T. R. (1982). Application of 31P-NMR spectroscopy to the study of striated muscle metabolism. Am. J. Physiol. 242, C1-C11.
Mingrone, G. (2004). Carnitine in type 2 diabetes. Ann. N.Y. Acad. Sci. 1033, 99–107. doi: 10.1196/annals.1320.009
Moon, R. B., and Richards, J. H. (1973). Determination of intracellular pH by 31P magnetic resonance. J. Biol. Chem. 248, 7276–7278.
Pesta, D., Paschke, V., Hoppel, F., Kobel, C., Kremser, C., Esterhammer, R., et al. (2013). Different metabolic responses during incremental exercise assessed by localized 31p mrs in sprint and endurance athletes and untrained individuals. Int. J. Sports Med. 34, 669–675. doi: 10.1055/s-0032-1327648
Petersen, K. F., Dufour, S., Befroy, D., Garcia, R., and Shulman, G. I. (2004). Impaired mitochondrial activity in the insulin-resistant offspring of patients with type 2 diabetes. New Engl. J. Med. 350, 664–671. doi: 10.1056/NEJMoa031314
Pette, D. (1998). Training effects on the contractile apparatus. Acta Physiol. Scand. 162, 367–376. doi: 10.1046/j.1365-201X.1998.0296e.x
Popadic Gacesa, J., Schick, F., Machann, J., and Grujic, N. (2017). Intramyocellular lipids and their dynamics assessed by 1H magnetic resonance spectroscopy. Clin. Physiol. Funct. Imaging 37, 558-556. doi: 10.1111/cpf.12346
Rana, P., Sripathy, G., Varshney, A., Kumar, P., Memita Devi, M., Marwaha, R. K., et al. (2012). Phosphorous magnetic resonance spectroscopy-based skeletal muscle bioenergetic studies in subclinical hypothyroidism. J. Endocrinol. Invest. 35, 129–134. doi: 10.3275/7676
Ren, J., Sherry, A. D., and Malloy, C. R. (2011). 1H MRS of intramyocellular lipids in soleus muscle at 7T: spectral simplification by using long echo times without water suppression. Magn. Reson. Med. 64, 662–671. doi: 10.1002/mrm.22345
Saltin, B., Henriksson, J., Nygaard, E., Andersen, P., and Jansson, E. (1977). Fiber types and metabolic potentials of skeletal muscles in sedentary man and endurance runners. Ann. N.Y. Acad. Sci. 301, 3–29.
Schmid, A. I., Schrauwen-Hinderling, V. B., Andreas, M., Wolzt, M., Moser, E., and Roden, M. (2012). Comparison of measuring energy metabolism by different 31P-magnetic resonance spectroscopy techniques in resting, ischemic, and exercising muscle. Magn. Reson. Med. 67, 898–905. doi: 10.1002/mrm.23095
Schunk, K., Pitton, M., Düber, C., Kersjes, W., Schadmand-Fischer, S., and Thelen, M. (1999). Dynamic phosphorus-31 magnetic resonance spectroscopy of the quadriceps muscle: effects of age and sex on spectroscopic results. Invest. Radiol. 34, 116–125. doi: 10.1097/00004424-199902000-00004
Seiler, S. E., Koves, T. R., Gooding, J. R., Wong, K. E., Stevens, R. D., Ilkayeva, O. R., et al. (2015). Carnitine acetyltransferase mitigates metabolic inertia and muscle fatigue during exercise. Cell Metab. 22, 65–76. doi: 10.1016/j.cmet.2015.06.003
Sleigh, A., Stears, A., Thackray, K., Watson, L., Gambineri, A., Nag, S., et al. (2012). Mitochondrial oxidative phosphorylation is impaired in patients with congenital lipodystrophy. J. Clin. Endocrinol. Metab. 97, E438–E442. doi: 10.1210/jc.2011-2587
Staron, R. S., Hagerman, F. C., Hikida, R. S., Murray, T. F., Hostler, D. P., Crill, M. T., et al. (2000). Fiber type composition of the vastus lateralis muscle of young men and women. J. Histochem. Cytochem. 48, 623–629. doi: 10.1177/002215540004800506
Szendroedi, J., Schmid, A. I., Chmelik, M., Krssak, M., Nowotny, P., Prikoszovich, T., et al. (2011). Skeletal muscle phosphodiester content relates to body mass and glycemic control. PLoS ONE 6:e21846. doi: 10.1371/journal.pone.0021846
Taylor, D. J., Bore, P. J., Styles, P., Gadian, D. G., and Radda, G. K. (1983). Bioenergetics of intact human muscle. A 31P nuclear magnetic resonance study. Mol. Biol. Med. 1, 77–94.
Taylor, D. J., Kemp, G. J., Thompson, C. H., and Radda, G. K. (1997). Ageing: effects on oxidative function of skeletal muscle in vivo. Mol. Cell. Biochem. 174, 321–324.
Taylor, D. J., Styles, P., Matthews, P. M., Arnold, D. A., Gadian, D. G., Bore, P., et al. (1986). Energetics of human muscle: exercise-induced ATP depletion. Magn. Reson. Med. 3, 44–54. doi: 10.1002/mrm.1910030107
Thamer, C., Machann, J., Bachmann, O., Haap, M., Dahl, D., Wietek, B., et al. (2003). Intramyocellular lipids: anthropometric determinants and relationships with maximal aerobic capacity and insulin sensitivity. J. Clin. Endocrinol. Metab. 88, 1785–1791. doi: 10.1210/jc.2002-021674
Tušek Jelenc, M., Chmelík, M., Bogner, W., Krššák, M., Trattnig, S., and Valkovič, L. (2016). Feasibility and repeatability of localized 31 P-MRS Four-Angle Saturation Transfer (FAST) of the human gastrocnemius muscle using a surface coil at 7 T. NMR Biomed. 29, 57–65. doi: 10.1002/nbm.3445
Valkovič, L., Chmelík, M., and Krššák, M. (2017). In-vivo 31P-MRS of skeletal muscle and liver: a way for non-invasive assessment of their metabolism. Anal. Biochem. 529, 193-215. doi: 10.1016/j.ab.2017.01.018
Valkovič, L., Chmelík, M., Kukurová, I. J., Jakubová, M., Kipfelsberger, M. C., Krumpolec, P., et al. (2014). Depth-Resolved Surface Coil MRS (DRESS)-Localized Dynamic 31 P-MRS of the exercising human gastrocnemius muscle at 7 T. NMR Biomed. 27, 1346–1352. doi: 10.1002/nbm.3196
Valkovič, L., Chmelík, M., Ukropcová, B., Heckmann, T., Bogner, W., Frollo, I., et al. (2016). Skeletal muscle alkaline pi pool is decreased in overweight-to-obese sedentary subjects and relates to mitochondrial capacity and phosphodiester content. Sci. Rep. 6:20087. doi: 10.1038/srep20087
van den Broek, N. M., De Feyter, H. M., de Graaf, L., Nicolay, K., and Prompers, J. J. (2007). Intersubject differences in the effect of acidosis on phosphocreatine recovery kinetics in muscle after exercise are due to differences in proton efflux rates. Am. J. Physiol. Cell Physiol. 293, C228–C237. doi: 10.1152/ajpcell.00023.2007
Vanhamme, L., van den Boogaart, A., and Van Huffel, S. (1997). Improved method for accurate and efficient quantification of MRS data with use of prior knowledge. J. Magn. Reson. 129, 35–43. doi: 10.1006/jmre.1997.1244
Walter, G., Vandenborne, K., Elliott, M., and Leigh, J. S. (1999). In vivo ATP synthesis rates in single human muscles during high intensity exercise. J. Physiol. 519(Pt 3), 901–910.
Walter, G., Vandenborne, K., McCully, K. K., and Leigh, J. S. (1997). Noninvasive measurement of phosphocreatine recovery kinetics in single human muscles. Am. J. Physiol. Cell Physiol. 272, C525–C534.
Keywords: proton and phosphorus magnetic resonance spectroscopy, skeletal muscle, oxygen uptake, muscle training status, energy metabolism
Citation: Klepochová R, Valkovič L, Hochwartner T, Triska C, Bachl N, Tschan H, Trattnig S, Krebs M and Krššák M (2018) Differences in Muscle Metabolism Between Triathletes and Normally Active Volunteers Investigated Using Multinuclear Magnetic Resonance Spectroscopy at 7T. Front. Physiol. 9:300. doi: 10.3389/fphys.2018.00300
Received: 25 October 2017; Accepted: 13 March 2018;
Published: 03 April 2018.
Edited by:
Bruce M. Damon, Vanderbilt University Medical Center, United StatesReviewed by:
Robert W. Wiseman, Michigan State University, United StatesTheodore Francis Towse, Grand Valley State University, United States
Copyright © 2018 Klepochová, Valkovič, Hochwartner, Triska, Bachl, Tschan, Trattnig, Krebs and Krššák. This is an open-access article distributed under the terms of the Creative Commons Attribution License (CC BY). The use, distribution or reproduction in other forums is permitted, provided the original author(s) and the copyright owner are credited and that the original publication in this journal is cited, in accordance with accepted academic practice. No use, distribution or reproduction is permitted which does not comply with these terms.
*Correspondence: Martin Krššák, bWFydGluLmtyc3Nha0BtZWR1bml3aWVuLmFjLmF0
†These authors have contributed equally to this work.