- 1Faculty of Kinesiology, University of Calgary, Calgary, AB, Canada
- 2Faculty of Environmental Design, University of Calgary, Calgary, AB, Canada
- 3Department of Biochemistry & Molecular Biology, Cumming School of Medicine, University of Calgary, Calgary, AB, Canada
Although aging has been associated to slower O2 kinetics, some evidence indicates that fitness status and not aging per se might modulate this response. The main goal of this study was to examine the O2, deoxygenated hemoglobin+myoglobin (deoxy-[Hb+Mb]) kinetics, and the NIRS-derived vascular reperfusion responses in older compared to young men of different training levels (i.e., inactive, recreationally active, and endurance trained). Ten young inactive [YI; 26 ± 5 yrs.; peak O2 (O2peak), 2.96 ± 0.55 L·min−1], 10 young recreationally active (YR; 26 ± 6 yrs.; 3.92 ± 0.33 L·min−1), 10 young endurance trained (YT; 30 ± 4 yrs.; 4.42 ± 0.32 L·min−1), 7 older inactive (OI; 69 ± 4 yrs.; 2.50 ± 0.31 L·min−1), 10 older recreationally active (OR; 69 ± 5 yrs.; 2.71 ± 0.42 L·min−1), and 10 older endurance trained (OT; 66 ± 3 yrs.; 3.20 ± 0.35 L·min−1) men completed transitions of moderate intensity cycling exercise (MODS) to determine O2 and deoxy-[Hb+Mb] kinetics, and the deoxy-[Hb+Mb]/O2 ratio. The time constant of O2 (τO2) was greater in YI (38.8 ± 10.4 s) and OI (44.1 ± 10.8 s) compared with YR (26.8 ± 7.5 s) and OR (26.6 ± 6.5 s), as well as compared to YT (14.8 ± 3.4 s), and OT (17.7 ± 2.7 s) (p < 0.05). τO2 was greater in YR and OR compared with YT and OT (p < 0.05). The deoxy-[Hb+Mb]/O2 ratio was greater in YI (1.23 ± 0.05) and OI (1.29 ± 0.08) compared with YR (1.11 ± 0.03) and OR (1.13 ± 0.06), as well as compared to YT (1.01 ± 0.03), and OT (1.06 ± 0.03) (p < 0.05). Similarly, the deoxy-[Hb+Mb]/ O2 ratio was greater in YR and OR compared with YT and OT (p < 0.05). There was a main effect of training (p = 0.033), whereby inactive (p = 0.018) and recreationally active men (p = 0.031) had significantly poorer vascular reperfusion than endurance trained men regardless of age. This study demonstrated not only that age-related slowing of O2 kinetics can be eliminated in endurance trained individuals, but also that inactive lifestyle negatively impacts the O2 kinetics response of young healthy individuals.
Introduction
Studies assessing the physiological responses to exercise across the aging spectrum have demonstrated age-related declines within the O2 transport system, as determined by reductions in peak oxygen uptake (O2peak) (Astrand, 1976; Fitzgerald et al., 1997). Although endurance trained older individuals demonstrate a greater O2peak compared to their untrained counterparts, an age-related decline in O2peak is still inevitable (Wilson and Tanaka, 2000). Despite the prognostic values of O2peak to predict a minimum level of fitness required to perform activities of daily living (ADL) without incurring fatigue that would negatively affect independent living, there are other measures of cardiovascular function including O2 kinetics and vascular responsiveness that are used to assess the ability of older individuals to perform ADLs. In fact, once a given functional threshold is achieved (i.e., a O2peak of ~30 mL·kg−1·min−1) (Paterson et al., 2007), ADLs are performed at submaximal intensities of exercise, which makes measures of cardiovascular adjustments at these intensities very important.
To further understand age-related limitations within the path of O2 transport during submaximal exercise performed within the moderate intensity domain, researchers have investigated the mechanisms that control the dynamic adjustment of oxygen consumption (O2) during square-wave exercise transitions from a given baseline metabolic rate, to a higher metabolic demand below the gas exchange threshold (GET) (Babcock et al., 1994; DeLorey et al., 2007; duManoir et al., 2010; Murias et al., 2010; Grey et al., 2015). Using this model, an instantaneous increase in metabolic demand [typically power output (PO) during cycling exercise] produces a mono-exponential increase in the adjustment of oxidative phosphorylation [represented by the phase II O2 time constant (τO2)]. The oxygen deficit generated by this mono-exponential increase in O2 represents an increase in metabolic demand that is partly sustained by non-oxidative sources until a steady state in O2 is achieved. Previous research has typically shown a slower rate of adjustment of oxidative phosphorylation (O2 kinetics) in older compared to young individuals (Bell et al., 2001; Grassi, 2001; Hughson et al., 2001; duManoir et al., 2010; Murias et al., 2014; McLay et al., 2017). Despite this observation, a faster O2 kinetics response can be observed in older individuals subsequent to endurance exercise training interventions (Babcock et al., 1994; Bell et al., 2001; Murias et al., 2010, 2016; Keir et al., 2016; McLay et al., 2017). This observation demonstrates that this population is still malleable to produce adaptations within the cardiovascular system and within the skeletal muscle (Belman and Gaesser, 1991) that would result in a faster adjustment of O2 at the onset of exercise. Indeed, a recent study in a population of chronically endurance trained older individuals demonstrated O2 kinetics responses in these individuals that were as fast as those observed in younger counterparts (Grey et al., 2015), prompting the authors to suggest that fitness level and not aging might be the main factor determining the dynamic adjustment of the O2 response during the exercise on-transient (Grey et al., 2015).
Interestingly, this previous study (Grey et al., 2015) not only indicated that endurance trained older individuals had the same O2 kinetics as those observed in young endurance trained participants, but also that recreationally active young and middle-aged individuals showed slower O2 kinetics as compared to their endurance trained counterparts. These data re-enforce the idea that even young individuals might have a relatively slow rate of adjustment of oxidative phosphorylation when they are not in the upper end of the fitness level continuum. Unfortunately, the study of Grey et al. (2015) did not consider evaluating sedentary individuals. If fitness level and not aging per se is a key factor modulating the dynamic adjustment of O2, then not only chronically endurance trained older individuals should demonstrate a O2 kinetics that is as fast as that observed in their younger counterparts, but also young sedentary individuals should demonstrate a O2 kinetics response that is as slow as that observed in their older counterparts.
Therefore, the main goal of this study was to examine the O2 kinetics response in older compared to young individuals of different training levels (i.e., inactive, recreationally active, and endurance trained). It was hypothesized that there would be a continuous increase in the τO2 from endurance trained to recreationally active to sedentary men, in both the young and older groups, so that fitness level would be a key modulator of the O2 kinetics response independently of age.
Materials and Methods
Participants
Fifty-seven men were recruited from community associations across the city of Calgary, online community groups, and through word of mouth. All participants were healthy, non-obese, non-smokers who were not taking any medications that would affect their hemodynamic responses to exercise. Participants were separated into two groups, young (18–45 years) and older (60–75 years). Each group was further separated into three categories: inactive, recreationally active and endurance trained yielding six groups: young inactive (YI; n = 10), young recreationally active (YR; n = 10), young endurance trained (YT; n = 10), older inactive (OI; n = 7), older recreationally active (OR; n = 10), and older endurance trained (OT; n = 10). This study was carried out in accordance with the recommendations of the University of Calgary Conjoint Health Research Ethics Board with written informed consent from all participants. All participants gave written informed consent in accordance with the Declaration of Helsinki. The protocol was approved by the University of Calgary Conjoint Health Research Ethics Board (REB16-0578).
Participant Fitness Level
The inactive men were engaged only in very light activity such as walking. The recreationally-trained men were regularly active (2–4 times per week), engaging in a mix of resistance and endurance exercise, as well as recreational sporting activities (i.e., exercising/playing sports for health). The endurance-trained men were competitive and/or active endurance athletes. All endurance athletes had been engaging in activity ≥4 times per week, with a mix of endurance and high-intensity exercise (i.e., exercising for competition) for at least the last 4 consecutive years.
Experimental Design
Participants were invited to the Laboratory to complete the experimental procedures on 3 separate days. All protocols were conducted in an environmentally-controlled laboratory (i.e., temperature ~21°C, relative humidity ~35%). Participants were instructed to refrain from vigorous physical activity, caffeine and alcohol for at least 12 h prior to each testing session.
On the first visit, participants performed a ramp incremental exercise test to exhaustion (50 W baseline for 4 min followed by a 15 W min−1 ramp for OU, 25 W min−1 ramp for YU, YR, OR, and OT and a 30 W min−1 ramp for YT) on a magnetically braked cycle ergometer (Velotron RacerMate Inc., Seattle, WA, USA) for determination of O2peak, peak power output (POpeak), and the GET. The test was terminated once participants were unable to continue pedaling at a constant cadence despite strong verbal encouragement. Participants only received external motivation near the end of the test to promote maximal effort. The O2peak was defined as the greatest 20 s O2 (O2) computed from a rolling average, and POpeak was defined as the PO achieved at termination of the ramp incremental test. The GET was determined by visual inspection and defined as the O2 at which the rate of CO2 output (CO2) began to increase out of proportion in relation to O2, with a systemic rise in minute ventilation (E) in relation to O2 and in end-tidal PO2, whereas the ventilatory equivalent of CO2 (E/CO2) and end-tidal PCO2 was stable (Beaver et al., 1986).
The second visit was separated by at least 48 h from the first one and participants underwent a vascular occlusion test to examine vascular responsiveness (described in detail below).
On the third day, participants performed a cycling test consisting of step transitions in PO from a 20 W baseline to a moderate-intensity, constant load PO that elicited a O2 corresponding to 80% of the GET. Each participant performed three repetitions of this step transition consisting of 6 min of pedaling at 20 W followed by 6 min of pedaling at a PO corresponding to 80% GET. Repeated transitions were performed to increase confidence in the parameter estimation as indicated by Lamarra et al. (1987) and Rossiter et al. (2000) and as experimentally recommended by Spencer et al. (2011).
Cycling Test Measurements
Breath-by-breath gas exchange and ventilation, were continuously measured using a metabolic cart (Quark CPET, COSMED, Rome, Italy), as previously described in our laboratory (Mattioni Maturana et al., 2017). Calibration occurred before each test as recommended by the manufacturer.
Local muscle deoxygenation (deoxy-[Hb+Mb]) of the quadriceps vastus lateralis muscle was measured using a frequency-domain multi-distance near-infrared spectroscopy (NIRS) system (OxiplexTS, Model 92505; ISS, Champaign, IL, USA) as described elsewhere (Spencer et al., 2012). Briefly the NIRS probe was secured using a tightened black elastic strap (to mitigate movement) and covered with an optically dense, black vinyl sheet to minimize the intrusion of extraneous light. An elastic tensor bandage was then loosely wrapped around the site, to avoid constricting blood flow, but to further minimize movement and light intrusion. The probe stayed attached to the participant for the duration of testing. The NIRS system was composed of a single channel consisting of eight laser diodes operating at two wavelengths (λ = 690 and 828 nm; four at each wavelength) that were pulsed in a rapid succession down a photomultiplier tube. A rigid plastic NIRS probe (connected to laser diodes and photomultiplier tube by optical fibers) consisted of two parallel rows of light-emitter fibers and one detector fiber bundle; the source–detector separations for this probe were 2.0, 2.5, 3.0, and 3.5 cm for both wavelengths. The probe was placed on the belly of the muscle, at the distal end of the vastus lateralis muscle. The NIRS measurements were collected continuously for the entire duration of each test.
The near-infrared spectrometer was calibrated at the beginning of each testing session after a warm-up period of at least 30 min. The calibration was performed with the probe placed on a calibration block (phantom) with absorption (μA) and reduced scattering coefficients (μs′) previously measured; thus, correction factors were determined and were automatically implemented by the manufacturer's software for the calculation of the μA and μs′ for each wavelength during the data collection. Calculation of deoxy-[Hb+Mb] reflected continuous measurements of μs′ made throughout each testing session (i.e., constant scattering value was not assumed). Data were stored online at an output frequency of 2 Hz but were reduced to 1 s bins for all subsequent analyses within the present study.
Vascular Occlusion Test
Participants laid supine on a plinth and following a 10-min rest-period, the NIRS probe was placed on the muscle belly of the tibialis anterior. A pneumatic cuff connected to an automatic rapid inflation system (Hokanson E20 AG101, Bellevue, WA, USA) was used for occlusion of blood flow, and placed below the knee (~5 cm distal to the popliteal fossa). Occlusion pressure was set to 250 mm Hg for the occlusion time. NIRS measurements were collected continuously at an output frequency of 2 Hz for the entire duration of each test (5 min of baseline, 5 min of occlusion, and 8 min following cuff release) (McLay et al., 2016a).
Data Analysis
O2 data were edited on an individual basis by removing aberrant data points that laid 3 SD outside of the local mean. During moderate intensity exercise (MOD) transitions, data for each repetition of a similar protocol were then linearly interpolated to 1 s intervals, time-aligned such that time zero represented the onset of the transition, and ensemble-averaged to yield a single averaged response for each participant for a given exercise protocol. These averaged responses were further time-averaged into 5 s bins. The on-transient responses for O2 were modeled using the following equation:
where Y(t) represents the O2 at any given time (t); Ybsln is the steady state baseline value of Y before an increase in WR; Amp is the amplitude of the increase in Y above Ybsln; and TD is the time delay (such that the model is not constrained to pass through the origin); τ is the time constant of the response (or the time required to attain 63% of the steady-state amplitude). The first 22 s of the O2 data were not included in the fitting of the phase II O2 response to minimize the effect of the phase I response in younger individuals (27 s for older participants), as previously recommended (Murias et al., 2011d). O2 data were modeled from the beginning of phase II to 4-min (240 s), ensuring that steady-state O2 had been achieved by that time. This strategy was selected so that data were fit to at least four time constants (>98% of the total response) during the on-transient of the response, avoiding superfluous sections of the steady-state data and thus maximizing the quality of the fit during the exercise on-transient. The model parameters were estimated by least-squares nonlinear regression (Origin, OriginLab Corp., Northampton, MA, USA) in which the best fit was defined by minimization of the residual sum of squares and minimal variation of residuals around the Y-axis (Y = 0). The 95% confidence interval for the estimated time constant was determined after preliminary fit of the data with Ybsln, Amp, and TD constrained to the best-fit values and the τ allowed to vary.
The NIRS-derived deoxy-[Hb+Mb] data were time aligned and averaged to 5-s bins to yield a single response for each participant at each of the testing points. The deoxy-[Hb+Mb] profile is described to consist of a time delay at the onset of exercise (referred to as the calculated time delay), followed by an increase in the signal with an “exponential-like” time-course (Murias et al., 2011c). The calculated time delay for the deoxy-[Hb+Mb] response (deoxy-[Hb+Mb]CTD) was determined using second-by-second data and corresponded to the time, after the onset of exercise, at which the deoxy-[Hb+Mb] signal began a systematic increase from its nadir value and was determined by calculating the deoxy-[Hb+Mb]CTD from the averaged response of the three trials. The deoxy-[Hb+Mb] data were modeled using Equation (1); the fitting window for the “exponential” response spanned from the end of the deoxy-[Hb+Mb]CTD to 90 s into the transition. As described previously (duManoir et al., 2010), different fitting strategies ranging from 90 to 180 s into a transition resulted in minimal differences in estimates of τ deoxy-[Hb+Mb]. Whereas the τ deoxy-[Hb+Mb] described the time course for the increase in deoxy-[Hb+Mb], the overall time course of the deoxy-[Hb+Mb] from the onset of the step transition was described by the effective deoxy-[Hb+Mb] (τ' deoxy-[Hb+Mb] = deoxy-[Hb+Mb]CTD + τ deoxy-[Hb+Mb]).
Calculations of the deoxy-[Hb+Mb]/O2 ratio were similar to those previously described (Murias et al., 2011e, 2012) Briefly, the second-by-second deoxy-[Hb+Mb] and O2 data were normalized for each participant (0–100% of the transition response). The normalized O2 was left shifted to account for the phase I-phase II transition (Murias et al., 2011d) so that the beginning of phase II O2 coincided with the onset of exercise as detailed elsewhere (Murias et al., 2011c). An overall average deoxy-[Hb+Mb]/O2 ratio for the adjustment period during the exercise on-transient was derived individually as the average of the ratio values from 20 to 120 s. The start point was selected to be 20 s to begin beyond the physiological deoxy-[Hb+Mb]CTD derived from NIRS. An end point of 120 s was selected to ensure that the deoxy-[Hb+Mb]/O2 had reached a value of 1.0, indicating a steady state in the matching of this response.
Vascular reperfusion rate was evaluated as previously established (McLay et al., 2016a,b). Briefly, the NIRS-derived O2 saturation (StO2) reperfusion rate (slope 2) was quantified as the slope of the StO2 signal over a 10-s window period immediately following cuff release (StO2%·s−1). It should be noted that immediately after cuff release there is a linear increase in the StO2, followed by a brief plateau around the peak StO2. This linear response is sustained for >10 s which allows for an accurate slope to be easily calculated when including the initial reperfusion phase immediately following cuff release.
Statistical Analysis
Statistical analysis was performed using SPSS 24.0 software (SPSS Inc., Chicago, IL) and Microsoft Excel. All results are presented as mean ± standard deviation (SD). The parameter estimates for O2 and deoxy-[Hb+Mb], as well as the deoxy-[Hb+Mb]/O2 ratio and the Slope 2 StO2 responses were analyzed with a two-way analysis of variance (ANOVA) using aging and fitness level as independent factors. Significant main effects and interactions were analyzed using Fisher's Least Significant Difference (LSD) post-hoc test. Pearson product-moment correlation was used to determine the association between variables. Statistical significance was accepted at p < 0.05.
Results
Participant characteristics, as well as O2peak and POpeak output determined from the ramp-incremental test are listed in Table 1. As expected by design, age was significantly greater in the older participants compared to younger participants in endurance trained, recreationally active and inactive groups (p < 0.05). The absolute O2peak and POpeak output were higher in younger groups compared with that of older groups across each fitness level (p < 0.05). Additionally, absolute O2peak and POpeak were lower in inactive groups than endurance trained groups in both younger and older participants (p < 0.05).
O2 and Deoxy-[Hb+Mb] Kinetics
Group means and SD for τ O2 data are displayed in Figure 1A and detailed in Table 2. Figures 2 A,B show a representative fit for the O2 kinetics in a young and older participant, respectively. The τO2 was greater in the: (1) inactive groups when compared to the recreationally active or endurance trained groups (p < 0.05), and (2) recreationally active groups compared to the endurance trained groups (p < 0.05). There was no main effect for aging or aging by fitness level interaction for τO2 (p > 0.05). Due to the differences in absolute WR during MOD, O2 amplitude was significantly lower in older recreationally trained and endurance trained individuals than their young training-matched counterparts (p < 0.05); however, there was no difference between young inactive and older inactive groups (p > 0.05). While, O2 amplitude and MOD WR were greater in older endurance trained individuals compared to both inactive and recreationally active (p < 0.05), there was a progressive increase in the O2 amplitude and MOD WR corresponding to any increase in fitness level in the younger groups (p < 0.05) (Table 2). The O2 baseline was greater in younger compared to older individuals (p < 0.05), however, the functional O2 gain (ΔO2/ΔWR) was not different in any of the groups (p > 0.05) (Table 2). τ deoxy-[Hb+Mb], CTD deoxy-[Hb+Mb] and τ' deoxy-[Hb+Mb] were not statistically different across all aging and training groups (p > 0.05) (Table 2). Figures 2C,D shows a representative fit for the deoxy-[Hb+Mb] kinetics in a young and in an older participant, respectively. There was a significant negative correlation between O2peak and τO2 in both older (r = 0.60; p < 0.05) and young (r = 0.63; p < 0.05) participants.
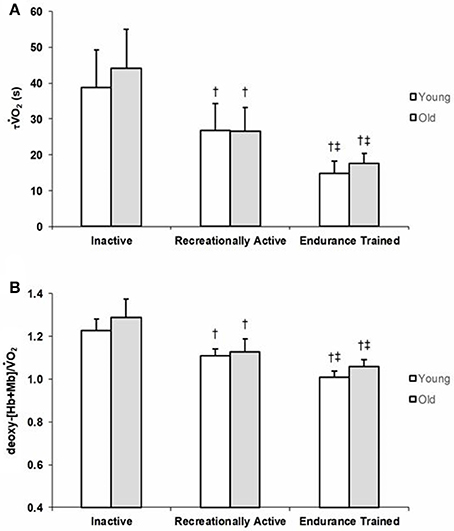
Figure 1. Group mean τO2 (A) and deoxy-[Hb+Mb]/O2 ratio (B) values for young and older inactive (I), recreationally active (R), and endurance trained (T) groups. Data are presented as mean ± SD. Significantly different from inactive group (p < 0.05). ‡Significantly different from recreationally active group (p < 0.05).
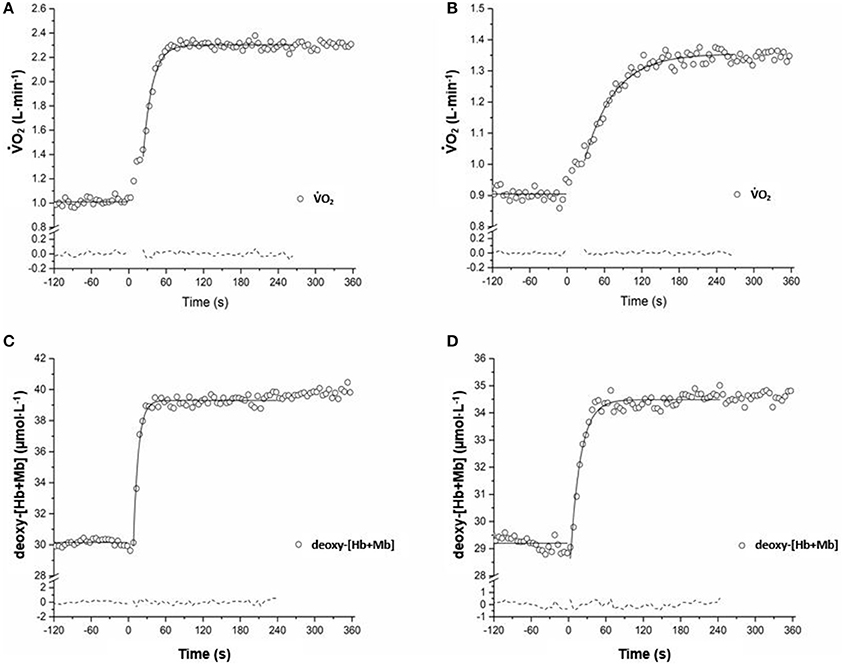
Figure 2. Adaptation of O2 (A,B) and vastus lateralis deoxygenation (C,D) during the transition to exercise at a relative WR corresponding to ~80% of the gas exchange threshold in a representative YT and OI adult. Model fits of the data are displayed with a solid line, and residuals of the fit are displayed with a dashed line.
Deoxy-[Hb+Mb]/O2 Ratio
Average group data for the deoxy-[Hb+Mb]/O2 ratio derived from the normalized responses of deoxy-[Hb+Mb] and O2 adjustments to a moderate-intensity WR are portrayed in Figure 1B. The deoxy-[Hb+Mb]/O2 ratio was significantly greater in both OI and YI compared to OR and YR as well as OT and YT (p < 0.05) (Table 2). Additionally, OR and YR displayed a significantly greater ratio than OT and YT (p < 0.05) (Table 2). There was a main effect for aging (p < 0.05) and no aging by training interaction for the deoxy-[Hb+Mb]/O2 ratio (p > 0.05).
NIRS-Derived Slope 2 of StO2
Figure 3 displays the Slope 2 StO2 values for young and older inactive, recreationally active, and endurance trained individuals. There was a main effect of training (p = 0.033), whereby inactive (p = 0.018) and recreationally active men (p = 0.031) had signifcantly lower values of slope 2 than endurance trained men regardless of age.
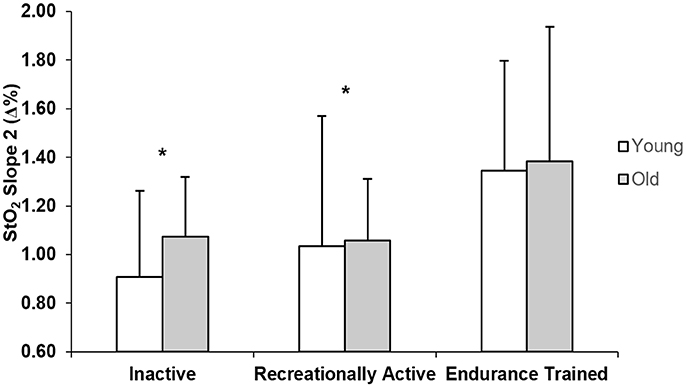
Figure 3. Group mean NIRS-derived StO2 values of slope 2 for young and older inactive (I), recreationally active (R), and endurance trained (T) groups. *Significantly different from endurance trained group (p < 0.05).
Discussion
The present study examined the effects of aging and fitness level on the O2 kinetics profiles of young and older inactive, recreationally active, and endurance trained men. The main findings are as follows. Firstly, across all training groups, O2 kinetics were not slower in older adults compared to their training-matched younger counterparts. This study is the first to show that fitness level and not aging per se determines the O2 kinetics response across a wide range of training levels. Secondly, the present data indicate that, along with other intracellular mechanisms of control that were not measured in the present study, improved matching of O2 delivery to O2 utilization seems to be an important factor in determining the speed of the O2 kinetics response, across all aging and training groups.
Studies assessing the O2 kinetics response in young and older adults have regularly shown slower τO2 values in the aging population (Chilibeck et al., 1996; DeLorey et al., 2004; Murias et al., 2010; Gravelle et al., 2012). Although debate exists in relation to the mechanisms that control the rate of adjustment of oxidative phosphorylation (Grassi, 2001; Hughson et al., 2001; Poole and Jones, 2012; Murias et al., 2014; Murias and Paterson, 2015), there is a stronger consensus supporting the idea that, in older populations, the slower dynamic adjustment of O2 is related to limitations within the O2 transport system and, more specifically, in the O2 provision to the active muscles which affects the matching of O2 delivery to O2 utilization (Murias et al., 2010; Poole and Jones, 2012). However, a slower adjustment of oxidative phosphorylation in older individuals is not always the case. In a review by Murias and Paterson (2015), the authors presented the τO2 values of older (n = 41) and younger (n = 49) individuals demonstrating a large overlap of τO2 values between age groups. These data denote that while a random sample of older individuals is likely to display a slower O2 kinetics, they can also have a τO2 just as fast as a young individual. In fact, some short-term (≤12 weeks) training studies have demonstrated an acceleration of the O2 kinetics response from ~40–45 to ~30–35 s in older individuals (Fukuoka et al., 2002; Murias et al., 2010), and from ~30–35 to ~20–25 s in young individuals (Berger et al., 2006; Murias et al., 2010). Furthermore, Grey et al. (2015) showed that older, endurance-trained individuals had a O2 kinetics response as rapid as their younger training-matched counterparts (~18 s) which aligns with the results of the current investigation (~16 s τO2 values). Importantly, this study fills a gap in the literature, as it includes young and older participants from across a wide training spectrum. One earlier study assessed O2 kinetics across the aging spectrum (i.e., young, middle-aged, and older men) in chronically trained and healthy active community dwelling individuals (Grey et al., 2015), however it was limited by the lack of an inactive group. This is an important component since, if fitness level and not aging per se is the main factor modulating the speed of the O2 kinetics response, then it would be expected that young inactive men would have a τO2 that is not different from that observed in their inactive older counterparts. This study is the first to demonstrate that regardless of age, τO2 is affected by fitness level in a group of young and older individuals that spanned over a wide range of training levels (Figure 1A, Table 2). Most notably, group mean τO2 of young inactive adults was not different from that of older inactive males, emphasizing that it is not only the accumulation of many years of an inactive lifestyle that produce detrimental cardiovascular adaptations affecting the O2 transport system, but that these negative effects can be observed early in the life span when inactive behaviors are adopted. Further support to the connection between fitness status and the dynamic adjustment of O2 comes from the large correlation between O2max and τO2.
The mechanisms responsible for the rate of adjustment of O2 have been a matter of debate for years. Although intracellular mechanisms of control are undeniably an important component controlling the dynamic adjustment of oxidative phosphorylation during exercise transitions to higher metabolic demands (Grassi, 2000; Grassi et al., 2002; Rossiter, 2010; Poole and Jones, 2012), several recent studies (Murias et al., 2010; Grey et al., 2015; McLay et al., 2017) have indicated that adequate provision of O2 to the active tissues is also a key rate limiting mechanism. In relation to the latter, the present study reports that the matching of O2 delivery to O2 utilization in the active muscles, as indicated by the deoxy-[Hb+Mb]/O2 ratio, is improved in recreationally active and endurance trained groups compared to inactive groups, and further improved in endurance trained groups compared to recreationally active groups (Figure 1, Table 2). Further support is thus provided for these studies that have proposed increased O2 distribution to the sites of increased metabolic demand playing an important role in the regulation of O2 during exercise (Murias et al., 2010, 2011a, 2014; McLay et al., 2016a). For example, improved matching of O2 delivery to O2 utilization, which was associated to a faster O2 kinetics response, has been demonstrated following brief (≤3 sessions) (Murias et al., 2016; McLay et al., 2017), short-term (~12 weeks) (Murias et al., 2010, 2011a) and long-term (Grey et al., 2015) training in both young and older participants. Although not investigated in this study, improved matching of O2 delivery to O2 utilization within the active tissues can be explained by different structural enhancements related to exercise training. For example, Behnke et al. (2012), demonstrated an increase in the cross-sectional area (CSA) of feed arteries to the muscle after training in old rats. This increased CSA was responsible for increased blood flow and improved matching of O2 delivery to O2 consumption. Similarly, Spier et al. (2007) indicated that exercise training restores flow-mediated vasodilatory responses in gastrocnemius muscle arterioles from old rats. Similarly, improved vascular responsiveness through enhanced endothelium dependent vasodilation has been indicated to occur in response to exercise training (DeSouza et al., 2000). Additionally, although the role of the capillaries for blood-myocyte O2 exchange has been questioned (Poole et al., 2013), improvements in the potential for O2 flux from capillaries to muscles fibers can be observed in chronically trained masters athletes who demonstrated similar capillary density to training-matched young athletes (Coggan et al., 1990), or by increases in measures of capillarization that take place in response to endurance training interventions (Coggan et al., 1992; Murias et al., 2011b).
Further support for this theory is provided through the results of the NIRS-derived measure of microvascular reperfusion presented in this study. There was a significant main effect of training for the Slope 2 StO2, that demonstrated not only improved vascular responsiveness in the endurance trained compared to the recreationally active and inactive individuals, but also a similar effect of training independently of the age group (Figure 3). Murias et al. (2010, 2011a) previously proposed that improved vascular responsiveness resulted in more adequate matching of O2 delivery to O2 utilization within the active tissues, which in turn played a role for speeding of the O2 kinetics response. That suggestion was further supported by a study showing that speeding and slowing of O2 kinetics were accompanied by decreases and increases in the deoxy-[Hb+Mb]/O2 ratio, as well as increased and decreased measures of flow mediated dilation (FMD), respectively. A novel aspect related to this study is the examination the differences in the Slope 2 StO2, which estimates vascular responsiveness within the microcirculation (near the tissues in which changes in O2 occur), rather than in a conduit artery as FMD (McLay et al., 2016a). The steeper slopes observed in the endurance trained group might be due to long-term endurance training-stimulated vascular remodeling that the muscles undergo to provide a system that is capable of properly matching the O2 delivery to the metabolic requirements of the fibers, at least during exercises performed in the moderate intensity domain. A limitation of the Slope 2 StO2 analysis is that, even though it has been indicated to show less variability than measures of FMD, ~25–30% of the response might be affected by variability (McLay et al., 2016b), making the dispersion of this response larger, and differences less noticeable in the groups with lower fitness level.
Although our data supports the idea that an O2 delivery limitation might explain part of the slower O2 kinetics observed in the individual with progressively lower fitness level, an alternative hypothesis suggests that the main mechanisms controlling the dynamic adjustment of oxidative phosphorylation reside intracellularly, and thus changes related to training are explained by intracellular adaptations that improves provision of substrates, other than O2, to the mitochondria (Grassi et al., 1998a,b; Poole and Jones, 2012). The use of 31-phosphorous magnetic resonance spectroscopy (31P-MRS) to infer the adjustment of muscle O2 by measuring PCr breakdown (McCreary et al., 1996) has been used by many research groups to support a theory of intracellular control (Barstow et al., 1994a,b; McCreary et al., 1996; Rossiter et al., 1999, 2003). Additionally, pyruvate dehydrogenase (PDH) has been investigated as a potential site of regulation for oxidative phosphorylation (Howlett et al., 1999; Bangsbo et al., 2002; Grassi et al., 2002; Rossiter et al., 2003; Jones et al., 2004). However, to date, experiments in humans (Bangsbo et al., 2002; Rossiter et al., 2003; Jones et al., 2004) have failed to demonstrate faster O2 kinetics following prior PDH activation via dichloroacetate supplementation. In relation to exercise training, one study has proposed that the speeding of the O2 kinetics response following a 20-week endurance training protocol was mainly due to an increase in each-step parallel activation rather than mitochondrial biogenesis (Zoladz et al., 2014). An important observation to make about that study is that changes in the O2 kinetics response were measured 20 weeks apart, and several investigations have shown that changes in the adjustment of the O2 kinetics occur within a few training sessions (Murias et al., 2016; McLay et al., 2017). Thus, there is a disconnection between the time of measurement and the time at which the changes occurred. Nevertheless, it has to be acknowledged that a large part of the literature supports the idea that intracellular mechanisms of control are the key regulators of the O2 kinetics response and that, consequently to this idea, exercise training not only results in improvements in vascular responsiveness and blood flow distribution, but also upregulate intracellular pathways linked to oxidative metabolism (Henriksson and Reitman, 1977; Örlander and Aniansson, 1980; Coggan et al., 1990, 1992; Belman and Gaesser, 1991; Murias et al., 2011b). Thus, the present study did not aim to isolate the mechanisms that control the dynamic adjustment of O2. Importantly, what this study highlights is that, independently of what the mechanisms of control are, the O2 kinetics response is linked to fitness status and that this link is not affected by the age of the participants (i.e., not only that older trained individuals might display O2 kinetics as fast as those observed in their younger counterparts, but also that inactive but otherwise healthy young individuals might have a O2 kinetics response that is as slow as that observed in their older counterparts).
Two different factors could be considered as limitations in this study: (1) although this study indicates that there is a connection between fitness status and the O2 kinetics response, it could be argued that the strong negative correlation between O2peak and τO2 is not that close to a perfect correlation. However, it should be noted that it is not the O2peak per se, but what having a high O2peak represents from a cardiovascular and metabolic adaptions perspective what modulates the O2p kinetics response. For example, a chronically trained older person would have a lower maximal heart rate, which will reduce maximal cardiac output, and thus O2peak. However, this same older person might still benefit from most of the other cardiovascular and metabolic adaptations as expected in a young trained individual. All these beneficial training adaptations will result in a very fast O2 kinetics response during submaximal tasks as measured in this study. Thus, the aspect that will limit O2peak in this example, should not impair the adjustment of the O2 kinetics response; (2) the adjustment of the deoxy-[Hb+Mb] was only tested in the vastus lateralis muscle. Different lines of research have indicated heterogeneous responses between and within the muscle in the amplitude and dynamic profiles of tissue oxygenation signals (Koga et al., 2007; Heinonen et al., 2015; Iannetta et al., 2017). Therefore, data from the vastus lateralis muscle should not be interpreted as a representation of the activity of all the muscles involved in cycling.
In conclusion, this study demonstrated not only that age-related slowing of O2 kinetics can be eliminated when endurance training exercise has been performed, but also that an inactive lifestyle has a negative impact on the O2 response of young and otherwise healthy individuals. Unique to this study was the lack of statistical differences between the τO2 values and deoxy-[Hb+Mb]/O2 ratios of every training group, regardless of the age of the participants. These data indicate that fitness level and not aging per se determine the O2 kinetics response and further highlight the importance of endurance training to improve or sustain cardiovascular function.
Author Contributions
MG: Participated in the design, recruited participants, collected and analyzed data, and prepared the manuscript for submission; KM: Assisted with data collection and design; RR and PD-B: Contributed to the design of the project and provided feedback on the manuscript; JM: Contributed to designing the project, analyzing the data, and writing the manuscript.
Funding
This work was supported by the Natural Sciences and Engineering Research Council of Canada (RGPIN/03698-2016).
Conflict of Interest Statement
The authors declare that the research was conducted in the absence of any commercial or financial relationships that could be construed as a potential conflict of interest.
References
Astrand, P. O. (1976). Quantification of exercise capability and evaluation of physical capacity in man. Prog. Cardiovasc. Dis. 19, 51–67. doi: 10.1016/0033-0620(76)90008-6
Babcock, M. A., Paterson, D. H., and Cunningham, D. A. (1994). Effects of aerobic endurance training on gas exchange kinetics of older men. Med. Sci. Sports Exerc. 26, 447–452. doi: 10.1249/00005768-199404000-00008
Bangsbo, J., Gibala, M. J., Krustrup, P., González-Alonso, J., and Saltin, B. (2002). Enhanced pyruvate dehydrogenase activity does not affect muscle O2 uptake at onset of intense exercise in humans. Am. J. Physiol. Regul. Integr. Comp. Physiol. 282, 273–280. doi: 10.1152/ajpregu.2002.282.1.R273
Barstow, T. J., Buchthal, S. D., Zanconato, S., and Cooper, D. M. (1994a). Changes in potential controllers of human skeletal muscle respiration during incremental calf exercise. J. Appl. Physiol. 77, 2169–2176. doi: 10.1152/jappl.1994.77.5.2169
Barstow, T. J., Buchthal, S., Zanconato, S., and Cooper, D. M. (1994b). Muscle energetics and pulmonary during moderate exercise oxygen uptake kinetics. J. Appl. Physiol. 77, 1742–1749. doi: 10.1152/jappl.1994.77.4.1742
Beaver, W. L., Wasserman, K., and Whipp, B. J. (1986). A new method for detecting anaerobic threshold by gas exchange. J. Appl. Physiol. 60, 2020–2027. doi: 10.1152/jappl.1986.60.6.2020
Behnke, B. J., Ramsey, M. W., Stabley, J. N., Dominguez, J. M., Davis, R. T., McCullough, D. J., et al. (2012). Effects of aging and exercise training on skeletal muscle blood flow and resistance artery morphology. J. Appl. Physiol. 113, 1699–1708. doi: 10.1152/japplphysiol.01025.2012
Bell, C., Paterson, D. H., Kowalchuk, J. M., Moy, A. P., Thorp, D. B., Noble, E. G., et al. (2001). Determinants of oxygen uptake kinetics in older humans following single limb endurance exercise training. Exp. Physiol. 86, 659–669. doi: 10.1113/eph8602209
Belman, M. J., and Gaesser, G. A. (1991). Exercise training below and above the lactate threshold in the elderly. Med. Sci. Sports Exerc. 23, 562–568. doi: 10.1249/00005768-199105000-00008
Berger, N. J., Tolfrey, K., Williams, A. G., and Jones, A. M. (2006). Influence of continuous and interval training on oxygen uptake on-kinetics. Med. Sci. Sports Exerc. 38, 504–512. doi: 10.1249/01.mss.0000191418.37709.81
Chilibeck, P. D., Paterson, D. H., Petrella, R. J., and Cunningham, D. A. (1996). The influence of age and cardiorespiratory fitness on kinetics of oxygen uptake. Can. J. Appl. Physiol. 21, 185–196. doi: 10.1139/h96-015
Coggan, A. R., Spina, R. J., King, D. S., Rogers, M. A., Brown, M., Nemeth, P. M., et al. (1992). Skeletal muscle adaptations to endurance training in 60- to 70-yr-old men and women. J. Appl. Physiol. 72, 1780–1786. doi: 10.1152/jappl.1992.72.5.1780
Coggan, A. R., Spina, R. J., Rogers, M. A., King, D. S., Brown, M., Nemeth, P. M., et al. (1990). Histochemical and enzymatic characteristics of skeletal muscle in master athletes. J. Appl. Physiol. 68, 1896–1901. doi: 10.1152/jappl.1990.68.5.1896
DeLorey, D. S., Kowalchuk, J. M., and Paterson, D. H. (2004). Effect of age on O2 uptake kinetics and the adaptation of muscle deoxygenation at the onset of moderate-intensity cycling exercise. J. Appl. Physiol. 97, 165–172. doi: 10.1152/japplphysiol.01179.2003
DeLorey, D. S., Paterson, D. H., and Kowalchuk, J. M. (2007). Effects of ageing on muscle O2 utilization and muscle oxygenation during the transition to moderate-intensity exercise. Appl. Physiol. Nutr. Metab. 32, 1251–1262. doi: 10.1139/H07-121
DeSouza, C. A., Shapiro, L. F., Clevenger, C. M., Dinenno, F. A., Monahan, K. D., Tanaka, H., et al. (2000). Regular aerobic exercise prevents and restores age-related declines in endothelium-dependent vasodilation in healthy men. Circulation 102, 1351–1357. doi: 10.1161/01.CIR.102.12.1351
duManoir, G. R., Delorey, D. S., Kowalchuk, J. M., and Paterson, D. H. (2010). Differences in exercise limb blood flow and muscle deoxygenation with age: contributions to O2uptake kinetics. Eur. J. Appl. Physiol. 110, 739–751. doi: 10.1007/s00421-010-1546-z
Fitzgerald, M. D., Tanaka, H., Tran, Z. V., and Seals, D. R. (1997). Age-related declines in maximal aerobic capacity in regularly exercising vs. sedentary women: a meta-analysis. J. Appl. Physiol. 83, 160–165. doi: 10.1152/jappl.1997.83.1.160
Fukuoka, Y., Grassi, B., Conti, M., Guiducci, D., Sutti, M., Marconi, C., et al. (2002). Early effects of exercise training on O2 on- and off-kinetics in 50-year-old subjects. Pflügers Arch. Eur. J. Physiol. 443, 690–697. doi: 10.1007/s00424-001-0748-y
Grassi, B. (2000). Skeletal muscle O2 on-kinetics: set by O2 delivery or by O2 utilization? New insights into an old issue. Med. Sci. Sports Exerc. 32, 108–116. doi: 10.1097/00005768-200001000-00017
Grassi, B. (2001). Regulation of oxygen consumption at exercise onset: is it really controversial? Exerc. Sport Sci. Rev. 29, 134–138. doi: 10.1097/00003677-200107000-00009
Grassi, B., Gladden, L. B., Samaja, M., Stary, C. M., and Hogan, M. C. (1998a). Faster adjustment of O2 delivery does not affect O2 on-kinetics in isolated in situ canine muscle. J. Appl. Physiol. 85, 1394–1403. doi: 10.1152/jappl.1998.85.4.1394
Grassi, B., Gladden, L. B., Stary, C. M., Wagner, P. D., and Hogan, M. C. (1998b). Peripheral O2 diffusion does not affect O2 on-kinetics in isolated in situ canine muscle. J. Appl. Physiol. 85, 1404–1412. doi: 10.1152/jappl.1998.85.4.1404
Grassi, B., Hogan, M. C., Greenhaff, P. L., Hamann, J. J., Kelley, K. M., Aschenbach, W. G., et al. (2002). Oxygen uptake on-kinetics in dog gastrocnemius in situ following activation of pyruvate dehydrogenase by dichloroacetate. J. Physiol. 538, 195–207. doi: 10.1113/jphysiol.2001.012984
Gravelle, B. M. R., Murias, J. M., Spencer, M. D., Paterson, D. H., and Kowalchuk, J. M. (2012). Adjustments of pulmonary O2 uptake and muscle deoxygenation during ramp incremental exercise and constant-load moderate-intensity exercise in young and older adults. J. Appl. Physiol. 113, 1466–1475. doi: 10.1152/japplphysiol.00884.2011
Grey, T. M., Spencer, M. D., Belfry, G. R., Kowalchuk, J. M., Paterson, D. H., and Murias, J. M. (2015). Effects of age and long-term endurance training on O2 kinetics. Med. Sci. Sports Exerc. 47, 289–298. doi: 10.1249/MSS.0000000000000398
Heinonen, I., Koga, S., Kalliokoski, K. K., Musch, T. I., and Poole, D. C. (2015). Heterogeneity of muscle blood flow and metabolism: influence of exercise, aging, and disease states. Exerc. Sport Sci. Rev. 43, 117–124. doi: 10.1249/JES.0000000000000044
Henriksson, J., and Reitman, J. S. (1977). Time Course of changes in human skeletal muscle succinate dehydrogenase and cytochrome oxidase activities and maximal oxygen uptake with physical activity and inactivity. Acta Physiol. Scand. 99, 91–97. doi: 10.1111/j.1748-1716.1977.tb10356.x
Howlett, R. A., Heigenhauser, G. J., Hultman, E., Hollidge-Horvat, M. G., and Spriet, L. L. (1999). Effects of dichloroacetate infusion on human skeletal muscle metabolism at the onset of exercise. Am. J. Physiol. 277, 18–25. doi: 10.1152/ajpendo.1999.277.1.E18
Hughson, R. L., Tschakovsky, M. E., and Houston, M. E. (2001). Regulation of oxygen consumption at the onset of exercise. Exerc. Sport Sci. Rev. 29, 129–133. doi: 10.1097/00003677-200107000-00008
Iannetta, D., Qahtani, A., Millet, G. Y., and Murias, J. M. (2017). Quadriceps muscles O2 extraction and EMG breakpoints during a ramp incremental test. Front. Physiol. 8:686. doi: 10.3389/fphys.2017.00686
Jones, A. M., Koppo, K., Wilkerson, D. P., Wilmshurst, S., and Campbell, I. T. (2004). Dichloroacetate does not speed phase-II pulmonary O2 kinetics following the onset of heavy intensity cycle exercise. Pflugers Arch. Eur. J. Physiol. 447, 867–874. doi: 10.1007/s00424-003-1209-6
Keir, D. A., Robertson, T. C., Benson, A. P., Rossiter, H. B., and Kowalchuk, J. M. (2016). The influence of metabolic and circulatory heterogeneity on the expression of pulmonary oxygen uptake kinetics in humans. Exp. Physiol. 101, 176–192. doi: 10.1113/EP085338
Koga, S., Poole, D. C., Ferreira, L. F., Whipp, B. J., Kondo, N., Saitoh, T., et al. (2007). Spatial heterogeneity of quadriceps muscle deoxygenation kinetics during cycle exercise. J. Appl. Physiol. 103, 2049–2056. doi: 10.1152/japplphysiol.00627.2007
Lamarra, N., Whipp, B. J., Ward, S. A., and Wasserman, K. (1987). Effect of interbreath fluctuations on characterizing exercise gas exchange kinetics. J. Appl. Physiol. 62, 2003–2012. doi: 10.1152/jappl.1987.62.5.2003
Mattioni Maturana, F., Keir, D. A., McLay, K. M., and Murias, J. M. (2017). Critical power testing or self-selected cycling: which one is the best predictor of maximal metabolic steady-state? J. Sci. Med. Sport 20, 795–799. doi: 10.1016/j.jsams.2016.11.023
McCreary, C. R., Chilibeck, P. D., Marsh, G. D., Paterson, D. H., Cunningham, D. A., and Thompson, R. T. (1996). Kinetics of pulmonary oxygen uptake and muscle phosphates during moderate-intensity calf exercise. J. Appl. Physiol. 81, 1331–1338. doi: 10.1152/jappl.1996.81.3.1331
McLay, K. M., Fontana, F. Y., Nederveen, J. P., Guida, F. F., Paterson, D. H., Pogliaghi, S., et al. (2016a). Vascular responsiveness determined by near-infrared spectroscopy measures of oxygen saturation. Exp. Physiol. 101, 34–40. doi: 10.1113/EP085406
McLay, K. M., Murias, J. M., and Paterson, D. H. (2017). Similar pattern of change in O2 kinetics, vascular function and tissue oxygen provision following an endurance training stimulus, in older and young adults. Am. J. Physiol. Regul. Integr. Comp. Physiol. 312, 1101–1114. doi: 10.1152/ajpregu.00399.2016
McLay, K. M., Nederveen, J. P., Pogliaghi, S., Paterson, D. H., and Murias, J. M. (2016b). Repeatability of vascular responsiveness measures derived from near-infrared spectroscopy. Physiol. Rep. 4:e12772. doi: 10.14814/phy2.12772
Murias, J. M., and Paterson, D. H. (2015). Slower O2 kinetics in older individuals: is it inevitable? Med. Sci. Sports Exerc. 47, 2308–2318. doi: 10.1249/MSS.0000000000000686
Murias, J. M., Edwards, J. A., and Paterson, D. H. (2016). Effects of short-term training and detraining on O2 kinetics: Faster O2 kinetics response after one training session. Scand. J. Med. Sci. Sport. 26, 620–629. doi: 10.1111/sms.12487
Murias, J. M., Kowalchuk, J. M., and Paterson, D. H. (2010). Speeding of O2 kinetics with endurance training in old and young men is associated with improved matching of local O2 delivery to muscle O2 utilization. J. Appl. Physiol. 108, 913–922. doi: 10.1152/japplphysiol.01355.2009
Murias, J. M., Kowalchuk, J. M., and Paterson, D. H. (2011a). Speeding of O2 kinetics in response to endurance-training in older and young women. Eur. J. Appl. Physiol. 111, 235–243. doi: 10.1007/s00421-010-1649-6
Murias, J. M., Kowalchuk, J. M., Ritchie, D., Hepple, R. T., Doherty, T. J., and Paterson, D. H. (2011b). Adaptations in capillarization and citrate synthase activity in response to endurance training in older and young men. J. Gerontol. Ser. A Biol. Sci. Med. Sci. 66A, 957–964. doi: 10.1093/gerona/glr096
Murias, J. M., Spencer, M. D., and Paterson, D. H. (2014). The critical role of O2 provision in the dynamic adjustment of oxidative phosphorylation. Exerc. Sport Sci. Rev. 42, 4–11. doi: 10.1249/JES.0000000000000005
Murias, J. M., Spencer, M. D., DeLorey, D. S., Gurd, B. J., Kowalchuk, J. M., and Paterson, D. H. (2011c). Speeding of O2 kinetics during moderate-intensity exercise subsequent to heavy-intensity exercise is associated with improved local O2 distribution. J. Appl. Physiol. 111, 1410–1415. doi: 10.1152/japplphysiol.00607.2011
Murias, J. M., Spencer, M. D., Kowalchuk, J. M., and Paterson, D. H. (2011d). Influence of phase I duration on phase II O2 kinetics parameter estimates in older and young adults. Am. J. Physiol. Regul. Integr. Comp. Physiol. 301, 218–224. doi: 10.1152/ajpregu.00060.2011
Murias, J. M., Spencer, M. D., Kowalchuk, J. M., and Paterson, D. H. (2011e). Muscle deoxygenation to O2 relationship differs in young subjects with varying tO2. Eur. J. Appl. Physiol. 111, 3107–3118. doi: 10.1007/s00421-011-1937-9
Murias, J. M., Spencer, M. D., Pogliaghi, S., and Paterson, D. H. (2012). Noninvasive estimation of microvascular O2 provision during exercise on-transients in healthy young males. Am. J. Physiol. Regul. Integr. Comp. Physiol. 303, 815–823. doi: 10.1152/ajpregu.00306.2012
Örlander, J., and Aniansson, A. (1980). Effects of physical training on skeletal muscle metabolism and ultrastructure in 70 to 75-year-old men. Acta Physiol. Scand. 109, 149–154. doi: 10.1111/j.1748-1716.1980.tb06580.x
Paterson, D. H., Jones, G. R., and Rice, C. L. (2007). Ageing and physical activity: evidence to develop exercise recommendations for older adults. Can. J. Public Health 98(Suppl. 2), S69–S108. doi: 10.1139/H07-111
Poole, D. C., and Jones, A. M. (2012). Oxygen uptake kinetics. Compr. Physiol. 2, 933–996. doi: 10.1002/cphy.c100072
Poole, D. C., Copp, S. W., Ferguson, S. K., and Musch, T. I. (2013). Skeletal muscle capillary function: contemporary observations and novel hypotheses. Exp. Physiol. 98, 1645–1658. doi: 10.1113/expphysiol.2013.073874
Rossiter, H. B. (2010). Exercise: kinetic considerations for gas exchange. Compr. Physiol. 203–244. doi: 10.1002/cphy.c090010
Rossiter, H. B., Howe, F. A., Ward, S. A., Kowalchuk, J. M., Griffiths, J. R., and Whipp, B. J. (2000). Intersample fluctuations in phosphocreatine concentration determined by 31P-magnetic resonance spectroscopy and parameter estimation of metabolic responses to exercise in humans. J. Physiol. 528(Pt 2), 359–369. doi: 10.1111/j.1469-7793.2000.t01-1-00359.x
Rossiter, H. B., Ward, S. A., Doyle, V. L., Howe, F. A., Griffiths, J. R., and Whipp, B. J. (1999). Inferences from pulmonary O2 uptake with respect to intramuscular [phosphocreatine] kinetics during moderate exercise in humans. J. Physiol. 518, 921–932. doi: 10.1111/j.1469-7793.1999.0921p.x
Rossiter, H. B., Ward, S. A., Howe, F. A., Wood, D. M., Kowalchuk, J. M., Griffiths, J. R., et al. (2003). Effects of dichloroacetate on O2 and intramuscular 31P metabolite kinetics during high-intensity exercise in humans. J. Appl. Physiol. 95, 1105–1115. doi: 10.1152/japplphysiol.00964.2002
Spencer, M. D., Murias, J. M., and Paterson, D. H. (2012). Characterizing the profile of muscle deoxygenation during ramp incremental exercise in young men. Eur. J. Appl. Physiol. 112, 3349–3360. doi: 10.1007/s00421-012-2323-y
Spencer, M. D., Murias, J. M., Lamb, H. P., Kowalchuk, J. M., and Paterson, D. H. (2011). Are the parameters of O2, heart rate and muscle deoxygenation kinetics affected by serial moderate-intensity exercise transitions in a single day? Eur. J. Appl. Physiol. 111, 591–600. doi: 10.1007/s00421-010-1653-x
Spier, S. A., Delp, M. D., Stallone, J. N., Dominguez, J. M., and Muller-Delp, J. M. (2007). Exercise training enhances flow-induced vasodilation in skeletal muscle resistance arteries of aged rats: role of PGI2 and nitric oxide. Am. J. Physiol. Circ. Physiol. 292, H3119–H3127. doi: 10.1152/ajpheart.00588.2006
Wilson, T. M., and Tanaka, H. (2000). Meta-analysis of the age-associated decline in maximal aerobic capacity in men: relation to training status. Am. J. Physiol. Hear. Circ. Physiol. 278, 829–834. doi: 10.1152/ajpheart.2000.278.3.H829
Zoladz, J., Grassi, B., Majerczak, J., Szkutnik, Z., Korostynski, M., Grandys, M., et al. (2014). Mechanisms responsible for the acceleration of pulmonary O2 on-kinetics in humans after prolonged endurance training. Am. J. Physiol. Regul. Integr. Comp. Physiol. 307, R1101–R1114. doi: 10.1152/ajpregu.00046.2014
Keywords: aging, training status, oxygen delivery, vascular responsiveness, near-infrared spectroscopy
Citation: George MA, McLay KM, Doyle-Baker PK, Reimer RA and Murias JM (2018) Fitness Level and Not Aging per se, Determines the Oxygen Uptake Kinetics Response. Front. Physiol. 9:277. doi: 10.3389/fphys.2018.00277
Received: 12 December 2017; Accepted: 08 March 2018;
Published: 29 March 2018.
Edited by:
Gary W. Mack, Brigham Young University, United StatesReviewed by:
David Poole, Kansas State University, United StatesUwe Hoffmann, German Sport University Cologne, Germany
Copyright © 2018 George, McLay, Doyle-Baker, Reimer and Murias. This is an open-access article distributed under the terms of the Creative Commons Attribution License (CC BY). The use, distribution or reproduction in other forums is permitted, provided the original author(s) and the copyright owner are credited and that the original publication in this journal is cited, in accordance with accepted academic practice. No use, distribution or reproduction is permitted which does not comply with these terms.
*Correspondence: Juan M. Murias, am1tdXJpYXNAdWNhbGdhcnkuY2E=