- Department of Physiology, University of Melbourne, Parkville, VIC, Australia
γ-Aminobutyric Acid (GABA) and its receptors, GABAA,B,C, are expressed in several locations along the gastrointestinal tract. Nevertheless, a role for GABA in enteric synaptic transmission remains elusive. In this study, we characterized the expression and function of GABA in the myenteric plexus of the mouse ileum. About 8% of all myenteric neurons were found to be GABA-immunoreactive (GABA+) including some Calretinin+ and some neuronal nitric oxide synthase (nNOS+) neurons. We used Wnt1-Cre;R26R-GCaMP3 mice, which express a genetically encoded fluorescent calcium indicator in all enteric neurons and glia. Exogenous GABA increased the intracellular calcium concentration, [Ca2+]i of some myenteric neurons including many that did not express GABA or nNOS (the majority), some GABA+, Calretinin+ or Neurofilament-M (NFM)+ but rarely nNOS+ neurons. GABA+ terminals contacted a significantly larger proportion of the cell body surface area of Calretinin+ neurons than of nNOS+ neurons. Numbers of neurons with GABA-induced [Ca2+]i transients were reduced by GABAA,B,C and nicotinic receptor blockade. Electrical stimulation of interganglionic fiber tracts was used to examine possible effects of endogenous GABA release. [Ca2+]i transients evoked by single pulses were unaffected by specific antagonists for each of the 3 GABA receptor subtypes. [Ca2+]i transients evoked by 20 pulse trains were significantly amplified by GABAC receptor blockade. These data suggest that GABAA and GABAB receptors are not involved in synaptic transmission, but suggest a novel role for GABAC receptors in modulating slow synaptic transmission, as indicated by changes in [Ca2+]i transients, within the ENS.
Introduction
γ-Aminobutyric Acid (GABA), a prominent neurotransmitter in the central nervous system (CNS) is expressed by neurons of the enteric nervous system (ENS), enteroendocrine cells in the mucosa and nerve terminals in the smooth muscle layers of the gastrointestinal tract (Jessen et al., 1986; Krantis et al., 1994; Sang and Young, 1996; Krantis, 2000). GABA has been proposed to be a neurotransmitter in the ENS for decades, as early studies on the guinea-pig intestine showed that GABA can excite enteric neurons (Krantis et al., 1980; Cherubini and North, 1984a,b), and that electrical field stimulation of cultured enteric neurons releases [3H]GABA (Jessen et al., 1983). Nonetheless, its role in synaptic transmission has remained elusive.
GABA is expressed in the ENS of several species; but significant interspecies differences in the distribution of GABA neurons and fibers have been reported (Furness et al., 1989; Messenger and Furness, 1990; Sang and Young, 1996; Timmermans and Scheuermann, 1998; Krantis, 2000). Cell bodies of GABA-containing neurons are predominantly found in the myenteric plexus of the ENS and have terminals in the myenteric plexus and the longitudinal and circular muscle, and so they are thought to be interneurons and motor neurons (Hills et al., 1987; Furness et al., 1989; Sang and Young, 1996). In the large intestine of rats and mice, GABA is expressed in the cell bodies of 5–14% of all myenteric neurons (Sang and Young, 1996; Krantis, 2000), while GABA cell bodies comprise about 5% of all myenteric neurons in the guinea-pig and mouse small intestine (Williamson et al., 1996; Li et al., 2011). In the mouse small intestine, expression of GABA is reported to be sparse and hence excluded from co-localisation studies (Sang and Young, 1996, 1998). GABA may have a role in synaptic transmission in this region as there are many GABA-immunoreactive myenteric terminals in the mouse small intestine (Jessen et al., 1986; Hills et al., 1987; Sang and Young, 1996), but these have not been examined in depth.
All three major subtypes of GABA receptors have been identified within the ENS. GABAA, GABAB, and GABAC receptors are all expressed in the rat small and large intestine (Krantis et al., 1995; Nakajima et al., 1996; Poulter et al., 1999; Fletcher et al., 2001). In mouse, GABAA receptor subunits and GABAB receptors are expressed in the large intestine (Casanova et al., 2009; Seifi et al., 2014); the presence of the 3 receptor subtypes in the small intestine has been deduced from functional contractility studies (Sanger et al., 2002; Zizzo et al., 2007) although GABAC receptor expression and function has been particularly elusive in comparison to its counterparts (Auteri et al., 2015).
Unlike its predominant inhibitory role in the CNS, GABA can have both an excitatory and inhibitory influence on enteric neurons depending on the receptor it activates. GABA can act through GABAA and GABAC receptor subtypes, which are both pentameric chloride channels, to excite enteric neurons (Auteri et al., 2015). This due to the high intracellular Cl− concentration in enteric neurons, which is maintained by a Na+-K+-2Cl− symporter (Liu et al., 2013). In contrast, activation of GABAB receptors causes neuronal inhibition, as they are metabotropic G protein-coupled receptors that inhibit presynaptic voltage-activated Ca2+ channels and activate postsynaptic inwardly rectifying K+ channels (Auteri et al., 2015). The net functional output of GABA is difficult to predict as it depends on the receptor subtypes involved and their species- and region-specific localization (Auteri et al., 2015). Even within the same gut region, varying concentrations of GABA can alter the receptor subtype that is recruited leading to changes in the behavioral output (Auteri et al., 2014). While several studies have reported modulatory roles for GABA and its receptors on contractile activity in the gut (Tonini et al., 1989a,b; Sanger et al., 2002; Zizzo et al., 2007; Auteri et al., 2014), a role for GABA in synaptic transmission has not been identified.
In this study, we employed Ca2+-imaging from Wnt1-Cre;R26R-GCaMP3 mice which express a genetically encoded fluorescent calcium indicator in all enteric neurons and glia as a high throughput assay to elucidate the elusive role of GABA in synaptic transmission in the murine small intestine. We identified myenteric neuronal subtypes that express functional GABA receptors via the use of specific GABA receptor antagonists and post-hoc immunohistochemistry. Our data suggest that GABAA and GABAB receptors do not have a synaptic function within the ENS, but demonstrate a novel role for GABAC receptor in modulating slow synaptic transmission within the ENS.
Materials and Methods
Experimental Animals and Tissue Preparation
Mice of either sex from a C57Bl/6 background aged 8–12 weeks, including Wnt1-Cre;R26R-GCaMP3 mice, in which neural crest-derived cells express the genetically encoded calcium indicator, GCaMP3 (Zariwala et al., 2012; Boesmans et al., 2013) were used. Wnt1-Cre;R26R-GCaMP3 mice were the progeny of Wnt1-Cre mice (Danielian et al., 1998) and R26R-GCaMP3 mice (The Jackson Laboratory). Mice were killed by cervical dislocation; a procedure approved by the University of Melbourne Animal Experimentation Ethics Committee. A segment of distal ileum, 2 cm proximal to the ileo-caecal junction, was removed from each mouse and immediately placed in physiological saline (composition in mM: NaCl 118, NaHCO3 25, D-glucose 11, KCl 4.8, CaCl2 2.5, MgSO4 1.2, NaH2PO4 1.0) bubbled with carbogen gas (95% O2, 5% CO2). The ileal segments were then cut along the mesenteric border, stretched and pinned flat mucosal side up in a Petri dish lined with a silicone elastomer (Sylgard 184; Dow Corning, North Ryde, NSW, Australia).
Expression of GABA and Other Neuronal Subtype Markers
Pinned and stretched segments of ileum of mice from a C57/Bl6 background were fixed overnight in 4% formaldehyde in 0.1 M phosphate buffer, pH 7.2, at 4°C, and then the tissue was given three washes with phosphate-buffered saline (PBS). Longitudinal muscle-myenteric plexus (LMMP) whole-mount preparations were obtained by firstly removing the mucosa-submucosa layer, then stripping away overlying circular muscle via microdissection. These preparations were incubated for 30 min with 1% triton X-100 (ProSciTech, Thuringowa, QLD, Australia) at room temperature. The tissue was then given three washes with PBS, followed by overnight incubation with a combination of primary antibodies (Table 1) at 4°C. After three washes with PBS, the tissue was incubated with a combination of relevant secondary antibodies (Table 1) for about 2.5 h at room temperature. The tissue was given another three washes with PBS, and then mounted onto a slide with Dako fluorescent mounting medium (Carpinteria, Califonia, USA).
Preparations of myenteric plexus were viewed using a Zeiss Axio Imager M2 microscope and images were acquired with a Axiocam 506 mono camera using Zen 2.3 (blue edition) software (all from Zeiss, Australia). Images were taken using 20x or 40x objectives. The proportion of Calretinin and/or nNOS and/or GABA expressing neurons was obtained by examining co-expression with the pan-neuronal marker, Hu. GABA staining within neuronal cell bodies in this study was found to be highly variable, but unambiguous. Myenteric ganglia were imaged and a neuron was considered to be immunoreactive for GABA if the staining of its cytoplasm was higher than the background such that an unstained nucleus could be seen. At least 200 Hu+ cell bodies were counted in each preparation. The mean proportion of each neuronal subtype was determined by calculating averages from 3 animals. The data are expressed as mean ± SEM and n = the number of cells examined. Statistical analyses were performed using unpaired t-tests with P < 0.05 considered statistically significant. Comparisons were performed using using GraphPad Prism 5.0 (GraphPad Softwares, San Diego California).
To quantify GABA-immunoreactive terminals apposing Calretinin- and nNOS- immunoreactive neurons, high-resolution z-stacks of preparations co-stained with GABA and Calretinin or nNOS were imaged using a Zeiss LSM800 confocal microscope. The images were sampled at a resolution of 1,024 × 1,024 pixels using the 63x/1.40 Oil DIC M27 objective, with a 1.8x software zoom and z steps of 0.43 μm. Fourteen to seventeen Calretinin- or nNOS-immunoreactive neurons were examined from each preparation. A total of 3 preparations, each from a different animal, was examined. 3D rendered surfaces of the neuronal cell-bodies and GABA terminals were generated using the 3D image analysis software Imaris x64 (Bitplane, version 9.0.0). The percentage of total cell surface area covered by GABA-ergic terminals was determined for each Calretinin- or nNOS-immunoreactive neuron. Data are presented as the mean % surface area ± SEM, where n = number of neurons examined. Statistical analyses were performed using unpaired t-tests with P < 0.05 considered statistically significant. Comparisons were performed using using GraphPad Prism 5.0 (GraphPad Softwares, San Diego California).
Calcium Imaging
Tissue Preparation
Segments of ileum were removed from Wnt1-Cre;R26R-GCaMP3 mice, cut along the mesenteric border and pinned flat, mucosa side up in a dish lined with silicone elastomer (Sylgard 184; Dow Corning). The mucosa and submucous plexus were removed from the underlying smooth muscle and myenteric plexus layers, then strips of circular muscle were carefully peeled off and the resultant LMMP preparations were stretched over a small inox ring and immobilized by a matched rubber O-ring (Vanden Berghe et al., 2002). A maximum of 5 rings was prepared from each segment of ileum. The rings were transferred to an organ bath for imaging. The bath was constantly superfused (1 ml/min) with 95% O2, 5% CO2 physiological saline at room temperature throughout the experiment via a gravity-fed inflow system that included a manual valve to switch between saline and drug-containing saline solutions.
Imaging
The ring preparations were imaged using a 20x (NA 0.5) water dipping objective on an upright Zeiss Axioskope microscope with a Zeiss AxioCam MRm camera, and images (278 × 278) were acquired at 1 Hz.
Neurons within the myenteric ganglia were stimulated either chemically or electrically. Chemical stimulation involved the pressure ejection (spritz, 2 s duration; 9 psi) of GABA (1 mM) using a micropipette (tip diameter ~20 μm) situated right at the edge of the imaged ganglion. During time control experiments GABA was applied to each ganglion via 2 repeated local applications, each separated by a 10 min time period. In initial studies, the amplitudes of GABA-evoked [Ca2+]i responses were significantly reduced at the second time point suggesting desensitization of receptors, in accordance with previous reports (Cherubini and North, 1984a,b). To prevent potential desensitization of GABA receptors, the spritz pipette was moved away from the ganglion in between applications. To electrically stimulate the neurons, a focal stimulating electrode (tungsten wire; 50 μm diameter) was placed on an interganglionic fiber tract leading into the ganglion of choice where a single pulse and a train of 20 pulses (20 Hz) were elicited. For time control experiments, ganglia were stimulated first with a single pulse and then a train of 20 pulses 5 min later. This stimulation regime was repeated 2 times 10 min apart.
To test the effects of antagonists, drug-containing saline was superfused for 10 min into the organ bath after either the first GABA spritz or electrical stimulation regime, so that the first spritz or electrical time point was taken as the control response. Each ringed preparation was only exposed to an antagonist, or a combination of antagonists, once.
Following live-imaging experiments, selected preparations tissues were fixed overnight with 4% formaldehyde at 4°C and immunostained using primary antisera to the neuron subtype markers, GABA, nNOS, neurofilament-M (NFM) and/or calretinin together with secondary appropriate antisera (Table 1), to identify the neurochemistry of the responding neurons.
Data Analysis and Statistics
Analyses were performed using custom-written directives in IGOR Pro (WaveMetrics, Lake Oswego, Oregon, USA). Regions of interest were drawn over a selected area of the cytoplasm for each neuron, excluding the nucleus because GCaMP3 is absent from the nuclei (Tian et al., 2009; Yamada and Mikoshiba, 2012). The intensity of the intracellular calcium ([Ca2+]i) transient signal for each response was calculated and expressed as the maximum increase in [Ca2+]i from the baseline signal (ΔFi/F0). [Ca2+]i transients were only considered if the intensity of the transient signal was more than 5 times the intrinsic noise. For both time control and antagonist experiments the ΔFi/F0 of the second GABA spritz, or second electrical stimulation response, was normalized and expressed as a fraction of the first (%ΔFi/F0). A minimum of 3 animals were examined for each experimental set; unless stated otherwise.
Ganglia of interest that were examined with Ca2+-imaging were processed for post-hoc immunohistochemistry and relocated using 20x or 40x objectives of a Zeiss Axio Imager M2 microscope. Images were acquired with a Axiocam 506 mono camera using Zen 2.3 (blue edition) software (all from Zeiss, Australia). Responding GCaMP3+ neurons were identified immunohistochemically by matching the micrographs with the Ca2+-imaging videos.
Data are presented as the mean % ΔFi/F0 of the control ± SEM where n = number of neurons examined or as mean ± SEM and n = the number of cells examined. Statistical analyses were performed using one-way analysis of variance (ANOVA) followed by Dunnett's post-hoc test with P < 0.05 considered statistically significant. Comparisons were performed using GraphPad Prism 5.0 (GraphPad Softwares, San Diego California).
Drugs Used
Drugs used included GABA, hexamethonium bromide (both from Sigma Aldrich, Castle Hill, New South Wales, Australia), Bicuculline, [S-(R*,R*)]-[3-[[1-(3,4-Dichlorophenyl)ethyl]amino]-2-hydroxypropyl](cyclohexylmethyl) phosphinic acid (CGP 54626) and (1,2,5,6-Tetrahydropyridin-4-yl)methylphosphinic acid (TPMPA) (all from Tocris Bioscience, Avonmouth Bristol UK). All drugs were diluted in distilled water to make stock solutions and then again in physiological saline to their working concentration on the day of experimentation.
Results
Expression of GABA in the Distal Ileal Myenteric Plexus
GABA+ neurons are relatively uncommon in the myenteric plexus of the mouse ileum and very little is known about their functional classification in this gut region (Sang and Young, 1996; Li et al., 2011). nNOS+ neurons have been described mainly as inhibitory motor neurons, while Calretinin+ neurons are intrinsic sensory neurons, interneurons and excitatory neurons to the muscle layers (Sang and Young, 1996; Li et al., 2011). In this study, GABA immunoreactivity was expressed in the somata of 8.2 ± 0.7% of Hu+ cells (n = 3 animals, Figure 1). GABA+ terminals and varicosities were also present within the myenteric ganglia and muscle layers (Figure 1A). nNOS immunoreactivity was observed in 24.3 ± 2%, while Calretinin immunoreactivity was found in 33 ± 1.4% of Hu+ neurons (both n = 3 animals, Figure 1). This is similar to previous reports in the mouse ileum (Sang and Young, 1996; Qu et al., 2008). Furthermore, of the total number of Hu-immunoreactive neurons examined, we found that GABA colocalised with some of the nNOS+ (2.0 ± 0.1%) and Calretinin+ (3.7 ± 0.6%) neurons.
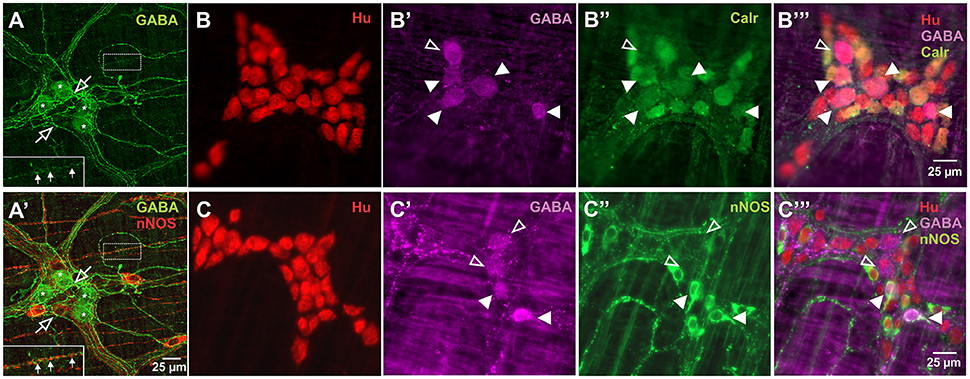
Figure 1. Expression of GABA in relation to Hu, Calretinin and nNOS stains in the myenteric plexus of the mouse ileum. Confocal micrographs of a myenteric ganglion showing GABA immunoreactivity in the somata (asterisks) of some non-nitrergic neurons, terminals and varicosities (open arrows) within the ganglion (A,A'). GABA and nNOS also stained different varicosities on muscle strips (A,A' inset, filled arrows). GABA (B',B”',C',C”'), Calretinin (B”,B”') and nNOS (C”,C”') immunoreactivity were found in some Hu+ neurons. Overall, GABA colocalised with some Calretinin+ and nNOS+ neurons. Example confocal micrographs showing GABA colocalisation with some Calretinin+ neurons (B), and nNOS+ neurons (C) (colocalisation—filled arrowheads, no colocalisation—open arrowheads).
Exogenous Application of GABA Evoked [Ca2+]i Transients Directly in Half of the Responding Neurons, the Vast Majority of Responding Neurons Do Not Express GABA or nNOS
GABA (1 mM) was spritzed onto 10 myenteric ganglia (from 6 animals) and induced a sharp increase in [Ca2+]i (ΔFi/F0 = 0.23 ± 0.03, n = 63) in 21.8 ± 2.6% of GCaMP3+ cells. GCaMP3+ cells include neurons and glia, but the responding cells were probably neurons as they had cell body sizes (~20 μm diameter) similar to those of neurons (Gabella and Trigg, 1984) and typically responded instantaneously to stimuli with a sharp increase in [Ca2+]i; glia are smaller and tend to respond with a delayed and gradual increase in [Ca2+]i (Boesmans et al., 2013). Some responding cells were identified via post-hoc immunohistochemistry, with the vast majority of all responding cells being neither GABA nor nNOS immunoreactive (GABA-/nNOS-, 72.0 ± 6.2%) or GABA+ (23.1 ± 7.1%) and rarely nNOS+ and almost never GABA+/nNOS+. The overall amplitude of calcium transients of GABA-/nNOS- responders (ΔFi/F0 = 0.24 ± 0.03, n = 47) did not differ from that of the other GABA+ and nNOS+ immunoreactive groups. However, nNOS+ responders exhibited significantly smaller response amplitudes than GABA+ responders, (GABA+: ΔFi/F0 = 0.21 ± 0.03, n = 11, nNOS+: ΔFi/F0 = 0.09 ± 0.02, n = 4, P < 0.05). Interestingly within each ganglion, most GABA+ neurons responded to GABA spritz (75.0 ± 12.0%), but nNOS+ neurons rarely did (3.4 ± 2.2%) (Figures 2A–C).
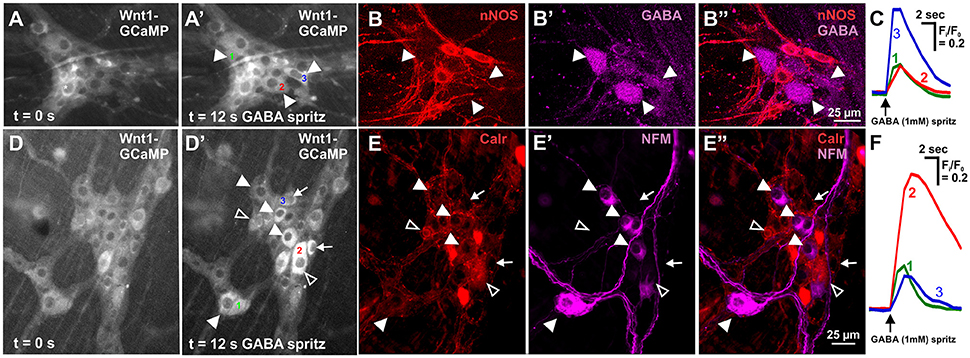
Figure 2. Neurochemistry of neurons in the mouse ileum that displayed GABA evoked [Ca2+]i transients. (A) Representative fluorescence micrographs of the GABA (1 mM) evoked [Ca2+]i response in myenteric neurons [GCaMP3 signal at rest (A, t = 0); and during GABA stimulation (A', t = 12 s)]. (A') In this ganglion, 3 neurons responded to GABA with a sharp increase in [Ca2+]i (responsive neurons marked 1–3). (B) Confocal micrographs of the myenteric ganglion showing the neurons that responded to GABA (A') were immunoreactive for GABA (filled arrowheads) and not nNOS. (C) Example traces from the 3 neurons (marked in A') that responded to GABA. (D) Representative fluorescence micrographs of the GABA (1 mM) evoked [Ca2+]i response in myenteric neurons [GCaMP3 signal at rest (D, t = 0); and during GABA stimulation (D', t = 12 s)]. (D') In this ganglion, many neurons responded to GABA with a sharp increase in [Ca2+]i (some marked with arrowheads and arrows, selected responsive neurons marked 1–3). (E) Confocal micrographs of the myenteric ganglion showing the neurons that responded to GABA (D') were immunoreactive for NFM (filled arrowheads), Calretinin (open arrowheads) or neither NFM or Calretinin (arrows). (F) Example traces from the selected 3 neurons (marked in D') that responded to GABA.
Acetylcholine activating nicotinic receptors is the primary means of neurotransmission in the ENS (Nurgali et al., 2004; Gwynne and Bornstein, 2007; Foong et al., 2012, 2014). Addition of hexamethonium (nicotinic antagonist, 200 μM) to the superfusing solution abolished GABA-evoked [Ca2+]i responses in 15/30 responding neurons (P = 0.008, Fisher's exact test; Table 2), and did not change the amplitude of GABA-evoked [Ca2+]i responses of the hexamethonium resistant neurons (n = 15, P > 0.05; Figure 3A). Ten initially responsive neurons were identified by post-hoc immunohistochemistry: 8/10 were GABA-/nNOS-, 2 were GABA+. Of these neurons, the responses of the 3 neurons that were GABA-/nNOS- and both GABA+ neurons were abolished by hexamethonium.
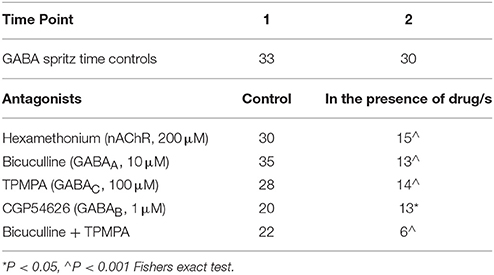
Table 2. Number of neurons responding to GABA in control conditions and in the presence of antagonists.
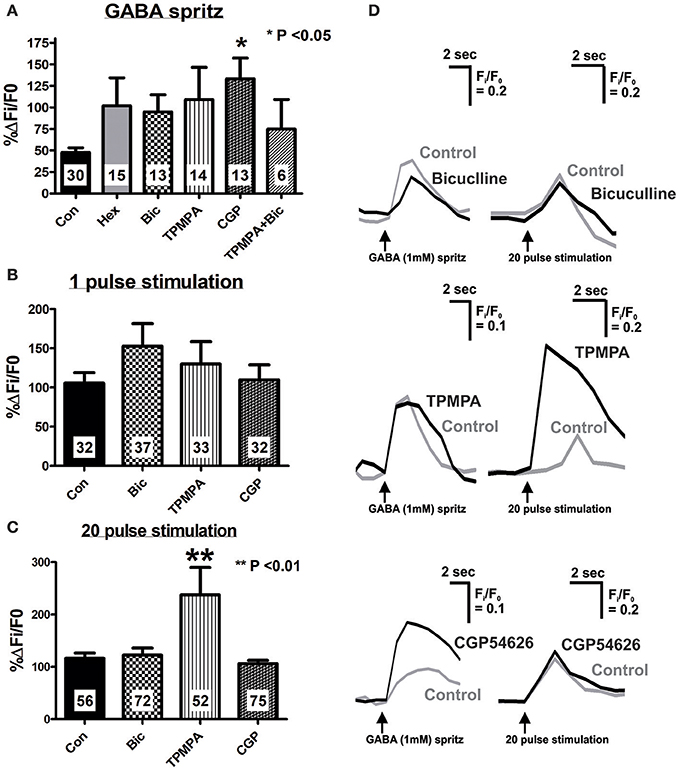
Figure 3. Effect of specific antagonists on GABA- and electrically-evoked [Ca2+]i transients. Neurons were stimulated twice, first in the present of control saline, and second after 10 min application of antagonists (Hexamethonium, Hex 100 μM; Bicuculline, Bic 10 μM; TPMPA, 100 μM; CGP54626, CGP 1 μM or a combination of antagonists). Time controls were also performed (Con) where both stimuli were executed in control saline, 10 min apart. Histograms summarizing the pooled dataset from all neurons stimulated with GABA (1 mM) (A), single pulse (B) or a train of 20 pulses (C), excluding those that were abolished in the presence of antagonists (see Tables 2, 3). Changes in amplitude after application of various antagonists are presented as a percentage of the first response in control saline. (A) CGP54626 (GABAB antagonist) significantly increased the [Ca2+]i response evoked by GABA. (B) None of the specific GABA receptor antagonists had any effect on 1 pulse evoked [Ca2+]i transients. (C) Only TPMPA had an effect on the 20 pulse evoked [Ca2+]i transients, where it significantly increased the amplitude of the [Ca2+]i response. (D) Example traces from a single neuron of GABA and 20 pulse-evoked changes in [Ca2+]i in control conditions (gray traces) and in the presence of GABA receptor antagonists, (black traces).
Calretinin Neurons Exhibit GABA Evoked [Ca2+]i Transients, and Have a Larger Proportion of Their Cell Body Surface Area Covered by GABA Terminals than nNOS Neurons
Calretinin and Neurofilament-M (NFM) are markers of excitatory and sensory neurons in the murine gut (Sang and Young, 1996; Qu et al., 2008). We found that the vast majority of GABA-responding neurons were GABA-/nNOS- and had large somata, which is suggestive of enteric sensory neurons (Qu et al., 2008). GABA (1 mM) was spritzed onto 6 myenteric ganglia (from 2 animals) and some GABA-responding neurons were found to be immunoreactive for NFM or Calretinin (Figures 2D–F). Twenty neurons responded to GABA with a sharp increase in [Ca2+]i; 16/20 were identified immunohistochemically with 9/16 being Calretinin-/NFM- and 7/16 being Calretinin+/NFM-. Of 36 neurons that responded to GABA in the presence of hexamethonium (200 μM), 35 were identified immunohistochemically: 15/35 were NFM-/Calretinin-, 9/35 were Calretinin-/NFM+, and 11/35 were Calretinin+/NFM-.
Calretinin and nNOS immunoreactivity reveals the whole shape of the neuron (Figures 4A,B) and has been previously used to examine numbers of varicosities contacting their cell bodies using confocal microscopy (Neal and Bornstein, 2007). In this study, we used high resolution confocal microscopy and Imaris surface rendering software to quantify GABA-immunoreactive terminals and varicosities that apposed cell bodies of Calretinin- and nNOS- immunoreactive neurons. Unlike synaptic proteins such as alpha-synuclein, synaptophysin, synaptotagmin and synaptobrevin which only stain varicosities (Sharrad et al., 2013), in our preparations, GABA stained axons and varicosities (Figures 4A,B). This made accurate counts of individual GABA+ varicosities onto Calretinin and nNOS cell bodies difficult, so we analyzed the % surface area of the neuronal cell bodies that were contacted by GABA-ergic axons and varicosities instead. We found that the % surface area of Calretinin+ cell bodies that was contacted by GABA-ergic axons and varicosities was significantly greater than the contacted surface area of nNOS+ cell-bodies (% surface area contacted by GABA+ structures: Calretinin 1.2 ± 0.1, 47 neurons; nNOS 0.8 ± 0.1, 44 neurons; P = 0.03) (Figure 4).
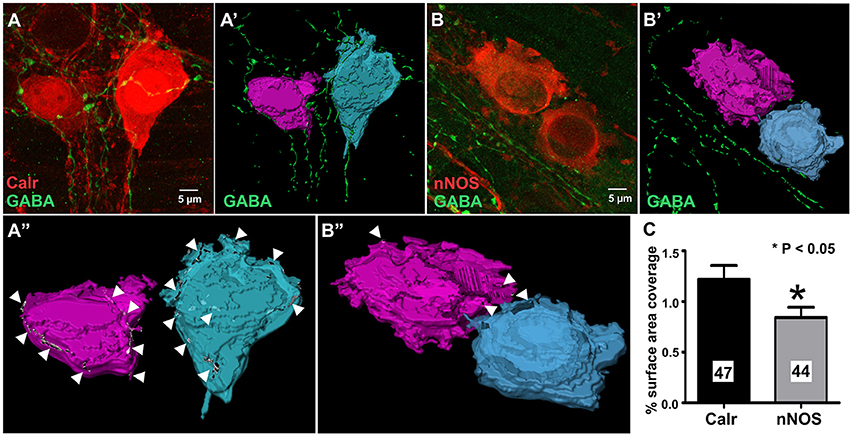
Figure 4. GABA terminals cover a larger proportion of the total cell surface area of 3D rendered calretinin- compared to nNOS- neurons. Example confocal micrographs of myenteric plexus stained for Calretinin and GABA (A), and nNOS and GABA (B). 3-D rendered surfaces of the calretinin- (A'), nNOS- (B') immunoreactive cell bodies, GABA-immunoreactive varicosities and terminals (A',B'). (A”,B”) Cell surface area receiving GABA terminals (arrow heads) detected by surface-surface contact analysis. (C) The mean percentage of total cell surface area covered by GABA terminals for Calretinin- or nNOS-immunoreactive neurons. Calretinin neurons have a significantly larger percentage surface cell body area that are covered by GABA terminals. All data were analyzed using the 3D image analysis software Imaris x64 (Bitplane, version 9.0.0).
GABA Evoked [Ca2+]i Transients Are Mediated through GABA A, B, and C Receptors
The GABAA receptor antagonist, bicuculline (10 μM, P = 0.0001, Fisher's exact test) and the GABAC receptor antagonist, TPMPA (100 μM, P = 0.005, Fisher's exact test) significantly reduced the number of neurons that displayed GABA-evoked [Ca2+]i responses (Table 2). The combined addition of bicuculline and TPMPA also abolished responses in a significant number of neurons (16/22 neurons, P = 0.0001, Fisher's exact test), but the proportion of neurons whose responses were abolished did not differ significantly from either antagonist alone (combination vs. bicuculline alone, P > 0.8; combination vs. TPMPA alone, P > 0.05, Fisher's exact test) (Table 2). The amplitudes of the responses in the remaining neurons were unchanged by GABAA (n = 13, P > 0.05; Figures 3A,D) and GABAC (n = 14, P >0.05; Figures 3A,D) blockade. Combined addition of bicuculline and TPMPA did not significantly alter the amplitudes of GABA-evoked [Ca2+]i responses that were not abolished, (%ΔFi/F0 control: 48 ± 6 %, n = 30; %ΔFi/F0 bicuculline & TPMPA: 75 ± 38% n = 6, P > 0.1) (Figure 3A).
The GABAB antagonist, CGP54626 (1 μM) abolished responses in a significant number of neurons that initially responded to GABA (P = 0.03, Fisher's exact test; Table 2). But amplitudes of [Ca2+]i responses in the remaining neurons were significantly larger than control responses (n = 13, P < 0.05; Figures 3A,D).
Involvement of Endogenous GABA in Synaptic Transmission
Electrical stimuli consisting of a single pulse or a train of 20 pulses (20 Hz) were applied via a stimulating electrode to an internodal strand leading to the ganglion imaged, which are respectively expected to evoke fast-intermediate, and fast-intermediate-slow excitatory postsynaptic potentials in the ENS (Shuttleworth and Smith, 1999; Nurgali et al., 2004; Gwynne and Bornstein, 2007; Foong et al., 2012, 2015).
Bicuculline, TPMPA and CGP54626 did not affect the number of neurons that exhibited electrically-evoked [Ca2+]i transients relative to time controls (all P > 0.1 Fisher's exact test; Table 3). The amplitude of single pulse-evoked [Ca2+]i responses was unaffected by TPMPA (n = 33, P > 0.4; Figure 3B), but the amplitudes of train-evoked [Ca2+]i transient responses were significantly potentiated by TPMPA (n = 52 P < 0.01; Figures 3C,D). Bicuculline and CGP54626 had no effect on the amplitudes of single or train-evoked responses (all P > 0.1; Figures 3B–D).
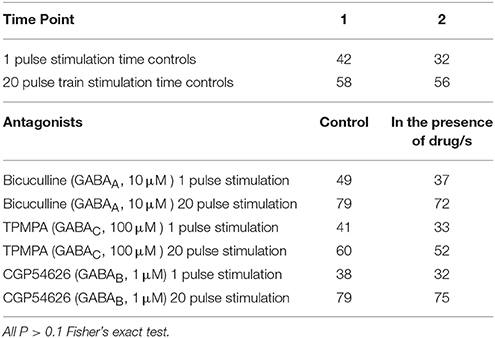
Table 3. Number of neurons responding to electrical stimulation in control conditions and in the presence of antagonists.
Discussion
The physiological roles of GABA and its receptors in the gastrointestinal tract have proved elusive as they can have excitatory or inhibitory effects on enteric neurons and their expression is highly specific to gastrointestinal regions and species (Auteri et al., 2015). In this study, we used a multifaceted approach to examine the role of GABA and its receptors in the myenteric plexus of the murine ileum. We characterized the expression of GABA in neurons and varicosities surrounding enteric neurons, and identified the neuronal subtypes that express GABA receptors using specific antagonists and Ca2+-imaging. We showed that GABA receptors are unlikely to mediate fast or intermediate EPSPs and revealed a novel role for GABAC receptors activated by endogenously released GABA in modulating slow excitatory neurotransmission in the ENS.
GABA Colocalises with Some Calretinin and nNOS Neurons in the Myenteric Plexus of the Mouse Ileum
In accordance with previous studies (Sang and Young, 1996; Li et al., 2011), we found that GABA neurons constitute 8% of all myenteric neurons in the murine ileum. Further, we found that GABA colocalised with a proportion of nNOS-immunoreactive neurons, which are often interneurons and inhibitory motor neurons, and some Calretinin-immunoreactive neurons which include intrinsic sensory, excitatory interneurons and motor neurons in the murine small intestine (Qu et al., 2008). Thus, GABA neurons may include subpopulations of such neurons. Indeed, we found GABA-immunoreactive varicosities in the circular muscle and within ganglia, where they closely apposed Calretinin-immunoreactive neurons and some nNOS-immunoreactive neurons. Thus, some GABA-containing neurons are probably interneurons contacting excitatory and inhibitory motor neurons as previously suggested for the rat colon (Krantis, 2000). This is consistent with findings from the murine colon, where widespread GABAergic innervation of neurons throughout the myenteric plexus has been identified by immunolabelling of the vesicular GABA transporter (vGAT) (Seifi et al., 2014).
Myenteric Neuronal Subtypes That Respond to GABA in the Mouse Ileum
Exogenous application of GABA evoked [Ca2+]i transients in some myenteric neurons. The vast majority of these neurons were GABA-/nNOS-, including some Calretinin- and NF-M-immunoreactive neurons. nNOS-immunoreactive neurons rarely responded to GABA. Moreover, while GABA+ terminals and varicosities made close contacts to many Calretinin+ and nNOS+ neurons, the % surface area of Calretinin+ cell bodies contacted by GABA-ergic terminals was significantly greater than the contacted area of nNOS+ cell bodies. Although most GABA-immunoreactive neurons responded to GABA, these neurons made up only a small proportion of all responding neurons, reflecting their overall numbers. Nonetheless, we cannot exclude the possibility that GABA neurons make functional contacts onto other myenteric neurons including nNOS+ neurons that we did not detect.
Earlier intracellular studies of guinea-pig ileum showed that GABA excites intrinsic sensory myenteric neurons (which display distinctive AH-type electrophysiology) via GABAA receptors. Conversely, all other types of neurons that display S-type electrophysiology, which includes interneurons and motor neurons, were insensitive to GABA (Cherubini and North, 1984a). Our study is congruent with this, as some neurons that expressed Calretinin and NF-M, both markers of intrinsic sensory neurons, responded to GABA (Qu et al., 2008). But Calretinin also labels excitatory interneurons and motor neurons, and some nNOS and GABA neurons responded, albeit in smaller numbers. Moreover, half the GABA-evoked responses were in secondary neurons of the circuitry as they were blocked by, hexamethonium indicating involvement of a cholinergic synapse. That neurons other than intrinsic sensory neurons respond to GABA, differs from the earlier findings in the guinea-pig ileum (Cherubini and North, 1984a), which may be due to differential GABA receptor expression, which has not been detailed across species.
GABAA, GABAB, and GABAC Receptors Are Expressed in the Myenteric Circuitry
GABA-mediated chloride conductance via GABAA and GABAC-receptors is known to produce depolarisations in enteric neurons, while GABAB receptor activation causes neuronal inhibition (Liu et al., 2013; Auteri et al., 2015). We found that GABAA, GABAB, and GABAC antagonists each prevent GABA-evoked calcium responses in many neurons. This suggests that some myenteric neurons, including nearly all GABA-immunoreactive neurons possess GABAA, GABAB, and GABAC receptors; some may possess more than one subtype. The concentrations of GABA receptor antagonists used were taken from previous publications (Sanger et al., 2002; Zizzo et al., 2007; Auteri et al., 2014) that showed selectivity in functional studies. However, a point to consider is that GABA antagonists have non-specific effects. In particular, bicuculline can block nicotinic receptors (Zhang and Feltz, 1991) and can act on SK (small conductance) Ca2+-activated K+ channels (Debarbieux et al., 1998; Khawaled et al., 1999; Johansson et al., 2001). Each of these actions may have implications for neuronal excitability. However, such “off-target” effects cannot account for the failure of bicuculline to alter the responses to electrical stimulation of interganglionic connectives, as nicotinic blockade would depress such responses (Foong et al., 2015) and there is no evidence for involvement of SK channels in the regulation of enteric neuron excitability. Further, at higher concentrations (~EC50 500 μM), TPMPA may weakly activate GABAB receptors (Ragozzino et al., 1996), but as 5-fold lower concentrations were used in this study, an action on GABAB receptors is unlikely.
A response to GABA that was not blocked by either GABAA or GABAC antagonists or both was identified, potentially mediated via GABAB receptors. GABAB receptor blockade abolishing responses in a significant number of neurons is a surprising finding, but application of a GABAB receptor agonist induced [Ca2+]i transients in cultured guinea-pig myenteric neurons (Reis et al., 2006). It is also possible that GABAB receptors are located on inhibitory inputs to the responding neurons, such that when they are blocked, inhibition is restored thereby decreasing the number of GABA responders.
Responses that were not abolished by a GABAB receptor antagonist were significantly larger than control responses, revealing a potential inhibitory action of GABA. Indeed GABAB receptors in the mouse gut act at the presynapse to prevent ACh release from excitatory cholinergic neurons (Sanger et al., 2002; Hyland and Cryan, 2010), which may account for the increase in neuronal activity observed following GABAB receptor blockade in this study.
In the mouse gut, GABAA and GABAB receptors have been identified in the colon (Casanova et al., 2009; Seifi et al., 2014; Auteri et al., 2015) and GABAA receptors are expressed on neurochemically diverse cell types including nNOS, ChAT, 5-HT, and SOM neurons (Seifi et al., 2014). Expression of GABAC receptors in the mouse was only deduced by contractility studies in the longitudinal muscle of the small intestine (Zizzo et al., 2007). We attempted to examine expression of GABA receptors on enteric neurons using immunohistochemistry, but failed to obtain satisfactory immunostaining with the available antisera. Thus, this component of the study was not pursued further.
Involvement of Endogenous GABA in Synaptic Transmission and a Role for GABAC Receptors
Electrical stimulation to evoke endogenous neurotransmitter release was used in this study to ascertain whether any GABA receptors and therefore endogenous GABA contribute to synaptic transmission. Using a reporter mouse which expresses GCaMP3 in a Cre-dependent manner in the Rosa26 locus (Tian et al., 2009; Zariwala et al., 2012) has revolutionized research into the examination of ENS excitability, allowing high-throughput analysis in previously challenging or impossible situations (Foong et al., 2015; Hao et al., 2017). With the omission of tissue loading steps, tissue viability and resolution are increased, background signals are reduced with the GCaMP3 indicator being specifically Wnt-1 driven to be selectively expressed in neural crest derivatives (enteric neurons and glia) within the heterogenous preparation (Boesmans et al., 2013). GCaMP3, although not the current fastest variant of genetically encoded calcium indicators in its family, can detect calcium transients with amplitudes linearly dependent on action potential number in the brain of intact mice (Tian et al., 2009). The stimulus regime and sampling frequency we used will detect [Ca2+]i transients due to neuronal activity. While the relatively slow inactivation kinetics of GCaMP3 will not resolve fast events like action potentials and subthreshold fast EPSPs (Tian et al., 2009; Michel et al., 2011), we can detect their longer term consequences as neuronal activity. The recorded single pulse-evoked [Ca2+]i transients in our study include responses to fast-intermediate synaptic events that are mediated by several neurotransmitters, but particularly include acetylcholine activating nicotinic receptors, the typical fast neurotransmitter in the ENS (Foong et al., 2015). While [Ca2+]i transients evoked by a 20 Hz train of pulses include responses to neurotransmitters mediating fast, intermediate and slower synaptic events.
In our study, single pulse-evoked [Ca2+]i responses were unaffected by any of the GABA receptor antagonists. Responses evoked by trains of stimuli were unaffected by GABAA or GABAB blockade, but potentiated by the GABAC antagonist, TPMPA, which has a strong selectivity (>100-fold) for GABAC receptors compared with GABAA or GABAB (Ragozzino et al., 1996; Johnston, 2002). Hence despite neural expression of all 3 GABA receptors, our data do not support a role for GABA in fast neurotransmission, but indicate that release of endogenous GABA modulates slow synaptic transmission, where GABA acting at GABAC receptors tonically inhibits the system. The exclusion of GABAA and GABAB receptors is surprising as there is strong evidence in the guinea-pig myenteric plexus that GABA excites neurons via GABAA receptors (Cherubini and North, 1984a,b, 1985; Zhou and Galligan, 2000), and in cultured guinea-pig myenteric neurons, application of GABA and agonists for GABAA and GABAB receptors induced [Ca2+]i transients, while GABAC agonist only elicited small responses in fewer neurons (Reis et al., 2006). Moreover, several studies have implicated functional roles for GABAA and GABAB receptors (Sanger et al., 2002; Zizzo et al., 2007; Auteri et al., 2014; Seifi et al., 2014), but there is only one report of involvement of GABAC receptors (Zizzo et al., 2007) in the control of smooth muscle contractility in the mouse gut. While interspecies and gut regional differences can be postulated, we cannot exclude a potential role of GABAA and GABAB receptors in synaptic transmission, as [Ca2+]i is only one indication of neuronal excitability so their contribution may be revealed using other types of measurements, sampling frequencies or stimulus regimes.
While GABAC receptors mediate excitation via a depolarising conductance, intrinsic differences in receptor kinetics may account for their inhibition of responses to trains of stimuli. GABAA receptors mediate rapid transient currents, while GABAC receptors produce more prolonged responses (Qian and Dowling, 1995; Lukasiewicz and Shields, 1998), where the deactivation rate or closing phase of GABAC receptors is much slower (Amin and Weiss, 1994). In addition, unlike GABAA receptors, GABAC receptors display little desensitization (Matthews et al., 1994). GABAC receptors, like those located on terminals of retinal bipolar cells produce a (tonic) standing current due to these properties (Hull et al., 2006; Palmer, 2006). Slow excitatory transmission in intrinsic sensory neurons displaying AH-type electrophysiology, is associated with a net decrease in membrane conductance due largely to inhibition of two potassium conductances; resting or “leak” K+ conductance (gK) and calcium-dependent K conductance (gKCa) (Grafe et al., 1980). While this applies to AH neurons, the situation involving S neurons is more complex as the K+ channels have not been clearly identified and there is potential for contribution by M- current channels like the KCNQ (Kv7) channels (Brown and Passmore, 2009; Hirst et al., 2015). In addition, slow transmission may also be due to an increase in chloride conductance (gCl) in myenteric neurons (Bertrand and Galligan, 1994). Inactivation of resting gK and activation of gCl leads to depolarisation of the neuron relative to the resting potential to a membrane potential approximating the reversal potential of Cl− which is estimated to be between −39 (Cherubini and North, 1984a) and −17 mV (Bertrand and Galligan, 1994) in guinea-pig myenteric neurons. Therefore, activation and prolonged opening of GABAC receptors and thus chloride conductance, would lead to a new membrane potential approximating the reversal potential of Cl−. The initial depolarisation of the cell at this membrane potential is likely to activate delayed rectifier K+ channels which are active when the membrane is depolarised to values above −30 mV (Hirst et al., 1985), producing a repolarising inhibition. This stabilization of the membrane would prevent the activation of voltage gated Na+ channels and further depolarisation, therefore allowing GABA to shunt action potential firing by a “clamping” of the membrane potential. Thus, following repetitive stimulation of interganglionic connectives, prolonged opening of these channels by endogenous GABA may be responsible for this shunting inhibition which would be released with GABAC receptor blockade, producing the potentiated response observed in this study.
Conclusions
Our study extended understanding GABA within the murine myenteric plexus in the small intestine, showing that some GABA-immunoreactive neurons are probably interneurons as in other species. Broadly surveying the ENS via Ca2+-imaging has enabled us to observe the previously elusive endogenous effects of GABA, to show expression of all 3 GABA receptor subtypes, and for the first time reveal a role for modulating synaptic transmission in the myenteric plexus via GABAC receptors. The specific actions of GABAC receptors will require more detailed analysis of the membrane potential, which would be via intracellular recording. However, recording individual neurons via sharp electrodes from a sufficient sample size of neurons is technically difficult and outside the scope of study. Predictions of neuronal behavior via realistic computational modeling experiments can also be employed in the future to examine the net effect of the activation of various receptors at neuronal synapses.
Author Contributors
KK, MS, JB, and JF conceived and designed the experiments. KK, CF, MS, and JF performed the experiments. KK, MS, and JF analyzed the data. JB and JF contributed reagents, materials, analysis tools. KK, JB, and JF wrote the manuscript. All authors contributed to editing and revising the manuscript. All authors read and approved the final manuscript.
Funding
This research was supported by National Health and Medical Research Council of Australia Project grants #1099016 (JF and JB), and Australian Research Council grant #DP130101596 (JB).
Conflict of Interest Statement
The authors declare that the research was conducted in the absence of any commercial or financial relationships that could be construed as a potential conflict of interest.
Acknowledgments
We thank H. Young for kindly providing the transgenic mice (Wnt1-Cre; R26R-GCaMP3) and Annette Bergner for excellent technical assistance.
References
Amin, J., and Weiss, D. S. (1994). Homomeric rho 1 GABA channels: activation properties and domains. Recept. Channels 2, 227–236.
Auteri, M., Zizzo, M. G., Mastropaolo, M., and Serio, R. (2014). Opposite role played by GABAA and GABAB receptors in the modulation of peristaltic activity in mouse distal colon. Eur. J. Pharmacol. 731, 93–99. doi: 10.1016/j.ejphar.2014.03.003
Auteri, M., Zizzo, M. G., and Serio, R. (2015). GABA and GABA receptors in the gastrointestinal tract: from motility to inflammation. Pharmacol. Res. 93, 11–21. doi: 10.1016/j.phrs.2014.12.001
Bertrand, P. P., and Galligan, J. J. (1994). Contribution of chloride conductance increase to slow EPSC and tachykinin current in guinea-pig myenteric neurones. J. Physiol. 481(Pt 1), 47–60. doi: 10.1113/jphysiol.1994.sp020418
Boesmans, W., Martens, M. A., Weltens, N., Hao, M. M., Tack, J., Cirillo, C., et al. (2013). Imaging neuron-glia interactions in the enteric nervous system. Front. Cell. Neurosci. 7:183. doi: 10.3389/fncel.2013.00183
Brown, D. A., and Passmore, G. M. (2009). Neural KCNQ (Kv7) channels. Br. J. Pharmacol. 156, 1185–1195. doi: 10.1111/j.1476-5381.2009.00111.x
Casanova, E., Guetg, N., Vigot, R., Seddik, R., Julio-Pieper, M., Hyland, N. P., et al. (2009). A mouse model for visualization of GABA(B) receptors. Genesis 47, 595–602. doi: 10.1002/dvg.20535
Cherubini, E., and North, R. A. (1984a). Actions of gamma-aminobutyric acid on neurones of guinea-pig myenteric plexus. Br. J. Pharmacol. 82, 93–100. doi: 10.1111/j.1476-5381.1984.tb16445.x
Cherubini, E., and North, R. A. (1984b). Inhibition of calcium spikes and transmitter release by gamma-aminobutyric acid in the guinea-pig myenteric plexus. Br. J. Pharmacol. 82, 101–105. doi: 10.1111/j.1476-5381.1984.tb16446.x
Cherubini, E., and North, R. A. (1985). Benzodiazepines both enhance gamma-aminobutyrate responses and decrease calcium action potentials in guinea-pig myenteric neurones. Neuroscience 14, 309–315. doi: 10.1016/0306-4522(85)90180-0
Danielian, P. S., Muccino, D., Rowitch, D. H., Michael, S. K., and McMahon, A. P. (1998). Modification of gene activity in mouse embryos in utero by a tamoxifen-inducible form of Cre recombinase. Curr. Biol. 8, 1323–1326. doi: 10.1016/S0960-9822(07)00562-3
Debarbieux, F., Brunton, J., and Charpak, S. (1998). Effect of bicuculline on thalamic activity: a direct blockade of IAHP in reticularis neurons. J. Neurophysiol. 79, 2911–2918. doi: 10.1152/jn.1998.79.6.2911
Fletcher, E. L., Clark, M. J., Senior, P., and Furness, J. B. (2001). Gene expression and localization of GABA(C) receptors in neurons of the rat gastrointestinal tract. Neuroscience 107, 181–189. doi: 10.1016/S0306-4522(01)00339-6
Foong, J. P., Hirst, C. S., Hao, M. M., McKeown, S. J., Boesmans, W., Young, H. M., et al. (2015). Changes in nicotinic neurotransmission during enteric nervous system development. J. Neurosci. 35, 7106–7115. doi: 10.1523/JNEUROSCI.4175-14.2015
Foong, J. P., Nguyen, T. V., Furness, J. B., Bornstein, J. C., and Young, H. M. (2012). Myenteric neurons of the mouse small intestine undergo significant electrophysiological and morphological changes during postnatal development. J. Physiol. 590, 2375–2390. doi: 10.1113/jphysiol.2011.225938
Foong, J. P., Tough, I. R., Cox, H. M., and Bornstein, J. C. (2014). Properties of cholinergic and non-cholinergic submucosal neurons along the mouse colon. J. Physiol. 592, 777–793. doi: 10.1113/jphysiol.2013.265686
Furness, J. B., Trussell, D. C., Pompolo, S., Bornstein, J. C., Maley, B. E., and Storm-Mathisen, J. (1989). Shapes and projections of neurons with immunoreactivity for gamma-aminobutyric acid in the guinea-pig small intestine. Cell Tissue Res. 256, 293–301. doi: 10.1007/BF00218886
Gabella, G., and Trigg, P. (1984). Size of neurons and glial cells in the enteric ganglia of mice, guinea-pigs, rabbits and sheep. J. Neurocytol. 13, 49–71. doi: 10.1007/BF01148318
Grafe, P., Mayer, C. J., and Wood, J. D. (1980). Synaptic modulation of calcium-dependent potassium conductance in myenteric neurones in the guinea-pig. J. Physiol. 305, 235–248. doi: 10.1113/jphysiol.1980.sp013360
Gwynne, R. M., and Bornstein, J. C. (2007). Synaptic transmission at functionally identified synapses in the enteric nervous system: roles for both ionotropic and metabotropic receptors. Curr. Neuropharmacol. 5, 1–17. doi: 10.2174/157015907780077141
Hao, M. M., Bergner, A. J., Hirst, C. S., Stamp, L. A., Casagranda, F., Bornstein, J. C., et al. (2017). Spontaneous calcium waves in the developing enteric nervous system. Dev. Biol. 428, 74–87. doi: 10.1016/j.ydbio.2017.05.018
Hills, J. M., Jessen, K. R., and Mirsky, R. (1987). An immunohistochemical study of the distribution of enteric GABA-containing neurons in the rat and guinea-pig intestine. Neuroscience 22, 301–312. doi: 10.1016/0306-4522(87)90220-X
Hirst, C. S., Foong, J. P., Stamp, L. A., Fegan, E., Dent, S., Cooper, E. C., et al. (2015). Ion channel expression in the developing enteric nervous system. PLoS ONE 10:e0123436. doi: 10.1371/journal.pone.0123436
Hirst, G. D., Johnson, S. M., and van Helden, D. F. (1985). The calcium current in a myenteric neurone of the guinea-pig ileum. J. Physiol. 361, 297–314. doi: 10.1113/jphysiol.1985.sp015647
Hull, C., Li, G. L., and von Gersdorff, H. (2006). GABA transporters regulate a standing GABAC receptor-mediated current at a retinal presynaptic terminal. J. Neurosci. 26, 6979–6984. doi: 10.1523/JNEUROSCI.1386-06.2006
Hyland, N. P., and Cryan, J. F. (2010). A Gut Feeling about GABA: focus on GABA(B) Receptors. Front. Pharmacol. 1:124. doi: 10.3389/fphar.2010.00124
Jessen, K. R., Hills, J. M., Dennison, M. E., and Mirsky, R. (1983). γ-aminobutyrate as an autonomic neurotransmitter: release and uptake of [3H]γ-aminobutyrate in guinea pig large intestine and cultured enteric neurons using physiological methods and electron microscopic autoradiography. Neuroscience 10, 1427–1442. doi: 10.1016/0306-4522(83)90124-0
Jessen, K. R., Hills, J. M., and Saffrey, M. J. (1986). Immunohistochemical demonstration of GABAergic neurons in the enteric nervous system. J. Neurosci. 6, 1628–1634.
Johansson, S., Druzin, M., Haage, D., and Wang, M. (2001). The functional role of a bicuculline-sensitive Ca2+-activated K+ current in rat medial preoptic neurons. J. Physiol. 532, 625–635. doi: 10.1111/j.1469-7793.2001.0625e.x
Johnston, G. A. (2002). Medicinal chemistry and molecular pharmacology of GABA(C) receptors. Curr. Top. Med. Chem. 2, 903–913. doi: 10.2174/1568026023393453
Khawaled, R., Bruening-Wright, A., Adelman, J. P., and Maylie, J. (1999). Bicuculline block of small-conductance calcium-activated potassium channels. Pflügers Archiv. 438, 314–321. doi: 10.1007/s004240050915
Krantis, A. (2000). GABA in the mammalian enteric nervous system. News Physiol. Sci. 15, 284–290. doi: 10.1152/physiologyonline.2000.15.6.284
Krantis, A., Costa, M., Furness, J. B., and Orbach, J. (1980). gamma-Aminobutyric acid stimulates intrinsic inhibitory and excitatory nerves in the guinea-pig intestine. Eur. J. Pharmacol. 67, 461–468. doi: 10.1016/0014-2999(80)90187-9
Krantis, A., Shabnavard, L., Nichols, K., de Blas, A. L., and Staines, W. (1995). Localization of GABAA receptor immunoreactivity in NO synthase positive myenteric neurones. J. Auton. Nerv. Syst. 53, 157–165. doi: 10.1016/0165-1838(94)00180-R
Krantis, A., Tufts, K., Nichols, K., and Morris, G. P. (1994). [3H]GABA uptake and GABA localization in mucosal endocrine cells of the rat stomach and colon. J. Auton. Nerv. Syst. 47, 225–232. doi: 10.1016/0165-1838(94)90183-X
Li, Z., Chalazonitis, A., Huang, Y. Y., Mann, J. J., Margolis, K. G., Yang, Q. M., et al. (2011). Essential roles of enteric neuronal serotonin in gastrointestinal motility and the development/survival of enteric dopaminergic neurons. J. Neurosci. 31, 8998–9009. doi: 10.1523/JNEUROSCI.6684-10.2011
Liu, S., Ji, T., Ren, W., Qu, M. H., Zhu, J. X., and Wood, J. D. (2013). Role of Na-K-2Cl symporter in GABA-evoked excitation in rat enteric neurons. FASEB J. 27, 1160–1165.
Lukasiewicz, P. D., and Shields, C. R. (1998). Different combinations of GABAA and GABAC receptors confer distinct temporal properties to retinal synaptic responses. J. Neurophysiol. 79, 3157–3167. doi: 10.1152/jn.1998.79.6.3157
Matthews, G., Ayoub, G. S., and Heidelberger, R. (1994). Presynaptic inhibition by GABA is mediated via two distinct GABA receptors with novel pharmacology. J. Neurosci. 14, 1079–1090.
Messenger, J. P., and Furness, J. B. (1990). Projections of chemically-specified neurons in the guinea-pig colon. Arch. Histol. Cytol. 53, 467–495. doi: 10.1679/aohc.53.467
Michel, K., Michaelis, M., Mazzuoli, G., Mueller, K., Vanden Berghe, P., and Schemann, M. (2011). Fast calcium and voltage-sensitive dye imaging in enteric neurones reveal calcium peaks associated with single action potential discharge. J. Physiol. 589, 5941–5947. doi: 10.1113/jphysiol.2011.219550
Nakajima, K., Tooyama, I., Kuriyama, K., and Kimura, H. (1996). Immunohistochemical demonstration of GABAB receptors in the rat gastrointestinal tract. Neurochem. Res. 21, 211–215. doi: 10.1007/BF02529137
Neal, K. B., and Bornstein, J. C. (2007). Mapping 5-HT inputs to enteric neurons of the guinea-pig small intestine. Neuroscience 145, 556–567. doi: 10.1016/j.neuroscience.2006.12.017
Nurgali, K., Stebbing, M. J., and Furness, J. B. (2004). Correlation of electrophysiological and morphological characteristics of enteric neurons in the mouse colon. J. Compar. Neurol. 468, 112–124. doi: 10.1002/cne.10948
Palmer, M. J. (2006). Functional segregation of synaptic GABAA and GABAC receptors in goldfish bipolar cell terminals. J. Physiol. 577, 45–53. doi: 10.1113/jphysiol.2006.119560
Poulter, M. O., Singhal, R., Brown, L. A., and Krantis, A. (1999). GABA(A) receptor subunit messenger RNA expression in the enteric nervous system of the rat: implications for functional diversity of enteric GABA(A) receptors. Neuroscience 93, 1159–1165. doi: 10.1016/S0306-4522(99)00174-8
Qian, H., and Dowling, J. E. (1995). GABAA and GABAC receptors on hybrid bass retinal bipolar cells. J. Neurophysiol. 74, 1920–1928. doi: 10.1152/jn.1995.74.5.1920
Qu, Z. D., Thacker, M., Castelucci, P., Bagyánszki, M., Epstein, M. L., and Furness, J. B. (2008). Immunohistochemical analysis of neuron types in the mouse small intestine. Cell Tissue Res. 334, 147–161. doi: 10.1007/s00441-008-0684-7
Ragozzino, D., Woodward, R. M., Murata, Y., Eusebi, F., Overman, L. E., and Miledi, R. (1996). Design and in vitro pharmacology of a selective gamma-aminobutyric acidC receptor antagonist. Mol. Pharmacol. 50, 1024–1030.
Reis, H. J., Vanden Berghe, P., Romano-Silva, M. A., and Smith, T. K. (2006). GABA-induced calcium signaling in cultured enteric neurons is reinforced by activation of cholinergic pathways. Neuroscience 139, 485–494. doi: 10.1016/j.neuroscience.2005.12.023
Sang, Q., and Young, H. M. (1996). Chemical coding of neurons in the myenteric plexus and external muscle of the small and large intestine of the mouse. Cell Tissue Res. 284, 39–53. doi: 10.1007/s004410050565
Sang, Q., and Young, H. M. (1998). The identification and chemical coding of cholinergic neurons in the small and large intestine of the mouse. Anatomical Record 251, 185–199. doi: 10.1002/(SICI)1097-0185(199806)251:2<185::AID-AR6>3.0.CO;2-Y
Sanger, G. J., Munonyara, M. L., Dass, N., Prosser, H., Pangalos, M. N., and Parsons, M. E. (2002). GABA(B) receptor function in the ileum and urinary bladder of wildtype and GABA(B1) subunit null mice. Autonomic Autacoid Pharmacol. 22, 147–154. doi: 10.1046/j.1474-8673.2002.00254.x
Seifi, M., Brown, J. F., Mills, J., Bhandari, P., Belelli, D., Lambert, J. J., et al. (2014). Molecular and functional diversity of GABA-A receptors in the enteric nervous system of the mouse colon. J. Neurosci. 34, 10361–10378. doi: 10.1523/JNEUROSCI.0441-14.2014
Sharrad, D. F., Gai, W. P., and Brookes, S. J. (2013). Selective coexpression of synaptic proteins, alpha-synuclein, cysteine string protein-alpha, synaptophysin, synaptotagmin-1, and synaptobrevin-2 in vesicular acetylcholine transporter-immunoreactive axons in the guinea pig ileum. J. Compar. Neurol. 521, 2523–2537. doi: 10.1002/cne.23296
Shuttleworth, C. W., and Smith, T. K. (1999). Action potential-dependent calcium transients in myenteric S neurons of the guinea-pig ileum. Neuroscience 92, 751–762. doi: 10.1016/S0306-4522(99)00012-3
Tian, L., Hires, S. A., Mao, T., Huber, D., Chiappe, M. E., Chalasani, S. H., et al. (2009). Imaging neural activity in worms, flies and mice with improved GCaMP calcium indicators. Nat. Methods 6, 875–881. doi: 10.1038/nmeth.1398
Timmermans, M. W., and Scheuermann, D. W. (1998). Distributional pattern and targets of GABA-containing neurons in the porcine small and large intestine. Eur. J. Morphol. 36, 133–142. doi: 10.1076/ejom.36.3.0133
Tonini, M., Costa, L. G., Candura, S. M., Olibet, G., Rizzi, C. A., Garlaschelli, L., et al. (1989a). Interaction of the pyrethroid insecticides tetramethrin and cypermethrin with enteric cholinergic transmission in the guinea-pig. Neurotoxicology 10, 707–715.
Tonini, M., De Petris, G., Onori, L., Manzo, L., Rizzi, C. A., and Crema, A. (1989b). The role of GABAA receptor function in peristaltic activity of the guinea-pig ileum: a comparative study with bicuculline, SR 95531 and picrotoxinin. Br. J. Pharmacol. 97, 556–562. doi: 10.1111/j.1476-5381.1989.tb11985.x
Vanden Berghe, P., Kenyon, J. L., and Smith, T. K. (2002). Mitochondrial Ca2+ uptake regulates the excitability of myenteric neurons. J. Neurosci. 22, 6962–6971.
Williamson, S., Pompolo, S., and Furness, J. B. (1996). GABA and nitric oxide synthase immunoreactivities are colocalized in a subset of inhibitory motor neurons of the guinea-pig small intestine. Cell Tissue Res. 284, 29–37. doi: 10.1007/s004410050564
Yamada, Y., and Mikoshiba, K. (2012). Quantitative comparison of novel GCaMP-type genetically encoded Ca2+ indicators in mammalian neurons. Front. Cell. Neurosci. 6:41. doi: 10.3389/fncel.2012.00041
Zariwala, H. A., Borghuis, B. G., Hoogland, T. M., Madisen, L., Tian, L., De Zeeuw, C. I., et al. (2012). A Cre-dependent GCaMP3 reporter mouse for neuronal imaging in vivo. J. Neurosci. 32, 3131–3141. doi: 10.1523/JNEUROSCI.4469-11.2012
Zhang, Z. W., and Feltz, P. (1991). Bicuculline blocks nicotinic acetylcholine response in isolated intermediate lobe cells of the pig. Br. J. Pharmacol. 102, 19–22. doi: 10.1111/j.1476-5381.1991.tb12125.x
Zhou, X., and Galligan, J. J. (2000). GABA(A) receptors on calbindin-immunoreactive myenteric neurons of guinea pig intestine. J. Auton. Nerv. Syst. 78, 122–135. doi: 10.1016/S0165-1838(99)00065-X
Keywords: GABA, GABA receptors, enteric nervous system, synaptic transmission, myenteric plexus
Citation: Koussoulas K, Swaminathan M, Fung C, Bornstein JC and Foong JPP (2018) Neurally Released GABA Acts via GABAC Receptors to Modulate Ca2+ Transients Evoked by Trains of Synaptic Inputs, but Not Responses Evoked by Single Stimuli, in Myenteric Neurons of Mouse Ileum. Front. Physiol. 9:97. doi: 10.3389/fphys.2018.00097
Received: 15 November 2017; Accepted: 29 January 2018;
Published: 13 February 2018.
Edited by:
Jan D. Huizinga, McMaster University, CanadaReviewed by:
Anthony Krantis, University of Ottawa, CanadaDe-Pei Li, University of Texas MD Anderson Cancer Center, United States
Copyright © 2018 Koussoulas, Swaminathan, Fung, Bornstein and Foong. This is an open-access article distributed under the terms of the Creative Commons Attribution License (CC BY). The use, distribution or reproduction in other forums is permitted, provided the original author(s) and the copyright owner are credited and that the original publication in this journal is cited, in accordance with accepted academic practice. No use, distribution or reproduction is permitted which does not comply with these terms.
*Correspondence: Jaime P. P. Foong, ai5mb29uZ0B1bmltZWxiLmVkdS5hdQ==
†Present Address: Candice Fung, Laboratory for Enteric Neuroscience, Translational Research Center for Gastrointestinal Disorders, University of Leuven, Leuven, Belgium