- 1Key Laboratory of Genetic Breeding and Aquaculture Biology of Freshwater Fishes, Ministry of Agriculture, Freshwater Fisheries Research Center, Chinese Academy of Fishery Sciences, Wuxi, China
- 2Guangxi Academy of Fishery Sciences, Nanning, China
- 3Agriculture Ministry Key Laboratory of Healthy Freshwater Aquaculture, Zhejiang Institute of Freshwater Fisheries, Huzhou, China
Hypoxia represents a major physiological challenge for prawns and is a problem in aquaculture. Therefore, an understanding of the metabolic response mechanism of economically important prawn species to hypoxia and re-oxygenation is essential. However, little is known about the intrinsic mechanisms by which the oriental river prawn Macrobrachium nipponense copes with hypoxia at the metabolic level. In this study, we conducted gas chromatography-mass spectrometry-based metabolomics studies and assays of energy metabolism-related parameters to investigate the metabolic mechanisms in the hepatopancreas of M. nipponense in response to 2.0 O2/L hypoxia for 6 and 24 h, and reoxygenation for 6 h following hypoxia for 24 h. Prawns under hypoxic stress displayed higher glycolysis-related enzyme activities and lower mRNA expression levels of aerobic respiratory enzymes than those in the normoxic control group, and those parameters returned to control levels in the reoxygenated group. Our results showed that hypoxia induced significant metabolomic alterations in the prawn hepatopancreas within 24 h. The main metabolic alterations were depletion of amino acids and 2-hydroxybutanoic acid and accumulation of lactate. Further, the findings indicated that hypoxia disturbed energy metabolism and induced antioxidant defense regulation in prawns. Surprisingly, recovery from hypoxia (i.e., reoxygenation) significantly affected 25 metabolites. Some amino acids (valine, leucine, isoleucine, lysine, glutamate, and methionine) were markedly decreased compared to the control group, suggesting that increased degradation of amino acids occurred to provide energy in prawns at reoxygenation conditions. This study describes the acute metabolomic alterations that occur in prawns in response to hypoxia and demonstrates the potential of the altered metabolites as biomarkers of hypoxia.
Introduction
The level of dissolved oxygen is a key indicator of water quality, and partially determines the intensity of crustacean aquaculture. Much research has focused on the negative effects of hypoxia on crustaceans. At hypoxic conditions, tissues must increase anaerobic energy production, improve energy use, or lower energy consumption. The responses of crustaceans to hypoxia may result in physiological, cellular, molecular and behavioral changes that depend on the duration and level of the hypoxic stress: these changes can be seen in the behavioral responses of some species (Wu, 2002; Bell and Eggleston, 2005; Craig et al., 2005), oxygen transport (Mangum and Rainer, 1988; Mangum, 1997), growth and reproduction (Ocampo et al., 2000; Brown-Peterson et al., 2008, 2011), immune response (Qiu et al., 2011; Kniffin et al., 2014), transcriptomic responses (Li and Brouwer, 2009, 2013; Sun et al., 2015), and proteomic responses (Jiang et al., 2009; Sun et al., 2016b). However, in physiology research on aquatic invertebrates, no study to date has reported a comparative analysis of the metabolome profiles in crustaceans under hypoxic conditions.
Recently, metabolomics studies have been widely applied in aquatic animals to elucidate the biological effects of hypoxic stressors on organisms (Hines et al., 2007; Hallman et al., 2008; Tuffnail et al., 2009; Lardon et al., 2013a,b). Several analytical techniques have been well established and are frequently applied in metabolomics studies (Luo et al., 2007; Nudi et al., 2008; Shao et al., 2015; Li et al., 2017), such as liquid chromatography–mass spectrometry (LC–MS), nuclear magnetic resonance (NMR), high-performance liquid chromatography (HPLC), and gas chromatography–mass spectrometry (GC–MS). Among these analytical techniques, GC-MS is frequently used in metabolomics studies and is highly sensitive and reproducible (Ruan et al., 2013; Ren et al., 2015). Importantly, GC-MS-based metabolomics analysis has been used to investigate the biological effects of sulfide pollution in shrimp (Li et al., 2017). Their work confirmed the applicability of GC-MS-based metabolomics analysis to characterize the biological effects of environmental stressors in crustaceans.
Macrobrachium nipponense (Crustacea; Decapoda; Palaemonidae), also called the oriental river prawn, is an important aquaculture species that is distributed widely in freshwater and low-salinity estuarine regions in Asia (Ma et al., 2011). Prawns are relatively susceptible to hypoxia compared with most crustaceans (Sun et al., 2015). Thus, in prawn production, hypoxia may cause large economic losses because of increased mortality and decreased growth rate. In crustaceans, the functions of the hepatopancreas include carbohydrate and lipid metabolism, oxidative stress, energy storage and breakdown (Wang et al., 2008). Therefore, we hypothesized that the M. nipponense hepatopancreas undergoes marked metabolomic changes in response to hypoxia (Liu et al., 2014; Song Q. et al., 2017). Nonetheless, the specific mechanisms by hepatopancreas of prawns respond to hypoxia stress are largely unknown and hypoxia-related metabolomics information remains limited. Thus, the effects of hypoxia and subsequent recovery on M. nipponense hepatopancreas were investigated using a GC-MS-based metabolomics approach. We also compared the activities of metabolic enzymes and electron transport chain-related gene expression level changes induced by hypoxia between M. nipponense and other species. This study provides insight into the metabolic pathways of M. nipponense that are affected by acute hypoxia and reoxygenation.
Materials and Methods
Experimental Prawn
All experimental procedures involving prawn were approved by the institution animal care and use committee of the Chinese Academy of Fishery Sciences. Healthy M. nipponense (wet weight 2.12–3.86 g) were obtained from Dapu experimental base near by Tai Lake, the Freshwater Fisheries Research Center of the Chinese Academy of Fishery Sciences (Wuxi, China). The prawns were acclimated in 12,300-L aerated freshwater tanks for 1 week and fed commercial flake food twice per day. The culture conditions were: 23.5 ± 0.5°C, pH 8.3 ± 0.09, 6.8 ± 0.2 mg/L dissolved oxygen, and < 0.1 mg/L total ammonia-nitrogen. The prawn were raised under the natural photoperiod.
Hypoxia and Recovery Stress
The control group was maintained in normoxic conditions (6.5 ± 0.2 mg O2/L). In the hypoxia groups, severe hypoxic conditions (2.0 ± 0.1 mg O2/L) for 24 h within the treatment tanks were maintained by adding N2 gas until the desired O2 concentrations were reached (Sun et al., 2014); oxygen levels were maintained by adding N2 gas when needed. The hypoxic DO value was chosen based on previous observations of juvenile oriental river prawn. DO and temperature were measured using a water-quality instrument (YSI Inc., Yellow Springs, OH, USA). Six hundred prawns were randomly allocated to 12 tanks (four treatments were conducted in triplicate): hypoxia for 0 h (control), severe hypoxia for 6 h, severe hypoxia for 24 h, or severe hypoxia for 24 h followed by recovery in normoxic conditions for 6 h. The mortality rate was 10% during experiment period. The hepatopancreas samples in each group (in triplicate) were also collected for the biochemical and gene expression assays (n = 9), and the other hepatopancreas samples in each group (in triplicate) were also stored at −80°C until the metabolomics assays were conducted (n = 8). All animal experiments were conducted in accordance with the Guidelines for Experimental Animals of the Ministry of Science and Technology (Chen et al., 2017). All experimental procedures were approved by the Animal Care and Use Committee of the Chinese Academy of Fishery Sciences.
Hepatopancreas Metabolomics Analysis
Samples (0.05 g, n = 8) in each group were extracted with 0.4 mL methanol-chloroform (v:v, 4:1). L-2-chlorophenylalanine (20 μL) was added as an internal standard and then centrifuged (11,000 × g, 15 min, 4°C). The supernatant was then transferred to a 2-ml GC/MS glass vial, and 15 μL from each sample were analyzed for quality control purposes. The hepatopancreas extracts were processed using a method described in a recent study (Li et al., 2017).
GC-time-of-flight (TOF)-MS analysis was conducted using an Agilent 7890 GC system coupled with a Pegasus HT TOF-MS, as previously described (Li et al., 2017). Each sample in the present study was analyzed eight times. Chroma TOF4.3X software (LECO Corporation, USA) and the LECO-Fiehn Rtx5 database were used for raw peak extraction, data baseline filtering and calibration, peak alignment, deconvolution analysis, peak identification, and integration of peak areas (Kind et al., 2009). Metabolomics data have been deposited to the EMBL-EBI MetaboLights database (Haug et al., 2013) with the identifier MTBLS481. The complete dataset can be accessed https://www.ebi.ac.uk/metabolights/MTBLS584.
Analysis of Differential Metabolites
Three-dimensional data including the peak number, sample name, and normalized peak area were processed using the SIMCA14 software package (Umetrics, Umea, Sweden) for principal component analysis (PCA) and orthogonal projections to latent structures-discriminate analysis (OPLS-DA). PCA showed the distribution of the original data. OPLS-DA showed a higher level of group separation and thus resulted in a better understanding of the variables responsible for classification (i.e., the differences between groups). We refined this analysis by obtaining the first principal component of variable importance projection values, with values >1.0 selected as changed metabolites. In step 2, the remaining variables were then assessed using Student's t-test (P < 0.05) (Storey and Tibshirani, 2003; Ren et al., 2015; Song T. et al., 2017). Metabolites and their biological roles and pathways were identified using databases including Chemical Entities of Biological Interest (http://www.ebi.ac.uk/chebi/init.do), the Kyoto Encyclopedia of Genes and Genomes (KEGG) (http://www.genome.jp/kegg/), and National Institute of Standards and Technology (NIST) (http://www.nist.gov/index.html). KEGG pathway analysis of different metabolites was performed using Metabo Analyst 3.0 software (http://www.metaboanalyst.ca/MetaboAnalyst/).
RNA Extraction and Quantitation of Gene Expression
Total RNA was extracted from hepatopancreas (~100 mg) using 1 mL of Trizol reagent following the manufacturer's protocol (TaKaRa, Japan). cDNAs were synthesized from 1 μg total DNA-free RNA using the PrimeScript RT reagent kit (TaKaRa, Japan). Quantitative real time-PCR was performed on a Bio-Rad iCycler iQ5 Real-Time PCR system, and β-actin was used as a reference gene (Sun et al., 2016a). Table 1 shows the primers used. The reaction was amplified with 35 cycles of 94°C for 30 s, 50°C for 30 s, and 72°C for 1 min, which were followed by 10 min of incubation at 72°C as a final extension step (Qiao et al., 2015). Dissociation curve analysis of the amplification products was performed at the end of each PCR reaction. mRNA expression levels were determined using the 2−ΔΔCT method (Livak and Schmittgen, 2001).
Analysis of Enzyme Activity
Hepatopancreas samples were homogenized (w:v, 1:10) in ice-chilled 0.86% saline buffer at 60 Hz for 30 s, and then centrifuged at 3,000 × g for 10 min at 4°C. The supernatant was collected for further analysis. The succinate dehydrogenase (SDH), hexokinase (HK), pyruvate kinase (PK), and lactate dehydrogenase (LDH) activities of each sample were determined using commercial kits (Nanjing Jiancheng Bioengineering Institute, Nanjing, China), including succinate dehydrogenase assay kit (A022, colorimetric method, 50 tubes), hexokinase assay kit (A077-1, ultraviolet colorimetric method, 30 tubes), pyruvate kinase assay kit (A076-1, ultraviolet colorimetric method, 50 tubes), and lactate dehydrogenase assay kit (A020-2, microplate method, 96 tubes).Protein concentration in the samples was determined according to the Bradford method 1976, with bovine serum albumin as the standard.
Statistical Analysis
Data are presented as the mean ± SE values (n = 9). Data were transformed if necessary after evaluating assumptions of normality, equality of variances and outliers, and subjected to one-way analysis of variance (ANOVA) using the software SPSS 19.0 (International Business Machines Corporation, Armonk, NY, USA) for Windows, with post-hoc comparison of means using the Turkey-Kramer HSD test.
Results
Hepatopancreas Metabolomic Profile of M. nipponense in Response to Hypoxia and Reoxygenation
Metabolic profiles of prawn hepatopancreas acquired in present study were shown in Figure S1. PCA analysis of GC-TOF/MS metabolic profiles of hepatopancreas showed in Figure 1, a certain trend in metabolite shift was observed among different groups in PC1. Due to the relatively short period of hypoxia stress compared to the lifespan of a river prawn, the major metabolite pathways might remain generally stable. As a result, we believe that the metabolic shift only covered a small portion of the total metabolome data in this experiment. OPLS-DA was also applied to each sample (hypoxia or reoxygenation), and data were compared with the control group (Figure 2). The classification parameters for the software were as follows: R2Y = 0.988 and Q2Y = 0.502, R2Y = 0.994 and Q2Y = 0.698, and R2Y = 0.996 and Q2Y = 0.777 for 6-h hypoxia, 24-h hypoxia and 6-h reoxygenation, respectively. Seven-fold cross-validation was used to estimate the robustness and predictive ability of our model, and permutation tests were used to further validate the model. The R2 and Q2 intercept values were 0.97, 0.95, 0.94, and −0.34, −0.55, −061, respectively, after 200 permutations for different treatments. The low Q2 intercept values indicate the robustness of the models, the low risk of overfitting and the reliability of the method. The OPLS-DA score plots of the first and second principal components (t [1] P and t [1] O) showed that the prawns under hypoxia for 6 h, hypoxia for 24 h, and hypoxia for 24 h followed by reoxygenation for 6 h were clearly separated from those in control conditions in the direction of t [1] P. Thus, the spectral characteristics of the three experimental groups were markedly different from those of the control group.
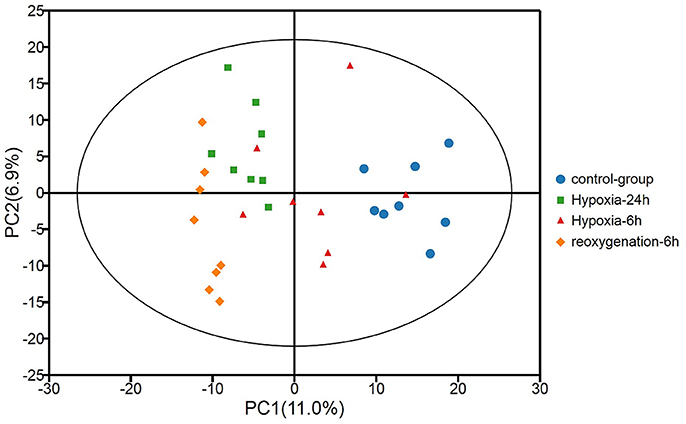
Figure 1. Principal component analysis (PCA) of metabolic profiles of the hepatopancreas of oriental river prawns in the control group, in response to hypoxia for 6 and 24 h, and in response to hypoxia for 24 h followed by reoxygenation for 6 h (eight biological replicates). R2X [1] = 0.111, R2X [2] = 0.179, Ellipse: Hotelling's T2 (95%).
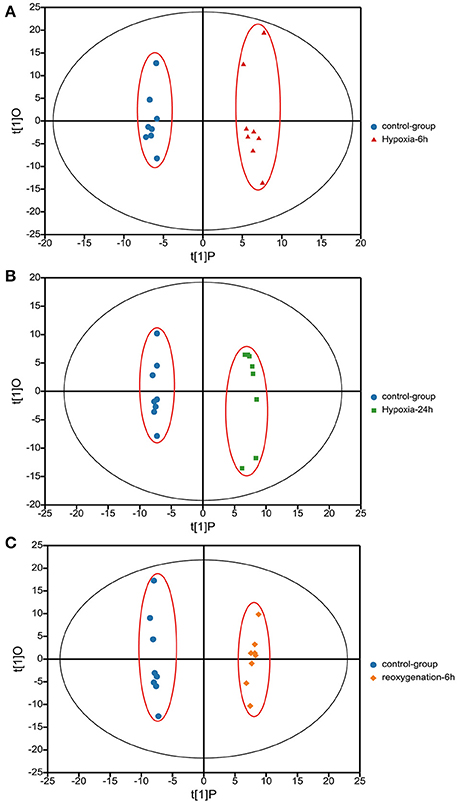
Figure 2. Orthogonal projections to latent structures-discriminate analysis (OPLS-DA) score plots based on the GC-MS spectra of hepatopancreas samples from M. nipponense in response to hypoxia for 6 h relative to the control (A), hypoxia for 24 h relative to the control (B), and hypoxia for 24 h followed by reoxygenation for 6 h relative to the control (C). “O” means “Orthogonal” and “P” means “Predictive” in OPLS-DA. R2X [1] = 0.139, 0.127, 0.094, R2X [2] = 0.125, 0.97, 0.151. Ellipse: Hotelling's T2 (95%).
Table 2 demonstrates that in the hepatopancreas of prawns, as a consequence of 6-h hypoxia, only 13 metabolites were altered (10 were upregulated and three were downregulated). In response to hypoxia for 24 h, the level of 20 metabolites changed significantly in relation to the control (seven were upregulated and 13 were downregulated) (Table 3). The main biochemicals were free amino acids and factors associated with the citrate cycle, glycolysis and redox homeostasis. In response to reoxygenation for 6 h following hypoxia for 24 h, the level of 25 metabolites changed significantly in relation to the control samples (20 were upregulated and five were downregulated) (Table 4). We observed significant changes in energy substrates and cofactors and the biochemicals associated with amino acid metabolism and glutathione synthesis.
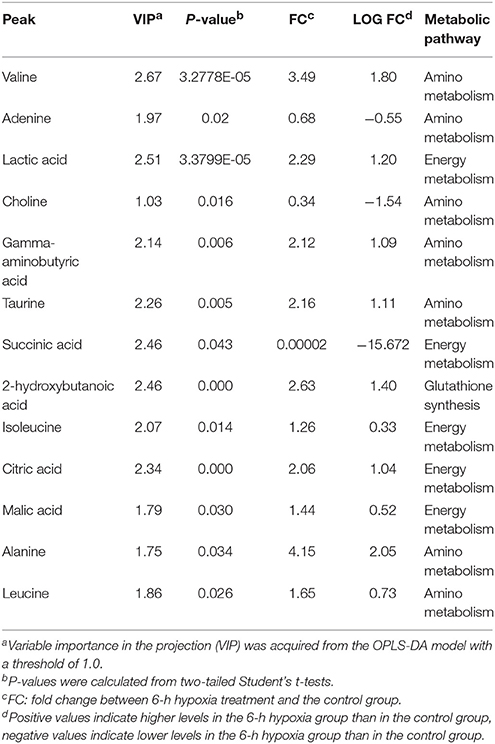
Table 2. Significantly changed metabolites in M. nipponense hepatopancreas between the control group and the 6-h hypoxia group.
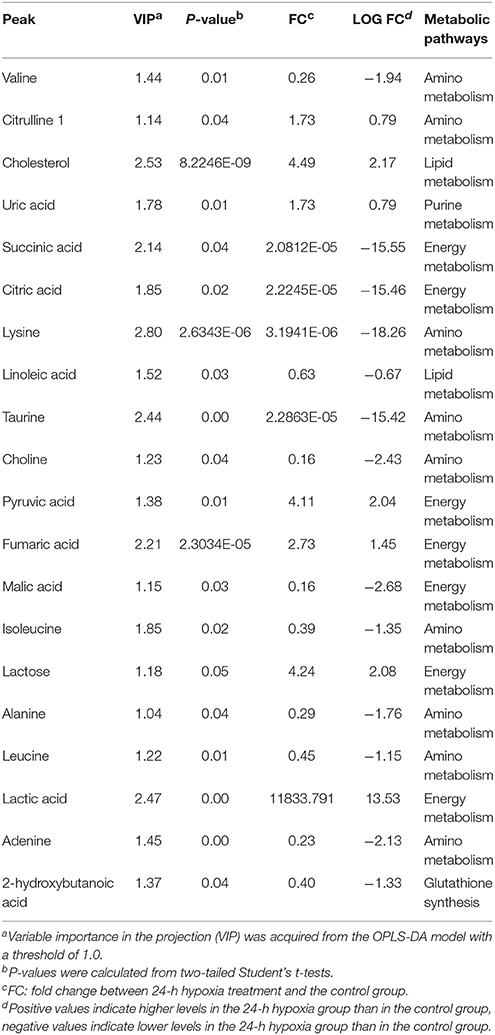
Table 3. Significantly changed metabolites in M. nipponense hepatopancreas between the control group and the 24-h hypoxia group.
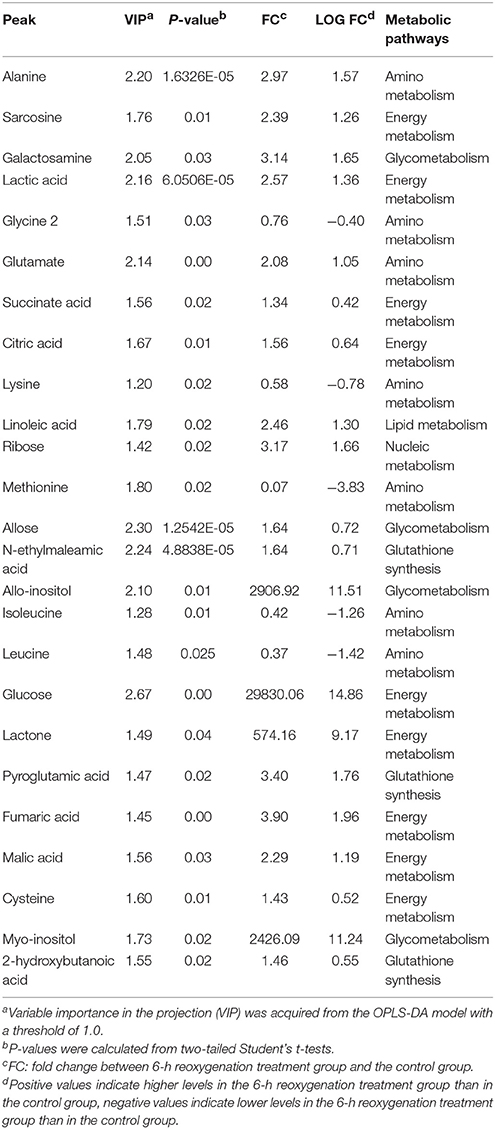
Table 4. Significantly changed metabolites in M. nipponense hepatopancreas between the control group and the 24-h hypoxia followed by 6-h reoxygenation group.
KEGG Pathway Analysis
KEGG pathway analysis was performed using Metabo Analyst 3.0 and differentially affected metabolites. Functional pathway analysis revealed the most relevant pathways affected by hypoxic stress included the citrate cycle (TCA cycle), pyruvate metabolism, glycolysis or gluconeogenesis, propanoate metabolism, valine, leucine, and isoleucine biosynthesis, and purine metabolism (Figures 3A,B). The significantly changed pathways identified from affected metabolites in hepatopancreas of prawns after hypoxia for 24 h followed by reoxygenation for 6 h included the TCA cycle, pyruvate metabolism, cysteine and methionine metabolism, and linoleic acid metabolism (Figure 3C).
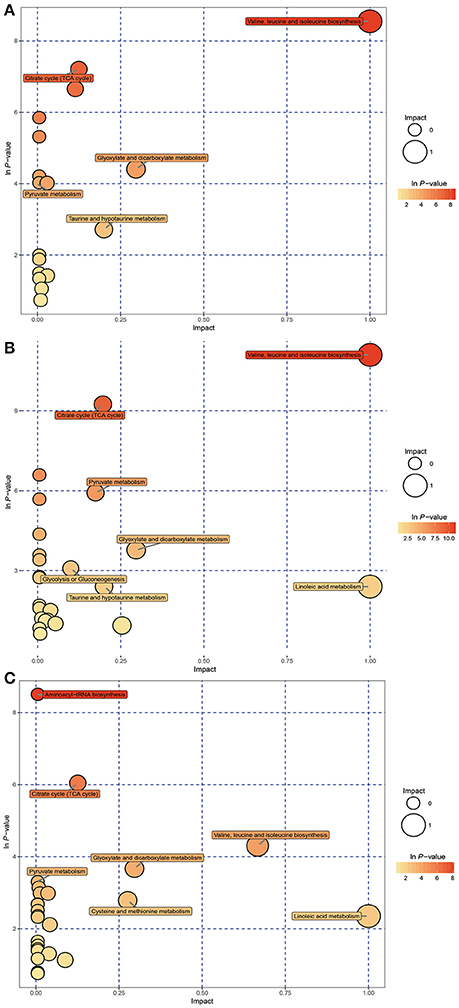
Figure 3. Metabolome view map of significant metabolic pathways characterized in hepatopancreas of prawns in response to hypoxia for 6 h (A), hypoxia for 24 h (B), and hypoxia for 24 h followed by reoxygenation for 6 h (C). This figure illustrates significantly changed pathways based on enrichment and topology analysis. Larger sizes and darker colors represent greater pathway enrichment and higher pathway impact values, respectively.
mRNA Expression Profiles of Aerobic Metabolism-Related Genes
Figure 4 shows the expression levels of cytochrome c oxidase subunit I (COX I) and ATP synthase subunits α and β (ATPα, ATPβ). The expression level of COX I mRNA was significantly lower in the 6- or 24-h hypoxia groups than in the other groups (F = 8.159, P < 0.05). However, after hypoxia followed by reoxygenation for 6 h, the expression level increased to the control levels. The mRNA expression levels of ATPα and ATPβ had no significant change between the control (normoxia) and hypoxia groups, and no significant differences were observed in the expression levels of ATPα and ATPβ between the control (normoxia) and hypoxia–reoxygenation groups.
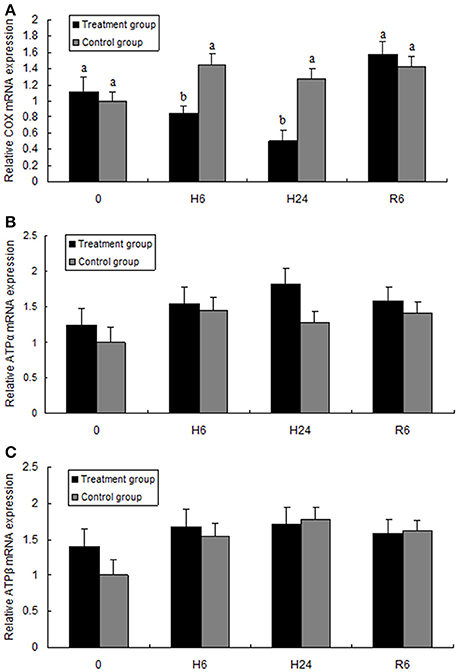
Figure 4. Quantitative real-time PCR analysis of (A) cytochrome c oxidase subunit I (COX I), (B) ATP synthase subunit α (ATPα), and (C) ATP synthase subunit β (ATPβ) mRNA expression in the hepatopancreas of juvenile oriental river prawn after exposure to hypoxia and reoxygenation. H6 = hypoxia for 6 h, H24 = hypoxia for 24 h, and R6 = hypoxia for 24 h followed by reoxygenation for 6 h. Significant differences (P < 0.05) among all treatment groups are indicated with different letters.
Activities of Energy Metabolism-Related Enzymes of M. nipponense in Response to Hypoxia and Reoxygenation
HK, PK, and LDH activities were significantly higher in the hepatopancreas of M. nipponense in the 6- and 24-h hypoxia groups than in the other treatment groups (F = 600.832, P < 0.05; F = 478.105, P < 0.05; F = 741.874, P < 0.05, respectively), but no significant differences were observed in these parameters between the reoxygenation group and the control groups (Figure 5). SDH activity significantly decreased with increase in hypoxia time (F = 76.680, P < 0.05), but SDH activity in the reoxygenated group returned to the levels in the control group (Figure 5).
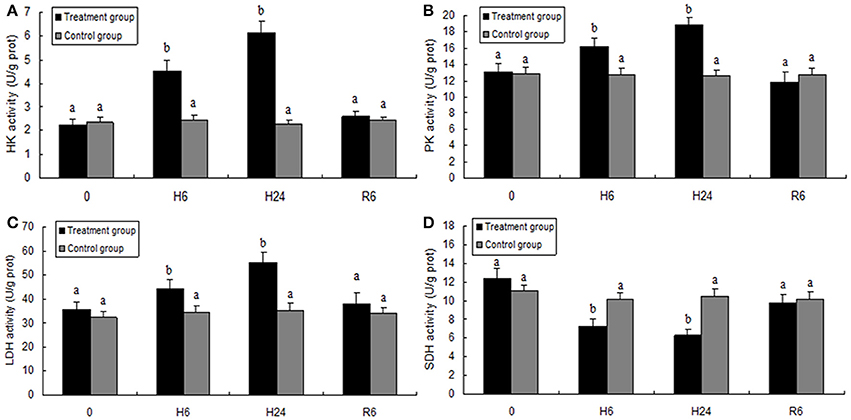
Figure 5. Enzyme activities of hexokinase (HK, A), pyruvate kinase (PK, B), lactate dehydrogenase (LDH, C), and succinate dehydrogenase (SDH, D) in the hepatopancreas of juvenile oriental river prawn after exposure to hypoxia and reoxygenation. H6 = hypoxia for 6 h, H24 = hypoxia for 24 h, and R6 = hypoxia for 24 h followed by reoxygenation for 6 h. Significant differences (P < 0.05) among all treatment groups are indicated with different letters.
Discussion
Crustaceans often exposed to hypoxia show a complex and highly integrated series of metabolic responses to maintain cellular homeostasis, and ATP synthesis/hydrolysis is crucial in the process of hypoxia-induced stress.
Compared with hypoxia sensitive M. nipponense, the survival of penaeid shrimp is not greatly affected by long-term moderate hypoxia, as reported for Pacific whiteleg shrimp Litopenaeus vannamei (Racotta et al., 2002), thus, we compared the mRNA expression levels of electron transport chain-related enzymes between M. nipponense and L. vannamei. First, we consider aerobic metabolism. The mitochondrial FOF1 ATP-synthase complex catalyzes ATP synthesis (Pedersen, 2007) via chemiosmotic coupling to the respiratory chain. We observed no significant changes in ATPα and ATPβ mRNA expression in prawns during hypoxia or subsequent reoxygenation; this is not consistent with previous findings in the L. vannamei (Martinez-Cruz et al., 2011) where mRNA level of ATPβ increased in response to hypoxia and a subsequent decreased when L. vannamei were re-oxygenated. A reasonable explanation is that prawns starts to use anaerobic metabolism during acute hypoxia, so it might not need to synthesize more new ATP-synthase complexes. The respiratory chain complex II (SDH) is the only enzyme of the tricarboxylic acid cycle (TCA) in the inner mitochondrial membrane, and SDH activity reflects the level of aerobic metabolism (Gao et al., 2016). Similarly with previous study in M. nipponense (Guan et al., 2010), the present results showed that significantly lower SDH activity was found in the M. nipponense exposed to hypoxia, indicating a lower level of aerobic metabolism in these animals. Inhibition of the mRNA expression of respiratory chain complex IV (COX), which we observed here in the hypoxic samples (Jimenez-Gutierrez et al., 2013, 2014), can affect the respiratory function of mitochondria, leading to cell hypoxia or death (Reiffienstcin et al., 1992). Finally, an increase in the beta-hydroxybutyric acid concentration was observed in all hypoxic groups; this probably reflects cessation of ketone-body metabolism as the TCA cycle is not functioning during hypoxia (Mimura and Furuya, 1995). Collectively, our data suggest a hypoxia-associated general inhibition of aerobic energy metabolism in M. nipponense.
In the present study, glycolysis-related enzyme activities of M. nipponense (HK, PK, and LDH) were significantly increased by hypoxia for 6 and 24 h compared with the control group, which was similar to the findings in a previous study of the white shrimp Litopenaeus vannamei (Soñanez-Organis et al., 2011, 2012; Cota-Ruiz et al., 2015). These findings suggest that hypoxia results in a shift from aerobic to anaerobic metabolism, and that sufficient ATP can be generated only by upregulating oxygen-independent mechanisms in hypoxic crustacea. Eventually, glucose/glycogen become exhausted and metabolic waste products such as lactate accumulate, as was also shown in our present study. Acute hypoxia caused a significant increase in the lactate concentration in the hepatopancreas extracts. Interestingly, reoxygenation treatment resulted in a higher lactate content in the hepatopancreas compared to the normoxia group; this indicates the presence of a Warburg effect-like response, as has been reported in other crustacean species (Su et al., 2014). In hypoxia, a sufficient and possibly augmented supply of glucose is required because anaerobic glycolysis is increased to obtain sufficient energy.
Levels of branched-chain amino acids including valine, isoleucine, leucine, decreased in hepatopancreas samples under 24-h hypoxia (relative to the normoxic controls), and branched-chain amino acids were still present at a lower level in prawns that were exposed to hypoxia for 24 then reoxygenated for 6 h. These essential amino acids contribute to global regulation of growth and metabolism (Wang et al., 2011). For example, a decreased concentration of isoleucine in the hepatopancreas was observed in a previous study of hypoxia in prawns and was possibly due to difficulty in taking up food because of the hypoxia (Ren et al., 2014), suggesting that hypoxia can suppress feeding behavior of prawns to some extent. Lysine is an essential amino acid for protein synthesis and a ketogenic amino acid (Sauer et al., 2015); the concentration of lysine was significantly decreased in prawns in response to hypoxia compared to the control group, suggesting that protein degradation was likely to be higher in the hypoxia group than in the control group. This could partly explain the slower growth trend of shrimp under hypoxic stress (Duan et al., 2013). The protective effect of taurine during acute hypoxia in mammalian tissues has recently been reported (Michalk et al., 1997). Prawns in the 24-h hypoxia group also had lower taurine and 2-hydroxybutanoic acid levels than the control group prawns; this was correlated with the synthesis of glutathione, a well-known antioxidant. This could explain why in previous studies, L. vannamei under hypoxic group showed higher antioxidant ability than that of the normoxic group (Parrilla-Taylor and Zenteno-Savín, 2011; Li et al., 2016).
Choline is the precursor of the osmolyte betaine, and is a substrate in the choline kinase-catalyzed conversion of ATP to phosphocholine and ADP. Here, the choline and betaine contents decreased in the hepatopancreas after hypoxic stress, suggesting that hypoxia may affect molecular pathways of the methionine cycle (Bertolo and McBreairty, 2013). Similar to the results of the present study, a higher flux toward the methionine cycle after environmental stress was demonstrated for gilthead sea bream Sparus aurata (Richard et al., 2016), the Manila clam Ruditapes philippinarum (Zhang et al., 2017), and rainbow smelt Osmerus mordax (Richards and Short, 2010). Choline and betaine are among several methyl-donors in the methionine cycle. Methionine plays an important role in protecting cells against reactive oxygen species (ROS) by neutralizing free radicals (Alirezaei et al., 2012; Wu et al., 2014). A previous study in our laboratory showed that hypoxia-induced H2O2 and ROS levels (Sun et al., 2017) and apoptosis were increased in hemocytes following hypoxia, presumably due to increased ROS production (Sun et al., 2016b). Taken together, the findings suggest that hypoxia leads to oxidative stress (Chen et al., 2017).
Thus, lower choline and betaine concentrations in M. nipponense in response to hypoxia may explain why M. nipponense is a hypoxia-sensitive prawn. Furthermore, higher pyroglutamic acid and n-ethylmaleamic acid levels were observed in the hepatopancreas of M. nipponense after hypoxia then reoxygenation for 6 h; these molecules are precursors in glutathione synthesis in the hepatopancreas of crustaceans (Niedzwiecka et al., 2011), which supports the hypothesis that antioxidant synthesis in the hepatopancreas occurs in prawns to help alleviate oxidative stress during recovery from hypoxia.
The significantly affected metabolites and pathways in the metabolic response of prawns to hypoxia and reoxygenation are shown in Figures 4, 6. According to the metabolic pathway analysis, a few very important pathways present distinct differences in the control vs. 6-h hypoxia groups and the control vs. 24-h hypoxia groups. For example, the valine, leucine and isoleucine biosynthesis and linoleic acid metabolism pathways are the key different metabolic pathways involved in energy supply. These results further reiterate that hypoxic prawns make use of amino acids and fatty acid metabolism to supply energy, with lower efficiency than aerobic metabolism.
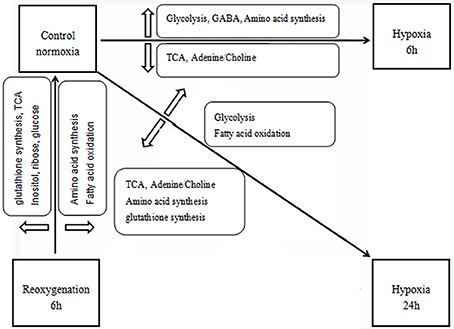
Figure 6. Schematic overview of the metabolic changes in the hepatopancreas of juvenile oriental river prawn in response to hypoxia (6 or 24 h) and subsequent 6-h reoxygenation. Metabolite TCA, tricarboxylic acid cycle; NAA, N-acetylaspartate, GABA, gamma-aminobutyric acid.
In summary, hypoxia has a significant effect on antioxidant defense factors as well as energy metabolism. These findings indicate that M. nipponense can be a sensitive bioindicator of hypoxic stress.
Author Contributions
SS, ZBG, and HF: Conceived and designed the experiments; SS, ZBG, JZ, and ZMG: Carried out the experiments and analyzed the data; SS, HF, and XG: Supervised the project; SS: Wrote the manuscript and all authors reviewed the manuscript.
Conflict of Interest Statement
The authors declare that the research was conducted in the absence of any commercial or financial relationships that could be construed as a potential conflict of interest.
Acknowledgments
This work was supported by the National Natural Science Foundation of China (Grant No. 31672633), the China Central Governmental Research Institutional Basic Special Research Project from Public Welfare Fund (Grant No. 2017JBFM02), the National Science & Technology Supporting Program of the 12th Five-year Plan of China (Grant No. 2012BAD26B04), the Special Fund for Agro-scientific Research in the Public Interest (Grant No. 201303056-6), the Science & Technology Supporting Program of Jiangsu Province (Grant No. BE2012334), and the Three New Projects of Jiangsu Province (Grant No. D2013-6).
Supplementary Material
The Supplementary Material for this article can be found online at: https://www.frontiersin.org/articles/10.3389/fphys.2018.00076/full#supplementary-material
Figure S1. Representative gas chromatography-mass spectrometry (GC-MS) total ion chromatograms from oriental river prawn (M. nipponense) hepatopancreas samples obtained from the control group (black), 6-h hypoxia treatment group (red), 24-h hypoxia group (blue), and the 24-h hypoxia followed by 6-h reoxygenation group (green).
References
Alirezaei, M., Reza, G. H., Reza, R. V., and Hajibemani, A. (2012). Betaine: a promising antioxidant agent for enhancement of broiler meat quality. Br. Poult. Sci. 53, 699–707. doi: 10.1080/00071668.2012.728283
Bell, G. W., and Eggleston, D. B. (2005). Species-specific avoidance responses by blue crabs and fish to chronic and episodic hypoxia. Mar. Biol. 146, 761–770. doi: 10.1007/s00227-004-1483-7
Bertolo, R. F., and McBreairty, L. E. (2013). The nutritional burden of methylation reactions. Curr. Opin. Clin. Nutr. Metab. Care. 16, 102–108. doi: 10.1097/MCO.0b013e32835ad2ee
Bradford, M. M. (1976). A rapid and sensitive method for the quantification of microgram quantities of protein utilizing the principle of protein-dye binding. Anal. Biochem. 72, 248–254. doi: 10.1016/0003-2697(76)90527-3
Brown-Peterson, N. J., Manning, C., Patel, V., Denslow, N., and Brouwer, M. (2008). Effects of cyclic hypoxia on gene expression and reproduction in a grass shrimp, Palaemonetes pugio. Biol. Bull. 214, 6–16. doi: 10.2307/25066655
Brown-Peterson, N. J., Manning, C. S., Denslow, N. D., and Brouwer, M. (2011). Impacts of cyclic hypoxia on reproductive and gene expression patterns in the grass shrimp: field versus laboratory comparison. Aquat. Sci. 73, 127–141. doi: 10.1007/s00027-010-0166-3
Chen, N., Wu, M., Tang, G. P., Wang, H. J., Huang, C. X., and Wu, X. J. (2017). Effects of acute hypoxia and reoxygenation on physiological and immune responses and redox balance of Wuchang bream (Megalobrama amblycephala Yih, 1955). Front. Physiol. 8:375. doi: 10.3389/fphys.2017.00375
Cota-Ruiz, K., Peregrino-Uriarte, A. B., Felix-Portillo, M., Martínez-Quintana, J. A., and Yepiz-Plascencia, G. (2015). Expression of fructose 1,6-bisphosphatase and phosphofructokinase is induced in hepatopancreas of the white shrimp Litopenaeus vannamei by hypoxia. Mar. Environ. Res. 106, 1–9. doi: 10.1016/j.marenvres.2015.02.003
Craig, J. K., Crowder, L. B., and Henwood, T. A. (2005). Spatial distribution of brown shrimp (Farfantepenaeus aztecus) on the northwestern Gulf of Mexico shelf: effects of abundance and hypoxia. Can. J. Fish. Aquat. Sci. 62, 1295–1308. doi: 10.1139/f05-036
Duan, Y., Zhang, X. M., and Zhang, Z. X. (2013). The effect of dissolve oxygen concentration on the growth and digestive enzyme activity of whiteleg shrimp Litopenaeus vannamei. J. Ocean. U. China 43, 9–14.
Gao, X. B., Zhang, M., Li, X. N., Shi, C., Song, C. B., and Liu, Y. (2016). Effects of LED light quality on the growth, metabolism, and energy budgets of Haliotis discus discus. Aquaculture 453, 31–39. doi: 10.1016/j.aquaculture.2015.11.033
Guan, Y. Q., Li, L. I., Wang, H. C., and Wang, Z. L. (2010). Effects of hypoxia on respiratory metabolism and antioxidant capability of Macrobrachium nipponense. J. Hebei. Uni. 30, 301–306.
Hallman, T. M., Rojas-Vargas, A. C., Jones, D. R., and Richards, J. G. (2008). Differential recovery from exercise and hypoxia exposure measured using (31)P- and (1)H-NMR in white muscle of the common carp Cyprinus carpio. J. Exp. Biol. 211, 3237–3248. doi: 10.1242/jeb.019257
Haug, K., Salek, R. M., Conesa, P., Hastings, J., De Matos, P., Rijnbeek, M., et al. (2013). MetaboLights–an open-access general-purpose repository for metabolomics studies and associated meta-data. Nucleic Acids Res. 41, D781–D786. doi: 10.1093/nar/gks1004
Hines, A., Oldiran, G. S., Bignell, J. P., Stentiford, G. D., and Viant, M. (2007). Direct sampling of organisms from the field and knowledge of their phenotype: key recommendations for environmental metabolomics. Environ. Sci. Technol. 41, 3375–3381. doi: 10.1021/es062745w
Jiang, H., Li, F. H., Xie, Y. S., Huang, B. X., Zhang, J. K., Zhang, J. Q., et al. (2009). Comparative proteomic profiles of the hepatopancreas in Fenneropenaeus chinensis response to hypoxic stress. Proteomics 9, 3353–3367. doi: 10.1002/pmic.200800518
Jimenez-Gutierrez, L. R., Hernandez-Lopez, J., Islas-Osuna, M. A., and Muhlia-Almazan, A. (2013). Three nucleus-encoded subunits of mitochondrial cytochrome c oxidase of the whiteleg shrimp Litopenaeus vannamei: cDNA characterization, phylogeny and mRNA expression during hypoxia and reoxygenation. Comp. Biochem. Physiol. B Biochem. Mol. Biol. 166, 30–39. doi: 10.1016/j.cbpb.2013.06.008
Jimenez-Gutierrez, L. R., Uribe-Carvajal, S., Sanchez-Paz, A., Chimeo, C., and Muhlia-Almazan, A. (2014). The cytochrome c oxidase and its mitochondrial function in the whiteleg shrimp Litopenaeus vannamei during hypoxia. J. Bioenerg. Biomembr. 46, 189–196. doi: 10.1007/s10863-013-9537-5
Kind, T., Wohlgemuth, G., Lee, D. Y., Lu, Y., Palazoglu, M., Shahbaz, S., et al. (2009). FiehnLib: mass spectral and retention index libraries for metabolomics basedon quadrupole and time-of-flight gas chromatography/mass spectrometry. Anal. Chem. 81, 10038–10048. doi: 10.1021/ac9019522
Kniffin, C. D., Burnett, L. E., and Burnett, K. G. (2014). Recovery from hypoxia and hypercapnic hypoxia: impacts on the transcription of key antioxidants in the shrimp Litopenaeus vannamei. Comp. Biochem. Physiol. B Biochem. Mol. Biol. 170, 43–49. doi: 10.1016/j.cbpb.2014.01.006
Lardon, I., Eyckmans, M., Vu, T. N., Laukens, K., Boeck, G. D., Dommisse, R., et al. (2013a). 1H-NMR study of the metabolome of a moderately hypoxia tolerant fish, the common carp (Cyprinus carpio). Metabolomics 9, 1216–1227. doi: 10.1007/s11306-013-0540-y
Lardon, I., Nilsson, G. E., Stecyk, J. A. W., Vu, N. T., Laukens, K., Dommisse, R., et al. (2013b). 1H-NMR study of the metabolome of an exceptionally anoxia tolerant vertebrate, the crucian carp (Carassius carassius). Metabolomics 9, 311–323. doi: 10.1007/s11306-012-0448-y
Li, T. D., and Brouwer, M. (2009). Gene expression profile of grass shrimp Palaemonetes pugio exposed to chronic hypoxia. Comp. Biochem. Physiol. Part D Genomics Proteomics 4, 196–208. doi: 10.1016/j.cbd.2009.03.004
Li, T. D., and Brouwer, M. (2013). Gene expression profile of hepatopancreas from grass shrimp Palaemonetes pugio exposed to cyclic hypoxia. Comp. Biochem. Physiol. Part D Genomics Proteomics 8, 1–10. doi: 10.1016/j.cbd.2012.10.003
Li, T. Y., Li, E. C., Suo, Y. T., Xu, Z. X., Jia, Y. Y., Qin, J. G., et al. (2017). Energy metabolism and metabolomics response of Pacific whiteshrimp Litopenaeus vannamei to sulfide toxicity. Aquat. Toxicol. 183, 28–37. doi: 10.1016/j.aquatox.2016.12.010
Li, Y., Wei, L., Cao, J., Qiu, L., Jiang, X., Li, P., et al. (2016). Oxidative stress, DNA damage and antioxidant enzyme activities in the pacific white shrimp (Litopenaeus vannamei) when exposed to hypoxia and reoxygenation. Chemosphere 144, 234–240. doi: 10.1016/j.chemosphere.2015.08.051
Liu, X., Sun, H., Wang, Y., Ma, M., and Zhang, Y. (2014). Gender-specific metabolic responses in hepatopancreas of mussel Mytilus galloprovincialis challenged by Vibrio harveyi. Fish. Shellfish. Immunol. 40, 407–413. doi: 10.1016/j.fsi.2014.08.002
Livak, K. J., and Schmittgen, T. D. (2001). Analysis of relative gene expression data using realtime quantitative PCR and the 2−ΔΔCT Method. Methods 5, 402–408. doi: 10.1006/meth.2001.1262
Luo, B., Groenke, K., Takors, R., Wandrey, C., and Oldiges, M. (2007). Simultaneous determination of multiple intracellular metabolites in glycolysis, pentose phosphate pathway and tricarboxylic acid cycle by liquid chromatography-mass spectrometry. J. Chromatogr. A 1147, 153–164. doi: 10.1016/j.chroma.2007.02.034
Ma, K., Feng, J., Lin, J., and Li, J. (2011). The complete mitochondrial genome of Macrobrachium nipponense. Gene 487, 160–165. doi: 10.1016/j.gene.2011.07.017
Mangum, C. P. (1997). Adaptation of the oxygen transport system to hypoxia in the blue crab, Callinectes sapidus. Am. Zool. 37, 604–611. doi: 10.1093/icb/37.6.604
Mangum, C. P., and Rainer, J. S. (1988). The relationship between subunit composition and O2 binding of blue crab hemocyanin. Biol. Bull. 174, 77–82. doi: 10.2307/1541761
Martinez-Cruz, O., Garcia-Carreño, F., Robles-Romo, A., Varela-Romero, A., and Muhlia-Almazan, A. (2011). Catalytic subunits atpα and atpβ from the Pacific white shrimp Litopenaeus vannamei FOF1 ATP-synthase complex: cDNA sequences, phylogenies, and mRNA quantification during hypoxia. J. Bioenerg. Biomembr. 43, 119–133. doi: 10.1007/s10863-011-9340-0
Michalk, D. V., Wingenfeld, P., and Licht, C. (1997). Protection against cell damage due to hypoxia and reoxygenation: the role of taurine and the involved mechanisms. Amino. Acids. 13, 337–346. doi: 10.1007/BF01372597
Mimura, Y., and Furuya, K. (1995). Mechanisms of adaptation to hypoxia in energy metabolism in rats. J. Am. Coll. Surg. 181, 437–443.
Niedzwiecka, N., Mika, A., Białk-Bielinska, A., Stepnowski, P., and Skorkowski, E. F. (2011). Effect of cadmium and glutathione on malic enzyme activity in brown shrimps (Crangon crangon) from the Gulf of Gda'nsk. Oceanologia 53, 793–805. doi: 10.5697/oc.53-3.793
Nudi, A. H., Angela, W., and Scofield, D. E. (2008). PAH metabolites in urine of crab ucides cordatus by HPLC/fluorescence detection. Mar. Environ. Res. 66, 187–189.
Ocampo, L., Villarreal, H., Vargas, M., Portillo, G., and Magallon, F. (2000). Effects of dissolved oxygen and temperature on growth, survival and body composition of juvenile Farfantepenaeus californiensis (Holmes). Aquac. Res. 31, 167–171. doi: 10.1046/j.1365-2109.2000.00405.x
Parrilla-Taylor, D. P., and Zenteno-Savín, T. (2011). Antioxidant enzyme activities in Pacific white shrimp (Litopenaeus vannamei) in response to environmental hypoxia and reoxygenation. Aquaculture 318, 379–383. doi: 10.1016/j.aquaculture.2011.05.015
Pedersen, P. (2007). Transport ATPases into the year 2008: a brief overview related to types, structures, functions and roles in health and diseas. J. Bioenerg. Biomembr. 39, 349–355. doi: 10.1007/s10863-007-9123-9
Qiao, H., Xiong, Y. W., Zhang, W. Y., Fu, H. G., Jiang, S. F., Sun, S. M., et al. (2015). Characterization, expression, and function analysis of gonad-inhibiting hormone in Oriental River prawn, Macrobrachium nipponense and its induced expression by temperature. Comp. Biochem. Physiol. A Comp. Physiol. 185, 1–8. doi: 10.1016/j.cbpa.2015.03.005
Qiu, R. J., Cheng, Y. X., Huang, X. X., Wu, X. G., Yang, X. Z., and Tong, R. (2011). Effect of hypoxia on immunological, physiological response, and hepatopancreatic metabolism of juvenile Chinese mitten crab Eriocheir sinensis. Aquac. Int. 19, 283–299. doi: 10.1007/s10499-010-9390-z
Racotta, I. S., Palacios, E., and Mendez, L. (2002). Metabolic responses to short and long-term exposure to hypoxia in white shrimp (Penaeus vannamei). Mar. Fresh. Behav. Physiol. 35, 269–275. doi: 10.1080/1023624021000019333
Reiffienstcin, R. J., Hullbert, W. C., and Roth, S. H. (1992). Toxicity of hydrogen sulfied. Annu. Rev. Pharmacol. Toxicol. 32, 109–134. doi: 10.1146/annurev.pa.32.040192.000545
Ren, W. K., Yin, J., Gao, W., Chen, S., Duan, J. L., Liu, G., et al. (2015). Metabolomics study of metabolic variations in enterotoxigenic Escherichia coli-infected piglets. RSC Adv. 5:59550–59555. doi: 10.1039/C5RA09513A
Ren, W., Yin, J., Duan, J., Liu, G., Zhu, X., Chen, S., et al. (2014). Mouse jejunum innate immune responses altered by enterotoxigenic Escherichia coli (ETEC) infection. Microbes Infect. 16, 954–961. doi: 10.1016/j.micinf.2014.09.005
Richard, N., Silva, T. S., Tune, W., Schrama, D., Dias, J. P., Rodrigues, P. M., et al. (2016). Nutritional mitigation of winter thermal stress in gilthead seabream: associated metabolic pathways and potential indicators of nutritional state. J. Proteome. 142, 1–14. doi: 10.1016/j.jprot.2016.04.037
Richards, R. C., and Short, C. E. (2010). Seasonal changes in hepatic gene expression reveal modulation of multiple processes in rainbow smelt (Osmerus mordax). Mar. Biotechnol. 12, 650–663. doi: 10.1007/s10126-009-9252-8
Ruan, Z., Lv, Y. F., Fu, X. F., He, Q. H., Deng, Z. Y., Liu, W. Q., et al. (2013). Metabolomic analysis of amino acid metabolism in colitic rats supplemented with lactosucrose. Amino. Acids. 45, 877–887. doi: 10.1007/s00726-013-1535-8
Sauer, S. W., Opp, S., Komatsuzaki, S., Blank, A. E., Mittelbronn, M., Burgard, P., et al. (2015). Multifactorial modulation of susceptibility to llysine in an animal model of glutaric aciduria type I. Biochim. Biophys. Acta Mol. Basis Dis. 1852, 768–777. doi: 10.1016/j.bbadis.2014.12.022
Shao, Y., Li, C. H., Chen, X. C., Zhang, P. J., Li, Y., Li, T. W., et al. (2015). Metabolomic responses of sea cucumber Apostichopus japonicus to thermal stresses. Aquaculture 435, 390–397. doi: 10.1016/j.aquaculture.2014.10.023
Soñanez-Organis, J. G., Peregrino-Uriarte, A. B., Sotelo-Mundo, R. R., Forman, H. J., and Yepiz-Plascencia, G. (2011). Hexokinase from the white shrimp Litopenaeus vannamei: cDNA sequence, structural protein model and regulation via HIF-1 in response to hypoxia. Comp. Biochem. Physiol. B Biochem. Mol. Biol. 158, 242–249. doi: 10.1016/j.cbpb.2010.12.006
Soñanez-Organis, J. G., Rodriguez-Armenta, M., Leal-Rubio, B., Peregrino-Uriarte, A. B., and Yepiz-Plascencia, G. (2012). Alternative splicing generates two lactate dehydrogenase subunits differentially expressed during hypoxia via HIF-1 in the shrimp Litopenaeus vannamei. Biochimie 94, 1250–1260. doi: 10.1016/j.biochi.2012.02.015
Song, Q., Zhou, H., Han, Q., and Diao, X. (2017). Toxic responses of Perna viridis hepatopancreas exposed to DDT, benzo(a)pyrene and their mixture uncovered by iTRAQ-based proteomics and NMR-based metabolomics. Aquat. Toxicol. 192, 48–57. doi: 10.1016/j.aquatox.2017.09.010
Song, T., Xu, H., Sun, N., Jiang, L., Tian, P., Yong, Y., et al. (2017). Metabolomic analysis of Alfalfa (Medicago sativa L.) root-symbiotic rhizobia responses under alkali stress. Front. Plant Sci. 8:1208. doi: 10.3389/fpls.2017.01208
Storey, J. D., and Tibshirani, R. (2003). Statistical significance for genomewide studies. Proc. Natl. Acad. Sci. U.S.A. 100, 9440–9445. doi: 10.1073/pnas.1530509100
Su, M. A., Huang, Y. T., Chen, I. T., Lee, D. Y., Hsieh, Y. C., Li, C. Y., et al. (2014). An invertebrate Warburg effect: a shrimp virus achieves successful replication by altering the host metabolome via the PI3K-Akt-mTOR pathway. PLoS Pathog 10:e1004196. doi: 10.1371/journal.ppat.1004196
Sun, S. M., Xuan, F. J., Fu, H. T., Ge, X. P., Zhu, J., Qiao, H., et al. (2016a). Molecular characterization and mRNA expression of hypoxia inducible factor-1 and cognate inhibiting factor in Macrobrachium nipponense in response to hypoxia. Comp. Biochem. Physiol. B Biochem. Mol. Biol. 196–197, 48–56. doi: 10.1016/j.cbpb.2016.02.002
Sun, S. M., Xuan, F. J., Fu, H. T., Ge, X. P., Zhu, J., Qiao, H., et al. (2016b). Comparative proteomic study of the response to hypoxia in themuscle of oriental river prawn (Macrobrachium nipponense). J. Proteomics 138, 115–123. doi: 10.1016/j.jprot.2016.02.023
Sun, S. M., Xuan, F. J., Fu, H. T., Zhu, J., Ge, X. P., and Gu, Z. M. (2015). Transciptomic and histological analysis of hepatopancreas, muscle and gill tissues of oriental river prawn (Macrobrachium nipponense) in response to chronic hypoxia. BMC Genomics 16:491. doi: 10.1186/s12864-015-1701-3
Sun, S. M., Xuan, F. J., Fu, H. T., Zhu, J., Ge, X. P., and Wu, X. G. (2017). Molecular cloning, characterization and expression analysis of caspase-3 from the oriental river prawn, Macrobrachium nipponense when exposed to acute hypoxia and reoxygenation. Fish. Shellfish. Immunol. 62, 291–302. doi: 10.1016/j.fsi.2017.01.045
Sun, S. M., Xuan, F. J., Ge, X. P., Fu, H. T., Zhu, J., and Zhang, S. Y. (2014). Identification of differentially expressed genes in hepatopancreas of oriental river prawn, Macrobrachium nipponense exposed to environmental hypoxia. Gene 534, 298–306. doi: 10.1016/j.gene.2013.10.036
Tuffnail, W., Mills, G. A., Cary, P., and Greenwood, R. (2009). An environmental 1H NMR metabolomic study of the exposure of the marine mussel Mytilus edulis to atrazine, lindane, hypoxia and starvation. Metabolomics 5, 33–43. doi: 10.1007/s11306-008-0143-1
Wang, L., Yan, B., Liu, N., Li, Y., and Wang, Q. (2008). Effects of cadmium on glutathione synthesis in hepatopancreas of freshwater crab, Sinopotamon yangtsekiense. Chemosphere 74, 51–56. doi: 10.1016/j.chemosphere.2008.09.025
Wang, Y., Haipeng, S., Lu, G., Ren, S., and Chen, J. (2011). Catabolism of branched-chain amino acids in heart failure: insights from genetic models. Pediatr. Cardiol. 32, 305–310. doi: 10.1007/s00246-010-9856-9
Wu, P., Jiang, W. D., Liu, Y., Chen, G. F., Jiang, J., Li, S. H., et al. (2014). Effect of choline on antioxidant defenses and gene expressions of Nrf2 signaling molecule in the spleen and head kidney of juvenile Jian carp (Cyprinus carpio var. Jian). Fish. Shellfish. Immunol. 38, 374–382. doi: 10.1016/j.fsi.2014.03.032
Wu, R. S. (2002). Hypoxia: from molecular responses to ecosystem responses. Mar. Pollut. Bull. 45, 35–45. doi: 10.1016/S0025-326X(02)00061-9
Keywords: Macrobrachium nipponense, metabolomics, hypoxia, hepatopancreas, oxidative stress
Citation: Sun S, Guo Z, Fu H, Ge X, Zhu J and Gu Z (2018) Based on the Metabolomic Approach the Energy Metabolism Responses of Oriental River Prawn Macrobrachium nipponense Hepatopancreas to Acute Hypoxia and Reoxygenation. Front. Physiol. 9:76. doi: 10.3389/fphys.2018.00076
Received: 18 October 2017; Accepted: 22 January 2018;
Published: 09 April 2018.
Edited by:
Youji Wang, Shanghai Ocean University, ChinaReviewed by:
Adriana Mulhia, Center for Research in Food and Development (CIAD), MexicoKatja Anttila, University of Turku, Finland
Hailong Zhou, Hainan University, China
Huanling Wang, Huazhong Agricultural University, China
Copyright © 2018 Sun, Guo, Fu, Ge, Zhu and Gu. This is an open-access article distributed under the terms of the Creative Commons Attribution License (CC BY). The use, distribution or reproduction in other forums is permitted, provided the original author(s) and the copyright owner are credited and that the original publication in this journal is cited, in accordance with accepted academic practice. No use, distribution or reproduction is permitted which does not comply with these terms.
*Correspondence: Hongtuo Fu, ZnVodEBmZnJjLmNu
†These authors have contributed equally to this work.