- 1Division of Heart & Lungs, Department of Medical Physiology, University Medical Center Utrecht, Utrecht, Netherlands
- 2Nanion Technologies, Munich, Germany
An important aspect of the Comprehensive In Vitro Proarrhythmia Assay (CiPA) proposal is the use of human stem cell-derived cardiomyocytes and the confirmation of their predictive power in drug safety assays. The benefits of this cell source are clear; drugs can be tested in vitro on human cardiomyocytes, with patient-specific genotypes if needed, and differentiation efficiencies are generally excellent, resulting in a virtually limitless supply of cardiomyocytes. There are, however, several challenges that will have to be surmounted before successful establishment of hSC-CMs as an all-round predictive model for drug safety assays. An important factor is the relative electrophysiological immaturity of hSC-CMs, which limits arrhythmic responses to unsafe drugs that are pro-arrhythmic in humans. Potentially, immaturity may be improved functionally by creation of hybrid models, in which the dynamic clamp technique joins simulations of lacking cardiac ion channels (e.g., IK1) with hSC-CMs in real-time during patch clamp experiments. This approach has been used successfully in manual patch clamp experiments, but throughput is low. In this study, we combined dynamic clamp with automated patch clamp of iPSC-CMs in current clamp mode, and demonstrate that IK1 conductance can be added to iPSC-CMs on an automated patch clamp platform, resulting in an improved electrophysiological maturity.
Introduction
The Comprehensive In Vitro Proarrhythmia Assay (CiPA) initiative aims to find new means of predicting the proarrhythmic risk of newly developed drugs (Gintant et al., 2016), which do not rely exclusively on hERG block, and not on QT prolongation at all. Key aspects are to include results of computer simulations of drug effects on heart rhythm and in vitro assays using human stem cell-derived cardiomyocytes (hSC-CMs).
Characterization of the hSC-CM electrophysiological phenotype has so far shown that these CM express most, but not all cardiac ion channels, which has implications for their use in safety pharmacological assays (Jonsson et al., 2012; van den Heuvel et al., 2014). Importantly, the inward rectifying potassium current, IK1 (de Boer et al., 2010), that is highly expressed in adult CMs is not, or hardly, expressed by hSC-CMs (Doss et al., 2012; Goversen et al., 2017), while the pacemaker current If is expressed consistently. As a result, hSC-CMs display a pacemaker-like phenotype, with a depolarized and unstable resting membrane potential, resulting in spontaneous triggering of action potentials. This is an important issue, as the action potential waveform affects the activity and availability of many cardiac ion channels, as these are voltage-sensitive and rely on a negative resting membrane potential between beats to recover from inactivation after an action potential. For example, in hSC-CMs, sodium channel availability during the action potential is minimal, even though the channels are expressed in sufficient levels. As a consequence, we found in a previous study that, except for drugs blocking the hERG channel, none of the tested drugs that are known to be proarrhythmic in adult cardiomyocytes triggered arrhythmias (early-after-depolarizations) in hSC-CMs (Jonsson et al., 2012). More recent studies have found that iPSC-CMs are not sufficiently mature to detect risks associated with inhibition of the late sodium current (Blinova et al., 2017), or peak sodium current (Ando et al., 2017). Interestingly, adenovirus mediated overexpression of IK1 channels in iPSC-CM was demonstrated to improve drug responses (Li et al., 2017).
Several approaches are being adopted to improve the electrophysiological phenotype, for instance overexpression of the IK1 channel, which has shown promising results (Vaidyanathan et al., 2016). Another, more controllable approach is to use dynamic clamp to add simulated IK1 channels to hSC-CMs (Wilders, 2006; Ortega et al., 2017). The essence of dynamic clamp is that a hybrid model is created by connecting a real cell with a computer simulation of (parts of) a cell. For this to work, one needs a computer simulation that is running in real-time—simultaneously with the experiment on the real cell—so there is an instantaneous interaction between the real cell and the simulation. This works well, and has been described by several groups that applied it in manual patch clamp experiments (Bett et al., 2013; Meijer van Putten et al., 2015). Addition of simulated or overexpressed IK1 channels results in a stable, more negative resting membrane potential, increased action potential amplitude, and upstroke velocity, thereby bringing the action potential waveform much closer to that of adult human ventricular cardiomyocytes. The expectation is that this approach will result in a more reliable prediction of drug effects, but this hypothesis has yet to be studied systematically (Goversen et al., 2017).
When considering the use of dynamic clamp in safety pharmacology, the low throughput and complex nature of manual patch clamp in combination with dynamic clamp is problematic. Additionally, as noted in the literature (Meijer van Putten et al., 2015), current implementations require the simultaneous use of two computers that both require user interaction during the experiment, which is not very practical. In this study, we have developed a remote-controlled dynamic clamp system with the purpose to couple and integrate it with automated patch clamp devices, in order to increase throughput and develop new predictive assays using hSC-CMs that are in line with the aims of the CiPA initiative. In this study, we demonstrate its application by creating hybrid human cardiomyocyte models by the addition of virtual IK1 current to single, suspended human iPSC-CMs, and recording action potentials on an automated patch clamp device.
Materials and Methods
iPSC-CM Culture and Dissociation
Differentiated iPSC-derived cardiomyocytes (Cor.4U, kindly provided by Axiogenesis AG, Germany and Cellartis Cardiomyocytes, kindly provided by Takara Bio Europe AB, Sweden) were cultured according to the suppliers' instructions. The cells were dissociated by incubating them for 15–30 min in TrypLE (Gibco) until detached from the surface of the culture flask, and then kept at 4°C for 30 min before pipetting them to individualize the cells.
Automated Patch Clamp Electrophysiology
Recordings from single iPSC-CMs were done using a Nanion Patchliner automated patch clamp device at 20°C and standard medium resistance NPC-16 chips. After catching an iPSC-CM, obtaining a gigaseal and breaking into whole cell configuration, several experiments were performed.
From a holding potential of −100 mV, current-voltage recordings were made using voltage steps from −80 to 40 mV for 20 ms increasing in 10 mV steps at 2 s intervals (sodium ion currents), and from −40 to 40 mV for 200 ms increasing in 10 mV steps at 5 s intervals (calcium ion currents), with a 100 ms pre-pulse to −40 mV to inactivate sodium ion currents. IK1 current were recorded from a holding potential of −40 mV, with voltage steps from −120 to +30 mV for 1,200 ms increasing in 10 mV steps. IK1 was blocked by adding 10 μM Ba2+, from these recordings we calculated the Ba2+-sensitive steady-state current and report these in I-V diagrams.
Next, the recording mode was switched to current clamp and the effect of adding simulated IK1 conductance to the iPSC-CM using dynamic clamp was tested. To this end, a current stimulus was optimized for each cell individually to reliably induce action potentials (APs) at a rate of 0.5 Hz. The stimulus was 1 ms long and ranged from 0.6 to 3 nA. The same stimulus was used to trigger APs while exposing the cells to increasing simulated IK1 conductance. If the conductance was set too low, no effect on the AP was detectable, if set too high, no AP could be induced. The simulated IK1 conductance range varied considerably between individual cells, 200 to 2,000 pS/pF were used across cells.
All experiments were done using extracellular solution containing (in mmol/L) 140 NaCl, 10 HEPES, 5 Glucose, 4 KCl, 2 CaCl2, 1 MgCl2, pH 7.4 (NaOH), 298 mOsm, and intracellular solution containing 110 KF, 10 KCl, 10 NaCl, 10 HEPES, 10 EGTA, pH7.2 (KOH), 285 mOsm. In some experiments, potassium salts were replaced by cesium salts to expose calcium ion currents otherwise obscured by potassium ion currents. Since potassium currents turned out to be negligible, no difference referring to (real) potassium conductance was observed between those recordings.
Dynamic Clamp System
Experiments were done using a dynamic clamp system developed in house at UMC Utrecht, Utrecht, The Netherlands. The system runs on the Labview RT operating system, and simulates IK1 current in response to membrane potential measured from the iPSC-CM. Real-time simulation of the IK1 current was done using the model by Ishihara et al. (2009), and simulations were done at 20 kHz, on four independent channels, allowing simultaneous dynamic clamp of four iPSC-CMs. We have included as supplementary information the used IK1 model (Supplementary Data Sheet 1), a diagram explaining our dynamic clamp implementation (Supplementary Figure 1) and a flowchart describing the various steps taken during the dynamic clamp experiments (Supplementary Figure 2).
The dynamic clamp system is coupled to the HEKA EPC-10 Quattro amplifier that is part of the Patchliner setup (see Figure 1). The connections couple the membrane potential of each iPSC-CM with each IK1 simulation channel and return the computed IK1 current to the iPSC-CM via the external stimulus input of the EPC-10 amplifier. Remote control is achieved via additional couplings that allow bi-directional communication between the dynamic clamp system and the Patchliner setup. For this we use the standard digital input and output channels of the HEKA EPC-10 Quattro amplifier. These are used to continuously read dynamic clamp system status and set model parameters (e.g., IK1 conductance, membrane capacitance or external K+ ion concentration) when needed. Converting a model parameter to a digital command was done using macros in Patchmaster.
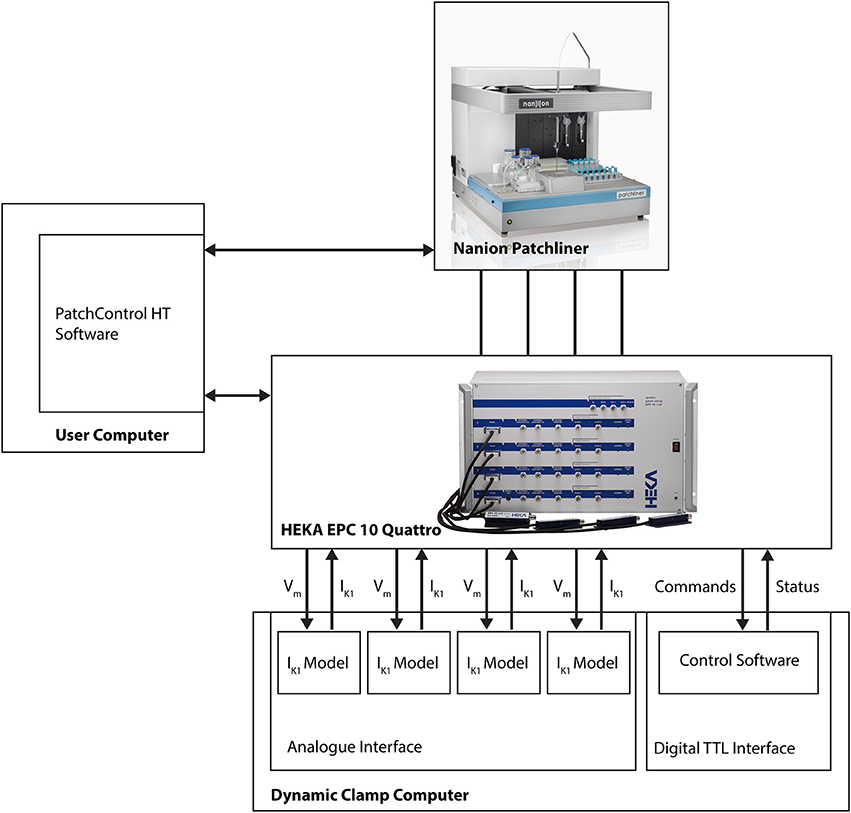
Figure 1. Block diagram showing connections between Patchliner, patch clamp amplifier and dynamic clamp system. For a more detailed depiction of the dynamic clamp system we refer to Supplementary Figure 1.
Because of this tight integration with the APC and its software, no direct user interaction with the dynamic clamp setup is necessary. The dynamic clamp system is completely controlled from within the HEKA PatchMaster or the Patchliner PatchControlHT software used to run experiments, and can be set automatically using the programming features in these software programs (i.e., Protocol Editor in PatchMaster or Tree Editor in PatchControlHT).
Statistics
All results are presented as mean ± standard error of the mean (s.e.m.). Differences in mean outcomes were tested using a One-way ANOVA followed by Tukey's multiple comparisons test, p-values smaller than 0.05 were considered significant.
Results
Na+ and Ca2+ Currents in iPSC-CMs
Single iPSC-CMs dissociated from iPSC-CM cultures were loaded in the automated patch clamp device and studied in voltage clamp mode to record Na+ and Ca2+ currents. Capture of the iPSC-CM in the patch clamp chip was efficient, with appropriate seals in 58% of captured cells (see Table 1). After obtaining whole cell configuration, Na+ and Ca2+ currents could be recorded in ~70% of iPSC-CMs (see Table 2).
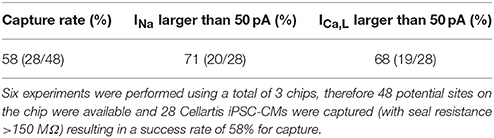
Table 1. iPSC-CMs can be captured efficiently using automated patch clamp and display Na+ and Ca2+ currents.
Current-voltage relations of both currents were similar to those reported for iPSC-CMs in manual patch clamp experiments (Ma et al., 2011), showing maximal peak current amplitudes at −30 mV for Na+ currents (Figures 2A,B) and 10 mV for Ca2+ currents (Figures 2C,D). The Ca2+ currents could be blocked by nifedipine with an IC50 of 252 ± 186 nM, confirming we recorded current conducted by L-type Ca2+ channels (Figures 2E,F).
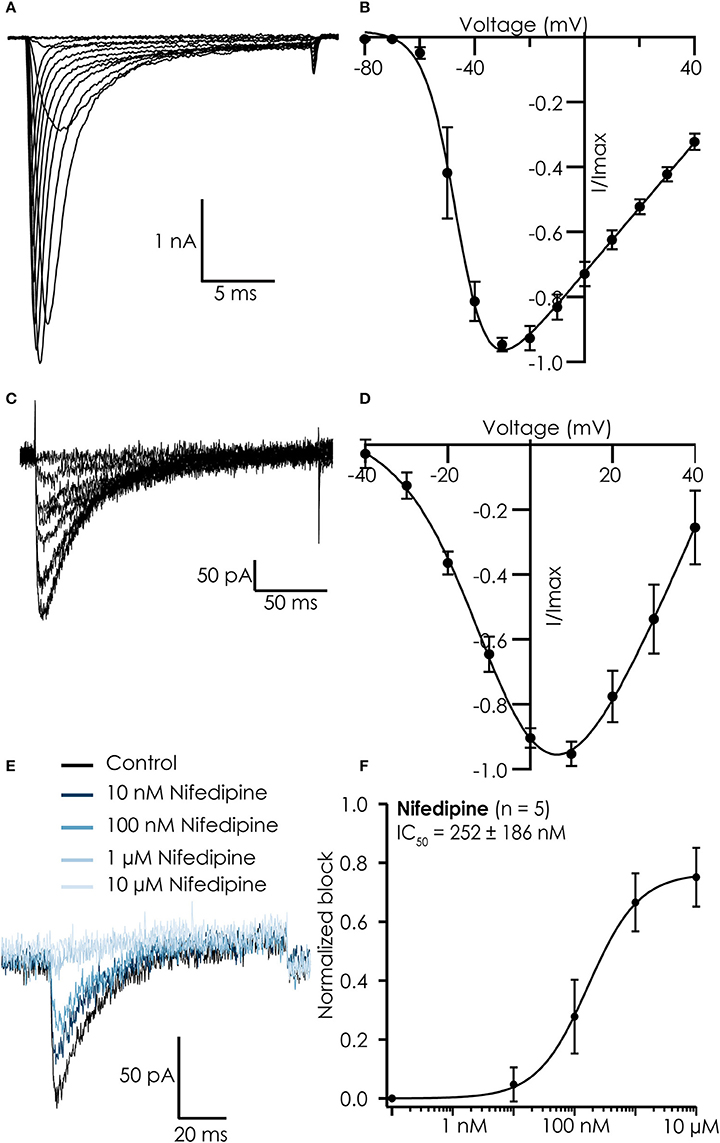
Figure 2. Typical recordings from hiPSC Cellartis Cardiomyocytes recorded on the Patchliner. (A) Na+ currents in response to increasing voltage steps. (B) Corresponding current-voltage plot for an average of 7 cells. A Boltzmann fit revealed a Vhalf of activation of −46 mV. (C) Ca2+ currents in response to increasing voltage steps. (D) Corresponding current-voltage plot for an average of 18 cells. A Boltzmann fit revealed a Vhalf of activation of −5.8 mV. (E) Raw traces of Ca2+ current in control conditions (black) and after inhibition by increasing concentrations of nifedipine (blue). (F) The concentration response curve (normalized to maximum block) for nifedipine for an average of 5 cells. The average concentration response curve was fitted with a standard Hill-equation which revealed an IC50 = 252 ± 186 nM (n = 5).
IK1 Currents in iPSC-CMs
Functionality of IK1 currents was studied in single dissociated iPSC-CMs that were loaded in the automated patch clamp device. Voltage clamp studies in control solution, and in presence of 10 μM Ba2+ to block IK1 currents, showed that we could record Ba2+-sensitive currents with some resemblance of IK1 in 7 out of 12 cells. Examples of cells showing Ba2+-sensitive and insensitive currents can be found in Figures 3A,C, respectively. However, current densities were low, and both the reversal potential (which was less negative than expected) and the rectification of the currents were not as observed in adult cardiomyocytes (Figures 3B,D).
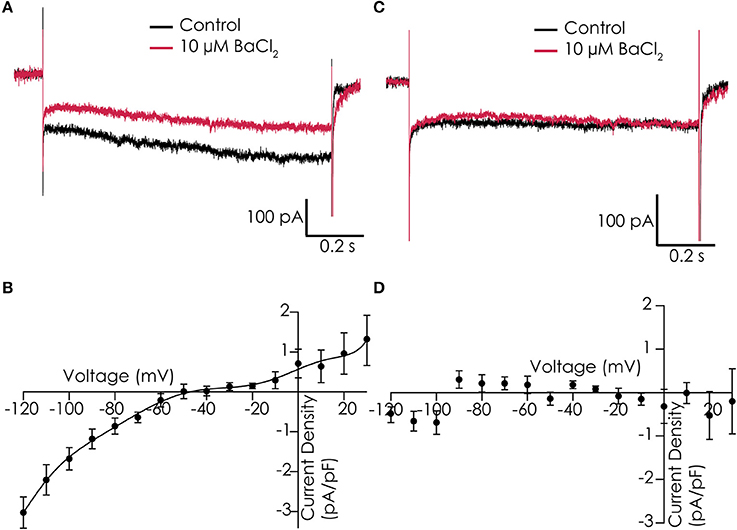
Figure 3. IK1 recorded in Cor.4U cells on the Patchliner. (A) Example of a cell with IK1, shown are responses to a voltage step protocol to −120 mV for 1,200 ms from a holding potential of −40 mV in control conditions (black) and in the presence of 10 μM BaCl2 (red). (B) Average current-voltage relationship of the Ba2+-sensitive current for an average of 7 cells. (C) Current traces of an example cell which does not express IK1 in control (black) and with 10 μM BaCl2 (red). (D) Corresponding current-voltage plot of an average of 3 cells showing no Ba2+-sensitive current.
Single Suspended iPSC-CMs Have Depolarized Membrane Potentials
While the voltage clamp experiments demonstrated that the cardiac Na+ and Ca2+ channels remain functional after dissociation, recording action potentials from iPSC-CMs in suspension was challenging. After switching to current clamp mode, the resting membrane potential of iPSC-CMs was typically between 0 and −15 mV. A negative resting membrane potential close to the reversal potential of K+ could be achieved by injecting a small constant, negative holding current. After that, action potentials could be triggered with a brief depolarizing stimulus. However, the action potentials were very short and had a monotonic repolarization without a plateau phase, which does not match well with adult human cardiac action potentials (see Figure 4, black trace labeled “−180 pA”). The IK1 channels expressed in fully differentiated human cardiomyocytes hyperpolarize the resting membrane potential of these cells, bringing it close to EK. An important difference with a constant hyperpolarizing current is that IK1 channels close upon strong depolarization, allowing development of the plateau phase of the cardiac action potential. In order to improve our methods, and obtain better action potential recordings from suspended single iPSC-CMs, we implemented the dynamic clamp technique on our automated patch clamp device in order to inject simulated IK1.
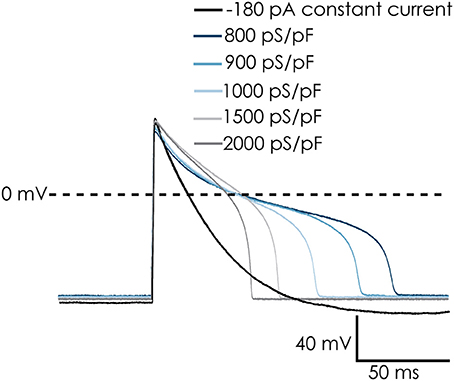
Figure 4. Dynamic clamp used to simulate IK1 conductance on action potentials (APs) of Cellartis Cardiomyocytes. The simulated IK1 conductance could replace injected current to achieve a native resting membrane potential (RMP) of approximately −94 mV. The cells repolarized faster and the AP duration decreased with increasing IK1 conductance. Note: in order to keep a RMP of −94 mV in the absence of simulated IK1 conductance, −180 pA of holding current was injected. This was removed upon addition of IK1 and RMP remains at −94 mV.
Hybrid Models of Suspended iPSC-CMs and Simulated IK1 Channels Produce Longer Action Potentials with a Plateau Phase
Our dynamic clamp implementation is in many ways similar to other published implementations (Bett et al., 2013; Ortega et al., 2014; Meijer van Putten et al., 2015), but differs in two aspects: the functional integration with existing patch clamp control software, and the model used to compute IK1. Earlier work has used IK1 formulations that model the current as a steady-state current, i.e., without time-dependence. The model by Ishihara et al. used in this study is a more detailed, time-dependent model that includes the rectifying effects of Mg2+ and polyamines, as well as two modes of channel closure by spermine (Ishihara et al., 2009). Including this more detailed model is relevant, as time and voltage dependent Mg2+ block or unblock can significantly affect action potential duration (Ishihara et al., 2002).
In current clamp experiments with suspended iPSC-CMs, we injected virtual IK1 current with varying conductance densities (GK1), depending on the specific cell. At depolarized potentials (>−40 mV), the Ishihara model does not generate much outward current, therefore increasing GK1 did not immediately induce hyperpolarization. This could be solved by briefly injecting a hyperpolarizing current, bringing the membrane potential to values inducing a sufficiently large outward IK1 current. As a result, the membrane potential was maintained at or close to EK (which was −95 mV for the simulated IK1 channels) due to the injected virtual IK1 current. After this, the constant hyperpolarizing current was switched off, as it was only needed to start the experiment.
Action potentials recorded from iPSC-CMs with addition of virtual IK1 differed from the earlier recorded brief and monotonically repolarizing action potentials. Injecting IK1 resulted in a stable resting membrane potential, fast upstroke velocities, and prolonged action potential duration with a clear plateau phase (Figure 4). Further increasing GK1, resulting in injection of more IK1, caused a small additional hyperpolarization, but more significantly, also shortened action potential duration (Figure 5A) and slightly decreased upstroke velocity (Figure 5B). This is consistent with the role of IK1 in cardiomyocyte electrophysiology, as it contributes to resting membrane potential stability and the final repolarization phase of the action potential (de Boer et al., 2010). A benefit of our newly developed approach is that experimental throughput can be moderately increased as we can record from, and inject IK1 into, 4 cells in parallel. In Figure 6 we show an example of an experiment in which we were able to record APs from 3 iPSC-CM in parallel (Figure 6A) and have also plotted the injected IK1 (Figure 6B). The fourth channel was available but for this channel no cell was captured successfully. Additional experiments, in which we studied the effect of GK1 on upstroke velocity, showed that we could perform a dynamic clamp experiment in 20 out of 28 wells in 7 experimental runs that used 4 wells per run (71% success rate), see Supplementary Figure 3.
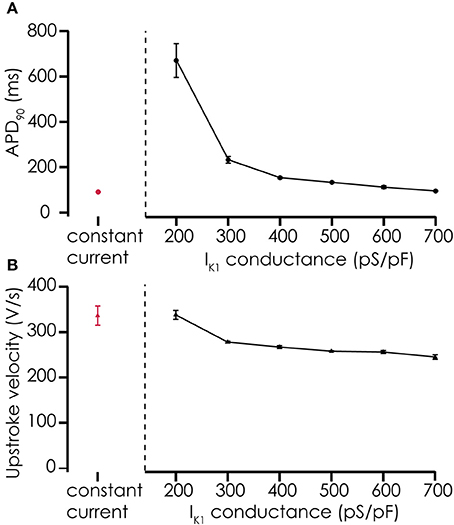
Figure 5. Effects of adding simulated IK1 on AP parameters. (A) Adding IK1 prolongs the APD90 of action potentials recorded from Cellartis Cardiomyocytes (n = 4) compared to constant current injection. With increasing IK1 conductance, the prolongation of the action potential becomes smaller, consistent with the role of IK1 in the final repolarization of the cardiac action potential. (B) Upstroke velocity of the action potentials after addition of simulated IK1 is high, as with constant current injections, and decreases slightly with increasing IK1 conductance.
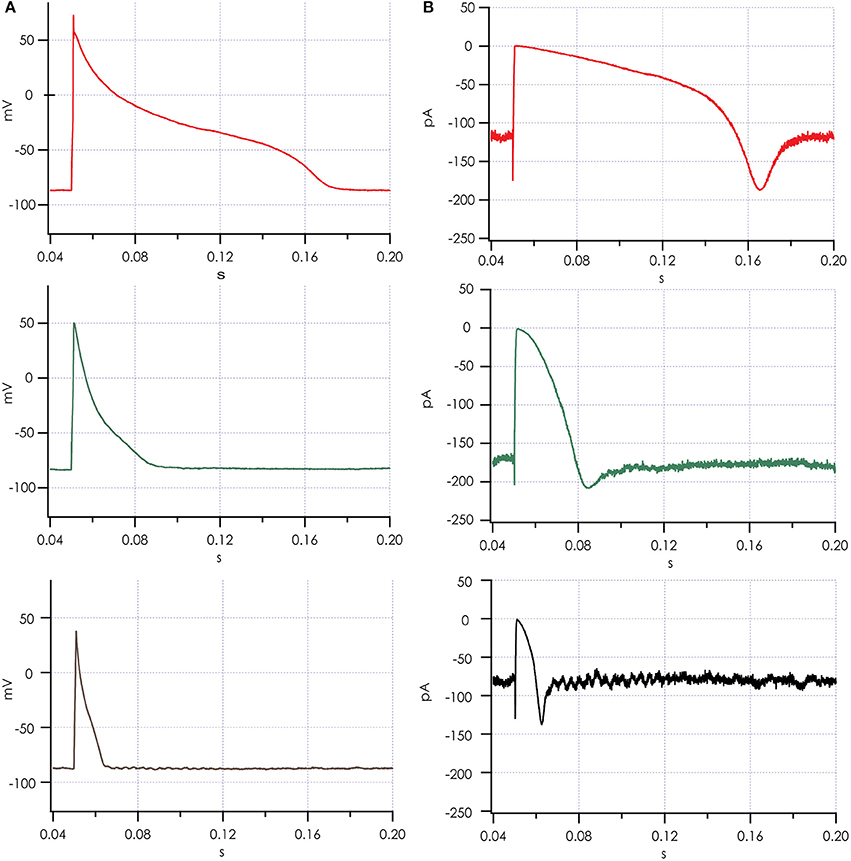
Figure 6. Action potentials recorded simultaneously after adding simulated IK1. (A) Action potentials from 3 cells recorded in parallel on a Patchliner Quattro. (B) Simulated IK1 was recorded for each of the 3 cells and is shown. Note the brief increase in IK1 during the upstroke of the action potential, where IK1 channels respond with increased current to the depolarization induced by the pacing stimulus, which is in line with the slight decrease observed in upstroke velocity with increasing IK1 conductance.
Addition of Virtual Ik1 Channels Restores Sensitivity of the Plateau Phase to Pharmacological Modulation
In earlier experiments, it proved difficult to observe effects of Ca2+ channel antagonists or agonists on the action potential shape of suspended iPSC-CMs when injecting a constant hyperpolarizing current (data not shown), most likely due to suppression of the plateau phase. After observing the restoration of the plateau phase when injecting virtual IK1 current, we tested if the action potential became again sensitive to manipulation of the L-type Ca2+ current. After injecting IK1 (on average GK1 was 267 ± 42 pS/pF) we observed an APD90 of 118 ± 21 ms (n = 6), which significantly prolonged after enhancing the L-type Ca2+ channel with 1 μM BayK-8644 to 155 ± 31 ms (n = 6, see Figures 7A,B). In contrast, blocking the L-type Ca2+ channel with 30 μM nifedipine caused a shortening to 81 ± 14 ms (n = 6). These findings demonstrate that enabling the plateau phase of the action potential of suspended iPSC-CMs by addition of virtual IK1 channels restores action potential sensitivity to L-type Ca2+ channel modulation.
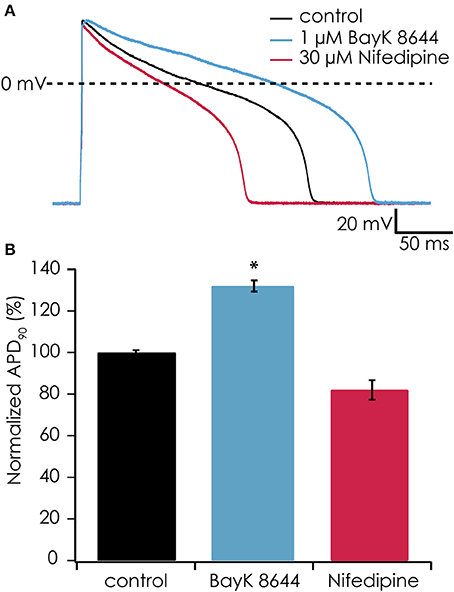
Figure 7. (A) The calcium channel agonist BayK-8644 increased AP duration while simulated IK1 was added (blue trace). Conversely, the calcium channel antagonist nifedipine decreased AP duration (red trace) as compared with control (black trace). In this experiment, IK1 was injected at 400 pS/pF and RMP was −94 mV. (B) Average responses from 6 cells showing significantly increased APD90 after exposure to 1 μM BayK-8644 (*p < 0.05) and decreasing APD90 after exposure to 30 μM nifedipine.
Discussion
In this study, as a proof of principle, we have demonstrated that the dynamic clamp technique can be used in combination with automated patch clamp devices, thereby creating a higher throughput alternative to manual patch clamp. Using dynamic clamp to add virtual IK1 channels to suspended iPSC-CMs allowed us to record action potentials with waveforms that are more representative of the human fully differentiated ventricular cardiomyocyte. This is especially relevant to the CiPA initiative, which aims to use hSC-CMs in drug safety testing.
Achieving higher throughput evaluation of drug effects on action potentials generated by iPSC-CMs will most likely require the use of isolated, suspended cells, as these can be used with automated patch clamp devices. The depolarized resting membrane potential of freshly isolated iPSC-CMs is a challenge that can be overcome, at least to some extent, by using the dynamic clamp technique (this study), but will also require improvement in the dissociation methods used. Dissociation of cardiomyocytes with enzymes disrupting the extracellular matrix, whether native or created in culture, is known to affect the function of IKr, IKs, and IK1 channels (Yue et al., 1996; Hoshino et al., 2012). Hoshino et al. demonstrated that the approach used to isolate cardiomyocytes from neonatal mouse hearts has a significant impact on IK1 channel function and resting membrane potential. Using enzymatic perfusion of the hearts preserved IK1 channels, while the chunk digestion method resulted in four to five times smaller IK1 currents and a depolarization of ~20 mV. The approach used in this and other studies to obtain single, suspended iPSC-CMs is very similar to the chunk digestion method, and we have indeed observed only small Ba2+-sensitive currents, which may be smaller than those observed in studies using adherent iPSC-CM (Ma et al., 2011; Doss et al., 2012; Nunes et al., 2013). Further improvement of cell dissociation protocols may improve results with iPSC-CMs on automated patch clamp devices. This is supported by recent work by Rajamohan et al. who have used a two-step dissociation protocol and recorded APs from the dissociated iPSC-CMs using both manual and automated patch clamping approaches (same device as used in this study). From the data in this study it appears that the two-step protocol yields cells with a more hyperpolarized membrane potential (Rajamohan et al., 2016).
In the present study, we provide proof-of-principle that automated patch clamp devices and dynamic clamp can be combined successfully. The resulting action potential durations and waveforms are very comparable to those obtained in manual patch clamp experiments in which IK1 was added to iPSC-CMs using dynamic clamp (Bett et al., 2013; Meijer van Putten et al., 2015), including the action potential prolongation in response to Bay-K8644. However, more research will be needed to establish the method, and to define its limits and benefits. A better insight into the effects of the specific IK1 model that is applied is needed, as well as a well-defined algorithm that allows us to determine which amount of added IK1 results in the most predictive results and therefore the best safety pharmacology assay. This should subsequently be demonstrated using the set of drugs defined by CiPA. If these goals can be reached, performing predictive patch clamp experiments with iPSC-CMs with an increased throughput becomes feasible.
Author Contributions
BG: data acquisition, data analysis, data interpretation, revising; NB: data acquisition, data analysis, data interpretation, study design, writing; SS-F, AO: data analysis, data interpretation, revising; TvV, MV, NF: data interpretation, revising; TdB: data acquisition, data analysis, data interpretation, study design, writing, revising. All authors meet the following criteria: (i) Substantial contributions to the conception or design of the work; or the acquisition, analysis, or interpretation of data for the work, (2) Drafting the work or revising it critically for important intellectual content, (3) Final approval of the version to be published, (4) Agreement to be accountable for all aspects of the work in ensuring that questions related to the accuracy or integrity of any part of the work are appropriately investigated and resolved.
Funding
This study is supported by MKMD grants 114021501 and 114022502 from ZonMW (TvV and TdB), and a grant from Utrecht Holdings (TdB).
Conflict of Interest Statement
The authors declare that the research was conducted in the absence of any commercial or financial relationships that could be construed as a potential conflict of interest.
Supplementary Material
The Supplementary Material for this article can be found online at: https://www.frontiersin.org/articles/10.3389/fphys.2017.01094/full#supplementary-material
Supplementary Figure 1. Diagram describing implementation of dynamic clamping.
Supplementary Figure 2. Flowchart describing steps in dynamic clamp experiment.
Supplementary Figure 3. Influence of IK1 conductance on upstroke velocity.
Supplementary Data Sheet 1. CellML implementation of IK1 model used in this study.
References
Ando, H., Yoshinaga, T., Yamamoto, W., Asakura, K., Uda, T., Taniguchi, T., et al. (2017). A new paradigm for drug-induced torsadogenic risk assessment using human iPS cell-derived cardiomyocytes. J. Pharmacol. Toxicol. Methods 84, 111–127. doi: 10.1016/j.vascn.2016.12.003
Bett, G. C. L., Kaplan, A. D., Lis, A., Cimato, T. R., Tzanakakis, E. S., Zhou, Q., et al. (2013). Electronic “expression” of the inward rectifier in cardiocytes derived from human induced pluripotent stem cells. Heart Rhythm. 10, 1903–1910. doi: 10.1016/j.hrthm.2013.09.061
Blinova, K., Stohlman, J., Vicente, J., Chan, D., Johannesen, L., Hortigon-Vinagre, M. P., et al. (2017). Comprehensive translational assessment of human-induced pluripotent stem cell derived cardiomyocytes for evaluating drug-induced arrhythmias. Toxicol. Sci. 155, 234–247. doi: 10.1093/toxsci/kfw200
de Boer, T. P., Houtman, M. J. C., Compier, M., and van der Heyden, M. A. G. (2010). The mammalian K(IR)2.x inward rectifier ion channel family: expression pattern and pathophysiology. Acta Physiol. 199, 243–256. doi: 10.1111/j.1748-1716.2010.02108.x
Doss, M. X., Di Diego, J. M., Goodrow, R. J., Wu, Y., Cordeiro, J. M., Nesterenko, V. V., et al. (2012). Maximum diastolic potential of human induced pluripotent stem cell-derived cardiomyocytes depends critically on I(Kr). PLoS ONE 7:e40288. doi: 10.1371/journal.pone.0040288
Gintant, G., Sager, P. T., and Stockbridge, N. (2016). Evolution of strategies to improve preclinical cardiac safety testing. Nat. Rev. Drug Discov. 15, 457–471. doi: 10.1038/nrd.2015.34
Goversen, B., Van der Heyden, M. A. G., van Veen, T. A. B., and de Boer, T. P. (2017). The immature electrophysiological phenotype of iPSC-CMs still hampers in vitro drug screening: Special focus on IK1. Pharmacol. Ther. doi: 10.1016/j.pharmthera.2017.10.001. [Epub ahead of print].
Hoshino, S., Omatsu-Kanbe, M., Nakagawa, M., and Matsuura, H. (2012). Postnatal developmental decline in I K1 in mouse ventricular myocytes isolated by the Langendorff perfusion method: comparison with the chunk method. Pflügers Arch. Eur. J. Physiol. 463, 649–668. doi: 10.1007/s00424-012-1084-0
Ishihara, K., Sarai, N., Asakura, K., Noma, A., and Matsuoka, S. (2009). Role of Mg2+ block of the inward rectifier K+ current in cardiac repolarization reserve: A quantitative simulation. J. Mol. Cell. Cardiol. 47, 76–84. doi: 10.1016/j.yjmcc.2009.03.008
Ishihara, K., Yan, D.-H., Yamamoto, S., and Ehara, T. (2002). Inward rectifier K2+ current under physiological cytoplasmic conditions in guinea-pig cardiac ventricular cells. J. Physiol. 540, 831–841. doi: 10.1113/jphysiol.2001.013470
Jonsson, M. K. B., Vos, M. A., Mirams, G. R., Duker, G., Sartipy, P., de Boer, T. P., et al. (2012). Application of human stem cell-derived cardiomyocytes in safety pharmacology requires caution beyond hERG. J. Mol. Cell. Cardiol. 52, 998–1008. doi: 10.1016/j.yjmcc.2012.02.002
Li, M., Kanda, Y., Ashihara, T., Sasano, T., Nakai, Y., Kodama, M., et al. (2017). Overexpression of KCNJ2 in induced pluripotent stem cell-derived cardiomyocytes for the assessment of QT-prolonging drugs. J. Pharmacol. Sci. 134, 75–85. doi: 10.1016/j.jphs.2017.05.004
Ma, J., Guo, L., Fiene, S. J., Anson, B. D., Thomson, J. A., Kamp, T. J., et al. (2011). High purity human-induced pluripotent stem cell-derived cardiomyocytes: electrophysiological properties of action potentials and ionic currents. Am. J. Physiol. Heart Circ. Physiol. 301, H2006–H2017. doi: 10.1152/ajpheart.00694.2011
Meijer van Putten, R. M. E., Mengarelli, I., Guan, K., Zegers, J. G., van Ginneken, A. C. G., Verkerk, A. O., et al. (2015). Ion channelopathies in human induced pluripotent stem cell derived cardiomyocytes: a dynamic clamp study with virtual IK1. Front Physiol 6:7. doi: 10.3389/fphys.2015.00007
Nunes, S. S., Miklas, J. W., Liu, J., Aschar-Sobbi, R., Xiao, Y., Zhang, B., et al. (2013). Biowire: a platform for maturation of human pluripotent stem cell–derived cardiomyocytes. Nat. Methods 10, 781–787. doi: 10.1038/nmeth.2524
Ortega, F. A., Butera, R. J., Christini, D. J., White, J. A., and Dorval, A. D. (2014). Dynamic clamp in cardiac and neuronal systems using RTXI. Methods Mol. Biol. 1183, 327–354. doi: 10.1007/978-1-4939-1096-0_21
Ortega, F. A., Grandi, E., Krogh-Madsen, T., and Christini, D. J. (2017). Applications of dynamic clamp to cardiac arrhythmia research: Role in drug target discovery and safety pharmacology testing. Front Physiol. 8:1094. doi: 10.3389/fphys.2017.01094
Rajamohan, D., Kalra, S., Duc Hoang, M., George, V., Staniforth, A., Russell, H., et al. (2016). Automated electrophysiological and pharmacological evaluation of human pluripotent stem cell-derived cardiomyocytes. Stem Cells Dev. 25, 439–452. doi: 10.1089/scd.2015.0253
Vaidyanathan, R., Markandeya, Y. S., Kamp, T. J., Makielski, J. C., January, C. T., and Eckhardt, L. L. (2016). IK1-enhanced human-induced pluripotent stem cell-derived cardiomyocytes: an improved cardiomyocyte model to investigate inherited arrhythmia syndromes. Am. J. Physiol. Heart Circ. Physiol. 310, H1611–H1621. doi: 10.1152/ajpheart.00481.2015
van den Heuvel, N. H. L., van Veen, T. A. B., Lim, B., and Jonsson, M. K. B. (2014). Lessons from the heart: Mirroring electrophysiological characteristics during cardiac development to in vitro differentiation of stem cell derived cardiomyocytes. J. Mol. Cell. Cardiol. 67, 12–25. doi: 10.1016/j.yjmcc.2013.12.011
Wilders, R. (2006). Dynamic clamp: a powerful tool in cardiac electrophysiology. J. Physiol. 576, 349–359. doi: 10.1113/jphysiol.2006.115840
Keywords: automated patch clamp electrophysiology, cardiomyocyte, stem cell, dynamic clamp, inward rectifying potassium ion channels, safety pharmacology
Citation: Goversen B, Becker N, Stoelzle-Feix S, Obergrussberger A, Vos MA, van Veen TAB, Fertig N and de Boer TP (2018) A Hybrid Model for Safety Pharmacology on an Automated Patch Clamp Platform: Using Dynamic Clamp to Join iPSC-Derived Cardiomyocytes and Simulations of Ik1 Ion Channels in Real-Time. Front. Physiol. 8:1094. doi: 10.3389/fphys.2017.01094
Received: 29 June 2017; Accepted: 12 December 2017;
Published: 19 January 2018.
Edited by:
Blanca Rodriguez, University of Oxford, United KingdomReviewed by:
David Christini, Weill Cornell Medical College, Cornell University, United StatesAntonio Zaza, Università degli studi di Milano Bicocca, Italy
Patrick M. McDonough, Vala Sciences, United States
Copyright © 2018 Goversen, Becker, Stoelzle-Feix, Obergrussberger, Vos, van Veen, Fertig and de Boer. This is an open-access article distributed under the terms of the Creative Commons Attribution License (CC BY). The use, distribution or reproduction in other forums is permitted, provided the original author(s) or licensor are credited and that the original publication in this journal is cited, in accordance with accepted academic practice. No use, distribution or reproduction is permitted which does not comply with these terms.
*Correspondence: Teun P. de Boer, dC5wLmRlYm9lckB1bWN1dHJlY2h0Lm5s
†These authors have contributed equally to this work.