- 1Department of Training and Movement Sciences, Humboldt-Universität zu Berlin, Berlin, Germany
- 2Berlin School of Movement Science, Berlin, Germany
Tendons feature the crucial role to transmit the forces exerted by the muscles to the skeleton. Thus, an increase of the force generating capacity of a muscle needs to go in line with a corresponding modulation of the mechanical properties of the associated tendon to avoid potential harm to the integrity of the tendinous tissue. However, as summarized in the present narrative review, muscle and tendon differ with regard to both the time course of adaptation to mechanical loading as well as the responsiveness to certain types of mechanical stimulation. Plyometric loading, for example, seems to be a more potent stimulus for muscle compared to tendon adaptation. In growing athletes, the increased levels of circulating sex hormones might additionally augment an imbalanced development of muscle strength and tendon mechanical properties, which could potentially relate to the increasing incidence of tendon overload injuries that has been indicated for adolescence. In fact, increased tendon stress and strain due to a non-uniform musculotendinous development has been observed recently in adolescent volleyball athletes, a high-risk group for tendinopathy. These findings highlight the importance to deepen the current understanding of the interaction of loading and maturation and demonstrate the need for the development of preventive strategies. Therefore, this review concludes with an evidence-based concept for a specific loading program for increasing tendon stiffness, which could be implemented in the training regimen of young athletes at risk for tendinopathy. This program incorporates five sets of four contractions with an intensity of 85–90% of the isometric voluntary maximum and a movement/contraction duration that provides 3 s of high magnitude tendon strain.
Introduction
Tendinopathy is a clinical condition that is associated with pathological processes within the tendon and pain (Fredberg and Stengaard-Pedersen, 2008). In specific sport disciplines (i.e., jump disciplines) about every second athlete develops a tendinopathy during the athletic career and most individuals suffer from chronic symptoms (Lian et al., 2005). Until recently, only few information was available on the prevalence of tendinopathy in children and adolescents. However, the available literature indicates that tendon overload injury is a common issue in youth sports and that the prevalence increases during maturation (Simpson et al., 2016). Some reports indicate that tendinopathy is the most frequent overuse injury in adolescent athletes (Le Gall et al., 2006). The present narrative review explores the hypothesis that an imbalanced adaptation of muscle and tendon might contribute to the etiology of tendinopathies in youth sports.
In the production of movement, muscles and tendons work as a unit, in which the tendon transmits the forces generated by the muscle to the skeleton (Józsa and Kannus, 1997; Nigg and Herzog, 2007). The resultant stress that is applied to the tendon (i.e., force normalized to tendon cross-sectional area) is a measure of absolute load on the tissue irrespective of its dimensions. Yet, ultimate stress (i.e., stress at tendon failure) varies markedly across different tendons and species (LaCroix et al., 2013). In contrast, the ultimate strain of tendons is remarkably constant (Abrahams, 1967; Loitz et al., 1989; LaCroix et al., 2013; Shepherd and Screen, 2013). This means that, from a mechanobiological perspective, tendon strain is the most adequate indicator of the mechanical demand for the tendon as a result of loading. Though it has been argued that an increase of stress might still contribute to either physiological adaptation (Wiesinger et al., 2015) or pathological changes (Couppé et al., 2013), experimental evidence clearly highlights habitual tendon strain as the most crucial parameter for the risk of injury (Wren et al., 2003; LaCroix et al., 2013; Veres et al., 2013). Therefore, an increase of the strength-generating capacity of a muscle needs to go in line with a corresponding modulation of the mechanical properties of the respective tendon. The increase of tendon stiffness (i.e., the slope of the force-elongation relationship) serves as a protective mechanism for integrity of the tendinous tissue and has been frequently observed in humans to accompany strength gains due to both mechanical loading (Kubo et al., 2001a; Arampatzis et al., 2007; Kongsgaard et al., 2007) as well as maturation (Kubo et al., 2001b, 2014; O'Brien et al., 2010b; Waugh et al., 2012; Mersmann et al., 2016). Yet, there is now growing evidence that the adaptation of muscle and tendon does not necessarily proceed in a uniform manner during a training process. In young athletes, maturation acts as an additional stimulus on the development of the muscle-tendon unit, which could potentially further challenge the uniformity of muscle strength and tendon stiffness changes. In the following chapters, we will summarize potentially influential factors for an imbalanced musculotendinous development during training and maturation. We discuss evidence of non-uniform muscle and tendon adaptation in adults and adolescent athletes and the potential implications. The review concludes with proposing a concept for prevention, which targets the increase of tendon stiffness and is based on recent advancements in our understanding of tendon adaptation.
Influential Factors of Imbalanced Muscle-Tendon Adaptation and Development
The following sections will briefly review the basic mechanisms of muscle and tendon adaptation and mechanotransduction. We will emphasize differences in the temporal dynamics of adaptive changes and the mechanical stimuli that effectively elicit changes of the respective tissue properties, structure and morphology. For a more comprehensive overview on muscle or tendon adaptation the reader is referred, for instance, to the reviews by Toigo and Boutellier (2006), Folland and Williams (2007), and Gonzalez et al. (2016), or Wang (2006), Magnusson et al. (2007), and Bohm et al. (2015), respectively.
Differences in the Temporal Dynamics of Muscle and Tendon Adaptation
Muscle and tendon tissue both adapt to increased mechanical loading from the subcellular to the macroscopic level. Here we give a synopsis about the changes that can be observed in the musculotendinous system that are most relevant for strength and power production. With regard to the scope of this review, we will specifically address the time course of these changes and the features of muscles and tendons that might be responsible for differences in the temporal dynamics of adaptation.
Basic Mechanisms of Muscle Adaptation
The adaptive changes that affect the strength generating capacity of a muscle can be categorized into (a) radial adaptation, (b) longitudinal adaptation and (c) adaptation of specific tension (Goldspink, 1985; Bottinelli, 2001). Radial adaptation describes the modulation of the number of sarcomeres in parallel and is best reflected by changes of the physiological cross-sectional area (PCSA) of a muscle (Haxton, 1944), which is the area of the muscle cross-section perpendicular to the orientation of the fibers. In humans, an increase in muscle PCSA following strength training has been demonstrated for the elbow flexors (Kawakami et al., 1995) and knee extensors (Kawakami et al., 1995; Seynnes et al., 2009; Erskine et al., 2010). Moreover, several authors reported an increase of pennation angle after applying interventions that promote muscle strength (Aagaard et al., 2001; Blazevich et al., 2007; Seynnes et al., 2007; Farup et al., 2012). An increase of pennation angle is considered to be a modulating factor of the PCSA (Alexander and Vernon, 1975) that enables fiber hypertrophy and hence radial muscle growth to exceed the changes of the whole muscle anatomical cross-sectional area (ACSA; Häkkinen et al., 1998; Aagaard et al., 2001). The increase in single muscle fiber cross-sectional area that governs the radial muscle adaptation is the main contributor to the increasing force generating potential of the muscle (Johnson and Klueber, 1991; Aagaard et al., 2001; Farup et al., 2012) and is in turn attributed to increased myofibrillar growth (McDougall et al., 1980) and proliferation (Goldspink, 1970). Longitudinal muscle adaptation refers to the modulation of the number of sarcomeres in series, which is positively associated with the maximum shortening velocity and mechanical power of muscle fibers (Goldspink, 1985). Animal models (Lynn and Morgan, 1994; Butterfield et al., 2005) and indirect evidence from human in vivo studies (Blazevich et al., 2007; Duclay et al., 2009; Potier et al., 2009; Reeves et al., 2009; Franchi et al., 2014; Sharifnezhad et al., 2014) both support the notion that eccentric loading can induce longitudinal muscle plasticity. Specific tension (or force) refers to the intrinsic strength generating capacity of the muscle tissue (i.e., active force normalized to PCSA upon maximum activation). However, in light of the conflicting results on loading-induced changes of single-fiber specific tension (Widrick et al., 2002; D'Antona et al., 2006; Pansarasa et al., 2009) it is still unclear if the modulation of specific tension is a relevant contributor to strength gains in response to exercise (Folland and Williams, 2007).
Basic Mechanisms of Tendon Adaptation
The early work of Ingelmark (1945, 1948) already suggested that tendons adapt to their mechanical environment. When a muscle-tendon unit is repeatedly exposed to increased mechanical loading, for instance by means of resistance exercise, it is commonly observed that the associated gains of muscle strength are accompanied by an increase of tendon stiffness (Kubo et al., 2001a; Arampatzis et al., 2007; Kongsgaard et al., 2007). When a shortening of the tendon is ruled out as a potential contributor, two candidate mechanisms can account for exercise-induced increases of tendon stiffness: (a) changes of the material properties (i.e., elastic modulus) and (b) radial tendon hypertrophy. Exercise intervention studies on human adults that reported an increase of tendon stiffness almost exclusively (with the exception of Kongsgaard et al., 2007) also found the tendon elastic modulus increasing by 17–77% (Kubo et al., 2001a; Arampatzis et al., 2007, 2010; Seynnes et al., 2009; Carroll et al., 2011; Malliaras et al., 2013b; Bohm et al., 2014). In comparison, indications of tendon hypertrophy were documented less consistently, with some evidence of moderate increases of tendon cross-sectional area (CSA; 4–10%) in response to increased mechanical loading (Arampatzis et al., 2007; Kongsgaard et al., 2007; Seynnes et al., 2009; Bohm et al., 2014), and several reports of increased tendon stiffness without concomitant radial tendon growth (Kubo et al., 2001a, 2002, 2007, 2010a; Arampatzis et al., 2010; Carroll et al., 2011; Malliaras et al., 2013b). However, cross-sectional comparisons between athletes and untrained adults suggest that tendon hypertrophy of 20–35% is well possible (Rosager et al., 2002; Magnusson and Kjaer, 2003; Kongsgaard et al., 2005; Seynnes et al., 2013). Negating potential selection bias and intersubject variations by comparing the dominant with the nondominant leg of badminton players and fencers, Couppé et al. (2008) found habitually increased loading to result in an average increase of tendon CSA of about 20%. Therefore, tendon hypertrophy is currently considered to contribute to increased tendon stiffness following long-term mechanical loading.
Temporal Dynamics of Adaptation
It is evident that both muscle and tendon are responsive to mechanical loading. However, the metabolism of tendons is designed to meet the functional demand of bearing loads over long durations and, thus, tendon tissue needs to tolerate low oxygen tension (Józsa and Kannus, 1997). Therefore, tendon tissue is characterized by a lower cell to overall dry mass ratio, vascularization, and metabolism compared to muscle tissue (Peacock, 1959; Smith, 1965; Laitinen, 1967; Ippolito et al., 1980). The half-life of tendon collagen is estimated to be almost tenfold higher compared to the muscle proteins actin and myosin (Lundholm et al., 1981; Thorpe et al., 2010). A recent study that investigated tissue renewal by means of comparing nuclear bomb 14C residues in forensic muscle and tendon samples provided strong support for the hypothesis of a lower rate of tissue remodeling in tendon, especially following the formation of the tendon core tissue during adolescence (Heinemeier et al., 2013). Though similar to muscle proteins, collagen synthesis increases rapidly following exercise (Miller et al., 2005), effective tissue turnover is markedly lower, leading to the suggestion that a considerable amount of the synthesized collagen molecules is not permanently incorporated in the tissue structure but broken down relatively quickly (Heinemeier et al., 2013). In accordance with this notion, it has been demonstrated in adults that changes of muscle morphology and architecture can occur as early as after 3 to 4 weeks in a heavy resistance training intervention (Seynnes et al., 2007; DeFreitas et al., 2011), while there are no reports of such rapid adaptations of tendon morphological or mechanical properties. Moreover, neuronal adaptation enables muscle strength to increase markedly even before major morphological changes occur (Folland and Williams, 2007). An increase of tendon stiffness on the other hand relies on a modulation of tissue metabolism and subsequent adaptive changes of tissue structure and tendon morphology.
Kubo and colleagues investigated the time course of muscle and tendon adaptation in two separate 3-month exercise intervention studies on the patellar (Kubo et al., 2010b) and the Achilles tendon (Kubo et al., 2012), respectively. In both studies, a marked increase of muscle strength preceded significant changes of tendon stiffness by 1–2 month and morphological (CSA) changes occurred at the muscle level only. In contrast, Urlando and Hawkins (2007) reported no significant changes of Achilles tendon strain during maximum voluntary contractions despite an increase of tendon force determined at six time-points over an 8-week strength training intervention. This finding indicates a uniform adaptation of muscle strength and tendon stiffness. However, it is interesting to note that the individual tendon strain values showed great fluctuations between the measurement sessions. The highest single values of tendon strain measured in each session, for example, ranged from 8.6 to 13.5%. This substantial variation indicates that the time course of muscle and tendon adaptation might show an individual development and that imbalances of muscle strength and tendon stiffness might have remained undetected in the study by Urlando and Hawkins (2007) due to the analysis of group mean values only.
In conclusion, both muscle and tendon are able to adapt to a change of their mechanical environment. Though information on the time course of muscle and tendon adaptation in vivo is still rare, the metabolic features and adaptive mechanisms differ between muscle and tendon. Muscle seems to be characterized by a greater rate of effective tissue renewal compared to tendon, and neural adaptation further increases the plasticity of the force generating capacity of the neuromuscular system. Thus, it is possible that imbalances of muscle strength and tendon stiffness can develop during a training process.
Differences of Muscle and Tendon in the Responsiveness to Certain Mechanical Stimuli
Besides the potentially different time course of adaptive changes reviewed above, substantial evidence suggest that muscle and tendon feature significant differences regarding the mechanical stimuli that effectively elicit adaptive changes. This section gives a short overview about the processes of mechanotransduction and the stimuli that seem to successfully activate the respective signaling pathways for both muscle and tendon, and then closes with a comparative discussion.
Mechanotransduction in Muscle
It is now well known that both mechanical and metabolic stress are important, separate but interacting stimuli that trigger muscle adaptation and growth (Goldberg et al., 1975; Vandenburgh and Kaufman, 1979; Rooney et al., 1994; Schott et al., 1995; Smith and Rutherford, 1995). Briefly, mechanical stress subjected to muscle tissue leads to the activation of mechanosensitive calcium channels (Kameyama and Etlinger, 1979), intracellular enzymes and second messengers (Hornberger et al., 2006) and stimulates insulin-like growth factor I (IGF-I) release from the muscle cells (Perrone et al., 1995). These events trigger a signaling cascade via autocrine and direct intracellular pathways that results in an increase of protein synthesis (Tidball, 2005; Toigo and Boutellier, 2006; Gonzalez et al., 2016). It has been demonstrated that the increase of muscle protein synthesis following an acute bout of resistance exercise exceeds the increase of protein breakdown, given a sufficient amino acid availability provided by appropriate feeding (Rennie et al., 1982; Biolo et al., 1995; Phillips et al., 1997). The positive net muscle protein balance remains elevated for several days and contributes to the remodeling of the contractile machinery and, subsequently, to hypertrophy (Kumar et al., 2009; McGlory et al., 2017 for reviews). The increase of protein synthetic capability is mediated by an increase of myonuclear number (Allen et al., 1999). Satellite cells are activated by nitric oxide efflux of stressed myofibers (Anderson, 2000) and proliferate under the regulatory influence of IGF-I (Barton-Davis et al., 1999). The proliferated satellite cells then fuse with existing myofibers as new myonuclei (Allen et al., 1999).
Metabolic stress refers to the exercise-related accumulation of metabolites (specifically lactate and hydrogen ions). The role of metabolic stress for muscle growth in response to exercise has been attributed to the associated systemic growth-related hormone and local myokine up-regulation and/or the increased fiber recruitment with muscle fatigue (Schoenfeld, 2013; Ozaki et al., 2016). Muscle hypertrophy was consequently suggested to be driven by the interaction of mechanical and metabolic stress, and that the degree of contribution depends on the exercise modality (i.e., greater mechanical stress at high intensities and greater metabolic stress at moderate intensities; Ozaki et al., 2016). It can be concluded from this assumption that, given a sufficient overall training volume, a wide range of exercise intensities effectively elicits muscle hypertrophy, which is convincingly supported by experimental evidence (Campos et al., 2002; Tanimoto and Ishii, 2006; Mitchell et al., 2012; Schoenfeld et al., 2015, 2016).
Mechanotransduction in Tendon
In tendon tissue, the load-induced strain of the extracellular matrix is transmitted to the cytoskeleton of the embedded fibroblast via specific transmembrane proteins (Wang, 2006; Heinemeier and Kjaer, 2011). The conformational changes of these transmembrane proteins upon load application and the activation of stretch-sensitive ion channels in the cell membrane activate intracellular signaling cascades of gene and growth factor expression for the up-regulation of collagen and matrix protein synthesis (Sackin, 1995; Chiquet, 1999; Wang, 2006; Lavagnino et al., 2015). Accordingly, studies demonstrate an increased concentration of both interstitial growth factors and binding proteins (Heinemeier et al., 2003; Olesen et al., 2006; Jones et al., 2013) as well as elevated collagen synthesis (Langberg et al., 1999, 2001; Miller et al., 2005) in mechanically loaded tendon tissue. The load-induced proliferation and collagen synthesis of tendon stem cells seems to contribute to this anabolic response of tendons to mechanical loading as well (Bi et al., 2007; Zhang et al., 2010). Further, it has been demonstrated in a rat model that mechanical loading leads to an increased production of enzymes that mediate collagen cross-linking (Heinemeier et al., 2007a), which is thought to be involved in the modulation of collagen cross-link profile in humans following resistance exercise (Kongsgaard et al., 2009).
From a mechanobiological point of view, fibroblast cell deformation and fluid flow-induced shear stress are important determinants of mechanotransduction and, thus, the adaptive response of tendons (Lavagnino et al., 2008). In vitro testing on the effects of cyclic load-application demonstrated that high-level magnitude strains are associated with greater tenocyte cell deformation (Arnoczky et al., 2002), collagen fiber recruitment (Kastelic et al., 1980; Hansen et al., 2002), and greater inhibition of catabolic activity (Lavagnino et al., 2003; Arnoczky et al., 2004) in comparison to lower levels of cyclic strain. These results correspond well to observations from human in vivo exercise intervention studies. Arampatzis et al. (2007, 2010) compared the effects of two equivolume loading regimen and found significant changes of tendon stiffness and elastic modulus of the Achilles tendon only in response to the high-strain protocols [i.e., 90% isometric maximum voluntary contraction (iMVC), corresponding to 4.6% of tendon strain], while no significant changes were induced by moderate-strain training (i.e., 55% iMVC, 2.9% tendon strain). Similar results from experimental studies on the patellar tendon (Kongsgaard et al., 2007; Malliaras et al., 2013b) and two recent meta-analyses (Bohm et al., 2015; Wiesinger et al., 2015) strengthened the conclusion that high-intensity loading is a crucial stimulus for in vivo tendon adaptation. The muscle contraction type (i.e., isometric, concentric, eccentric) for load application does on the other hand not seem to be of particular relevance for the adaptive response (Kjaer and Heinemeier, 2014; Bohm et al., 2015).
Interestingly, against the assumptions of finite-element modeling (Lavagnino et al., 2008), the main body of experimental in vivo evidence suggests that the induction of high strain rates and associated increased fluid flow-related shear stress by means of plyometric exercise fails to elicit significant adaptive changes of human tendons (Kubo et al., 2007; Fouré et al., 2009, 2010; Houghton et al., 2013), even at high-intensity loading magnitudes (Bohm et al., 2014). Though it needs to be acknowledged that there are also reports of an increase of tendon stiffness in response to plyometric loading (Burgess et al., 2007; Wu et al., 2010; Hirayama et al., 2017), some of these findings might have been biased by the lack of consideration of the contribution of antagonistic activity in the calculation of tendon forces (Wu et al., 2010; Hirayama et al., 2017), which leads to an overestimation of the increase of tendon stiffness upon a training-induced reduction of antagonistic coactivation. Yet, more importantly, the few existing studies directly comparing the effects of plyometric training to low-strain-rate loading regimen consistently show lower adaptive responses in the plyometric training groups (Burgess et al., 2007; Kubo et al., 2007; Bohm et al., 2014). It has been argued that a short duration of strain-application (e.g., high-frequency load-relaxation cycles or plyometric loading) might reduce the effectiveness of mechanotransduction processes (Arampatzis et al., 2010; Bohm et al., 2014). In a systematic experimental modulation of strain duration at high strain magnitude in vivo, an increase by up to 3 s facilitated tendon adaptation (Arampatzis et al., 2010; Bohm et al., 2014). However, a further increase of strain duration to 12 s per cycle did not further promote adaptive effects. It seems possible that longer sustained tendon strains become less effective if the strain duration is increased at the expense of the number of loading cycles (Bohm et al., 2014).
Collectively, current in vivo evidence on human tendon adaptation suggests that tendons can be most effectively strengthened if loading regimen incorporate slow repetitive high-magnitude tendon strain application. High strain rate and frequency modes of loading as, for instance, plyometric exercise, do not seem to consistently stimulate tendon adaptation.
Comparison of Muscle and Tendon Mechanical Stimulation
Comparing the types of mechanical stimulation that effectively elicit muscle compared to tendon adaptation, it seems that fatiguing training with moderate loads can trigger increases of muscle strength and size (Moss et al., 1997; Wernbom et al., 2007; Mitchell et al., 2012; Schoenfeld et al., 2016), but do not provide a sufficient stimulus for tendon adaptation (Arampatzis et al., 2007, 2010; Kongsgaard et al., 2007). This way, an increase of muscle strength without a concomitant modulation of tendon stiffness following moderate intensity loading can result in higher tendon strain during maximal voluntary contractions, which implies an increase of the mechanical demand placed upon the tendon by the working muscle (Arampatzis et al., 2007, 2010; Figure 1). Furthermore, numerous studies demonstrated that plyometric loading effectively promotes muscle strength development, also in trained athletes (Sáez-Sáez de Villarreal et al., 2010 for review), while evidence suggests that plyometric loading does not consistently increase tendon stiffness (Kubo et al., 2007; Fouré et al., 2009, 2010; Houghton et al., 2013). Accordingly, Kubo et al. (2007) reported increased tendon elongation (and, thus, tendon strain, given that the rest length did not change) during maximum muscle contractions following their plyometric training intervention. Such differences in the responsiveness of muscles and tendons to plyometric loading and its mechanical implications could be of particular significance in light of the high prevalence of tendon overuse injuries in sports with a plyometric loading profile like volleyball, basketball or athletic jump disciplines (Lian et al., 2005). Studies on growth factor transcription following loading also support the idea of differently graded responses of muscle and tendon to specific types of loading. For example, Heinemeier et al. (2007a,b) found a contraction type-specific expression of growth factors in muscle but not tendon tissue using a rat model. More recently, it was also demonstrated in humans that following a fatiguing one-leg kicking exercise with moderate loads the expression of tissue-specific growth factors increased only at the muscle but not tendon level (Heinemeier et al., 2011). This observation led the authors to conclude that an imbalanced adaptation of muscle and tendon might develop under specific loading conditions (Heinemeier et al., 2007a, 2011).
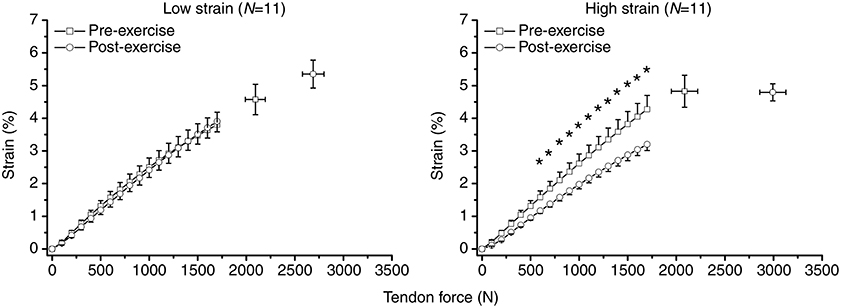
Figure 1. Achilles tendon-aponeurosis force-strain relationship before and after two isometric exercise protocols. The two legs of eleven adults were subjected to either moderate [i.e., 55% maximum voluntary contraction (MVC); low strain] or high loading (90% MVC; high strain) for 14 weeks, respectively. Both protocols induced an increase of tendon force. However, following moderate loading (i.e., low strain protocol) tendon stiffness did not change significantly and, thus, there was a significant increase of tendon strain during maximum muscle contractions. This implies an increase of the mechanical demand placed upon the tendon. No change was observed in a control group (data not shown). *Significant difference between pre- and post-exercise values (Arampatzis et al., 2007, adapted with permission from The Company of Biologists Limited).
In summary, muscle responds well to a wide range of exercise modalities with an increase of strength. Tendon tissue on the other hand seems only to be responsive to high magnitude loading and repetitive loading cycles featuring long tendon strain durations show greater effects compared to modes of loading where single-cycle strain duration is short. Those differences in the responsiveness to certain stimuli might promote the development of imbalances of muscle strength and tendon stiffness in the training process in specific sport disciplines (e.g., jump disciplines).
The Effects of Maturation on Muscle and Tendon Development and Plasticity
Aside from mechanical loading, maturation induces profound changes of the skeletal, neuromuscular and tendinous system in young athletes. The following section briefly reviews muscle and tendon development from child to adulthood, then focuses on somatic and hormonal changes that might challenge the balance of the development of muscle and tendon properties. The section closes with a synopsis of recent experimental evidence of an imbalanced muscle and tendon development in adolescent athletes.
Maturation and Muscle Development
Whole body muscle mass increases progressively from childhood to adulthood, with a pronounced rise during adolescence, especially in boys (Malina et al., 2004; McCarthy et al., 2014; Kim et al., 2016). Even when normalized to body mass, these changes are still marked in boys yet modest in girls (McCarthy et al., 2014; Kim et al., 2016). Studies investigating single muscle development consequently reported an increase in length, ACSA and volume (Kanehisa et al., 1995a,b; Kubo et al., 2001b; Neu et al., 2002; Tonson et al., 2008; O'Brien et al., 2010c). It seems that the gain of muscle volume is governed by both an increase in PCSA and fascicle length, yet the gains in PCSA exceed those of fascicle length in pennate muscles, which is an indication of a remodeling in favor of force production (Morse et al., 2008; O'Brien et al., 2010c; Bénard et al., 2011). Together with an increase of moment arm lengths (O'Brien et al., 2009; Waugh et al., 2012) and muscle activation (Dotan et al., 2012), this leads to a disproportionate increase of muscle strength (O'Brien et al., 2010a). The development of muscle PCSA from childhood to adulthood is most likely based on single fiber hypertrophy and not hyperplasia (Bowden and Goyer, 1960; Aherne et al., 1971; Oertel, 1988; Lexell et al., 1992). The growth hormone-IGF-I axis, which is markedly activated during adolescence for the regulation of overall body growth, stimulates fiber hypertrophy and protein synthesis (Grohmann et al., 2005). For example, myoblast proliferation and fusion with myotubes—a prerequisite for radial and longitudinal fiber growth—depends on growth hormone and IGF-I secretion (Cheek et al., 1971; Allen et al., 1999; Grohmann et al., 2005). Consequently, there is a close association between single fiber CSA and body height (Aherne et al., 1971). The alteration of the endocrine environment during adolescence, specifically the increasing systemic levels of sex steroid hormones, further initiate the development of the muscle functional, morphological and structural differences between boys and girls (Oertel, 1988; Round et al., 1999).
Maturation and Tendon Development
First information on the development of the mechanical properties of human tendinous tissue in vivo was provided by Kubo et al. (2001b). Their comparison of vastus lateralis tendon-aponeurosis compliance between children, adolescents and adults indicated a progressive increase of tendinous stiffness (i.e., the inverse of compliance) from childhood to adulthood, despite the longitudinal growth of the muscle-tendon unit. Theoretically, an increase in length of the series elastic elements would reduce their stiffness (given similar material properties and CSA; Butler et al., 1978). However, O'Brien et al. (2010b) found greater stiffness of the patellar tendon in adults compared to pre-pubertal children as well, and Mersmann et al. (2016) recently demonstrated an increase of patellar tendon stiffness in a longitudinal study over 1 year throughout adolescence. More detailed information on the time course of human tendon development in vivo is strongly limited. Kubo et al. (2014) compared the mechanical and morphological properties of the patellar tendon of elementary and high school boys to adult men. The results indicated that the major developmental increase of tendon elastic modulus from childhood to adulthood occurs until early-adolescence. This corresponds to the observations on the Achilles tendon by Waugh et al. (2012), who found that the differences in Achilles tendon material properties between younger (5–7 years) and older pre-pubertal children (aged 8–10 years) were of similar order as the differences between the latter group and the notably older adults (~26 years). Thus, the material properties of tendons might demonstrate their most pronounced development early in youth (i.e., before the growth spurt at the onset of adolescence), while it seems that tendon hypertrophy progresses further throughout adolescence (Kubo et al., 2014). This assumption also parallels observations in rodent models (Ansorge et al., 2011; Miller et al., 2012). The development can probably be attributed to a great extent to the increase of mechanical loading due to gains in body mass and muscle strength, as predicted by Waugh et al. (2012) using a stepwise multiple regression model and data of the Achilles tendon properties of children and adults. Interestingly, age was shown to be an additional significant predictor of elastic modulus in the regression model, independent of body mass and tendon stress, explaining 31 and 52% of the variance of the elastic modulus in children and both age groups combined, respectively. Thus, it seems very likely that maturation is a separate factor for tendon development, besides the effects of increased mechanical loading. Indeed, several hormones and growth factors that are involved in the regulation of somatic growth (see Murray and Clayton, 2013 for review) have been shown to mediate tendon metabolism as well. For instance, growth hormone and IGF-I stimulate gene expression, collagen synthesis and cross-linking (Abrahamsson et al., 1991; Choy et al., 2005; Doessing et al., 2010; Nielsen et al., 2014) and thyroid hormones are involved in the regulation of tenocyte proliferation, growth and collagen synthesis (Oliva et al., 2013; Berardi et al., 2014).
Challenges to the Musculotendinous System Induced by Maturation
Neugebauer and Hawkins (2012) reported that the longitudinal growth of the muscle-tendon unit during adolescence can go in line with a temporary reduction of tendon CSA. At given tendon material properties, the resultant increase of tendon stress would lead to higher tendon strain. However, as Neugebauer and Hawkins (2012) found both elastic modulus and maximum tendon strain to increase only in tendency, the implications of the observed morphological development of tendons during the adolescent growth spurt still need to be elucidated. Similarly, little information is available on the effects of the rapid increase of circulating sex hormones (i.e., testosterone in boys and estrogens in girls) during puberty on the muscle-tendon unit. Yet, it seems possible that the change of the endocrine milieu could affect the uniformity of the development of muscle strength and tendon stiffness. It is well established that testosterone is one of the most potent hormones promoting muscle hypertrophy and thus increasing muscle strength (see Vingren et al., 2010 for review). Its role in the development of tendinous tissue on the other hand is basically unknown to date (Hansen and Kjaer, 2014). In adults it has been shown that anabolic-androgenic steroid supplementation stimulates collagen synthesis (Pärssinen et al., 2000) and increases tendon stiffness (Inhofe et al., 1995; Marqueti et al., 2011; Seynnes et al., 2013), but impairs tissue remodeling (Marqueti et al., 2006) and reduces ultimate stress and strain (Inhofe et al., 1995; Marqueti et al., 2011; Tsitsilonis et al., 2014). Though it seems likely that steroid supplementation is not necessarily representative of the physiological mechanisms of testosterone action, it can at least be concluded that the anabolic and strength-promoting effects of testosterone are more clearly established for muscle than tendon tissue. The effects of estrogens on muscle and tendon metabolism have been studied more extensively. In a recent review, Hansen and Kjaer (2014) concluded that current scientific evidence from studies on humans renders estrogens as muscle-anabolic, since they decrease protein turnover and increase the responsiveness to mechanical loading. Conversely, estrogens seem to reduce tendon collagen synthesis and the plasticity of tendon mechanical properties in response to exercise (Miller et al., 2007; Hansen et al., 2009). While it must be stated clearly that our current understanding of the effects of sex hormones on the muscle-tendon unit is based primarily on studies administering exogenous hormones to adults, these findings allow the hypothesis that the change of endogenous sex hormone levels in child to adulthood development could contribute to an imbalanced adaptation of muscle and tendon in youth athletes.
Evidence of Imbalanced Muscle and Tendon Development in Adolescent Athletes
A first series of studies that explicitly investigated the uniformity of muscle and tendon development and adaptation during adolescence supports the idea that the two-fold stimulus of (a) maturation and (b) a predominantly plyometric loading profile can lead to a musculotendinous imbalance that increases the load (i.e., stress) and internal demand (i.e., strain) for the tendon. Mersmann et al. (2014) compared mid-adolescent to middle-aged elite volleyball athletes, which were subjected to many years of sport-specific loading. While there were no significant differences in vastus lateralis PCSA and in the force applied to the patellar tendon during maximum isometric knee extension contractions, the adolescents had a deficit with regard to patellar tendon CSA compared to the adult counterparts and, as a consequence, were subjected to increased levels of tendon stress and strain. The conclusion that the morphological plasticity of the tendon unfolds at later stages during development was supported by the results of a subsequent 2-year longitudinal study. The observations suggested that the muscular development was already far progressed in the mid-adolescent athletes, demonstrating only minor changes until the end of adolescence, while the tendon still showed remarkable radial growth and an associated increase of stiffness (Mersmann et al., 2017a). Finally, the time course of muscle and tendon development in mid-adolescent athletes was investigated in five measurement sessions over 1 year in more detail and the effects of maturation and mechanical loading were differentiated by including a similar-aged control group of non-athletes (Mersmann et al., 2016). It was found that the development in elite volleyball athletes was characterized by significantly greater fluctuations in muscle strength and a non-uniformity of muscle and tendon adaptation. Consequently, tendon strain during maximum contractions was not only increased chronically in comparison to controls, but also demonstrated significantly greater fluctuations during the period of investigation (Figure 2). In addition to the discordant changes of muscle strength and tendon stiffness that occur during a training process, results of a very recent study indicate that also the adaptive potential, at least in response to predominantly plyometric long-term loading, is lower with regard to tendon stiffness compared to muscle strength in both adolescent boys and girls (Mersmann et al., 2017b). Though from this study it still remains an assumption that maturation contributed to the development musculotendinous imbalance in addition to the unfavorable type of loading, the increased stress and strain observed in the earlier study (Mersmann et al., 2014) in adolescent athletes compared to the adults, which were habitually subjected to intense plyometric loading as well, carefully suggest that the risk might be increased during adolescence.
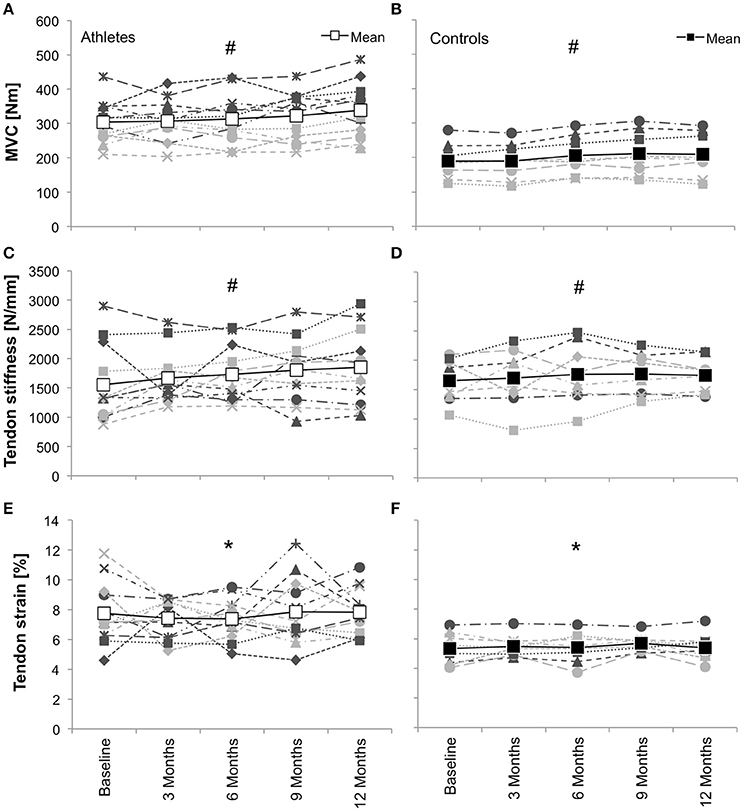
Figure 2. On-year development (in 3-month intervals) of knee extensor muscle strength (MVC; A,B), patellar tendon stiffness (C,D) and tendon strain during maximum contractions (E,F) in adolescent volleyball athletes (n = 12; A,C,E; white symbols show mean values) and controls (n = 8; B,D,F; black symbols show mean values), including individual data of female (light gray) and male participants (dark gray) in both groups. *Significant difference between groups (i.e., intercept; p < 0.05); #significant change over time (i.e., slope; p < 0.05). Note that differences between groups were not tested for MVC and stiffness. The data shows greater fluctuations of muscle strength in the athletes and, as a result of an imbalanced adaptation of muscle and tendon, both greater average tendon strain during maximum contractions as well as greater fluctuations of strain over time (Mersmann et al., 2016, with permission from American Physiological Society).
Summary
Collectively, the evidence reviewed in this chapter strongly suggests that muscle and tendon show differences in the time course of adaptation to mechanical loading and in the types of mechanical stimulation that effectively elicits adaptive processes. Maturation acts as an additional stimulus on the muscle-tendon unit of young athletes and could further contribute to a development of an imbalance of muscle strength and tendon stiffness. Adolescence could be a critical phase in that context due to the associated increase of sex hormones. However, the interplay of mechanical loading and changes of the hormonal milieu on muscle and tendon plasticity in general, and with regard to adolescence in particular, is still largely unknown. Similarly, though recent evidence demonstrated that an imbalanced musculotendinous adaptation can occur during adolescence (Mersmann et al., 2014, 2016, 2017a,b), it is yet unclear how the likelihood of an imbalanced adaptation develops as a function of maturation and how it relates to specific types of loading.
Implications and Concepts for Prevention
It has been described above that muscle and tendon properties might not develop homogeneously during a training process. The following chapter provides an overview about the potential implications for the risk of tendon injury based on experimental and epidemiological observations. Finally, we give recommendations for the design of preventive interventions and critically discuss the current lack of knowledge with regard to the efficacy and timing of such preventive measures.
Potential Implications for the Risk of Tendinopathy
Experimental Observations
An imbalanced adaptation of muscle and tendon—when the development of the force generating capacity of a muscle is not paralleled by an adequate change of the properties of the associated tendon—increases the mechanical demand placed upon the tendon at a given activation of the muscle and, therefore, might impose a challenge for the integrity of the tendinous tissue (especially during maximum efforts). Though tendinopathy has certainly a multifactorial etiology, the mechanical strain theory is currently considered the most probable injury mechanism and attributes the histological, molecular and functional changes of the affected tissue to mechanical overload (Archambault et al., 1995; Fredberg and Stengaard-Pedersen, 2008; Magnusson et al., 2010; Legerlotz, 2013). There is convincing evidence that repetitive loading of tendon tissue at high strain magnitudes (Butler et al., 1978; Lavagnino et al., 2006; Legerlotz et al., 2013) leads to cumulative damage in the extracellular matrix by successive collagen denaturation and fibril tears (Woo, 1982; Veres et al., 2013). The subsequent load redistribution among intact fibrils probably increases the risk of damage upon further loading cycles (Neviaser et al., 2012) and might explain the associated decrease of stiffness and ultimate stress (Schechtman and Bader, 2002; Fung et al., 2009, 2010; Legerlotz et al., 2013). The manifestation of tendinopathy is commonly ascribed to the progression of cumulative microtrauma to higher structural levels of the tissue and successive matrix breakdown (Kannus, 1997; Cook and Purdam, 2009). The degenerative cascade might also be related to the discontinued mechanotransduction of ruptured fibrils (Knörzer et al., 1986) and the associated catabolic responses of under-stimulated fibroblasts (Arnoczky et al., 2007). As both scenarios (i.e., mechanical over- or understimulation) are based on initial strain-induced damage, it seems plausible that an imbalanced adaptation of muscle and tendon could increase the risk of overload-induced tendinopathy. Wren et al. (2003) performed static and cyclic loading experiments on human Achilles tendons and demonstrated that the initial level of strain induced by a given load was inversely correlated with time or loading cycles until failure (Figure 3). This suggests that if tendon strain increases during muscle contractions due to an imbalanced musculotendinous adaptation, repetitive loading could induce significant sub-rupture fatigue and trigger pathological processes. Indeed, there are in vivo studies on patients and athletes with tendinopathy, which report increased levels of tendon strain during maximum voluntary contractions (Arya and Kulig, 2010; Child et al., 2010). Though other studies did not identify increased levels of strain—which might be a methodological issue as pain reduces the level of muscle activation (Hart et al., 2010; Palmieri-Smith et al., 2013) and, thus, the tendon strain measured during voluntary contraction—they found other indications of a mechanical weakening of the tendon, like a decrease of stiffness (Helland et al., 2013) or increase of tendon stress (Couppé et al., 2013).
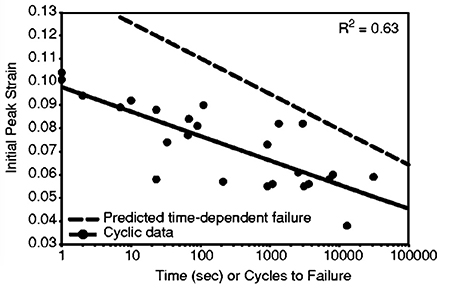
Figure 3. Cyclic loading lifetime results of human oder AT specimen Achilles tendons as a function of initial (peak) tendon strain during loading and associated coefficients of determination (R2). These data demonstrate that the tendon strain magnitude determines the challenge for the tissue integrity. Greater tendon strain at a given load reduces the lifetime of the tendon during cyclic loading (Wren et al., 2003, with permission from Springer).
Epidemiological Observations
The assumption that an imbalanced adaptation of the muscle-tendon unit could contribute to the development of overuse injuries with increasing risk during adolescence also finds support from several epidemiological observations. First, it is interesting to note that the probability of non-contact soft-tissue injury rises when training loads are increased rapidly (Gabbett, 2016). Though this increase clearly could also have different origins, it still indicates that the differing time course of muscle and tendon adaption (i.e., delayed tendon adaptation compared to the increase of muscle strength) might potentially be of clinical relevance. Second, the prevalence of tendinopathy in both elite and recreational athletes is particularly high in sports with predominantly plyometric loading (Lian et al., 2005; Zwerver et al., 2011). In adolescent volleyball players, it was found that jumping ability and the weekly hours of volleyball training (and not strength training) increase the risk of tendinopathy (Visnes et al., 2013). It seems that especially the frequency of jumps during training and competition could be a major determinant of the risk of tendon overload (Bahr and Bahr, 2014). These observations could well be related to the different responsiveness of muscle and tendon to plyometric loading and increased risk of tendon fatigue damage upon repetitive loading with high magnitude strains. Third, a recent epidemiological meta-analysis on tendinopathy in children and adolescents regularly participating in sports indicated an increasing risk with age (Simpson et al., 2016), which corresponds to the incidence of general soft-tissue overuse injuries reported earlier (Stracciolini et al., 2014). These findings underline the potential influence of maturation on the balance of muscle and tendon adaptation and the necessity to deepen our understanding of the interaction of maturation and loading.
Summary
In summary, the pathogenesis and epidemiology of tendinopathy provides a solid theoretical background for the hypothesis that an imbalanced adaptation of muscle and tendon could have consequences for the risk of tendon injury as (a) the resultant increased mechanical demand is a candidate mechanism to induce overload, (b) the prevalence of soft-tissue overuse injuries is high at time-points in the training process (i.e., sudden increase of loading) and in sport disciplines that favor the development of a muscle-tendon imbalance from a mechanobiological point of view, and (c) maturation seems to be a potential risk factor for the development of both tendinopathy and musculotendinous imbalances in young athletes. There certainly is a need to provide more direct support for an association between imbalanced muscle and tendon adaptation and overuse. Yet, it appears that the interaction of maturation with mechanical loading could potentially increase the likelihood of the occurrence of such imbalances, which in turn might be related to the increasing risk of tendon overuse in adolescence. However, these assumptions need to be supported by further research.
Concepts for Prevention
Following the hypothesis that an imbalanced development of muscle strength and tendon stiffness could increase the risk for tendon overuse injury, it might be promising to target the increase of tendon stiffness in groups at risk (e.g., athletes of jump disciplines, adolescents commencing with resistance exercise). The following chapter provides evidence-based suggestions for an effective tendon training and comments on open issues. The chapter concludes with a critical discussion of the current evidence on the effects of preventive interventions outlines the anticipated effects of an intervention-induced increase of tendon stiffness on athletic performance.
Effective Tendon Training
Current evidence on human tendon adaptation in vivo (see section Mechanotransduction in Tendon) suggests that both contraction intensity and contraction duration need to exceed a certain threshold to provide an efficient training stimulus. The training intensity is considered to be optimal around 85–90% of iMVC and the contraction duration around 3 s (Arampatzis et al., 2010; Bohm et al., 2014, 2015; Wiesinger et al., 2015). The contraction mode (i.e., isometric, concentric, eccentric) does not seem to be relevant (Kjaer and Heinemeier, 2014; Bohm et al., 2015), however, it needs to be considered that during classic dynamic training (i.e., eccentric-concentric exercises) the necessary high tendon forces occur only in specific ranges of joint angles due to the change of gear ratios during movement (e.g., between 60° and 100° of knee flexion in a parallel squat; 0° = full extension) (Flanagan et al., 2003; Peñailillo et al., 2015). Therefore, it is recommendable to increase the movement duration (e.g., to ~6 s) when a large range of motion is used during the exercise. Isometric training should be performed in joint angles close to the optimum for force generation (i.e., ~60° knee flexion for patellar tendon training or ~10° ankle dorsiflexion and extended knee for the training the Achilles tendon) and has the advantage that the training stimulus in terms of intensity and duration can be controlled quite easily. Moreover, it does not require the complex technical skills of free weight training for a safe execution and can be performed without expensive equipment (e.g., using non-elastic slings). Figure 4 illustrates a training stimulus for increasing tendon stiffness based on the most effective training protocol of a series investigations that systematically modulated mechanical strain parameters (Arampatzis et al., 2007, 2010; Bohm et al., 2014). We suggest to apply the training protocol three times a week for at least 12 weeks. These recommendations also correspond to the conclusions of two recent meta-analyses on tendon adaptation (Bohm et al., 2015; Wiesinger et al., 2015). Suggestions for specific exercises are provided as Supplementary Material to this review.
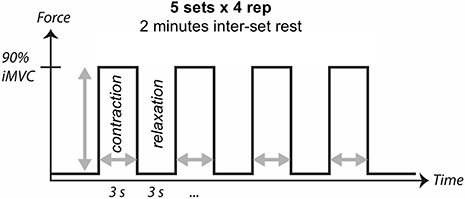
Figure 4. Evidence-based recommendations for an effective stimulus for tendon adaptation. High intensity loading to the tendon (85–90% iMVC) should be applied in five sets of four repetitions with a contraction and relaxation duration of 3 s each, and an inter-set rest of 2 min. We suggest the training to be applied three times a week for at least 12 weeks.
There are several important, but currently open issues that need to be elucidated with regard to the stimulation of tendon adaptation in general, its implementation in elite athletic training as a preventive measure in particular and with regard to its application in youth sports. There have been experimental investigations systematically modulating strain magnitude, rate, duration and frequency (Arampatzis et al., 2007, 2010; Kongsgaard et al., 2007; Bohm et al., 2014), however, the effects of overall training volume, number of sessions per week or rest between sets are basically unexplored thus far, and meta-analyses are limited in their potential to improve our understanding in this regard (Gentil et al., 2017) due to the heterogeneity of intervention studies (Bohm et al., 2015). In a recent study, Waugh et al. (2017) modulated inter-cycle rest duration (i.e., 3 and 10 s) between two equivolume isometric loading protocols. While the improvements of stiffness and material properties were similar between protocols, some potentially unfavorable structural changes were detected by means of ultrasound tissue characterization (UTC) following the short rest loading. It should be noted that it is still unclear if such changes of UTC-parameters indicate overload and the overall loading volume in the study by Waugh and colleagues was 2.5 times the volume of the program that we recommend based on our experience. Nevertheless, these findings highlight the necessity to deepen our understanding of tendon tissue recovery during cyclic loading, especially with regard to injury prevention and rehabilitation.
It is currently a matter of speculation when to implement the preventive intervention during the course of athletic training, if the training stimulus should be applied continuously or in periods, and how a specific tendon training interferes with training regimen that target other determinants of athletic performance. Given the moderate overall loading volume of the program recommended in this review, it seems realistic to implement the tendon exercises without any major reductions of other training contents. It can be considered that the program increases muscle strength as well (Arampatzis et al., 2007, 2010) and, therefore, could complement or replace some routines used for muscle strength development. We are currently applying a preventive tendon training in adolescent athletes to investigate its efficacy. The overall duration of exercises implemented in the regular training schedule that target the increase of tendon stiffness is around 15 min. Considering this low time-effort and the uncertainty if training at specific time-points during a season would be equally effective, we are applying this intervention over the whole season. Future research might help to further develop this approach and investigate if scheduling tendon training in advance of marked increases of loading that is assumed to provide a more potent stimulus for muscle strength compared to tendon stiffness development (i.e., plyometric loading or fatiguing moderate intensity loading) yields similar results.
The recommendations given in this chapter are based on our current knowledge on tendon adaptation, which is derived from studies on adults. Though it is known that the tendons of pre-pubertal children and adolescents are able to adapt to mechanical loading (Waugh et al., 2014; Mersmann et al., 2017b), dose-response relationships specific for the immature tendinous system still need to be established in the future. However, as we are convinced that the basic mechanisms of mechanotransduction should not be profoundly different in children and adults, we can argue that it is likely that the characteristics of an effective stimulus for tendon adaptation (i.e., high strain magnitude and ~3 s strain duration applied with low frequency) is widely independent of age. Nevertheless, there might be restrictions with regard to overall mechanical loading and intensity control. The number of sets and repetitions could then be used to adjust the overall training volume to the loading capacity of the young athletes. Moreover, in age groups where maximum strength testing is contraindicated, perceived exertion scales can be used to estimate training intensity (Waugh et al., 2014).
In conclusion, adult tendons, and likely immature tendons as well, receive effective mechanical stimulation upon high strain magnitude loading with an appropriate strain duration. Though the role of recovery is widely unexplored, the recommendations outlined above could have the potential to decrease the likelihood of an imbalanced muscle and tendon development in a training process. However, it needs to be stated clearly that specific considerations for the implementation in athletic training schedules of different sports and for the application in youth athletes need to be elucidated in future research.
Potential Effects on Risk of Injury and Athletic Performance
The prevention or reduction of musculotendinous imbalances could, in our view, have beneficial effects on (a) the risk of tendon injury and (b) athletic performance. A recent systematic review on the effects of preventive interventions for tendinopathy concluded that evidence for their efficacy is only limited (Peters et al., 2016). However, the exercise interventions examined were either not targeting the improvement of the mechanical properties of the tendon (e.g., balance training, stretching) or did not apply training stimuli that are in accordance with the current view on the mechanobiological basis of human tendon adaptation in vivo (e.g., Alfredson eccentric training or Silbernagel's combined concentric-eccentric exercise; Malliaras et al., 2013a for a discussion). Conventional eccentric training approaches, for example, are characterized by high training volume but only moderate load intensity (i.e., body weight), which might even increase discrepancies between muscle strength and tendon stiffness (Arampatzis et al., 2007, 2010). Though conventional eccentric training is associated with pain relief in patients with tendinopathy, its application as a preventive measure can also increase injury risk of tendons that already feature structural abnormalities (Fredberg et al., 2008). A load-based intervention with scientific evidence supporting its efficacy on promoting tendon stiffness is likely to be a more effective preventive strategy following the hypothesis that an imbalance of muscle and tendon predispose for tendinopathy. An increase of stiffness that parallels the increase of the force generating capacity of the neuromuscular system would serve as a protective mechanism against increased strain during maximum muscle contractions. Moreover, if the increase of stiffness is governed by radial hypertrophy upon long-term training, this would also reduce tendon stress.
Aside from the potentially beneficial effects for the health of young athletes, tendon stiffness and the interaction of muscle and tendon during movement are important contributors to movement performance. For example, increased tendon stiffness is associated with a lower electromechanical delay, a greater rate of force development and jump height (Bojsen-Møller et al., 2005; Waugh et al., 2013). Certainly, the elasticity of the tendon and the associated storage and release of mechanical strain energy are important contributors to sportive performance as well (Roberts, 1997; Kawakami et al., 2002). Greater elongation at a given force allows more energy to be stored in the tendon and has been positively associated, for example, with sprint performance (Stafilidis and Arampatzis, 2007; Kubo et al., 2011). However, a greater muscle force output combined with higher tendon stiffness increases the potential exchange of mechanical energy between muscle and tendon as well (Wu et al., 2010). Moreover, if the force production during a movement task increases due to an increase of the muscular capacity, an increase of stiffness of the series elastic elements is necessary to maintain fascicle kinetics within an optimal working range (Lichtwark and Wilson, 2007). For example, it has been recently shown that vastus lateralis fascicles operate around optimum length and close to optimum velocity for power production (Nikolaidou et al., 2017) during vertical jumping. A change of the balance of muscle strength and series elastic stiffness could lead to a distortion of the musculotendinous interaction and in turn increase the demand for neuromuscular control (i.e., due to a change of fascicle kinetics), which could reduce the extent to which the increased muscular capacity can be exploited. Therefore, it is likely that a facilitation of tendon stiffness in line with muscle strength would lead to greater improvements of movement performance as opposed to a sole increase of muscle strength.
Conclusions and Future Directions
Current scientific evidence strongly supports the idea that the development of muscle strength during a training process is not necessarily accompanied by an adequate modulation of tendon stiffness. The differences in the time course of adaptation and in the mechanical stimuli that trigger adaptive processes provide two mechanisms that can account for a dissociation of the muscular and tendinous development. Though the additional influence of maturation is still a heavily under-investigated topic, it is likely that an imbalanced development of muscle strength and tendon stiffness is a relevant issue for youth sports and it seems that the risk might even be increased compared to adults. Adolescence, with its associated somatic and endocrine processes, could be a critical phase in that regard. Due to the mechanical loading profile, musculotendinous imbalances especially concern athletes from jump disciplines and the high prevalence of tendinopathy in those sports as well as the increasing incidence during adolescence support the hypothesis that imbalances of muscle strength and tendon stiffness could have implications for the health of young athletes. The implementation of interventions targeting the improvement of tendon mechanical properties could be a promising approach to prevent such imbalances, promote athletic performance and reduce the risk of tendon injury. However, there is still a clear lack of information on the time course of changes of the musculotendinous system during premature development and the interaction of maturation and mechanical loading. The effects of changing sex hormone levels on tendon properties and plasticity is also widely unknown. Similarly, the association of musculotendinous imbalances with tendon overuse injury as well as the preventive value of interventions that promote the development of tendon mechanical properties has not been established thus far. The effects of recovery for tendon adaptation in general are largely unexplored and a future challenge with regard to the application of preventive tendon training in youth sports is the determination of age-specific dose-response relationships and the implementation in the training schedule in elite sports. The increasing prevalence of tendinopathy in athletic adolescents certainly calls for further research on these issues.
Author Contributions
All authors substantially contributed to the interpretation of the literature addressed in this review. FM drafted and finalized the manuscript. SB and AA made important intellectual contributions in revision of all sections of the manuscript. AA supervised the preparation of the manuscript. All authors approved the final version of the manuscript and agree to be accountable for the content of the work.
Funding
This review was conducted within the scope of the research project “Resistance Training in Youth Athletes” (http://www.uni-potsdam.de/kraftprojekt/english.php) that was funded by the German Federal Institute of Sport Science (ZMVI1-08190114-18).
Conflict of Interest Statement
The authors declare that the research was conducted in the absence of any commercial or financial relationships that could be construed as a potential conflict of interest.
Supplementary Material
The Supplementary Material for this article can be found online at: https://www.frontiersin.org/articles/10.3389/fphys.2017.00987/full#supplementary-material
References
Aagaard, P., Andersen, J. L., Dyhre-Poulsen, P., Leffers, A. M., Wagner, A., Magnusson, S. P., et al. (2001). A mechanism for increased contractile strength of human pennate muscle in response to strength training: changes in muscle architecture. J. Physiol. 534, 613–623. doi: 10.1111/j.1469-7793.2001.t01-1-00613.x
Abrahams, M. (1967). Mechanical behaviour of tendon in vitro. a preliminary report. Med. Biol. Eng. 5, 433–443. doi: 10.1007/BF02479137
Abrahamsson, S. O., Lundborg, G., and Lohmander, L. S. (1991). Recombinant human insulin-like growth factor-I stimulates in vitro matrix synthesis and cell proliferation in rabbit flexor tendon. J. Orthop. Res. 9, 495–502. doi: 10.1002/jor.1100090405
Aherne, W., Ayyar, D. R., Clarke, P. A., and Walton, J. N. (1971). Muscle fibre size in normal infants, children and adolescents. An autopsy study. J. Neurol. Sci. 14, 171–182. doi: 10.1016/0022-510X(71)90085-2
Alexander, R., and Vernon, A. (1975). The dimensions of knee and ankle muscles and the forces they exert. J. Hum. Mov. Stud. 1, 115–123.
Allen, D. L., Roy, R. R., and Edgerton, V. R. (1999). Myonuclear domains in muscle adaptation and disease. Muscle Nerve 22, 1350–1360. doi: 10.1002/(SICI)1097-4598(199910)22:10<1350::AID-MUS3>3.0.CO;2-8
Anderson, J. E. (2000). A role for nitric oxide in muscle repair: nitric oxide-mediated activation of muscle satellite cells. Mol. Biol. Cell 11, 1859–1874. doi: 10.1091/mbc.11.5.1859
Ansorge, H. L., Adams, S., Birk, D. E., and Soslowsky, L. J. (2011). Mechanical, compositional, and structural properties of the post-natal mouse Achilles tendon. Ann. Biomed. Eng. 39, 1904–1913. doi: 10.1007/s10439-011-0299-0
Arampatzis, A., Karamanidis, K., and Albracht, K. (2007). Adaptational responses of the human Achilles tendon by modulation of the applied cyclic strain magnitude. J. Exp. Biol. 210, 2743–2753. doi: 10.1242/jeb.003814
Arampatzis, A., Peper, A., Bierbaum, S., and Albracht, K. (2010). Plasticity of human Achilles tendon mechanical and morphological properties in response to cyclic strain. J. Biomech. 43, 3073–3079. doi: 10.1016/j.jbiomech.2010.08.014
Archambault, J. M., Wiley, J. P., and Bray, R. C. (1995). Exercise loading of tendons and the development of overuse injuries. A review of current literature. Sports Med. 20, 77–89. doi: 10.2165/00007256-199520020-00003
Arnoczky, S. P., Lavagnino, M., and Egerbacher, M. (2007). The mechanobiological aetiopathogenesis of tendinopathy: is it the over-stimulation or the under-stimulation of tendon cells? Int. J. Exp. Pathol. 88, 217–226. doi: 10.1111/j.1365-2613.2007.00548.x
Arnoczky, S. P., Lavagnino, M., Whallon, J. H., and Hoonjan, A. (2002). In situ cell nucleus deformation in tendons under tensile load; a morphological analysis using confocal laser microscopy. J. Orthop. Res. 20, 29–35. doi: 10.1016/S0736-0266(01)00080-8
Arnoczky, S. P., Tian, T., Lavagnino, M., and Gardner, K. (2004). Ex vivo static tensile loading inhibits MMP-1 expression in rat tail tendon cells through a cytoskeletally based mechanotransduction mechanism. J. Orthop. Res. 22, 328–333. doi: 10.1016/S0736-0266(03)00185-2
Arya, S., and Kulig, K. (2010). Tendinopathy alters mechanical and material properties of the Achilles tendon. J. Appl. Physiol. 108, 670–675. doi: 10.1152/japplphysiol.00259.2009
Bahr, M. A., and Bahr, R. (2014). Jump frequency may contribute to risk of jumper's knee: a study of interindividual and sex differences in a total of 11,943 jumps video recorded during training and matches in young elite volleyball players. Br. J. Sports Med. 48, 1322–1326. doi: 10.1136/bjsports-2014-093593
Barton-Davis, E. R., Shoturma, D. I., and Sweeney, H. L. (1999). Contribution of satellite cells to IGF-I induced hypertrophy of skeletal muscle. Acta Physiol. Scand. 167, 301–305. doi: 10.1046/j.1365-201x.1999.00618.x
Bénard, M. R., Harlaar, J., Becher, J. G., Huijing, P. A., and Jaspers, R. T. (2011). Effects of growth on geometry of gastrocnemius muscle in children: a three-dimensional ultrasound analysis. J. Anat. 219, 388–402. doi: 10.1111/j.1469-7580.2011.01402.x
Berardi, A. C., Oliva, F., Berardocco, M., la Rovere, M., Accorsi, P., and Maffulli, N. (2014). Thyroid hormones increase collagen I and cartilage oligomeric matrix protein (COMP) expression in vitro human tenocytes. Muscles Ligaments Tendons J. 4, 285–291. doi: 10.11138/mltj/2014.4.3.285
Bi, Y., Ehirchiou, D., Kilts, T. M., Inkson, C. A., Embree, M. C., Sonoyama, W., et al. (2007). Identification of tendon stem/progenitor cells and the role of the extracellular matrix in their niche. Nat. Med. 13, 1219–1227. doi: 10.1038/nm1630
Biolo, G., Maggi, S. P., Williams, B. D., Tipton, K. D., and Wolfe, R. R. (1995). Increased rates of muscle protein turnover and amino acid transport after resistance exercise in humans. Am. J. Physiol. 268, E514–E520.
Blazevich, A. J., Cannavan, D., Coleman, D. R., and Horne, S. (2007). Influence of concentric and eccentric resistance training on architectural adaptation in human quadriceps muscles. J. Appl. Physiol. 103, 1565–1575. doi: 10.1152/japplphysiol.00578.2007
Bohm, S., Mersmann, F., and Arampatzis, A. (2015). Human tendon adaptation in response to mechanical loading: a systematic review and meta-analysis of exercise intervention studies on healthy adults. Sports Med. Open 1:7. doi: 10.1186/s40798-015-0009-9
Bohm, S., Mersmann, F., Tettke, M., Kraft, M., and Arampatzis, A. (2014). Human Achilles tendon plasticity in response to cyclic strain: effect of rate and duration. J. Exp. Biol. 217, 4010–4017. doi: 10.1242/jeb.112268
Bojsen-Møller, J., Magnusson, S. P., Rasmussen, L. R., Kjaer, M., and Aagaard, P. (2005). Muscle performance during maximal isometric and dynamic contractions is influenced by the stiffness of the tendinous structures. J. Appl. Physiol. 99, 986–994. doi: 10.1152/japplphysiol.01305.2004
Bottinelli, R. (2001). Functional heterogeneity of mammalian single muscle fibres: do myosin isoforms tell the whole story? Pflugers Arch. 443, 6–17. doi: 10.1007/s004240100700
Bowden, D. H., and Goyer, R. A. (1960). The size of muscle fibers in infants and children. Arch. Pathol. 69, 188–189.
Burgess, K. E., Connick, M. J., Graham-Smith, P., and Pearson, S. J. (2007). Plyometric vs. isometric training influences on tendon properties and muscle output. J. Strength Cond. Res. 21, 986–985. doi: 10.1519/R-20235.1
Butler, D. L., Grood, E. S., Noyes, F. R., and Zernicke, R. F. (1978). Biomechanics of ligaments and tendons. Exerc. Sport Sci. Rev. 6, 125–181.
Butterfield, T. A., Leonard, T. R., and Herzog, W. (2005). Differential serial sarcomere number adaptations in knee extensor muscles of rats is contraction type dependent. J. Appl. Physiol. 99, 1352–1358. doi: 10.1152/japplphysiol.00481.2005
Campos, G. E., Luecke, T. J., Wendeln, H. K., Toma, K., Hagerman, F. C., Murray, T. F., et al. (2002). Muscular adaptations in response to three different resistance-training regimens: specificity of repetition maximum training zones. Eur. J. Appl. Physiol. 88, 50–60. doi: 10.1007/s00421-002-0681-6
Carroll, C. C., Dickinson, J. M., LeMoine, J. K., Haus, J. M., Weinheimer, E. M., Hollon, C. J., et al. (2011). Influence of acetaminophen and ibuprofen on in vivo patellar tendon adaptations to knee extensor resistance exercise in older adults. J. Appl. Physiol. 111, 508–515. doi: 10.1152/japplphysiol.01348.2010
Cheek, D. B., Holt, A. B., Hill, D. E., and Talbert, J. L. (1971). Skeletal muscle cell mass and growth: the concept of the deoxyribonucleic acid unit. Pediatr. Res. 5, 312–328. doi: 10.1203/00006450-197107000-00004
Child, S., Bryant, A. L., Clark, R. A., and Crossley, K. M. (2010). Mechanical properties of the achilles tendon aponeurosis are altered in athletes with achilles tendinopathy. Am. J. Sports Med. 38, 1885–1893. doi: 10.1177/0363546510366234
Chiquet, M. (1999). Regulation of extracellular matrix gene expression by mechanical stress. Matrix Biol. 18, 417–426. doi: 10.1016/S0945-053X(99)00039-6
Choy, V. E., Kyparos, A., Vailas, A. C., Crenshaw, T. D., and Martinez, D. A. (2005). The biphasic response of porcine tendon to recombinant porcine growth hormone. Growth Horm. IGF Res. 15, 39–46. doi: 10.1016/j.ghir.2004.11.003
Cook, J. L., and Purdam, C. R. (2009). Is tendon pathology a continuum? A pathology model to explain the clinical presentation of load-induced tendinopathy. Br. J. Sports Med. 43, 409–416. doi: 10.1136/bjsm.2008.051193
Couppé, C., Kongsgaard, M., Aagaard, P., Hansen, P., Bojsen-Moller, J., Kjaer, M., et al. (2008). Habitual loading results in tendon hypertrophy and increased stiffness of the human patellar tendon. J. Appl. Physiol. 105, 805–810. doi: 10.1152/japplphysiol.90361.2008
Couppé, C., Kongsgaard, M., Aagaard, P., Vinther, A., Boesen, M., Kjaer, M., et al. (2013). Differences in tendon properties in elite badminton players with or without patellar tendinopathy. Scand. J. Med. Sci. Spor. 23, e89–e95. doi: 10.1111/sms.12023
D'Antona, G., Lanfranconi, F., Pellegrino, M. A., Brocca, L., Adami, R., Rossi, R., et al. (2006). Skeletal muscle hypertrophy and structure and function of skeletal muscle fibres in male body builders. J. Physiol. 570, 611–627. doi: 10.1113/jphysiol.2005.101642
DeFreitas, J. M., Beck, T. W., Stock, M. S., Dillon, M. A., and Kasishke, P. R. (2011). An examination of the time course of training-induced skeletal muscle hypertrophy. Eur. J. Appl. Physiol. 111, 2785–2790. doi: 10.1007/s00421-011-1905-4
Doessing, S., Heinemeier, K. M., Holm, L., Mackey, A. L., Schjerling, P., Rennie, M., et al. (2010). Growth hormone stimulates the collagen synthesis in human tendon and skeletal muscle without affecting myofibrillar protein synthesis. J. Physiol. 588, 341–351. doi: 10.1113/jphysiol.2009.179325
Dotan, R., Mitchell, C., Cohen, R., Klentrou, P., Gabriel, D., and Falk, B. (2012). Child-adult differences in muscle activation–a review. Pediatr. Exerc. Sci. 24, 2–21. doi: 10.1123/pes.24.1.2
Duclay, J., Martin, A., Duclay, A., Cometti, G., and Pousson, M. (2009). Behavior of fascicles and the myotendinous junction of human medial gastrocnemius following eccentric strength training. Muscle Nerve 39, 819–827. doi: 10.1002/mus.21297
Erskine, R. M., Jones, D. A., Williams, A. G., Stewart, C. E., and Degens, H. (2010). Resistance training increases in vivo quadriceps femoris muscle specific tension in young men. Acta Physiol. 199, 83–89. doi: 10.1111/j.1748-1716.2010.02085.x
Farup, J., Kjølhede, T., Sørensen, H., Dalgas, U., Møller, A. B., Vestergaard, P. F., et al. (2012). Muscle morphological and strength adaptations to endurance vs. resistance training. J. Strength Cond. Res. 26, 398–407. doi: 10.1519/JSC.0b013e318225a26f
Flanagan, S., Salem, G. J., Wang, M. Y., Sanker, S. E., and Greendale, G. A. (2003). Squatting exercises in older adults: kinematic and kinetic comparisons. Med. Sci. Sport. Exer. 35, 635–643. doi: 10.1249/01.MSS.0000058364.47973.06
Folland, J. P., and Williams, A. G. (2007). The adaptations to strength training: morphological and neurological contributions to increased strength. Sports Med. 37, 145–168. doi: 10.2165/00007256-200737020-00004
Fouré, A., Nordez, A., and Cornu, C. (2010). Effects of plyometric training on plantar flexor mechanical properties. Comput. Methods Biomech. Biomed. Engin. 13, 57–59. doi: 10.1080/10255842.2010.493723
Fouré, A., Nordez, A., Guette, M., and Cornu, C. (2009). Effects of plyometric training on passive stiffness of gastrocnemii and the musculo-articular complex of the ankle joint. Scand. J. Med. Sci. Spor. 19, 811–818. doi: 10.1111/j.1600-0838.2008.00853.x
Franchi, M. V., Atherton, P. J., Reeves, N. D., Flück, M., Williams, J., Mitchell, W. K., et al. (2014). Architectural, functional and molecular responses to concentric and eccentric loading in human skeletal muscle. Acta Physiol. 210, 642–654. doi: 10.1111/apha.12225
Fredberg, U., Bolvig, L., and Andersen, N. T. (2008). Prophylactic training in asymptomatic soccer players with ultrasonographic abnormalities in Achilles and patellar tendons: the Danish super league study. Am. J. Sports Med. 36, 451–460. doi: 10.1177/0363546507310073
Fredberg, U., and Stengaard-Pedersen, K. (2008). Chronic tendinopathy tissue pathology, pain mechanisms, and etiology with a special focus on inflammation. Scand. J. Med. Sci. Spor. 18, 3–15. doi: 10.1111/j.1600-0838.2007.00746.x
Fung, D. T., Wang, V. M., Andarawis-Puri, N., Basta-Pljakic, J., Li, Y., Laudier, D. M., et al. (2010). Early response to tendon fatigue damage accumulation in a novel in vivo model. J. Biomech. 43, 274–279. doi: 10.1016/j.jbiomech.2009.08.039
Fung, D. T., Wang, V. M., Laudier, D. M., Shine, J. H., Basta-Pljakic, J., Jepsen, K. J., et al. (2009). Subrupture tendon fatigue damage. J. Orthop. Res. 27, 264–273. doi: 10.1002/jor.20722
Gabbett, T. J. (2016). The training—injury prevention paradox: should athletes be training smarter and harder? Br. J. Sports Med. 50, 273–280. doi: 10.1136/bjsports-2015-095788
Gentil, P., Arruda, A., Souza, D., Giessing, J., Paoli, A., Fisher, J., et al. (2017). Is there any practical application of meta-analytical results in strength training? Front. Physiol. 8:1. doi: 10.3389/fphys.2017.00001
Goldberg, A. L., Etlinger, J. D., Goldspink, D. F., and Jablecki, C. (1975). Mechanism of work-induced hypertrophy of skeletal muscle. Med. Sci. Sports 7, 185–198.
Goldspink, G. (1970). The proliferation of myofibrils during muscle fibre growth. J. Cell. Sci. 6, 593–603.
Goldspink, G. (1985). Malleability of the motor system: a comparative approach. J. Exp. Biol. 115, 375–391.
Gonzalez, A. M., Hoffman, J. R., Stout, J. R., Fukuda, D. H., and Willoughby, D. S. (2016). Intramuscular anabolic signaling and endocrine response following resistance exercise: implications for muscle hypertrophy. Sports Med. 46, 671–685. doi: 10.1007/s40279-015-0450-4
Grohmann, M., Foulstone, E., Welsh, G., Holly, J., Shield, J., Crowne, E., et al. (2005). Isolation and validation of human prepubertal skeletal muscle cells: maturation and metabolic effects of IGF-I, IGFBP-3 and TNFalpha. J. Physiol. 568, 229–242. doi: 10.1113/jphysiol.2005.093906
Häkkinen, K., Kallinen, M., Izquierdo, M., Jokelainen, K., Lassila, H., Mälki,ä, E., et al. (1998). Changes in agonist-antagonist EMG, muscle CSA, and force during strength training in middle-aged and older people. J. Appl. Physiol. 84, 1341–1349.
Hansen, K. A., Weiss, J. A., and Barton, J. K. (2002). Recruitment of tendon crimp with applied tensile strain. J. Biomech. Eng. 124, 72–77. doi: 10.1115/1.1427698
Hansen, M., and Kjaer, M. (2014). Influence of sex and estrogen on musculotendinous protein turnover at rest and after exercise. Exerc. Sport Sci. Rev. 42, 183–192. doi: 10.1249/JES.0000000000000026
Hansen, M., Kongsgaard, M., Holm, L., Skovgaard, D., Magnusson, S. P., Qvortrup, K., et al. (2009). Effect of estrogen on tendon collagen synthesis, tendon structural characteristics, and biomechanical properties in postmenopausal women. J. Appl. Physiol. 106, 1385–1393. doi: 10.1152/japplphysiol.90935.2008
Hart, J. M., Pietrosimone, B., Hertel, J., and Ingersoll, C. D. (2010). Quadriceps activation following knee injuries: a systematic review. J. Athl. Train. 45, 87–97. doi: 10.4085/1062-6050-45.1.87
Haxton, H. A. (1944). Absolute muscle force in the ankle flexors of man. J. Physiol. 103, 267–273. doi: 10.1113/jphysiol.1944.sp004075
Heinemeier, K. M., and Kjaer, M. (2011). In vivo investigation of tendon responses to mechanical loading. J. Musculoskelet. Neuronal Interact. 11, 115–123.
Heinemeier, K., Langberg, H., Olesen, J. L., and Kjaer, M. (2003). Role of TGF-beta1 in relation to exercise-induced type I collagen synthesis in human tendinous tissue. J. Appl. Physiol. 95, 2390–2397. doi: 10.1152/japplphysiol.00403.2003
Heinemeier, K. M., Bjerrum, S. S., Schjerling, P., and Kjaer, M. (2011). Expression of extracellular matrix components and related growth factors in human tendon and muscle after acute exercise. Scand. J. Med. Sci. Spor. 23, e150–e161. doi: 10.1111/j.1600-0838.2011.01414.x
Heinemeier, K. M., Olesen, J. L., Haddad, F., Langberg, H., Kjaer, M., Baldwin, K. M., et al. (2007a). Expression of collagen and related growth factors in rat tendon and skeletal muscle in response to specific contraction types. J. Physiol. 582, 1303–1316. doi: 10.1113/jphysiol.2007.127639
Heinemeier, K. M., Olesen, J. L., Schjerling, P., Haddad, F., Langberg, H., Baldwin, K. M., et al. (2007b). Short-term strength training and the expression of myostatin and IGF-I isoforms in rat muscle and tendon: differential effects of specific contraction types. J. Appl. Physiol. 102, 573–581. doi: 10.1152/japplphysiol.00866.2006
Heinemeier, K. M., Schjerling, P., Heinemeier, J., Magnusson, S. P., and Kjaer, M. (2013). Lack of tissue renewal in human adult Achilles tendon is revealed by nuclear bomb (14)C. FASEB J. 27, 2074–2079. doi: 10.1096/fj.12-225599
Helland, C., Bojsen-Møller, J., Raastad, T., Seynnes, O. R., Moltubakk, M. M., Jakobsen, V., et al. (2013). Mechanical properties of the patellar tendon in elite volleyball players with and without patellar tendinopathy. Br. J. Sports Med. 47, 862–868. doi: 10.1136/bjsports-2013-092275
Hirayama, K., Iwanuma, S., Ikeda, N., Yoshikawa, A., Ema, R., and Kawakami, Y. (2017). Plyometric training favors optimizing muscle-tendon behavior during depth jumping. Front. Physiol. 8:16. doi: 10.3389/fphys.2017.00016
Hornberger, T. A., Chu, W. K., Mak, Y. W., Hsiung, J. W., Huang, S. A., and Chien, S. (2006). The role of phospholipase D and phosphatidic acid in the mechanical activation of mTOR signaling in skeletal muscle. Proc. Natl. Acad. Sci. U.S.A. 103, 4741–4746. doi: 10.1073/pnas.0600678103
Houghton, L. A., Dawson, B. T., and Rubenson, J. (2013). Effects of plyometric training on achilles tendon properties and shuttle running during a simulated cricket batting innings. J. Strength Cond. Res. 27, 1036–1046. doi: 10.1519/JSC.0b013e3182651e7a
Ingelmark, B. E. (1945). Über den bau der sehnen während verschiedener altersperioden und unter verschiedenen funktionellen bedingungen. eine untersuchung der distalen sehnen des musculus biceps brachii und musculus semitendineus des menschen sowie der achillessehnen von kaninchen und weissen mäusen. Uppsala Läk-Fören Förh. 50, 357–395.
Ingelmark, B. E. (1948). Der bau der sehnen während verschiedener altersperioden und unter wechselnden funktionellen Bedingungen. Acta Anat. 6, 113–140. doi: 10.1159/000140347
Inhofe, P. D., Grana, W. A., Egle, D., Min, K. W., and Tomasek, J. (1995). The effects of anabolic steroids on rat tendon. An ultrastructural, biomechanical, and biochemical analysis. Am. J. Sports Med. 23, 227–232. doi: 10.1177/036354659502300217
Ippolito, E., Natali, P. G., Postacchini, F., Accinni, L., and De Martino, C. (1980). Morphological, immunochemical, and biochemical study of rabbit achilles tendon at various ages. J. Bone Joint Surg. Am. 62, 583–598. doi: 10.2106/00004623-198062040-00014
Johnson, T. L., and Klueber, K. M. (1991). Skeletal muscle following tonic overload: functional and structural analysis. Med. Sci. Sport. Exer. 23, 49–55. doi: 10.1249/00005768-199101000-00009
Jones, E. R., Jones, G. C., Legerlotz, K., and Riley, G. P. (2013). Cyclical strain modulates metalloprotease and matrix gene expression in human tenocytes via activation of TGFβ. Biochim. Biophys. Acta 1833, 2596–2607. doi: 10.1016/j.bbamcr.2013.06.019
Kameyama, T., and Etlinger, J. D. (1979). Calcium-dependent regulation of protein synthesis and degradation in muscle. Nature 279, 344–346. doi: 10.1038/279344a0
Kanehisa, H., Ikegawa, S., Tsunoda, N., and Fukunaga, T. (1995a). Strength and cross-sectional areas of reciprocal muscle groups in the upper arm and thigh during adolescence. Int. J. Sports Med. 16, 54–60. doi: 10.1055/s-2007-972964
Kanehisa, H., Yata, H., Ikegawa, S., and Fukunaga, T. (1995b). A cross-sectional study of the size and strength of the lower leg muscles during growth. Eur. J. Appl. Physiol. 72, 150–156. doi: 10.1007/BF00964130
Kannus, P. (1997). Etiology and pathophysiology of chronic tendon disorders in sports. Scand. J. Med. Sci. Spor. 7, 78–85. doi: 10.1111/j.1600-0838.1997.tb00123.x
Kastelic, J., Palley, I., and Baer, E. (1980). A structural mechanical model for tendon crimping. J. Biomech. 13, 887–893. doi: 10.1016/0021-9290(80)90177-3
Kawakami, Y., Abe, T., Kuno, S. Y., and Fukunaga, T. (1995). Training-induced changes in muscle architecture and specific tension. Eur. J. Appl. Physiol. 72, 37–43. doi: 10.1007/BF00964112
Kawakami, Y., Muraoka, T., Ito, S., Kanehisa, H., and Fukunaga, T. (2002). In vivo muscle fibre behaviour during counter-movement exercise in humans reveals a significant role for tendon elasticity. J. Physiol. 540, 635–646. doi: 10.1113/jphysiol.2001.013459
Kim, K., Hong, S., and Kim, E. Y. (2016). Reference values of skeletal muscle mass for Korean children and adolescents using data from the Korean national health and nutrition examination survey 2009–2011. PLoS ONE 11:e0153383. doi: 10.1371/journal.pone.0153383
Kjaer, M., and Heinemeier, K. M. (2014). Eccentric exercise: acute and chronic effects on healthy and diseased tendons. J. Appl. Physiol. 116, 1435–1438. doi: 10.1152/japplphysiol.01044.2013
Knörzer, E., Folkhard, W., Geercken, W., Boschert, C., Koch, M. H., Hilbert, B., et al. (1986). New aspects of the etiology of tendon rupture. An analysis of time-resolved dynamic-mechanical measurements using synchrotron radiation. Arch. Orthop. Trauma. Surg. 105, 113–120. doi: 10.1007/BF00455845
Kongsgaard, M., Aagaard, P., Kjaer, M., and Magnusson, S. P. (2005). Structural Achilles tendon properties in athletes subjected to different exercise modes and in Achilles tendon rupture patients. J. Appl. Physiol. 99, 1965–1971. doi: 10.1152/japplphysiol.00384.2005
Kongsgaard, M., Kovanen, V., Aagaard, P., Doessing, S., Hansen, P., Laursen, A. H., et al. (2009). Corticosteroid injections, eccentric decline squat training and heavy slow resistance training in patellar tendinopathy. Scand. J. Med. Sci. Spor. 19, 790–802. doi: 10.1111/j.1600-0838.2009.00949.x
Kongsgaard, M., Reitelseder, S., Pedersen, T. G., Holm, L., Aagaard, P., Kjaer, M., et al. (2007). Region specific patellar tendon hypertrophy in humans following resistance training. Acta Physiol. 191, 111–121. doi: 10.1111/j.1748-1716.2007.01714.x
Kubo, K., Ikebukuro, T., Maki, A., Yata, H., and Tsunoda, N. (2012). Time course of changes in the human Achilles tendon properties and metabolism during training and detraining in vivo. Eur. J. Appl. Physiol. 112, 2679–2691. doi: 10.1007/s00421-011-2248-x
Kubo, K., Ikebukuro, T., Yata, H., Tomita, M., and Okada, M. (2011). Morphological and mechanical properties of muscle and tendon in highly trained sprinters. J. Appl. Biomech. 27, 336–344. doi: 10.1123/jab.27.4.336
Kubo, K., Ikebukuro, T., Yata, H., Tsunoda, N., and Kanehisa, H. (2010a). Effects of training on muscle and tendon in knee extensors and plantar flexors in vivo. J. Appl. Biomech. 26, 316–323. doi: 10.1123/jab.26.3.316
Kubo, K., Ikebukuro, T., Yata, H., Tsunoda, N., and Kanehisa, H. (2010b). Time course of changes in muscle and tendon properties during strength training and detraining. J. Strength Cond. Res. 24, 322–331. doi: 10.1519/JSC.0b013e3181c865e2
Kubo, K., Kanehisa, H., and Fukunaga, T. (2002). Effects of resistance and stretching training programmes on the viscoelastic properties of human tendon structures in vivo. J. Physiol. 538, 219–226. doi: 10.1113/jphysiol.2001.012703
Kubo, K., Kanehisa, H., Ito, M., and Fukunaga, T. (2001a). Effects of isometric training on the elasticity of human tendon structures in vivo. J. Appl. Physiol. 91, 26–32.
Kubo, K., Kanehisa, H., Kawakami, Y., and Fukanaga, T. (2001b). Growth changes in the elastic properties of human tendon structures. Int. J. Sports Med. 22, 138–143. doi: 10.1055/s-2001-11337
Kubo, K., Morimoto, M., Komuro, T., Yata, H., Tsunoda, N., Kanehisa, H., et al. (2007). Effects of plyometric and weight training on muscle-tendon complex and jump performance. Med. Sci. Sport. Exer. 39, 1801–1810. doi: 10.1249/mss.0b013e31813e630a
Kubo, K., Teshima, T., Hirose, N., and Tsunoda, N. (2014). Growth changes in morphological and mechanical properties of human patellar tendon in vivo. J. Appl. Biomech. 30, 415–422. doi: 10.1123/jab.2013-0220
Kumar, V., Atherton, P., Smith, K., and Rennie, M. J. (2009). Human muscle protein synthesis and breakdown during and after exercise. J. Appl. Physiol. 106, 2026–2039. doi: 10.1152/japplphysiol.91481.2008
LaCroix, A. S., Duenwald-Kuehl, S. E., Lakes, R. S., and Vanderby, R. (2013). Relationship between tendon stiffness and failure: a metaanalysis. J. Appl. Physiol. 115, 43–51. doi: 10.1152/japplphysiol.01449.2012
Laitinen, O. (1967). The metabolism of collagen and its hormonal control in the rat with special emphasis on the interactions of collagen and calcium in the bones. Acta Endocrinol. 56(Suppl. 120), 1–86.
Langberg, H., Rosendal, L., and Kjaer, M. (2001). Training-induced changes in peritendinous type I collagen turnover determined by microdialysis in humans. J. Physiol. 534, 297–302. doi: 10.1111/j.1469-7793.2001.00297.x
Langberg, H., Skovgaard, D., Petersen, L. J., Bulow, J., and Kjaer, M. (1999). Type I collagen synthesis and degradation in peritendinous tissue after exercise determined by microdialysis in humans. J. Physiol. 521(Pt 1), 299–306. doi: 10.1111/j.1469-7793.1999.00299.x
Lavagnino, M., Arnoczky, S. P., Egerbacher, M., Gardner, K. L., and Burns, M. E. (2006). Isolated fibrillar damage in tendons stimulates local collagenase mRNA expression and protein synthesis. J. Biomech. 39, 2355–2362. doi: 10.1016/j.jbiomech.2005.08.008
Lavagnino, M., Arnoczky, S. P., Kepich, E., Caballero, O., and Haut, R. C. (2008). A finite element model predicts the mechanotransduction response of tendon cells to cyclic tensile loading. Biomech. Model. Mechanobiol. 7, 405–416. doi: 10.1007/s10237-007-0104-z
Lavagnino, M., Arnoczky, S. P., Tian, T., and Vaupel, Z. (2003). Effect of amplitude and frequency of cyclic tensile strain on the inhibition of MMP-1 mRNA expression in tendon cells: an in vitro study. Connect. Tissue Res. 44, 181–187. doi: 10.1080/03008200390215881
Lavagnino, M., Wall, M. E., Little, D., Banes, A. J., Guilak, F., and Arnoczky, S. P. (2015). Tendon mechanobiology: current knowledge and future research opportunities. J. Orthop. Res. 33, 813–822. doi: 10.1002/jor.22871
Le Gall, F., Carling, C., Reilly, T., Vandewalle, H., Church, J., and Rochcongar, P. (2006). Incidence of injuries in elite French youth soccer players: a 10-season study. Am. J. Sports Med. 34, 928–938. doi: 10.1177/0363546505283271
Legerlotz, K. (2013). Rehabilitation of tendopathies in human athletes. Comp. Exerc. Physiol. 9, 153–160. doi: 10.3920/CEP13030
Legerlotz, K., Jones, G. C., Screen, H. R., and Riley, G. P. (2013). Cyclic loading of tendon fascicles using a novel fatigue loading system increases interleukin-6 expression by tenocytes. Scand. J. Med. Sci. Sports 23, 31–37. doi: 10.1111/j.1600-0838.2011.01410.x
Lexell, J., Sjöström, M., Nordlund, A. S., and Taylor, C. C. (1992). Growth and development of human muscle: a quantitative morphological study of whole vastus lateralis from childhood to adult age. Muscle Nerve 15, 404–409. doi: 10.1002/mus.880150323
Lian, O. B., Engebretsen, L., and Bahr, R. (2005). Prevalence of jumper's knee among elite athletes from different sports: a cross-sectional study. Am. J. Sports Med. 33, 561–567. doi: 10.1177/0363546504270454
Lichtwark, G. A., and Wilson, A. M. (2007). Is Achilles tendon compliance optimised for maximum muscle efficiency during locomotion? J. Biomech. 40, 1768–1775. doi: 10.1016/j.jbiomech.2006.07.025
Loitz, B. J., Zernicke, R. F., Vailas, A. C., Kody, M. H., and Meals, R. A. (1989). Effects of short-term immobilization versus continuous passive motion on the biomechanical and biochemical properties of the rabbit tendon. Clin. Orthop. Relat. Res. 265–271.
Lundholm, K., Edström, S., Ekman, L., Karlberg, I., Walker, P., and Scherstén, T. (1981). Protein degradation in human skeletal muscle tissue: the effect of insulin, leucine, amino acids and ions. Clin. Sci. 60, 319–326. doi: 10.1042/cs0600319
Lynn, R., and Morgan, D. L. (1994). Decline running produces more sarcomeres in rat vastus intermedius muscle fibers than does incline running. J. Appl. Physiol. 77, 1439–1444.
Magnusson, S. P., and Kjaer, M. (2003). Region-specific differences in Achilles tendon cross-sectional area in runners and non-runners. Eur. J. Appl. Physiol. 90, 549–553. doi: 10.1007/s00421-003-0865-8
Magnusson, S. P., Langberg, H., and Kjaer, M. (2010). The pathogenesis of tendinopathy: balancing the response to loading. Nat. Rev. Rheumatol. 6, 262–268. doi: 10.1038/nrrheum.2010.43
Magnusson, S. P., Narici, M. V., Maganaris, C. N., and Kjaer, M. (2007). Human tendon behaviour and adaptation, in vivo. J. Physiol. 586, 71–81. doi: 10.1113/jphysiol.2007.139105
Malina, R. M., Bouchard, C., and Bar-Or, O. (2004). Growth, Maturation, and Physical Activity. Champaign, IL: Human Kinetics.
Malliaras, P., Barton, C. J., Reeves, N. D., and Langberg, H. (2013a). Achilles and patellar tendinopathy loading programmes: a systematic review comparing clinical outcomes and identifying potential mechanisms for effectiveness. Sports Med. 43, 267–286. doi: 10.1007/s40279-013-0019-z
Malliaras, P., Kamal, B., Nowell, A., Farley, T., Dhamu, H., Simpson, V., et al. (2013b). Patellar tendon adaptation in relation to load-intensity and contraction type. J. Biomech. 46, 1893–1899. doi: 10.1016/j.jbiomech.2013.04.022
Marqueti, R. C., Parizotto, N. A., Chriguer, R. S., Perez, S. E., and Selistre-de-Araujo, H. S. (2006). Androgenic-anabolic steroids associated with mechanical loading inhibit matrix metallopeptidase activity and affect the remodeling of the Achilles tendon in rats. Am. J. Sports Med. 34, 1274–1280. doi: 10.1177/0363546506286867
Marqueti, R. C., Prestes, J., Wang, C. C., Ramos, O. H., Perez, S. E. A., Nakagaki, W. R., et al. (2011). Biomechanical responses of different rat tendons to nandrolone decanoate and load exercise. Scand. J. Med. Sci. Spor. 21, e91–e99. doi: 10.1111/j.1600-0838.2010.01162.x
McCarthy, H. D., Samani-Radia, D., Jebb, S. A., and Prentice, A. M. (2014). Skeletal muscle mass reference curves for children and adolescents. Pediatr. Obes. 9, 249–259. doi: 10.1111/j.2047-6310.2013.00168.x
McDougall, J. D., Elder, G., Sale, D. G., and Moroz, J. R. (1980). Effects of strength training and immobilization on human muscle fibers. Eur. J. Appl. Physiol. 43, 25–34. doi: 10.1007/BF00421352
McGlory, C., Devries, M. C., and Phillips, S. M. (2017). Skeletal muscle and resistance exercise training; the role of protein synthesis in recovery and remodeling. J. Appl. Physiol. 122, 541–548. doi: 10.1152/japplphysiol.00613.2016
Mersmann, F., Bohm, S., Schroll, A., Boeth, H., Duda, G., and Arampatzis, A. (2014). Evidence of imbalanced adaptation between muscle and tendon in adolescent athletes. Scand. J. Med. Sci. Spor. 24, E283–E289. doi: 10.1111/sms.12166
Mersmann, F., Bohm, S., Schroll, A., Boeth, H., Duda, G. N., and Arampatzis, A. (2017a). Muscle and tendon adaptation in adolescent athletes: a longitudinal study. Scand. J. Med. Sci. Spor. 27, 75–82. doi: 10.1111/sms.12631
Mersmann, F., Bohm, S., Schroll, A., Marzilger, R., and Arampatzis, A. (2016). Athletic training affects the uniformity of muscle and tendon adaptation during adolescence. J. Appl. Physiol. 121, 893–899. doi: 10.1152/japplphysiol.00493.2016
Mersmann, F., Charcharis, G., Bohm, S., and Arampatzis, A. (2017b). Muscle and tendon adaptation in adolescence: elite volleyball athletes compared to untrained boys and girls. Front. Physiol. 8:417. doi: 10.3389/fphys.2017.00417
Miller, B. F., Hansen, M., Olesen, J. L., Schwarz, P., Babraj, J. A., Smith, K., et al. (2007). Tendon collagen synthesis at rest and after exercise in women. J. Appl. Physiol. 102, 541–546. doi: 10.1152/japplphysiol.00797.2006
Miller, B. F., Olesen, J. L., Hansen, M., Døssing, S., Crameri, R. M., Welling, R. J., et al. (2005). Coordinated collagen and muscle protein synthesis in human patella tendon and quadriceps muscle after exercise. J. Physiol. 567, 1021–1033. doi: 10.1113/jphysiol.2005.093690
Miller, K. S., Connizzo, B. K., and Soslowsky, L. J. (2012). Collagen fiber re-alignment in a neonatal developmental mouse supraspinatus tendon model. Ann. Biomed. Eng. 40, 1102–1110. doi: 10.1007/s10439-011-0490-3
Mitchell, C. J., Churchward-Venne, T. A., West, D. W. D., Burd, N. A., Breen, L., Baker, S. K., et al. (2012). Resistance exercise load does not determine training-mediated hypertrophic gains in young men. J. Appl. Physiol. 113, 71–77. doi: 10.1152/japplphysiol.00307.2012
Morse, C. I., Tolfrey, K., Thom, J. M., Vassilopoulos, V., Maganaris, C. N., and Narici, M. V. (2008). Gastrocnemius muscle specific force in boys and men. J. Appl. Physiol. 104, 469–474. doi: 10.1152/japplphysiol.00697.2007
Moss, B. M., Refsnes, P. E., Abildgaard, A., Nicolaysen, K., and Jensen, J. (1997). Effects of maximal effort strength training with different loads on dynamic strength, cross-sectional area, load-power and load-velocity relationships. Eur. J. Appl. Physiol. 75, 193–199. doi: 10.1007/s004210050147
Murray, P. G., and Clayton, P. E. (2013). Endocrine control of growth. Am. J. Med. Genet. C Semin. Med. Genet. 163C, 76–85. doi: 10.1002/ajmg.c.31357
Neu, C. M., Rauch, F., Rittweger, J., Manz, F., and Schoenau, E. (2002). Influence of puberty on muscle development at the forearm. Am. J. Physiol. Endocrinol. Metab. 283, E103–E107. doi: 10.1152/ajpendo.00445.2001
Neugebauer, J. M., and Hawkins, D. A. (2012). Identifying factors related to Achilles tendon stress, strain, and stiffness before and after 6 months of growth in youth 10-14 years of age. J. Biomech. 45, 2457–2461. doi: 10.1016/j.jbiomech.2012.06.027
Neviaser, A., Andarawis-Puri, N., and Flatow, E. (2012). Basic mechanisms of tendon fatigue damage. J. Shoulder Elbow Surg. 21, 158–163. doi: 10.1016/j.jse.2011.11.014
Nielsen, R. H., Clausen, N. M., Schjerling, P., Larsen, J. O., Martinussen, T., List, E. O., et al. (2014). Chronic alterations in growth hormone/insulin-like growth factor-I signaling lead to changes in mouse tendon structure. Matrix Biol. 34, 96–104. doi: 10.1016/j.matbio.2013.09.005
Nigg, B. M., and Herzog, W. (2007). Biomechanics of the Musculo-Skeletal System, 3rd Edn. Chichester: Wiley.
Nikolaidou, M. E., Marzilger, R., Bohm, S., Mersmann, F., and Arampatzis, A. (2017). Operating length and velocity of human M. vastus lateralis fascicles during vertical jumping. R. Soc. Open Sci. 4:170185. doi: 10.1098/rsos.170185
O'Brien, T. D., Reeves, N. D., Baltzopoulos, V., Jones, D. A., and Maganaris, C. N. (2009). Moment arms of the knee extensor mechanism in children and adults. J. Anat. 215, 198–205. doi: 10.1111/j.1469-7580.2009.01088.x
O'Brien, T. D., Reeves, N. D., Baltzopoulos, V., Jones, D. A., and Maganaris, C. N. (2010a). In vivo measurements of muscle specific tension in adults and children. Exp. Physiol. 95, 202–210. doi: 10.1113/expphysiol.2009.048967
O'Brien, T. D., Reeves, N. D., Baltzopoulos, V., Jones, D. A., and Maganaris, C. N. (2010b). Mechanical properties of the patellar tendon in adults and children. J. Biomech. 43, 1190–1195. doi: 10.1016/j.jbiomech.2009.11.028
O'Brien, T. D., Reeves, N. D., Baltzopoulos, V., Jones, D. A., and Maganaris, C. N. (2010c). Muscle-tendon structure and dimensions in adults and children. J. Anat. 216, 631–642. doi: 10.1111/j.1469-7580.2010.01218.x
Oertel, G. (1988). Morphometric analysis of normal skeletal muscles in infancy, childhood and adolescence. An autopsy study. J. Neurol. Sci. 88, 303–313. doi: 10.1016/0022-510X(88)90227-4
Olesen, J. L., Heinemeier, K. M., Haddad, F., Langberg, H., Flyvbjerg, A., Kjaer, M., et al. (2006). Expression of insulin-like growth factor I, insulin-like growth factor binding proteins, and collagen mRNA in mechanically loaded plantaris tendon. J. Appl. Physiol. 101, 183–188. doi: 10.1152/japplphysiol.00636.2005
Oliva, F., Berardi, A. C., Misiti, S., Verga Falzacappa, C., Falzacappa, C. V., Iacone, A., et al. (2013). Thyroid hormones enhance growth and counteract apoptosis in human tenocytes isolated from rotator cuff tendons. Cell Death Dis. 4:e705. doi: 10.1038/cddis.2013.229
Ozaki, H., Loenneke, J. P., Buckner, S. L., and Abe, T. (2016). Muscle growth across a variety of exercise modalities and intensities: contributions of mechanical and metabolic stimuli. Med. Hypotheses 88, 22–26. doi: 10.1016/j.mehy.2015.12.026
Palmieri-Smith, R. M., Villwock, M., Downie, B., Hecht, G., and Zernicke, R. (2013). Pain and effusion and quadriceps activation and strength. J. Athl. Train. 48, 186–191. doi: 10.4085/1062-6050-48.2.10
Pansarasa, O., Rinaldi, C., Parente, V., Miotti, D., Capodaglio, P., and Bottinelli, R. (2009). Resistance training of long duration modulates force and unloaded shortening velocity of single muscle fibres of young women. J. Electromyogr. Kinesiol. 19, e290–e300. doi: 10.1016/j.jelekin.2008.07.007
Pärssinen, M., Karila, T., Kovanen, V., and Seppälä, T. (2000). The effect of supraphysiological doses of anabolic androgenic steroids on collagen metabolism. Int. J. Sports Med. 21, 406–411. doi: 10.1055/s-2000-3834
Peacock, E. E. (1959). A study of the circulation in normal tendons and healing grafts. Ann. Surg. 149, 415–428. doi: 10.1097/00000658-195903000-00011
Peñailillo, L., Blazevich, A. J., and Nosaka, K. (2015). Muscle fascicle behavior during eccentric cycling and its relation to muscle soreness. Med. Sci. Sport. Exer. 47, 708–717. doi: 10.1249/MSS.0000000000000473
Perrone, C. E., Fenwick-Smith, D., and Vandenburgh, H. H. (1995). Collagen and stretch modulate autocrine secretion of insulin-like growth factor-1 and insulin-like growth factor binding proteins from differentiated skeletal muscle cells. J. Biol. Chem. 270, 2099–2106. doi: 10.1074/jbc.270.5.2099
Peters, J. A., Zwerver, J., Diercks, R. L., Elferink-Gemser, M. T., and van den Akker-Scheek, I. (2016). Preventive interventions for tendinopathy: a systematic review. J. Sci. Med. Sport 19, 205–211. doi: 10.1016/j.jsams.2015.03.008
Phillips, S. M., Tipton, K. D., Aarsland, A., Wolf, S. E., and Wolfe, R. R. (1997). Mixed muscle protein synthesis and breakdown after resistance exercise in humans. Am. J. Physiol. 273, E99–E107.
Potier, T. G., Alexander, C. M., and Seynnes, O. R. (2009). Effects of eccentric strength training on biceps femoris muscle architecture and knee joint range of movement. Eur. J. Appl. Physiol. 105, 939–944. doi: 10.1007/s00421-008-0980-7
Reeves, N. D., Maganaris, C. N., Longo, S., and Narici, M. V. (2009). Differential adaptations to eccentric versus conventional resistance training in older humans. Exp. Physiol. 94, 825–833. doi: 10.1113/expphysiol.2009.046599
Rennie, M. J., Edwards, R. H., Halliday, D., Matthews, D. E., Wolman, S. L., and Millward, D. J. (1982). Muscle protein synthesis measured by stable isotope techniques in man: the effects of feeding and fasting. Clin. Sci. 63, 519–523. doi: 10.1042/cs0630519
Roberts, T. J. (1997). Muscular force in running Turkeys: the economy of minimizing work. Science 275, 1113–1115. doi: 10.1126/science.275.5303.1113
Rooney, K. J., Herbert, R. D., and Balnave, R. J. (1994). Fatigue contributes to the strength training stimulus. Med. Sci. Sport. Exer. 26, 1160–1164.
Rosager, S., Aagaard, P., Dyhre-Poulsen, P., Neergaard, K., Kjaer, M., and Magnusson, S. P. (2002). Load-displacement properties of the human triceps surae aponeurosis and tendon in runners and non-runners. Scand. J. Med. Sci. Spor. 12, 90–98. doi: 10.1034/j.1600-0838.2002.120205.x
Round, J. M., Jones, D. A., Honour, J. W., and Nevill, A. M. (1999). Hormonal factors in the development of differences in strength between boys and girls during adolescence: a longitudinal study. Ann. Hum. Biol. 26, 49–62. doi: 10.1080/030144699282976
Sackin, H. (1995). Mechanosensitive channels. Annu. Rev. Physiol. 57, 333–353. doi: 10.1146/annurev.ph.57.030195.002001
Sáez-Sáez de Villarreal, E., Requena, B., and Newton, R. U. (2010). Does plyometric training improve strength performance? A meta-analysis. J. Sci. Med. Sport 13, 513–522. doi: 10.1016/j.jsams.2009.08.005
Schechtman, H., and Bader, D. L. (2002). Fatigue damage of human tendons. J. Biomech. 35, 347–353. doi: 10.1016/S0021-9290(01)00177-4
Schoenfeld, B. J. (2013). Potential mechanisms for a role of metabolic stress in hypertrophic adaptations to resistance training. Sports Med. 43, 179–194. doi: 10.1007/s40279-013-0017-1
Schoenfeld, B. J., Peterson, M. D., Ogborn, D., Contreras, B., and Sonmez, G. T. (2015). Effects of Low- vs. high-load resistance training on muscle strength and hypertrophy in well-trained men. J. Strength Cond. Res. 29, 2954–2963. doi: 10.1519/JSC.0000000000000958
Schoenfeld, B. J., Wilson, J. M., Lowery, R. P., and Krieger, J. W. (2016). Muscular adaptations in low- versus high-load resistance training: a meta-analysis. Eur. J. Sport Sci. 16, 1–10. doi: 10.1080/17461391.2014.989922
Schott, J., McCully, K., and Rutherford, O. M. (1995). The role of metabolites in strength training. II. Short versus long isometric contractions. Eur. J. Appl. Physiol. 71, 337–341. doi: 10.1007/BF00240414
Seynnes, O. R., de Boer, M., and Narici, M. V. (2007). Early skeletal muscle hypertrophy and architectural changes in response to high-intensity resistance training. J. Appl. Physiol. 102, 368–373. doi: 10.1152/japplphysiol.00789.2006
Seynnes, O. R., Erskine, R. M., Maganaris, C. N., Longo, S., Simoneau, E. M., Grosset, J. F., et al. (2009). Training-induced changes in structural and mechanical properties of the patellar tendon are related to muscle hypertrophy but not to strength gains. J. Appl. Physiol. 107, 523–530. doi: 10.1152/japplphysiol.00213.2009
Seynnes, O. R., Kamandulis, S., Kairaitis, R., Helland, C., Campbell, E. L., Brazaitis, M., et al. (2013). Effect of androgenic-anabolic steroids and heavy strength training on patellar tendon morphological and mechanical properties. J. Appl. Physiol. 115, 84–89. doi: 10.1152/japplphysiol.01417.2012
Sharifnezhad, A., Marzilger, R., and Arampatzis, A. (2014). Effects of load magnitude, muscle length and velocity during eccentric chronic loading on the longitudinal growth of the vastus lateralis muscle. J. Exp. Biol. 217, 2726–2733. doi: 10.1242/jeb.100370
Shepherd, J. H., and Screen, H. R. (2013). Fatigue loading of tendon. Int. J. Exp. Pathol. 94, 260–270. doi: 10.1111/iep.12037
Simpson, M., Rio, E., and Cook, J. (2016). At what age do children and adolescents develop lower limb tendon pathology or tendinopathy? A systematic review and meta-analysis. Sports Med. 46, 545–557. doi: 10.1007/s40279-015-0438-0
Smith, J. W. (1965). Blood supply of tendons. Am. J. Surg. 109, 272–276. doi: 10.1016/S0002-9610(65)80073-3
Smith, R. C., and Rutherford, O. M. (1995). The role of metabolites in strength training. I. A comparison of eccentric and concentric contractions. Eur. J. Appl. Physiol. 71, 332–336. doi: 10.1007/BF00240413
Stafilidis, S., and Arampatzis, A. (2007). Muscle - tendon unit mechanical and morphological properties and sprint performance. J. Sports Sci. 25, 1035–1046. doi: 10.1080/02640410600951589
Stracciolini, A., Casciano, R., Levey Friedman, H., Stein, C. J., Meehan, W. P., and Micheli, L. J. (2014). Pediatric sports injuries: a comparison of males versus females. Am. J. Sports Med. 42, 965–972. doi: 10.1177/0363546514522393
Tanimoto, M., and Ishii, N. (2006). Effects of low-intensity resistance exercise with slow movement and tonic force generation on muscular function in young men. J. Appl. Physiol. 100, 1150–1157. doi: 10.1152/japplphysiol.00741.2005
Thorpe, C. T., Streeter, I., Pinchbeck, G. L., Goodship, A. E., Clegg, P. D., and Birch, H. L. (2010). Aspartic acid racemization and collagen degradation markers reveal an accumulation of damage in tendon collagen that is enhanced with aging. J. Biol. Chem. 285, 15674–15681. doi: 10.1074/jbc.M109.077503
Tidball, J. G. (2005). Mechanical signal transduction in skeletal muscle growth and adaptation. J. Appl. Physiol. 98, 1900–1908. doi: 10.1152/japplphysiol.01178.2004
Toigo, M., and Boutellier, U. (2006). New fundamental resistance exercise determinants of molecular and cellular muscle adaptations. Eur. J. Appl. Physiol. 97, 643–663. doi: 10.1007/s00421-006-0238-1
Tonson, A., Ratel, S., Le Fur, Y., Cozzone, P., and Bendahan, D. (2008). Effect of maturation on the relationship between muscle size and force production. Med. Sci. Sport. Exer. 40, 918–925. doi: 10.1249/MSS.0b013e3181641bed
Tsitsilonis, S., Panayiotis, C. E., Athanasios, M. S., Stavros, K. K., Ioannis, V. S., George, A., et al. (2014). Anabolic androgenic steroids reverse the beneficial effect of exercise on tendon biomechanics: an experimental study. Foot Ankle Surg. 20, 94–99. doi: 10.1016/j.fas.2013.12.001
Urlando, A., and Hawkins, D. (2007). Achilles tendon adaptation during strength training in young adults. Med. Sci. Sport. Exer. 39, 1147–1152. doi: 10.1249/mss.0b013e31805371d1
Vandenburgh, H., and Kaufman, S. (1979). In vitro model for stretch-induced hypertrophy of skeletal muscle. Science 203, 265–268. doi: 10.1126/science.569901
Veres, S. P., Harrison, J. M., and Lee, J. M. (2013). Repeated subrupture overload causes progression of nanoscaled discrete plasticity damage in tendon collagen fibrils. J. Orthop. Res. 31, 731–737. doi: 10.1002/jor.22292
Vingren, J. L., Kraemer, W. J., Ratamess, N. A., Anderson, J. M., Volek, J. S., and Maresh, C. M. (2010). Testosterone physiology in resistance exercise and training: the up-stream regulatory elements. Sports Med. 40, 1037–1053. doi: 10.2165/11536910-000000000-00000
Visnes, H., Aandahl, H. Å., and Bahr, R. (2013). Jumper's knee paradox–jumping ability is a risk factor for developing jumper's knee: a 5-year prospective study. Br. J. Sports Med. 47, 503–507. doi: 10.1136/bjsports-2012-091385
Wang, J. (2006). Mechanobiology of tendon. J. Biomech. 39, 1563–1582. doi: 10.1016/j.jbiomech.2005.05.011
Waugh, C. M., Alktebi, T., de Sa, A., and Scott, A. (2017). Impact of rest duration on Achilles tendon structure and function following isometric training. Scand. J. Med. Sci. Spor. doi: 10.1111/sms.12930. [Epub ahead of print].
Waugh, C. M., Blazevich, A. J., Fath, F., and Korff, T. (2012). Age-related changes in mechanical properties of the Achilles tendon. J. Anat. 220, 144–155. doi: 10.1111/j.1469-7580.2011.01461.x
Waugh, C. M., Korff, T., Fath, F., and Blazevich, A. J. (2013). Rapid force production in children and adults. Med. Sci. Sport. Exer. 45, 762–771. doi: 10.1249/MSS.0b013e31827a67ba
Waugh, C. M., Korff, T., Fath, F., and Blazevich, A. J. (2014). Effects of resistance training on tendon mechanical properties and rapid force production in prepubertal children. J. Appl. Physiol. 117, 257–266. doi: 10.1152/japplphysiol.00325.2014
Wernbom, M., Augustsson, J., and Thomeé, R. (2007). The influence of frequency, intensity, volume and mode of strength training on whole muscle cross-sectional area in humans. Sports Med. 37, 225–264. doi: 10.2165/00007256-200737030-00004
Widrick, J. J., Stelzer, J. E., Shoepe, T. C., and Garner, D. P. (2002). Functional properties of human muscle fibers after short-term resistance exercise training. Am. J. Physiol. 283, R408–R416. doi: 10.1152/ajpregu.00120.2002
Wiesinger, H. P., Kösters, A., Müller, E., and Seynnes, O. R. (2015). Effects of increased loading on in vivo tendon properties: a systematic review. Med. Sci. Sports Exerc. 47, 1885–1895. doi: 10.1249/MSS.0000000000000603
Woo, S. L. (1982). Mechanical properties of tendons and ligaments. I. Quasi-static and nonlinear viscoelastic properties. Biorheology 19, 385–396. doi: 10.3233/BIR-1982-19301
Wren, T. A. L., Lindsey, D. P., Beaupré, G. S., and Carter, D. R. (2003). Effects of creep and cyclic loading on the mechanical properties and failure of human Achilles tendons. Ann. Biomed. Eng. 31, 710–717. doi: 10.1114/1.1569267
Wu, Y. K., Lien, Y. H., Lin, K. H., Shih, T. T., Wang, T. G., and Wang, H. K. (2010). Relationships between three potentiation effects of plyometric training and performance. Scand. J. Med. Sci. Spor. 20, e80–e86. doi: 10.1111/j.1600-0838.2009.00908.x
Zhang, J., Pan, T., Liu, Y., and Wang, J. H. C. (2010). Mouse treadmill running enhances tendons by expanding the pool of tendon stem cells (TSCs) and TSC-related cellular production of collagen. J. Orthop. Res. 28, 1178–1183. doi: 10.1002/jor.21123
Keywords: muscle, tendon, adaptation, athletes, adolescence, tendinopathy, imbalance
Citation: Mersmann F, Bohm S and Arampatzis A (2017) Imbalances in the Development of Muscle and Tendon as Risk Factor for Tendinopathies in Youth Athletes: A Review of Current Evidence and Concepts of Prevention. Front. Physiol. 8:987. doi: 10.3389/fphys.2017.00987
Received: 16 August 2017; Accepted: 17 November 2017;
Published: 01 December 2017.
Edited by:
Kimberly Huey, Drake University, United StatesReviewed by:
Matthew M. Robinson, Oregon State University, United StatesHans-Peter Wiesinger, University of Salzburg, Austria
David Hawkins, University of California, Davis, United States
Copyright © 2017 Mersmann, Bohm and Arampatzis. This is an open-access article distributed under the terms of the Creative Commons Attribution License (CC BY). The use, distribution or reproduction in other forums is permitted, provided the original author(s) or licensor are credited and that the original publication in this journal is cited, in accordance with accepted academic practice. No use, distribution or reproduction is permitted which does not comply with these terms.
*Correspondence: Adamantios Arampatzis, YS5hcmFtcGF0emlzQGh1LWJlcmxpbi5kZQ==