- 1Dorothy M. Davis Heart and Lung Research Institute, Wexner Medical Center, The Ohio State University, Columbus, OH, United States
- 2Department of Physiology & Cell Biology, Wexner Medical Center, The Ohio State University, Columbus, OH, United States
- 3Department of Internal Medicine, Division of Cardiovascular Medicine, College of Medicine, Wexner Medical Center, The Ohio State University, Columbus, OH, United States
Ankyrins are adaptor proteins critical for the expression and targeting of cardiac membrane proteins, signaling molecules, and cytoskeletal elements. Findings in humans and animal models have highlighted the in vivo roles for ankyrins in normal physiology and in cardiovascular disease, most notably in cardiac arrhythmia. For example, human ANK2 loss-of-function variants are associated with a complex array of electrical and structural phenotypes now termed “ankyrin-B syndrome,” whereas alterations in the ankyrin-G pathway for Nav channel targeting are associated with human Brugada syndrome. Further, both ankyrin-G and -B are now linked with acquired forms of cardiovascular disease including myocardial infarction and atrial fibrillation. Spectrins are ankyrin-associated proteins and recent studies support the critical role of ankyrin-spectrin interactions in normal cardiac physiology as well as regulation of key ion channel and signaling complexes. This review will highlight the roles of ankyrins and spectrins in cardiovascular physiology as well as illustrate the link between the dysfunction in ankyrin- and spectrin-based pathways and disease.
Introduction
Cardiovascular disease is the leading cause of death in the United States (Rosamond et al., 2008). In 2013, the overall prevalence of death attributable to cardiovascular diseases (CVD) was 222.9 per 100,000 Americans. On the basis of 2013 data, more than 2,200 Americans die of CVD each day, representing an average of 1 death every 40 s. Arrhythmia is the direct cause of death in many cases. Nearly 80% of cases of sudden cardiac death are the result of ventricular arrhythmias (Mehra, 2007). Thus, understanding the molecular mechanisms underlying human arrhythmias has been an active area of cardiovascular research (Cunha and Mohler, 2006), with a major focus on ion channels, transporters and receptors that regulate the action potential at baseline and in disease. Ankyrins and spectrins are adaptor proteins now linked with both electrical and structural forms of heart disease. Here, we will review the roles of ankyrin and spectrin polypeptides as critical components of cardiac myocytes using findings from human and animal models.
Ankyrin Genes
Ankyrins are a family of intracellular adaptor proteins that organize and anchor integral membrane protein complexes to the spectrin- and actin-based cytoskeleton (Bennett and Baines, 2001). In vertebrates, three genes (ANK1, ANK2, ANK3), encode ankyrin polypeptides. ANK1 is located on human chromosome 8p11 and encodes ankyrin-R polypeptides (Lambert et al., 1990). ANK2 is located on human chromosome 4q25-27 and encodes ankyrin-B polypeptides (Otto et al., 1991). ANK3 is located on human chromosome 10q21 and encodes ankyrin-G polypeptides (Kordeli et al., 1995). Alternative splicing produces a series of ankyrin gene products with unique subcellular distributions and functional properties (Bennett and Baines, 2001; Cunha and Mohler, 2006; Cunha et al., 2008). For example, alternative splicing of ANK2 results in 440 kDa and 220 kDa ankyrin-B (Kunimoto, 1995; Hashemi et al., 2009). Ankyrins R, B, and G polypeptides have been identified in ventricular myocytes (Li et al., 1993; Mohler et al., 2002, 2004a,b). However, the full extent of alternative splicing, expression, localization, and function is still under investigation.
Ankyrin Domains and Binding Partners
Canonical ankyrins have four domains: a membrane-binding domain (MBD), a spectrin-binding domain (SBD), a death domain (DD) and a C-terminal domain (CTD) (Mohler, 2006). Together, DD and CTD encompass the “regulatory domain” (RD). Ankyrin-B and ankyrin-G are closely related in amino acid sequence with 67% amino acid identity between the MBD, SBD, and DD. Despite this homology and shared expression in cardiac myocytes, ankyrin-B and ankyrin-G maintain differential distributions and non-overlapping functions. For example, while ankyrin-B is required for the localization of the Na/K-ATPase and Na/Ca exchanger (NCX) (Figure 1) to transverse-tubule membranes, ankyrin-G is required for targeting Nav1.5 to the intercalated disc (Mohler et al., 2004a; Makara et al., 2014; Wu et al., 2015).
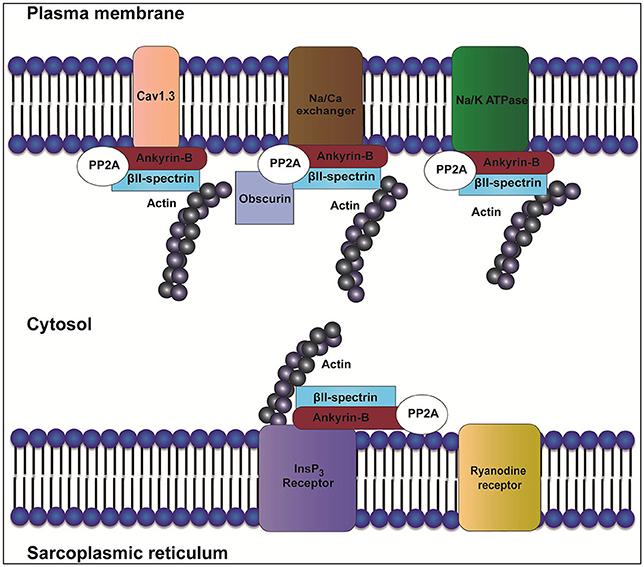
Figure 1. Role of ankyrin-B in localization of the InsP3 receptor, Na/K-ATPase, Cav1.3, and NCX. In heart, ankyrin-B targets and localizes ion channels and transporters such as inositol trisphosphate receptor (InsP3R), sodium/potassium ATPase (Na/K-ATPase), Cav1.3, and Na/Ca exchanger (NCX). Ankyrin-B also targets protein phosphatase type 2A (PP2A) through its regulatory subunit B56α. Interaction between ankyrin-B and βII spectrin forms a complex that is important for the localization and stability of ion channels and transporters such as Na/K-ATPase, Cav1.3, InsP3R, and NCX.
The MBD is comprised of 24 consecutive ANK repeats. In heart, this domain is essential for the interaction with ion channels and transporters (Figure 2) including the Na/K-ATPase (Koob et al., 1988), NCX (Li et al., 1993), voltage-gated Nav channel (Mohler et al., 2004a), the inward rectifier subunit (Kir6.2) (Li et al., 2010), voltage-gated Ca2+ channels (Cav1.3) (Cunha et al., 2011), and inositol trisphosphate receptor (InsP3 receptor) (Bourguignon et al., 1993; Hortsch et al., 2009). MBD binds to a variety of cell adhesion molecules including members of the L1 family (Davis et al., 1993). The impact of these interactions in the heart is not well studied, but an important area for future research.
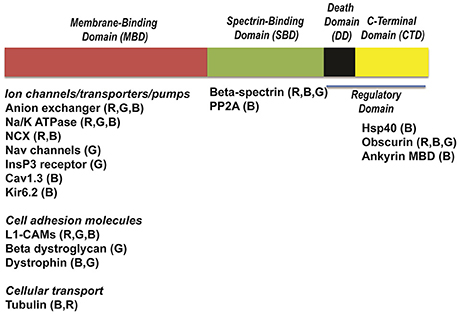
Figure 2. Structure of ankyrins and ankyrin-binding partners. Ankyrins are formed of four distinct domains: a membrane-binding domain (MBD), a spectrin-binding domain (SBD), a death domain (DD) and a C-terminal domain (CTD). Each domain interacts with distinct ion channels, transporters and pumps. Cav1.3, calcium channel, voltage-dependent, L type, alpha 1D subunit; Na/K-ATPase, sodium/potassium ATPase; NCX, Na/Ca exchanger; InsP3R, Inositol trisphosphate receptor; Kir6.2, Inward-rectifier potassium ion channel; Nav channels, voltage-gated sodium channels; L1-CAMs, The L1 family of neural cell adhesion molecules; PP2A, protein phosphatase type 2A; Hsp40, Heat shock protein 40.
The SBD associates with high affinity to β spectrin polypeptides (Bennett and Stenbuck, 1979) via the N-terminal ZU5 (Zu5N) domain (Ipsaro and Mondragon, 2010). This interaction is critical for a number of physiological functions including maintenance of normal erythrocyte cell membrane stability (Bodine et al., 1984). As described later, human ANK2 variants that alter spectrin-binding are now linked with potentially fatal forms of cardiac arrhythmia (Smith et al., 2015). The SBD also interacts with signaling molecules such as B56α, the regulatory subunit of protein phosphatase 2A (PP2A) (Bhasin et al., 2007; Little et al., 2015).
The ankyrin regulatory domain (RD) interacts with proteins including obscurin. Obscurin is a giant scaffolding protein with key roles in muscle myosin incorporation, A-band formation and lateral alignment of M-lines in adjacent myofibrils (Benian et al., 1996; Borisov et al., 2006; Cunha and Mohler, 2008). Cunha and colleagues demonstrated that ankyrin-B/obscurin interaction recruits PP2A to the cardiac M-line (Cunha and Mohler, 2008) (Figure 1). In addition to the intermolecular interactions, the regulatory domain regulates the intramolecular interactions with the ankyrin MBD (Abdi et al., 2006).
Spectrin Polypeptides in Cardiac Physiology
Spectrins are actin-associated proteins that provide structural support to the plasma membrane and play roles in the localization of membrane and signaling proteins in the heart (Hund and Mohler, 2010). Spectrin alpha (α) and beta (β) subunits are assembled laterally to form anti-parallel heterodimers, and these heterodimers are assembled to form a flexible hetero-tetramic molecule (Bennett and Baines, 2001). There are two α spectrin genes and five β spectrin genes that encode a diverse group of alternatively-spliced spectrin isoforms with specific functions (Bennett and Healy, 2009). In ventricular myocytes, αII and βII spectrin are found at transverse-tubule and sarcoplasmic reticulum (SR) membranes (Baines and Pinder, 2005), whereas βIV spectrin is located at the intercalated disc (Hund et al., 2010).
In heart, βII spectrin directly interacts with ankyrin-B at transverse-tubule membranes (Mohler et al., 2004c; Smith et al., 2015). In fact, βII spectrin is a critical element of the larger ankyrin-B complex that directly links membrane and signaling proteins including NCX, Na/K ATPase, and β56α with the underlying actin-based cytoskeleton. Loss of βII spectrin in adult ventricular myocytes directly impacts the expression and localization of ankyrin-B as well as ankyrin-B-associated proteins (Smith et al., 2015). Beyond loss of ankyrin-binding proteins, βII spectrin null myocytes unexpectedly showed abnormal localization of the cardiac ryanodine receptor. The relationship of βII spectrin and ryanodine receptor is currently unknown. At the animal level, loss of βII spectrin in mice is lethal in mid-gestation due to incomplete development of the heart (Tang et al., 2003). Conditional deletion of βII spectrin in ventricular myocytes results in sinus node dysfunction and ventricular arrhythmia. These mice also display cytoskeletal remodeling and accelerated heart failure following transverse aortic constriction. As described later, human ANK2 variants that alter the interaction with βII spectrin are linked with congenital arrhythmia (Smith et al., 2015). Further, βII spectrin levels are altered in acquired forms of human heart failure and arrhythmia, as well as in animal models of cardiovascular disease (Smith et al., 2016). Finally, individuals with Beckwith-Wiedemann syndrome (BWS) with a reduction in βII spectrin expression (Yao et al., 2010) show congenital heart failure and ventricular septal defects (Drut et al., 2006).
In heart, βIV spectrin directly associates with ankyrin-G and is required for the targeting of calcium/calmodulin-dependent kinase II (CaMKII) to the intercalated disc (Hund et al., 2010). Notably, this interaction is critical for the regulation of INa by modulating CaMKII-dependent phosphorylation of Nav1.5 (Hund et al., 2010; Koval et al., 2012). Beyond, ankyrin-G and CaMKII, βIV spectrin regulates TREK-1, a mechano-sensitive K2P channel (Heurteaux et al., 2004). More specifically, βIV spectrin and TREK-1 associate, and βIV spectrin is required for TREK-1 localization and function in ventricular myocytes (Hund et al., 2014). Mice lacking TREK-1 binding display delayed action potential repolarization and arrhythmia. These data support a broader role for βIV spectrin in cardiovascular electrophysiology by regulation of TREK-1 membrane localization and function. Like βII spectrin, βIV spectrin levels are altered in human heart failure as well as in animal models of cardiovascular disease (Hund et al., 2014).
Finally, αII spectrin-deficient embryos are embryonic lethal with abnormal cardiac shape, cardiac dilatation and changes in myocardium (Stankewich et al., 2011). Beyond ankyrins, Mena and VASP, important regulators of actin dynamics form a complex with αII spectrin in adult cardiomyocytes (Benz et al., 2013). The in vivo role(s) of αII spectrin in adult heart is currently unknown but an important area for future research.
In vivo Roles for Cardiac Ankyrins
Ankyrin-G is Required for Targeting Nav1.5
In neurons, the link between ankyrin-G and Nav channel targeting is well established (Jenkins and Bennett, 2001; Saifetiarova et al., 2017). In heart, rat ventricular myocytes lacking ankyrin-G display a reduction in Nav1.5 expression, localization, and INa (Lowe et al., 2008). In fact, in myocytes with reduced ankyrin-G expression, Nav1.5 localization is limited in the perinuclear region of rat ventricular myocytes. Notably, this role is specific for ankyrin-G vs. ankyrin-B as ankyrin-B-null neonatal cardiomyocytes show no difference in Nav1.5 expression or localization. Further, loss of ankyrin-G is specific for INa vs. other cardiac currents (e.g., ICa, L) (Lowe et al., 2008).
In animals, ankyrin-G expression is required for the targeting of Nav1.5 and CaMKII to the intercalated disc (Makara et al., 2014). Nav1.5 expression and INa is reduced in myocytes derived from mice with selective loss of cardiomyocyte ankyrin-G (cKO) (Makara et al., 2014). Further, βIV spectrin is absent in the intercalated disc of myocytes of ankyrin cKO mice. Electrocardiographic studies of these mice show significant reduction in heart rate, impaired atrioventricular conduction, and an increase in PR interval. Moreover, cKO mice display a significant increase in the QRS interval and delayed intraventricular conduction. Administration of flecainide or catecholamines (elevated adrenergic stimulation) induces arrhythmia in these mice.
Beyond animals, work in humans supports the link between ankyrin-G and Nav channel targeting. Priori et al. (2000) identified an individual with Brugada syndrome harboring an SCN5A variant in the Nav1.5 motif that interacts with ankyrin-G (Mohler et al., 2004a). The group identified the SCN5A variant in a 47-year-old female with a history of palpitations and an unexplained syncope at rest. Using in vitro binding assays, the p.E1053K variant was found to disrupt the interaction between Nav1.5 and ankyrin-G resulting in abnormal targeting in myocytes.
A canine myocardial infarction (MI) model was used to study the role of ankyrin-G in acquired arrhythmic disease. At 5 days, there was a 42% reduction in Nav1.5 protein expression and a 50% increase in ankyrin-G expression in the epicardial border zone. These data suggest that ankyrin-G levels increase to regulate Nav1.5 recruitment to sarcolemmal membrane (Dun et al., 2013).
Ankyrin-B
First identified in brain, ankyrin-B has now been directly linked with a host of functions in the heart. Further, ankyrin-B dysfunction is now well documented in multiple forms of congenital and acquired cardiovascular disease. While ankyrin-B was known to directly associate with critical cardiac ion channels and transporters, an in vivo role for ankyrin-B in cardiac function was first illustrated using mice heterozygous for an ankyrin-B null mutation. While ankyrin-B null mice die shortly after birth, ankyrin-B+/− mice live to adulthood but display bradycardia, heart rate variability, prolonged QT intervals, and sudden cardiac death in response to exercise combined with catecholaminergic stimulation (Mohler et al., 2003). While adult ventricular myocytes from ankyrin-B+/− mice display normal action potential duration, these myocytes display early and delayed after-depolarizations in response to catecholamines. At the molecular level, ankyrin-B+/− ventricular myocytes display reduced expression and abnormal localization of ankyrin-B-binding partners including Na/K ATPase and NCX. As illustrated in computational models (Wolf et al., 2010), loss of Na/K ATPase and NCX results in increased SR calcium stores that result in an arrhythmogenic substrate in the ankyrin-B+/− animals (Mohler et al., 2004b). Subsequent work from Despa and colleagues revealed significant alterations in local [Ca2+] as well as hyper-phosphorylation of ryanodine receptors in ankyrin-B+/− ventricular myocytes (Camors et al., 2012; Popescu et al., 2016) potentially due to altered PP2A regulation (DeGrande et al., 2012). Further characterization of ankyrin-B+/− mice showed premature senescence and reduced longevity with mice showing loss of adiposity starting at 6 months of age (Mohler et al., 2007a).
Ankyrin-B dysfunction is directly linked with human cardiovascular disease. Over two decades ago, Schott and colleagues identified type 4 long QT syndrome in a large French kindred with sinus node dysfunction and episodes of atrial fibrillation (Schott et al., 1995). Prolonged QTc was observed among the 25 affected patients (21 adults and 4 children). Individuals in the family also displayed catecholaminergic polymorphic ventricular arrhythmia and sudden death. Sequencing of the gene encoding ankyrin-B (ANK2) revealed a positional transition (A to G) mutation at position 4,274 in exon 36, leading to the substitution of a glycine for a glutamic acid at amino acid residue 1,425 (p.E1425G) near the regulatory domain of ankyrin-B in forty-five family members (24 carriers and 21 non-carriers). The p.E1425G variant, subsequently identified as a loss-of-function variant in myocytes, showed prevalence in 22 out of 24 individuals with the LQT4 phenotype and was absent in non-affected individuals (Mohler et al., 2003). Since this initial discovery, a number of new ankyrin-B loss-of-function variants have been identified in individuals with ventricular arrhythmias. However, as discussed below, due to the diversity of non-ventricular phenotypes associated with the disease (including many lacking QTc prolongation), the term “ankyrin-B syndrome” has been adopted to describe individuals harboring ANK2 loss-of-function variants (Mohler et al., 2004b, 2007b). As in the case of many cardiovascular disease genes, the penetrance of phenotypes in ANK2 variant carriers is variable. As ankyrin-B polypeptide stability and function is directly impacted by physiological and pathological stress (e.g., inflammation, elevated reactive oxygen species), we predict that a “second hit” may be necessary for full disease penetrance.
Active areas of research in this field are currently focused on identifying the mechanisms underlying both congenital and acquired ankyrin-B loss in human disease as well as the discovery of new variants. For example, while loss-of-function variants were limited to the RD, new variants in the ankyrin-B MBD have shed light on new phenotypes associated with “ankyrin-B syndrome.” Recently, a novel MBD variant in the ANK2 gene (p.S646F) was identified in two Gitxan families with LQTS phenotypes by the Swayne and Arbour groups (Swayne et al., 2017). The first person identified was a 28-year old man with a clinical diagnosis of LQTS and recurrent syncope. Five of this patient's relatives were confirmed to harbor this variant. This variant was also identified in a second family with a clinical diagnosis of LQTS. Within these two families, a total of 13 adults and 5 children have been identified with this novel ANK2 variant. In vitro evaluation illustrated that the expression of ankyrin MBD with p.S646F variant in bacteria was normal and MBD structural properties were intact. However, expression of ankyrin-B p.S646F variant in neonatal cardiomyocytes showed a reduction in ankyrin-B expression and alteration of expression of NCX (Swayne et al., 2017). Finally, beyond LQT and catecholaminergic polymorphic ventricular tachycardia, ANK2 variants have been associated with other forms of ventricular arrhythmia including early repolarization syndrome (Krogh Broendberg et al., 2015).
ANK2 variants are not limited to MBD or RD. Smith et al. identified an ANK2 arrhythmia variant (c.2969G>A) caused by the substitution of Arg to Gln at position 990 (p. R990Q). This variant is rare with a minor allele frequency of 0.007% and resides near the central ZU5 binding surface for βII spectrin. Using myocytes from ankyrin-B conditional knockout mice (cKO), exogenous expression of WT ankyrin-B, but not ankyrin-B p.R990Q was able to rescue the localization of NCX (Smith et al., 2015).
Ankyrin-B dysfunction is also linked with non-ventricular forms of human arrhythmia. Individuals with select ANK2 loss-of-function variants display atrial fibrillation and/or sinus node dysfunction (Le Scouarnec et al., 2008; Cunha et al., 2011). Consistent with these data, ankyrin-B+/− mice also display both atrial and sinus node phenotypes. At the cellular level, primary atrial and sinus node myocytes from ankyrin-B+/− mice display shortened action potentials and loss of L-type Ca2+ current. In ankyrin-B+/− atrial myocytes, voltage-gated L-type Ca2+ channel Cav1.3 (but not Cav1.2) expression and function were reduced.
Until recently, ANK2-based disease was linked only to individuals with single “point mutations” (Mohler et al., 2004b, 2007b; Sherman et al., 2005; Krogh Broendberg et al., 2015; Swayne et al., 2017). However, recently a family was identified with an ANK2 transection in chromosome 4 (Huq et al., 2017). The proband had a balanced translocation between the long arms of chromosomes 4 and 9. Five other family members were carriers of the same balanced translocation. The 16-week-old fetus of the proband from a terminated pregnancy was found to have multiple cardiac abnormalities. The proband showed normal echocardiography findings and only Holter monitor recorded few episodes of bradycardia at night. Isolated lymphoblasts from the proband demonstrated a significant reduction in ankyrin-B and binding partners, confirming a model of ankyrin-B haploinsufficiency. The mother of the proband was also a carrier of the translocation and displayed frequent ventricular ectopy and periods of bigeminy.
Beyond congenital disease, dysfunction in ankyrin-B-based pathways has been linked with acquired forms of cardiovascular disease. In a canine model of myocardial infarction, ankyrin-B mRNA levels were increased and protein levels were decreased in day 5-post infarction in the border zone (Hund et al., 2009). At the myocyte level, abnormal distribution of ankyrin-B was noted. The authors further showed changes in expression and/or distribution of downstream ankyrin-associated proteins Na/K ATPase, NCX, InsP3 receptor and PP2A (Hund et al., 2009). In addition to its role in myocardial infarction, Li et al. identified the association of ATP-sensitive K+ channel (KATP) with ankyrin-B as a cardio-protective mechanism against acute ischemia (Li et al., 2010). Ankyrin-B levels are now also known to be reduced in patients with atrial fibrillation (Cunha et al., 2011).
Ankyrin-R
To date, human variants in ANK1 have not been directly linked with cardiac arrhythmia phenotypes. While not the focus on this review, it is of note that variants in ANK1 are a primary cause for hereditary spherocytosis (Eber et al., 1996; Tse and Lux, 1999; Perrotta et al., 2008; Satchwell et al., 2016).
Summary
Cardiac ankyrins and spectrins are key multifunctional molecules in the heart. In myocytes, ankyrins, and spectrins serve essential roles in targeting ion channels and transporters to critical membrane domains. However, beyond membrane proteins, these molecules serve as crucial cellular nodes to integrate membrane proteins with critical signaling and structural proteins in diverse cardiac cell types. While animals have served as important models to define ankyrin and spectrin function in vivo, more recent findings in patients have both solidified the importance of ankyrins and spectrin in human physiology and disease, and raised important questions related to unexpected roles of these polypeptides. Key future questions in the field remain the impact of environment or “second hit” gene variants on disease susceptibility/penetrance in ANK2 variant carriers, as well as potential non-myocyte/non-cardiac phenotypes in patients with ankyrin gene variants.
Author Contributions
All authors listed, have made substantial, direct and intellectual contribution to the work, and approved it for publication.
Conflict of Interest Statement
The authors declares that the research was conducted in the absence of any commercial or financial relationships that could be construed as a potential conflict of interest.
Acknowledgments
This work was supported by National Heart, Lung and Blood Institute grants HL-135754, HL-134824, and HL-114383 (PM). ME is supported by Dorothy M. Davis Heart and Lung Research Institute Post-doctoral training fellowship in Congenital and Acquired Heart Diseases (5T32HL098039).
Abbreviations
MBD, Membrane-binding domain; CTD, C-terminal domain; SBD, Spectrin-binding domain; DD, Death domain; LQTS, Long QT syndrome; NCX, Sodium-Calcium exchanger; Na/K ATPase, Sodium-potassium ATPase; IP3R, Inositol trisphosphate receptor; cKO, Conditional knock-out; INa,L, Late component of Nav current; CaMKII, Calcium/calmodulin-dependent kinase; KATP, ATP-sensitive K+ channel; Cav1.3, Voltage gated L-type Ca2+ channel; PP2A, Protein phosphatase 2A; Nav1.5, Voltage-gated sodium channel; TREK-1, TWIK-related potassium channel-1.
References
Abdi, K. M., Mohler, P. J., Davis, J. Q., and Bennett, V. (2006). Isoform specificity of ankyrin-B: a site in the divergent C-terminal domain is required for intramolecular association. J. Biol. Chem. 281, 5741–5749. doi: 10.1074/jbc.M506697200
Baines, A. J., and Pinder, J. C. (2005). The spectrin-associated cytoskeleton in mammalian heart. Front. Biosci. 10, 3020–3033. doi: 10.2741/1759
Benian, G. M., Tinley, T. L., Tang, X., and Borodovsky, M. (1996). The Caenorhabditis elegans gene unc-89, required fpr muscle M-line assembly, encodes a giant modular protein composed of Ig and signal transduction domains. J. Cell Biol. 132, 835–848. doi: 10.1083/jcb.132.5.835
Bennett, V., and Baines, A. J. (2001). Spectrin and ankyrin-based pathways: metazoan inventions for integrating cells into tissues. Physiol. Rev. 81, 1353–1392. Available online at: http://physrev.physiology.org/content/81/3/1353.full-text.pdf+html
Bennett, V., and Healy, J. (2009). Membrane domains based on ankyrin and spectrin associated with cell-cell interactions. Cold Spring Harb. Perspect. Biol. 1:a003012. doi: 10.1101/cshperspect.a003012
Bennett, V., and Stenbuck, P. J. (1979). The membrane attachment protein for spectrin is associated with band 3 in human erythrocyte membranes. Nature 280, 468–473. doi: 10.1038/280468a0
Benz, P. M., Merkel, C. J., Offner, K., Abeßer, M., Ullrich, M., Fischer, T., et al. (2013). Mena/VASP and alphaII-spectrin complexes regulate cytoplasmic actin networks in cardiomyocytes and protect from conduction abnormalities and dilated cardiomyopathy. Cell Commun. Signal 11:56. doi: 10.1186/1478-811X-11-56
Bhasin, N., Cunha, S. R., Mudannayake, M., Gigena, M. S., Rogers, T. B., and Mohler, P. J. (2007). Molecular basis for PP2A regulatory subunit B56alpha targeting in cardiomyocytes. Am. J. Physiol. Heart Circ. Physiol. 293, H109–H119. doi: 10.1152/ajpheart.00059.2007
Bodine, D. M., Birkenmeier, C. S., and Barker, J. E. (1984). Spectrin deficient inherited hemolytic anemias in the mouse: characterization by spectrin synthesis and mRNA activity in reticulocytes. Cell 37, 721–729. doi: 10.1016/0092-8674(84)90408-2
Borisov, A. B., Sutter, S. B., Kontrogianni-Konstantopoulos, A., Bloch, R. J., Westfall, M. V., and Russell, M. W. (2006). Essential role of obscurin in cardiac myofibrillogenesis and hypertrophic response: evidence from small interfering RNA-mediated gene silencing. Histochem. Cell Biol. 125, 227–238. doi: 10.1007/s00418-005-0069-x
Bourguignon, L. Y., Jin, H., Iida, N., Brandt, N. R., and Zhang, S. H. (1993). The involvement of ankyrin in the regulation of inositol 1,4,5-trisphosphate receptor-mediated internal Ca2+ release from Ca2+ storage vesicles in mouse T-lymphoma cells. J. Biol. Chem. 268, 7290–7297.
Camors, E., Mohler, P. J., Bers, D. M., and Despa, S. (2012). Ankyrin-B reduction enhances Ca spark-mediated SR Ca release promoting cardiac myocyte arrhythmic activity. J. Mol. Cell Cardiol. 52, 1240–1248. doi: 10.1016/j.yjmcc.2012.02.010
Cunha, S. R., Hund, T. J., Hashemi, S., Voigt, N., Li, N., Wright, P., et al. (2011). Defects in ankyrin-based membrane protein targeting pathways underlie atrial fibrillation. Circulation 124, 1212–1222. doi: 10.1161/CIRCULATIONAHA.111.023986
Cunha, S. R., Le Scouarnec, S., Schott, J. J., and Mohler, P. J. (2008). Exon organization and novel alternative splicing of the human ANK2 gene: implications for cardiac function and human cardiac disease. J. Mol. Cell Cardiol. 45, 724–734. doi: 10.1016/j.yjmcc.2008.08.005
Cunha, S. R., and Mohler, P. J. (2006). Cardiac ankyrins: Essential components for development and maintenance of excitable membrane domains in heart. Cardiovasc. Res. 71, 22–29. doi: 10.1016/j.cardiores.2006.03.018
Cunha, S. R., and Mohler, P. J. (2008). Obscurin targets ankyrin-B and protein phosphatase 2A to the cardiac M-line. J. Biol. Chem. 283, 31968–31980. doi: 10.1074/jbc.M806050200
Davis, J. Q., McLaughlin, T., and Bennett, V. (1993). Ankyrin-binding proteins related to nervous system cell adhesion molecules: candidates to provide transmembrane and intercellular connections in adult brain. J. Cell Biol. 121, 121–133. doi: 10.1083/jcb.121.1.121
DeGrande, S., Nixon, D., Koval, O., Curran, J. W., Wright, P., Wang, Q., et al. (2012). CaMKII inhibition rescues proarrhythmic phenotypes in the model of human ankyrin-B syndrome. Heart Rhythm 9, 2034–2041. doi: 10.1016/j.hrthm.2012.08.026
Drut, R., Quijano, G., Altamirano, M. E., Jones, M. C., and Maffessoli, O. B. (2006). Vascular malformation and choroid plexus adrenal heterotopia: new findings in Beckwith-Wiedemann syndrome? Fetal Pediatr. Pathol. 25, 191–197. doi: 10.1080/15513810601015704
Dun, W., Lowe, J. S., Wright, P., Hund, T. J., Mohler, P. J., and Boyden, P. A. (2013). Ankyrin-G participates in INa remodeling in myocytes from the border zones of infarcted canine heart. PLoS ONE 8:e78087. doi: 10.1371/journal.pone.0078087
Eber, S. W., Gonzalez, J. M., Lux, M. L., Scarpa, A. L., Tse, W. T., Dornwell, M., et al. (1996). Ankyrin-1 mutations are a major cause of dominant and recessive hereditary spherocytosis. Nat. Genet. 13, 214–218. doi: 10.1038/ng0696-214
Hashemi, S. M., Hund, T. J., and Mohler, P. J. (2009). Cardiac ankyrins in health and disease. J. Mol. Cell Cardiol. 47, 203–209. doi: 10.1016/j.yjmcc.2009.04.010
Heurteaux, C., Guy, N., Laigle, C., Blondeau, N., Duprat, F., Mazzuca, M., et al. (2004). TREK-1, a K+ channel involved in neuroprotection and general anesthesia. EMBO J. 23, 2684–2695. doi: 10.1038/sj.emboj.7600234
Hortsch, M., Nagaraj, K., and Godenschwege, T. A. (2009). The interaction between L1-type proteins and ankyrins–a master switch for L1-type CAM function. Cell Mol. Biol. Lett. 14, 57–69. doi: 10.2478/s11658-008-0035-4
Hund, T. J., Koval, O. M., Li, J., Wright, P. J., Qian, L., Snyder, J. S., et al. (2010). A beta(IV)-spectrin/CaMKII signaling complex is essential for membrane excitability in mice. J. Clin. Invest. 120, 3508–3519. doi: 10.1172/JCI43621
Hund, T. J., and Mohler, P. J. (2010). Cardiac spectrins: alternative splicing encodes functional diversity. J. Mol. Cell Cardiol. 48, 1031–1032. doi: 10.1016/j.yjmcc.2010.02.002
Hund, T. J., Snyder, J. S., Wu, X., Glynn, P., Koval, O. M., Onal, B., et al. (2014). beta(IV)-Spectrin regulates TREK-1 membrane targeting in the heart. Cardiovasc. Res. 102, 166–175. doi: 10.1093/cvr/cvu008
Hund, T. J., Wright, P. J., Dun, W., Snyder, J. S., Boyden, P. A., and Mohler, P. J. (2009). Regulation of the ankyrin-B-based targeting pathway following myocardial infarction. Cardiovasc. Res. 81, 742–749. doi: 10.1093/cvr/cvn348
Huq, A. J., Pertile, M. D., Davis, A. M., Landon, H., James, P. A., Kline, C. F., et al. (2017). A novel mechanism for human cardiac Ankyrin-B syndrome due to reciprocal chromosomal translocation. Heart Lung Circ. 26, 612–618. doi: 10.1016/j.hlc.2016.09.013
Ipsaro, J. J., and Mondragón, A. (2010). Structural basis for spectrin recognition by ankyrin. Blood 115, 4093–4101. doi: 10.1182/blood-2009-11-255604
Jenkins, S. M., and Bennett, V. (2001). Ankyrin-G coordinates assembly of the spectrin-based membrane skeleton, voltage-gated sodium channels, and L1 CAMs at Purkinje neuron initial segments. J. Cell Biol. 155, 739–746. doi: 10.1083/jcb.200109026
Koob, R., Zimmermann, M., Schoner, W., and Drenckhahn, D. (1988). Colocalization and coprecipitation of ankyrin and Na+,K+-ATPase in kidney epithelial cells. Eur. J. Cell Biol. 45, 230–237.
Kordeli, E., Lambert, S., and Bennett, V. (1995). AnkyrinG. a new ankyrin gene with neural-specific isoforms localized at the axonal initial segment and node of ranvier. J. Biol. Chem. 270, 2352–2359. doi: 10.1074/jbc.270.5.2352
Koval, O. M., Snyder, J. S., Wolf, R. M., Pavlovicz, R. E., Glynn, P., Curran, J., et al. (2012). Ca2+/calmodulin-dependent protein kinase II-based regulation of voltage-gated Na+ channel in cardiac disease. Circulation 126, 2084–2094. doi: 10.1161/CIRCULATIONAHA.112.105320
Krogh Broendberg, A., Pedersen, L. N., Nielsen, J. C., and Jensen, H. K. (2015). Ankyrin-2 variants associated with idiopathic ventricular fibrillation storm in patients with intermittent early repolarization pattern. HeartRhythm Case Rep. 1, 337–341. doi: 10.1016/j.hrcr.2015.05.008
Kunimoto, M. (1995). A neuron-specific isoform of brain ankyrin, 440-kD ankyrinB, is targeted to the axons of rat cerebellar neurons. J. Cell Biol. 131(6 Pt 2), 1821–1829. doi: 10.1083/jcb.131.6.1821
Lambert, S., Yu, H., Prchal, J. T., Lawler, J., Ruff, P., Speicher, D., et al. (1990). cDNA sequence for human erythrocyte ankyrin. Proc. Natl. Acad. Sci. U.S.A. 87, 1730–1734. doi: 10.1073/pnas.87.5.1730
Le Scouarnec, S., Bhasin, N., Vieyres, C., Hund, T. J., Cunha, S. R., Koval, O., et al. (2008). Dysfunction in ankyrin-B-dependent ion channel and transporter targeting causes human sinus node disease. Proc. Natl. Acad. Sci. U. S. A. 105, 15617–15622. doi: 10.1073/pnas.0805500105
Li, J., Kline, C. F., Hund, T. J., Anderson, M. E., and Mohler, P. J. (2010). Ankyrin-B regulates Kir6.2 membrane expression and function in heart. J. Biol. Chem. 285, 28723–28730. doi: 10.1074/jbc.M110.147868
Li, Z. P., Burke, E. P., Frank, J. S., Bennett, V., and Philipson, K. D. (1993). The cardiac Na+-Ca2+ exchanger binds to the cytoskeletal protein ankyrin. J. Biol. Chem. 268, 11489–11491.
Little, S. C., Curran, J., Makara, M. A., Kline, C. F., Ho, H. T., Xu, Z., et al. (2015). Protein phosphatase 2A regulatory subunit B56alpha limits phosphatase activity in the heart. Sci Signal 8, ra72. doi: 10.1126/scisignal.aaa5876
Lowe, J. S., Palygin, O., Bhasin, N., Hund, T. J., Boyden, P. A., Shibata, E., et al. (2008). Voltage-gated Nav channel targeting in the heart requires an ankyrin-G dependent cellular pathway. J. Cell Biol. 180, 173–186. doi: 10.1083/jcb.200710107
Makara, M. A., Curran, J., Little, S. C., Musa, H., Polina, I., Smith, S. A., et al. (2014). Ankyrin-G coordinates intercalated disc signaling platform to regulate cardiac excitability in vivo. Circ. Res. 115, 929–938. doi: 10.1161/CIRCRESAHA.115.305154
Mehra, R. (2007). Global public health problem of sudden cardiac death. J. Electrocardiol. 40, S118–122. doi: 10.1016/j.jelectrocard.2007.06.023
Mohler, P. J. (2006). Ankyrins and human disease: what the electrophysiologist should know. J. Cardiovasc. Electrophysiol. 17, 1153–1159. doi: 10.1111/j.1540-8167.2006.00540.x
Mohler, P. J., Gramolini, A. O., and Bennett, V. (2002). The ankyrin-B C-terminal domain determines activity of ankyrin-B/G chimeras in rescue of abnormal inositol 1,4,5-trisphosphate and ryanodine receptor distribution in ankyrin-B (-/-) neonatal cardiomyocytes. J. Biol. Chem. 277, 10599–10607. doi: 10.1074/jbc.M110958200
Mohler, P. J., Healy, J. A., Xue, H., Puca, A. A., Kline, C. F., Allingham, R. R., et al. (2007a). Ankyrin-B syndrome: enhanced cardiac function balanced by risk of cardiac death and premature senescence. PLoS ONE 2:e1051. doi: 10.1371/journal.pone.0001051
Mohler, P. J., Le Scouarnec, S., Denjoy, I., Lowe, J. S., Guicheney, P., Caron, L., et al. (2007b). Defining the cellular phenotype of “ankyrin-B syndrome” variants: human ANK2 variants associated with clinical phenotypes display a spectrum of activities in cardiomyocytes. Circulation 115, 432–441. doi: 10.1161/CIRCULATIONAHA.106.656512
Mohler, P. J., Rivolta, I., Napolitano, C., LeMaillet, G., Lambert, S., Priori, S. G., et al. (2004a). Nav1.5 E1053K mutation causing Brugada syndrome blocks binding to ankyrin-G and expression of Nav1.5 on the surface of cardiomyocytes. Proc. Natl. Acad. Sci. U.S.A. 101, 17533–17538. doi: 10.1073/pnas.0403711101
Mohler, P. J., Schott, J. J., Gramolini, A. O., Dilly, K. W., Guatimosim, S., duBell, W. H., et al. (2003). Ankyrin-B mutation causes type 4 long-QT cardiac arrhythmia and sudden cardiac death. Nature 421, 634–639. doi: 10.1038/nature01335
Mohler, P. J., Splawski, I., Napolitano, C., Bottelli, G., Sharpe, L., Timothy, K., et al. (2004b). A cardiac arrhythmia syndrome caused by loss of ankyrin-B function. Proc. Natl. Acad. Sci. U.S.A. 101, 9137–9142. doi: 10.1073/pnas.0402546101
Mohler, P. J., Yoon, W., and Bennett, V. (2004c). Ankyrin-B targets beta2-spectrin to an intracellular compartment in neonatal cardiomyocytes. J. Biol. Chem. 279, 40185–40193. doi: 10.1074/jbc.M406018200
Otto, E., Kunimoto, M., McLaughlin, T., and Bennett, V. (1991). Isolation and characterization of cDNAs encoding human brain ankyrins reveal a family of alternatively spliced genes. J. Cell Biol. 114, 241–253. doi: 10.1083/jcb.114.2.241
Perrotta, S., Gallagher, P. G., and Mohandas, N. (2008). Hereditary spherocytosis. Lancet 372, 1411–1426. doi: 10.1016/S0140-6736(08)61588-3
Popescu, I., Galice, S., Mohler, P. J., and Despa, S. (2016). Elevated local [Ca2+] and CaMKII promote spontaneous Ca2+ release in ankyrin-B-deficient hearts. Cardiovasc. Res. 111, 287–294. doi: 10.1093/cvr/cvw093
Priori, S. G., Napolitano, C., Gasparini, M., Pappone, C., Della Bella, P., Brignole, M., et al. (2000). Clinical and genetic heterogeneity of right bundle branch block and ST-segment elevation syndrome: a prospective evaluation of 52 families. Circulation 102, 2509–2515. doi: 10.1161/01.CIR.102.20.2509
Rosamond, W., Flegal, K., Furie, K., Go, A., Greenlund, K., Haase, N., et al. (2008). Heart disease and stroke statistics−2008 update: a report from the American Heart Association Statistics Committee and Stroke Statistics Subcommittee. Circulation 117, e25–e146. doi: 10.1161/CIRCULATIONAHA.107.187998
Saifetiarova, J., Taylor, A. M., and Bhat, M. A. (2017). Early and late loss of the Cytoskeletal Scaffolding protein, Ankyrin G reveals its role in maturation and maintenance of nodes of Ranvier in Myelinated Axons. J. Neurosci. 37, 2524–2538. doi: 10.1523/JNEUROSCI.2661-16.2017
Satchwell, T. J., Bell, A. J., Hawley, B. R., Pellegrin, S., Mordue, K. E., van Deursen, C. T., et al. (2016). Severe Ankyrin-R deficiency results in impaired surface retention and lysosomal degradation of RhAG in human erythroblasts. Haematologica 101, 1018–1027. doi: 10.3324/haematol.2016.146209
Schott, J. J., Charpentier, F., Peltier, S., Foley, P., Drouin, E., Bouhour, J. B., et al. (1995). Mapping of a gene for long QT syndrome to chromosome 4q25-27. Am. J. Hum. Genet. 57, 1114–1122.
Sherman, J., Tester, D. J., and Ackerman, M. J. (2005). Targeted mutational analysis of ankyrin-B in 541 consecutive, unrelated patients referred for long QT syndrome genetic testing and 200 healthy subjects. Heart Rhythm 2, 1218–1223. doi: 10.1016/j.hrthm.2005.07.026
Smith, S. A., Hughes, L. D., Kline, C. F., Kempton, A. N., Dorn, L. E., Curran, J., et al. (2016). Dysfunction of the beta2-spectrin-based pathway in human heart failure. Am. J. Physiol. Heart Circ. Physiol. 310, H1583–H1591. doi: 10.1152/ajpheart.00875.2015
Smith, S. A., Sturm, A. C., Curran, J., Kline, C. F., Little, S. C., Bonilla, I. M., et al. (2015). Dysfunction in the betaII spectrin-dependent cytoskeleton underlies human arrhythmia. Circulation 131, 695–708. doi: 10.1161/CIRCULATIONAHA.114.013708
Stankewich, M. C., Cianci, C. D., Stabach, P. R., Ji, L., Nath, A., and Morrow, J. S. (2011). Cell organization, growth, and neural and cardiac development require alphaII-spectrin. J. Cell Sci. 124(Pt 23), 3956–3966. doi: 10.1242/jcs.080374
Swayne, L. A., Murphy, N. P., Asuri, S., Chen, L., Xu, X., McIntosh, S., et al. (2017). Novel variant in the ANK2 membrane-binding domain is associated with Ankyrin-B syndrome and structural heart disease in a first nations population with a high rate of long QT syndrome. Circ. Cardiovasc. Genet. 10:e001537. doi: 10.1161/CIRCGENETICS.116.001537
Tang, Y., Katuri, V., Dillner, A., Mishra, B., Deng, C. X., and Mishra, L. (2003). Disruption of transforming growth factor-beta signaling in ELF beta-spectrin-deficient mice. Science 299, 574–577. doi: 10.1126/science.1075994
Tse, W. T., and Lux, S. E. (1999). Red blood cell membrane disorders. Br. J. Haematol. 104, 2–13. doi: 10.1111/j.1365-2141.1999.01130.x
Wolf, R. M., Mitchell, C. C., Christensen, M. D., Mohler, P. J., and Hund, T. J. (2010). Defining new insight into atypical arrhythmia: a computational model of ankyrin-B syndrome. Am. J. Physiol. Heart Circ. Physiol. 299, H1505–H1514. doi: 10.1152/ajpheart.00503.2010
Wu, H. C., Yamankurt, G., Luo, J., Subramaniam, J., Hashmi, S. S., Hu, H., et al. (2015). Identification and characterization of two ankyrin-B isoforms in mammalian heart. Cardiovasc. Res. 107, 466–477. doi: 10.1093/cvr/cvv184
Keywords: ankyrin, spectrin, arrhythmia, heart disease, ion channel, signaling
Citation: El Refaey MM and Mohler PJ (2017) Ankyrins and Spectrins in Cardiovascular Biology and Disease. Front. Physiol. 8:852. doi: 10.3389/fphys.2017.00852
Received: 10 September 2017; Accepted: 12 October 2017;
Published: 27 October 2017.
Edited by:
Hugues Abriel, University of Bern, SwitzerlandReviewed by:
Toon Van Veen, Utrecht University, NetherlandsWayne Rodney Giles, University of Calgary, Canada
Copyright © 2017 El Refaey and Mohler. This is an open-access article distributed under the terms of the Creative Commons Attribution License (CC BY). The use, distribution or reproduction in other forums is permitted, provided the original author(s) or licensor are credited and that the original publication in this journal is cited, in accordance with accepted academic practice. No use, distribution or reproduction is permitted which does not comply with these terms.
*Correspondence: Mona M. El Refaey, bW9uYS5lbHJlZmFleUBvc3VtYy5lZHU=