- 1Departamento de Zoología, Facultad de Ciencias, Universidad de Granada, Granada, Spain
- 2Centro Oceanográfico de Canarias, Instituto Español de Oceanografía, Santa Cruz de Tenerife, Spain
The culture of the common octopus (Octopus vulgaris) is promising since the species has a relatively short lifecycle, rapid growth, and high food conversion ratios. However, recent attempts at successful paralarvae culture have failed due to slow growth and high mortality rates. Establishing an optimal nutritional regime for the paralarvae seems to be the impeding step in successful culture methods. Gaining a thorough knowledge of food regulation and assimilation is essential for paralarvae survival and longevity under culture conditions. The aim of this study, then, was to elucidate the characteristic metabolic organization of octopus paralarvae throughout an ontogenic period of 12 days post-hatching, as well as assess the effect of diet enrichment with live prey containing abundant marine phospholipids. Our results showed that throughout the ontogenic period studied, an increase in anaerobic metabolism took place largely due to an increased dependence of paralarvae on exogenous food. Our studies showed that this activity was supported by octopine dehydrogenase activity, with a less significant contribution of lactate dehydrogenase activity. Regarding aerobic metabolism, the use of amino acids was maintained for the duration of the experiment. Our studies also showed a significant increase in the rate of oxidation of fatty acids from 6 days after-hatching. A low, although sustained, capacity for de novo synthesis of glucose from amino acids and glycerol was also observed. Regardless of the composition of the food, glycerol kinase activity significantly increased a few days prior to a massive mortality event. This could be related to a metabolic imbalance in the redox state responsible for the high mortality. Thus, glycerol kinase might be used as an effective nutritional and welfare biomarker. The studies in this report also revealed the important finding that feeding larvae with phospholipid-enriched Artemia improved animal viability and welfare, significantly increasing the rate of survival and growth of paralarvae.
Introduction
Commercial octopus fishing has been exploited for several decades leading to strict regulations on fishing practices. As a result, attempts have been made to culture Octopus vulgaris in captivity. These practices remain promising since the species has a relatively short lifecycle, fast growth, and high food conversion ratios. However, under culture conditions, paralarvae of this cephalopod species show slow growth and high mortality rates, reflecting the main obstacle to successful culture. Nutritional deficiencies and food source imbalances are considered the primary causes for low paralarvae survival and growth, although other factors related to zootechnical conditions (such as, tank volume, culture density, and light) cannot be ruled out (Iglesias and Fuentes, 2014).
In terms of nutrition, Lee (1994) highlighted the importance of closely balanced amino acid levels in larval food needed as substrates for metabolism and protein synthesis. On the other hand, Navarro et al. (2014) reported that a nutritional imbalance in both content and profile of the fatty acid in artificial food sources may be responsible for high mortalities since reared paralarvae differ from recently hatched individuals especially in these aspects. Establishing an optimal nutritional regime is of paramount importance for successful thriving of paralarvae and efficient aquaculture. Thus, it is essential to have a thorough knowledge of the physiological processes regulating food assimilation and metabolism.
Regarding metabolic capabilities of cephalopods, studies have shown predominance in protein catabolism, regardless of individual body mass (Boucher-Rodoni and Mangold, 1985; Lee, 1994; Katsanevakis et al., 2005; Petza et al., 2006). This implies a high requirement of protein in available food sources. Additionally, a low capacity to use lipids as metabolic fuel has been reported throughout the cephalopod literature (Ballantyne et al., 1981; Storey and Storey, 1983; O'Dor and Webber, 1986; Lee, 1994; Hochachka, 1995).
After the larval yolk reserves are exhausted, paralarvae depend solely on exogenous food. Octopuses in this stage survive on live prey, which are actively captured using bursts of anaerobic swimming (Baldwin, 1982). In vertebrates, energy needed for early stage activity is supported by the creatine phosphate/creatine kinase ATP regeneration system, followed by the pyruvate fermentation by lactate dehydrogenase (LDH). Interestingly, in mollusks, anaerobic conditions, in addition to the LDH activity, have been shown to produce energy by the arginine phosphate/arginine kinase system in which octopine dehydrogenase (ODH) is involved (Lyzlova and Stefanov, 1991). In many species of mollusks only ODH activity is present; when both ODH and LDH are operating, ODH exhibits the higher activity (Regnouf and van Thoai, 1970; Gäde, 1980; Speers-Roesch et al., 2016). Regarding the advantage of ODH over LDH, Fields and Quinn (1981) reported that ODH maintains a lower cytosolic redox ratio (NADH/NAD+) than LDH during anoxia, where the glycolytic pathway is prevalent.
Although metabolic capacities in adult cephalopods have been assessed in several studies (O'Dor and Wells, 1987; Lee, 1994), such studies have not been reported for early stage larvae. Thus, this report seeks to elucidate the metabolic organization in common octopus paralarvae throughout ontogenic development and to assess the capacity of adaptations to changes in food composition.
Materials and Methods
All experimental work was performed according to Spanish law (RD 53/2013) based on the European Union's directive on animal welfare for the protection of animals used for scientific purposes (Directive 2010/63/EU). Guidelines for the care and welfare of cephalopods proposed by Fiorito et al. (2015) were followed in this study. The present study was also approved (register document CEIBA2014-0108) by the Ethics Committee for Animal Research and Welfare (Comité de Ética de la Investigación y Bienestar Animal, CEIBA) from the University of La Laguna (Spain).
Paralarvae Rearing Conditions
Two experiments were carried out to characterize the metabolic profile in common octopus paralarvae. The first experiment analyzed the time course of metabolic capacities during the first 12 days of life. The second experiment analyzed the influence of food composition (with a diet rich in highly unsaturated fatty acids and phospholipids) on metabolic capacities and survival.
To carry out both experiments, a total of 20 adult Octopus vulgaris were captured by local fishermen using artisanal octopus traps in Tenerife coastal waters (Canary Islands, Spain) and maintained in the facilities of the Oceanographic Centre of the Canary Islands (Spanish Institute of Oceanography). Adult specimens were kept in 1,000 L tanks (with a maximum density of 10 kg/tank) with water renovation (5 L/min), under oxygen saturation conditions and low light intensity. Two batches of paralarvae obtained from this broodstock were used in the experiment described below.
Experiment 1. Time Course of Metabolic Capacities During the First 12 Days of Life
A total of 15,000 paralarvae, (5,000 paralarvae/tank; 5 paralarvae /L), were reared in triplicate during 12 days in 1,000 L black fiberglass cylinder-conical tanks with a flow-through seawater system at 60 mL/s from 18:00 to 8:00 (over 2.5 renewals/day). The renovation flow allowed the unfed Artemia to go through a 500 μm outflow mesh located in the middle of the tank. Moderated flux aeration stones were placed on the edge of the tanks. Green water (1 × 106 cell/mL Nannochloropsis sp supplied by Phytobloom Green Formula®, Olhão, Portugal) was added at 8:00. The natural photoperiod was attained at a maximum intensity (around mid-day) of 300 lx. Temperature and oxygen were measured daily, and nitrite, ammonium, and salinity once a week (see Table 1). Paralarvae were fed with Artemia (Sep-Art BF INVE Aquaculture, Dendermonde, Belgium) enriched for 20 h after hatching with freeze dried Isochrysis galbana (supplied by easy algae®, Cádiz, Spain; 10 metanauplii/mL, 1·107 cell/mL). Artemia was supplied at 0.3 Artemia/mL divided in three times a day (at 10:00, 13:00, and 16:00). In order to avoid enrichment lost, Artemia cultures were kept in the dark at 4°C with soft aeration until the moment of feeding.
Experiment 2. Influence of Food Composition on Metabolic Capacities and Survival
A total of 30,000 paralarvae, (5,000 paralarvae per tank; 10 paralarvae/L) were reared over 28 days in 500 L black fiberglass cylinder-conical tanks. Two fluorescent lights (OSRAM Dulux superstar 36W/840) were placed above each tank to attain 700 lx focused in the middle of the tank surface with a 12L:12D photoperiod (8:00–20:00). A flow-through seawater system equipped with 20, 5, and 1 μm filter cartridges and UV lamps was used. A water flow per tank of 1 L/min (over 1.5 renewals/day) was applied from 18:00 to 8:00. In similar protocol to the first experiment, the renovation flow allowed the unfed Artemia to go through a 500 μm outflow mesh located in the middle of the tanks. Two moderated flux aeration stones were placed in front each other in the edges of the tanks. Green-water system using 5 × 105 cell/mL of Nannochloropsis sp (Phytobloom Green Formula®, Olhão, Portugal) was added to the tanks before illumination. Temperature and oxygen were measured daily, and nitrite, ammonium and salinity once a week (see Table 1).
Paralarvae were fed with the same Artemia used in the first experiment, but in this case, the control group (C) was enriched with microalgae (freezer dried Isochrysis galbana, and Nannochloropsis sp) or with an experimental diet of Marine Lecithin LC 60® (PhosphoTech Laboratoires, Saint Herblain, France; LC60). Each treatment was carried out in triplicate. To quantitate Artemia size over the duration of the experimental period, three prey sizes were used: first, nauplii from day 0 to 3; second, metanauplii from day 4 to 11; and third, 8 day old metanauplii from day 12 to 27. Enrichments and on-growing of Artemia were made according to Garrido et al. (2017). Artemia was supplied at 0.5 Artemia/mL divided in three times a day (at 10:00, 13:00, and 16:00). In order to avoid enrichment loss, Artemia cultures were kept in the dark at 4°C with soft aeration until the moment of feeding.
Growth and Survival
No dry weight was determined in Experiment 1. In the second experiment, 15 individuals' dry weight (DW) was determined for each treatment at day 0, 12, and 28. Paralarvae were euthanized in chilled seawater (−2°C), washed in distilled water, oven dried (110°C, 20 h) and weighed. Specific growth rate (SGR, % DW/day) was calculated as (Ln DWf-Ln DWi) 100/(tf-ti), where DWf and DWi are the dry weight at final time (tf) and initial time (ti) respectively. Survival was assessed in both experiments at the termination of the experiment. Survival (S, %) was calculated as S = 100 Xf/(Xi-Xs), where Xf is the number of live individuals at the end of experiment, Xi is the initial number of individuals and Xs is the number of individuals sacrificed during the experiment.
Sample Collection
Due to the usual mortality in this type of culture and the large size of samples that require analyses, sampling could only be extended up to 12 days. Thus, sample collections in experiment 1 were carried out at 0, 3, 6, 9, and 12 days.
Likewise in the second experiment, samples were taken at 12 days from C and LC groups, and also at 28 days in LC groups since this treatment showed greater survival allowing for a suitable sample size.
Pools of 300 paralarvae per tank were taken in each sampling. Paralarvae were euthanized using ice seawater (−2°C), frozen in liquid nitrogen and stored at −80°C until further analysis.
Enzyme Assays
Pooled paralarvae of each sample were homogenized in four volumes of ice-cold 100 mM Tris-HCl buffer containing 0.1 mM EDTA and 0.1% (v/v) Triton X-100, pH 7.8. All procedures were performed on ice. Homogenates were centrifuged at 30,000 × g for 30 min at 4°C and the resultant supernatants were kept in aliquots and stored at −80°C for further enzyme assays.
All enzyme assays were performed at 25°C using a PowerWaveX microplate scanning spectrophotometer (Bio-Tek Instruments, Inc., USA) and run in duplicate in 96-well microplates (UVStar Greiner Bio-One, Germany). The optimal substrate and protein concentrations for the measurement of maximal activity for each enzyme in each tissue were established by preliminary assays. The millimolar extinction coefficients used for NADH/NADPH and DTNB, were 6.22 and 13.6 mM−1 cm−1, respectively.
Activities of fructose 1,6-bisphosphatase (FBPase; EC 3.1.3.11), glycerol kinase (GyK; EC 2.7.1.30), pyruvate kinase (PK, EC 2.7.1.40), glucose-6-phosphate dehydrogenase (G6PDH; EC 1.1.1.49), citrate synthase (CS; EC 4.1.3.7), β-hydroxyacyl CoA dehydrogenase (HOAD; EC 1.1.1.35), glutamate pyruvate transaminase (GPT; EC 2.6.1.2), glutamate oxaloacetate transaminase (GOT; EC 2.6.1.1), and glutamate dehydrogenase (GDH; EC 1.4.1.2) were determined as previously described by Hidalgo et al. (2017). Octopine dehydrogenase (ODH; EC 1.5.1.11), and lactate dehydrogenase (LDH, EC 1.1.1.27) were assayed according to the method of Baldwin and England (1980). See Supplementary Material provided for detailed assay conditions in a final volume of 200 microliters.
Soluble protein concentration in homogenates was analyzed using the method of Bradford (1976), with bovine serum albumin used as standard.
Data Analysis and Statistic
Data were checked for normal distribution with one-sample Kolmogorov-Smirnoff test, as well as for homogeneity of variances with the Levene's test (Zar, 1999) and transformed (natural logarithm) when needed (Fowler et al., 1998). Differences between two groups were assessed by Student's t-test. Multiple comparisons in experiment 1 were performed by mean of one-way ANOVA test and Tukey's HSD post hoc test. When normal distribution and/or homoscedasticity were not achieved, data were subjected to Kruskall–Wallis non-parametric test, followed by Games-Howell non-parametric multiple comparison test (Zar, 1999). Statistical significance was established at P < 0.05. Statistical analyses were performed using the SPSS package version 15.0 (SPSS Inc., Chicago, USA).
Results
Table 2 shows the time course of metabolic capacities during the first 12 days of life: newly hatched (0), 3, 6, 9, and 12 day old paralarvae (Experiment 1).

Table 2. Ontogenic changes in the activity of key enzymes of intermediary metabolism in Octopus vulgaris palarvae.
The PK (glycolysis), G6PDH (NADPH provision), CS (oxidative metabolism), and GPT (amino acid catabolism) activities did not show significant changes during the period analyzed. Nevertheless, FBPase activity decrease at day 3, to subsequently increase and remain unchanged until the end of the experimental period.
Activity of enzymes involved in anaerobic metabolism revealed that LDH reached maximum activity on day 6, after decreasing to values similar to the 0 day. ODH activity increased progressively from day 3 of life, reaching a significant maximum at 12 days old.
Activity of β-oxidation of fatty acids (HOAD) showed a significant increase from day 6 onwards. Enzymes involved in protein metabolism, specifically, the activity of GOT, showed significant increase from day 3 post-hatching, remaining significantly higher until day 9. However, at day 12 the activity was similar to that of day 0. In turn, GDH activity increased gradually, reaching statistical significance at day 9 post-hatching. Again on day 12, activity significantly decreased.
The HOAD/CS ratio increased since the sixth day forward, and the GPT/CS ratio was higher in newly hatched paralarvae and decreased from the third day onwards (Figure 1).
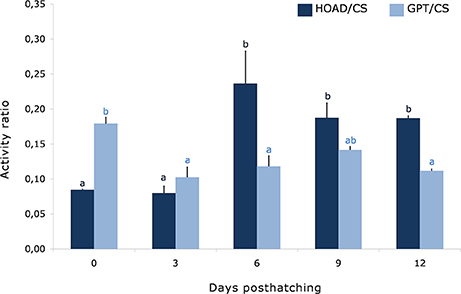
Figure 1. Time course of the ratio between glutamate pyruvate transaminase and citrate synthase (GPT/CS), and β-hydroxyacyl CoA dehydrogenase and citrate synthase (HOAD/CS) activities in octopus paralarvae from Experiment 1. Different superscripts indicate significant differences between sampling points for each parameter (n = 3).
In experiment 2, the effect of feeding with Artemia enriched with marine phospholipids on metabolic enzyme activity of hatchlings, 12 day and 28 day old paralarvae was studied. Table 3 shows the results of dry weight (DW), specific growth rate (SGR), and survival (S) at the hatchling stage, 12 day, and 28 day-old paralarvae fed with Artemia enriched with phytoplankton (Control Diet) or Artemia enriched with Marine Lecithin (LC diet). The results showed that DW was significantly higher in paralarvae from the LC group compared to the control group at 12 days old (P < 0.05). Also, survival in 28 day old paralarvae was significantly higher in the LC group compared to the control group (P < 0.05).
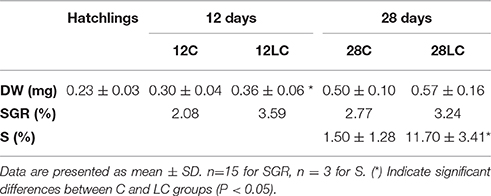
Table 3. Dry weight (DW), specific growth rate (SGR), and survival (S) in common octopus paralarvae at hatching and reared for 12 and 28 days with control diet (C, Artemia enriched with phytoplankton) or LC diet (Artemia enriched with Marine Lecithin).
Table 4 shows the results of metabolic enzyme activities in newly hatched paralarvae, 12 day old paralarvae fed on microalgae-enriched (12C) and on LC60-enriched (12LC) Artemia, and in 28 day old paralarvae fed on LC60-enriched Artemia (28LC). Of these activities, ODH, CS, HOAD, and GOT increased significantly in 12 day old paralarvae. The only difference among 12C and 12LC paralarvae was the significantly higher GyK activity in 12C. The 28 day old paralarvae fed on LC60-enriched Artemia (28LC) showed significant increases in GyK, PK, G6PDH, LDH, GOT, and GDH activities with respect to 12LC.
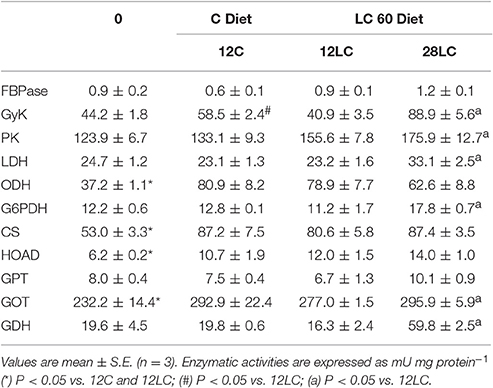
Table 4. Activity of key enzymes of intermediary metabolism in Octopus vulgaris palarvae at hatching (0) and after 12 and 28 days of feeding with control diet (C, Artemia enriched with phytoplankton) or LC60 diet (Artemia enriched with Marine Lecithin).
Discussion
Time Course of Metabolic Capacities during the First 12 Days of Life
Common octopus paralarvae have a planktonic lifestyle that lasts until the first 30–60 days of life. Remaining yolk reserves provide energy substrates during the first few days post-hatching, however paralarvae immediately begin to actively capture live prey (Iglesias et al., 2007). This simultaneous use of endogenous reserves and exogenous food in cephalopods may last up to several days or weeks (Boletzky and Villanueva, 2014). Specifically, this type of feeding pattern has been shown in the common octopus to last approximately 5 days (Nande et al., 2017). Once the remaining yolk reserves are exhausted, paralarvae depend on active foraging involving episodes of burst swimming under anaerobic conditions (Baldwin, 1982). Our results indicated that both LDH and ODH provide energy to support such episodes of burst swimming during the first days of life. However, by day 9 ODH adopts a predominant role while LDH activity decreases. Therefore, the relative role of both systems in providing energy would be opposite to what has been observed in vertebrates (Lyzlova and Stefanov, 1991). This increase in anaerobic activity from day 9 could be due to the depletion of yolk reserves that would force paralarvae to increase their swimming activity for prey capture. Most mollusks only express ODH activity, and in the few cases where LDH is also present, ODH exhibits a higher level of activity (Regnouf and van Thoai, 1970; Gäde, 1980). Additionally, in a recent study on the enzymatic capacities of juvenile cuttlefish, no LDH activity was reported (Speers-Roesch et al., 2016). The coexistence of both pathways indicates that glycogen and arginine phosphate deposits in mantle muscle are used during burst swimming. Sustained PK activity would ensure the provision of pyruvate necessary for both reactions. Regarding the role of ODH, octopine and arginine are among the predominant free amino acids in cephalopod tissue (Lee, 1994). It has been reported that production of octopine might be advantageous in maintaining a low cytosolic redox ratio and, thus, glycolysis (Fields and Quinn, 1981) as well as intracellular osmotic pressure (Fields, 1983). Arginine has been reported to be the most abundant free amino acid in common octopus paralarvae (Villanueva et al., 2004), ensuring adequate levels of arginine phosphate and substrate for ODH. The importance of glycogen deposits in cephalopod muscle have been noted in the literature (O'Dor et al., 1984; Lee, 1994), and confirmed by LDH activity in the present study.
Once episodes of burst swimming ends, it is necessary for paralarvae to restore glycogen and arginine phosphate pools. However, data showing the importance of gluconeogenesis in cephalopods are scarce and contradictory. For example, where some studies indicate that gluconeogenesis occurs in several cephalopod tissues (Ballantyne et al., 1981; Fields and Hochachka, 1982; Hochachka and Fields, 1982), a recent study by Speers-Roesch et al. (2016) reported that de novo glucose synthesis is restricted to the digestive gland of juvenile cuttlefish, although the carbon source was unclear. In the present study, after an initial decrease at day 3, a significant increase occurred from day 6 post-hatching. Such increased activity, in parallel to that reported for anaerobic activity, might indicate an increased glycogen use or deposition. Based on the activity of transaminases and GyK in the present study, the substrate for glucose synthesis might be both amino acids and glycerol. It has been amply reported that amino acids are excellent gluconeogenic substrates that are incorporated into glycogen deposits of cephalopod mantle (Hochachka and Fields, 1982). Likewise, transaminases, mainly GOT, are among the enzymes that show higher specific activity in nature. The high GyK activity in hatched paralarvae seems consistent with a high hydrolysis of yolk lipids, resulting in glycerol incorporation into gluconeogenic/glycolytic pathways. Likewise, composition of yolk in cephalopods seems to include approximately 15% lipids (Caamal-Monsreal et al., 2015; Matozzo et al., 2015). Bouchaud and Galois (1990) reported that glyceryl monoesters are abundant in cuttlefish yolk and that probably plays an important role in energy metabolism. The slight decline in GyK activity until the sixth day post-hatching could be due to the depletion of yolk reserves while the subsequent increase may be attributed to greater availability of glycerol derived from catabolism of triacylglycerol present in live prey (Iglesias and Fuentes, 2014). Regarding the use of glycerol as a metabolic intermediate, overexpression of GAPDH has been reported in 4 day old common octopus paralarvae (Varó et al., 2017), which may be related to a higher rate of incorporation of G3P derived from GyK, to gluconeogenesis/glycolysis. Also, GAPDH would provide the NADH required for ODH and LDH reactions. Therefore, the present results seem to indicate that paralarvae develop metabolic capacities in a short period of time. A poor catabolic capacity would lead to a deficit of energy in the development of burst swimming which is necessary for capturing prey. These results also confirm the necessity of early metabolic capacity to fuel the capture of prey, even when yolk reserves have not been exhausted.
In addition to the energy demands imposed by the capture of prey supported by anaerobic metabolism, paralarvae need a supply of energy for growth, food processing, and maintenance of routine activity. These demands are typically supported by aerobic metabolism. In the present study, CS activity did not show significant changes during the ontogenic period analyzed, although the slight tendency to increase from third day could be related to growth and development processes that impose greater energy needs. GOT activity increased significantly in parallel to the observed changes in CS, indicating a positive correlation between both activities (r = 0.802, P < 0.001). This would ensure the provision of oxaloacetate necessary for the CS reaction. The data indicate that aspartate (a substrate of GOT) and glutamate, representing almost half of the non-essential amino acids in the body of cephalopods, are relevant (Villanueva et al., 2004). Protein catabolism in cephalopods has been widely accepted as the necessary fuel for aerobic metabolism, regardless of the body mass of the animal (Boucher-Rodoni and Mangold, 1985; Lee, 1994; Katsanevakis et al., 2005; Petza et al., 2006). This would imply a high requirement of this macronutrient in food (Houlihan et al., 1990; Navarro et al., 2014). Sustained GPT and GDH activities would also reflect active protein catabolism during the ontogenic period analyzed. However, the GPT/CS ratio (Figure 1) is higher in newly hatched paralarvae, possibly related to the predominance of the catabolism of protein, consisting of the major constituent of cephalopod yolk (Quintana et al., 2015). The reduced GPT/CS ratio from the third day onwards seems to indicate that part of the acetyl CoA incorporated to the CS reaction would come from a source different to amino acids. In this way, both HOAD activity and the HOAD/CS ratio (Figure 1) clearly show that since the sixth day forward a significant increase of fatty acid oxidation occurs. It has been widely accepted that cephalopods have a low capacity for lipid metabolism (Ballantyne et al., 1981; Storey and Storey, 1983; O'Dor and Webber, 1986; Lee, 1994; Hochachka, 1995), and as such, the requirement for lipid in food was very low (Seixas, 2009). However, Speers-Roesch et al. (2016) recently reported that cuttlefish efficiently use lipid-based fuels. The present study also shows that, at least during this phase, fatty acids are actively oxidized by common octopus paralarvae. The significant lower HOAD activity detected the first days of life would indicate a predominance of protein catabolism, as described above, probably due to the small proportion of lipid content in yolk and the higher protein content (Quintana et al., 2015).
Influence of Food Composition on Metabolic Capacities and Survival
The main result in this nutritional assay was that the paralarvae that were fed on LC60-enriched Artemia showed a 12% survival rate after 28 days, whereas less than 2% of the C group survived before that day. The results for SGR in the present study were similar to those reported previously for O. vulgaris paralarvae by Seixas et al. (2010) and Villanueva et al. (2004) using HUFA-enriched Artemia as live prey. Conversely, SGR results were lower than those previously found when LC60-enriched Artemia was provided (Garrido et al., 2017). However, SGR in both 12 and 28 day old paralarvae were higher in LC groups than in C groups. Thus, these data would indicate that food enrichment provided a beneficial effect on the welfare of paralarvae, although those paralarvae remaining after the 28 day sampling did not survive much longer.
Regarding the possible beneficial effect of food enrichment, in spite of the increased lipid content of LC60-enriched Artemia, oxidation rate of fatty acids did not increase in LC group, either at 12 or 28 days. This result may indicate that rather than being used as fuel, the higher amount of lipids could be contributing to the development of the nervous system, essential in such early stages (Nixon and Mangold, 1998). In this sense, the lipid-rich nervous system of O. vulgaris paralarvae hatchlings represents approximately one quarter of the animal's fresh weight (Packard and Albergoni, 1970). This may indicate the role of lipids for suitable growth during planktonic life. Increased G6PDH activity observed in 28LC groups would also contribute to such biosynthetic processes. Also, free glycerol derived from triacylglycerol hydrolysis might be used as substrate for glycolysis as indicates by the result of GyK, PK, and LDH at day 28. Increasing lipid levels in food would be not exerting the sparing effect of protein for growth amply reported for fish (Watanabe, 2002). According to Houlihan et al. (1990) and Moltschaniwskyj and Carter (2010), such a sparing effect of protein should give a high rate of protein synthesis and low protein degradation. However, 28 day old paralarvae showed a significant increase in GDH activity. It has been reported that the primary role of GDH in mantle muscle of squid is to regulate the catabolism of amino acids for energy production (Storey et al., 1978). The increased amino acid catabolism, registered in 28LC paralarvae, might be indicative of some kind of nutritional deficiency/imbalance.
In searching for a possible metabolic biomarker, GyK might be considered, since a significant increased activity was observed in both 12C and 28LC groups and a few days later an event of massive mortality took place. Although the role of G3P in cephalopods is controversial (Storey and Hochachka, 1975; Zammit and Newsholme, 1976), this metabolic intermediate can act as a carrier of cytosolic NADH to the mitochondrial electron transport chain. This shuttle activity is thought to coordinate glycolytic and mitochondrial metabolism in highly active tissues and is linked to ROS generation in mammals (Orr et al., 2012). Overproduction of ROS has been reported to exert detrimental effects on aquatic organisms (Abele et al., 2012). Hence, an increased G3P production might be linked to oxidative stress responsible for the low paralarvae survival.
Conclusion
In summary, throughout the ontogenic period studied, an increase in anaerobic metabolism takes place, probably related to the increased dependence of paralarvae on exogenous food. Such activity is mainly supported by ODH activity, although LDH activity also contributes to the process. Regarding fuels that support aerobic metabolism, the use of amino acids was maintained and a significant increase in the rate of fatty acid oxidation was observed from the sixth day post-hatching. A low, albeit sustained, capacity for de novo synthesis of glucose from amino acids and glycerol was also observed. Feeding of larvae with phospholipid-enriched Artemia improved animal welfare, since there was a significant increase in the rate of survival and growth. Regardless of the composition of the food, GyK activity significantly increased a few days prior to a massive mortality event. This data may, in fact, indicate an imbalance in the redox state that could be responsible for the observed high mortality. Thus, GyK might be used as a nutritional and welfare biomarker.
Author Contributions
AM Analytical procedures, interpretation of the findings, and design, writing, and revision of the manuscript. GC Analysis, interpretation of the findings, and design, writing, and revision of the manuscript. MH Analysis and interpretation of the findings, and revision of the manuscript. DG and MM octopus paralarvae cultures and execution of the experiments, revision of the manuscript. EA design and execution of experiments, interpretation of results and revision of the manuscript. All authors read and approved the submitted manuscript.
Funding
This research has been supported by the Ministerio de Economía y Competitividad (Spanish Government) through the Projects OCTOWELF (AGL2013-49101-C2-1-R and AGL2013-49101-C2-2-R). The authors acknowledge COST for funding the Action FA1301 “A network for improvement of cephalopod welfare and husbandry in research, aquaculture and fisheries (CephsInAction),” supporting this work. DG was financed by Ph.D. grant by Spanish Institute of Oceanography (MINECO, Spanish Government) (BOE 3rd November 2011).
Conflict of Interest Statement
The authors declare that the research was conducted in the absence of any commercial or financial relationships that could be construed as a potential conflict of interest.
The reviewer AT and handling Editor declared their shared affiliation, and the handling Editor states that the process nevertheless met the standards of a fair and objective review.
Acknowledgments
The authors acknowledge FRONTIERS for supporting part of the costs of the present publication.
Supplementary Material
The Supplementary Material for this article can be found online at: http://journal.frontiersin.org/article/10.3389/fphys.2017.00427/full#supplementary-material
References
Abele, T., Vázquez-Medina, J. P., and Zenteno-Savín, T. (2012). Oxidative Stress in Aquatic Ecosystems. England: Wiley-Blackwell.
Baldwin, J. (1982). Correlations between enzyme profiles in cephalopods muscle and swimming behavior. Pac. Sci. 36, 349–356.
Baldwin, J., and England, W. R. (1980). A comparison of anaerobic energy metabolism in mantle and tentacle muscle of the blue-ringed octopus, Hapalochlaena maculosa, during swimming. Aust. J. Zool. 28, 407–412. doi: 10.1071/ZO9800407
Ballantyne, J. S., Hochachka, P. W., and Mommsen, T. P. (1981). Studies on the metabolism of the migratory squid, Loligo opalescens: enzymes of tissues and heart mitochondria. Mar. Biol. Lett. 2, 75–85.
Boletzky, S., and Villanueva, R. (2014). “Cephalopod biology,” in Cephalopod Culture, ed J. Iglesias, L. Fuentes, and R. Villanueva (Dordrecht: Springer), 3–16.
Bouchaud, O., and Galois, R. (1990). Utilization of egg-yolk lipids during the embryonic development of Sepia officinalis L. in relation to temperature of the water. Comp. Biochem. Physiol. 97B, 611–615. doi: 10.1016/0305-0491(90)90168-s
Boucher-Rodoni, R., and Mangold, K. (1985). Ammonia excretion during feeding and starvation in Octopus vulgaris. Mar. Biol. 86, 193–197. doi: 10.1007/BF00399026
Bradford, M. M. (1976). A rapid and sensitive method for the quantification of microgram quantities of protein utilizing the principle of protein dye-binding. Anal. Biochem. 72, 248–254. doi: 10.1016/0003-2697(76)90527-3
Caamal-Monsreal, C., Mascaró, M., Gallardo, P., Rodríguez, S., Nore-a-Barroso, E., Domingues, P., et al. (2015). Effects of maternal diet on reproductive performance of O. maya and its consequences on biochemical characteristics of the yolk, morphology of embryos and hatchling quality. Aquaculture 441, 84–94. doi: 10.1016/j.aquaculture.2015.01.020
Fields, J. H. A. (1983). Alternatives to lactic acid: possible advantages. J. Exp. Zool. 228A, 445–457. doi: 10.1002/jez.1402280306
Fields, J. H. A., and Hochachka, P. W. (1982). Glucose and proline metabolism in Nautilus. Pac. Sci. 36, 337–341.
Fields, J. H. A., and Quinn, J. F. (1981). Some theoretical considerations on cytosolic redox balance during anaerobiosis in marine invertebrates. J. Theor. Biol. 88, 35–45. doi: 10.1016/0022-5193(81)90327-1
Fiorito, G., Affuso, A., Basil, J., Cole, A., de Girolamo, P., D'Angelo, L., et al. (2015). Guidelines for the care and welfare of cephalopods in research -a consensus based on an initiative by CephRes, FELASA and the Boyd Group. Lab. Anim. 49, 1–90. doi: 10.1177/0023677215580006
Fowler, J., Cohen, L., and Jarvis, P. (1998). Practical Statistics for Field Biology, 2nd Edn. England: Wiley.
Gäde, G. (1980). A comparative study of octopine dehydrogenase isoenzymes in gastropod, bivalve and cephalopod mollusks. Comp. Biochem. Physiol. 67B, 575–582.
Garrido, D., Varó, I., Morales, A. E., Hidalgo, M. C., Navarro, J. C., Hontoria, F., et al. (2017). Assessment of stress and nutritional biomarkers in cultured Octopus vulgaris paralarvae: effects of geographical origin and dietary regime. Aquaculture 468, 558–568. doi: 10.1016/j.aquaculture.2016.11.023
Hidalgo, M. C., Morales, A. E., Arizcun, M., Abellán, E., and Cardenete, G. (2017). Regional asymmetry of metabolic and antioxidant profile in the sciaenid fish shi drum (Umbrina cirrosa) white muscle. Response to starvation and refeeding. Redox. Biol. 11, 682–687. doi: 10.1016/j.redox.2017.01.022
Hochachka, P. W. (1995). Oxygen efficient design of cephalopod muscle metabolism. Mar. Freshw. Behav. Physiol. 25, 61–67. doi: 10.1080/10236249409378908
Hochachka, P. W., and Fields, J. H. A. (1982). Arginine, glutamate, and proline as substrates for oxidation and for glycogenesis in cephalopod tissues. Pac. Sci. 36, 325–335.
Houlihan, D. F., McMillan, D. N., Agnisola, C., Genoin, I. T., and Foti, L. (1990). Protein synthesis and growth in Octopus vulgaris. Mar. Biol. 106, 251–259. doi: 10.1007/BF01314808
Iglesias, J., and Fuentes, L. (2014). “Octopus vulgaris. paralarval culture,” in Cephalopod Culture, ed J. Iglesias, L. Fuentes, and R. Villanueva (Dordrecht: Springer), 427–450.
Iglesias, J., Sánchez, F. J., Bersano, J. G. F., Carrasco, J. F., Dhont, J., Fuentes, L., et al. (2007). Rearing of Octopus vulgaris paralarvae: present status, bottlenecks and trends. Aquaculture 266, 1–15. doi: 10.1016/j.aquaculture.2007.02.019
Katsanevakis, S., Stephanopoulou, S., Miliou, H., Moraitou-Apostolopoulou, M., and Verriopoulos, G. (2005). Oxygen consumption and ammonia excretion of Octopus vulgaris (Cephalopoda) in relation to body mass and temperature. Mar. Biol. 146, 725–732. doi: 10.1007/s00227-004-1473-9
Lee, P. G. (1994). Nutrition of cephalopods: fueling the system. Mar. Freshw. Behav. Physiol. 25, 35–51. doi: 10.1080/10236249409378906
Matozzo, V., Conenna, I., Riedl, V. M., Marin, M. G., Marčeta, T., and Mazzoldi, C. (2015). A first survey on the biochemical composition of egg yolk and lysozyme-like activity of egg envelopment in the cuttlefish Sepia officinalis from the Northern Adriatic Sea (Italy). Fish Shellfish Inmunol. 45, 528–533. doi: 10.1016/j.fsi.2015.04.026
Moltschaniwskyj, N. A., and Carter, C. G. (2010). Protein synthesis, degradation, and retention: mechanisms of indeterminate growth in cephalopods. Physiol. Biochem. Zool. 83, 997–1008. doi: 10.1086/656387
Nande, M., Iglesias, J., Domingues, P., and Pérez, M. (2017). Effect of temperature on energetic demands during the last stages of embryonic development and early life of Octopus vulgaris (Cuvier, 1797) paralarvae. Aquacult. Res. 48, 1951–1961. doi: 10.1111/are.13032
Navarro, J. C., Monroig, O., and Sykes, A. V. (2014). “Nutrition as a key factor for cephalopod aquaculture,” in Cephalopod Culture, ed J. Iglesias, L. Fuentes, and R. Villanueva (Dordrecht: Springer), 77–96.
Nixon, M., and Mangold, K. (1998). The early life of Sepia officinalis, and the contrast with that of Octopus vulgaris (Cephalopoda). J. Zool. 245, 407–421. doi: 10.1111/j.1469-7998.1998.tb00115.x
O'Dor, R. K., Mangold, K., Boucher-Rodoni, R., Wells, M. J., and Wells, J. (1984). Nutrient absorption, storage and remobilization in Octopus vulgaris. Mar. Behav. Physiol. 11, 239–258. doi: 10.1080/10236248409387049
O'Dor, R. K., and Webber, D. M. (1986). The constraints on cephalopods: why squid aren't fish. Can. J. Zool. 64, 1591–1605. doi: 10.1139/z86-241
O'Dor, R. K., and Wells, M. J. (1987). “Energy and nutrient flow in cephalopods,” in Cephalopod Life Cycles, Vol. 2, ed P. Boyle (London: Academic Press), 109–133.
Orr, A. L., Quinlan, C. L., Perevoshchikova, I. V., and Brand, M. D. (2012). A refined analysis of superoxide production by mitochondrial sn-glycerol 3-phosphate dehydrogenase. J. Biol. Chem. 287, 42921–42935. doi: 10.1074/jbc.M112.397828
Packard, A., and Albergoni, V. (1970). Relative growth, nucleic acid content and cell numbers of brain in Octopus vulgaris (Lamarck). J. Exp. Biol. 52, 539–552.
Petza, D., Katsanevakis, S., and Verriopoulos, G. (2006). Experimental evaluation of the energy balance in Octopus vulgaris, fed ad libitum on a high-lipid diet. Mar. Biol. 148, 827–832. doi: 10.1007/s00227-005-0129-8
Quintana, D., Márquez, L., Arévalo, J. R., Lorenzo, A., and Almansa, E. (2015). Relationships between spawn quality and biochemical composition of eggs and hatchlings of Octopus vulgaris under different parental diets. Aquaculture 446, 206–216. doi: 10.1016/j.aquaculture.2015.04.023
Regnouf, F., and van Thoai, N. (1970). Octopine and lactate dehydrogenases in mollusc muscles. Comp. Biochem. Physiol. 32, 411–416. doi: 10.1016/0010-406X(70)90458-5
Seixas, P. (2009). Composición Bioquímica y Crecimiento de Paralarvas de Pulpo (Octopus vulgaris Cuvier, 1797), Alimentadas Con Juveniles de Artemia Enriquecidos Con Microalgas y otros Suplementos Nutricionales. PhD thesis. University of Santiago de Compostela. 259.
Seixas, P., Otero, A., Valente, L. M. P., Dias, J., and Rey-Méndez, M. (2010). Growth and fatty acid composition of Octopus vulgaris paralarvae fed with enriched Artemia or co-fed with an inert diet. Aquacult. Int. 18, 1121–1135. doi: 10.1007/s10499-010-9328-5
Speers-Roesch, B., Callaghan, N. I., MacCormack, T. J., Lamarre, S. G., Sykes, A. V., and Driedzic, V. R. (2016). Enzymatic capacities of metabolic fuel use in cuttlefish (Sepia officinalis) and responses to food deprivation: insight into the metabolic organization and starvation survival strategy of cephalopods. J. Comp. Physiol. 186B, 711–725. doi: 10.1007/s00360-016-0991-3
Storey, K. B., Fields, J. H. A., and Hochachka, P. W. (1978). Purification and properties of glutamate dehydrogenase from the mantle muscle of the squid, Loligopealeii. Role of the enzyme in energy production from amino acids. J. Exp. Zool. 205A, 111–118. doi: 10.1002/jez.1402050113
Storey, K. B., and Hochachka, P. W. (1975). Squid muscle glyceraldehyde-3-P dehydrogenase: control of the enzyme in a tissue with an active alpha-GP cycle. Comp. Biochem. Physiol. 52B, 179–182.
Storey, K. B., and Storey, J. M. (1983). “Carbohydrate metabolism in cephalopod mollusks,” in The Mollusca, vol. 1, ed P. W. Hochachka and K. M. Wilbur (New York, NY: Academic Press), 91–136.
Varó, I., Cardenete, G., Hontoria, F., Monroig, O., Iglesias, J., Otero, J. J., et al. (2017). Dietary effect on the proteome of the common octopus (Octopus vulgaris) paralarvae. Front. Physiol. 8:309. doi: 10.3389/fphys.2017.00309
Villanueva, R., Riba, J., Ruíz-Capillas, C., González, A. V., and Baeta, M. (2004). Amino acid composition of early stages of cephalopods and effect of amino acid dietary treatments on Octopus vulgaris paralarvae. Aquaculture 242, 455–478. doi: 10.1016/j.aquaculture.2004.04.006
Watanabe, T. (2002). Strategies for further development of aquatic feeds. Fish. Sci. 68, 242–252. doi: 10.1046/j.1444-2906.2002.00418.x
Zammit, V. A., and Newsholme, E. A. (1976). The maximum activities of hexokinase, phosphorylase, phosphofructokinase, glycerol phosphate dehydrogenases, lactate dehydrogenase, octopine dehydrogenase, phosphoenolpyruvate carboxykinase, nucleoside diphosphatekinase, glutamate-oxaloacetate transaminase and arginine kinase in relation to carbohydrate utilization in muscles from marine invertebrates. Biochem. J. 160, 447–462. doi: 10.1042/bj1600447
Keywords: Octopus vulgaris, paralarvae, metabolic organization, nutritional imbalance, biomarkers
Citation: Morales AE, Cardenete G, Hidalgo MC, Garrido D, Martín MV and Almansa E (2017) Time Course of Metabolic Capacities in Paralarvae of the Common Octopus, Octopus vulgaris, in the First Stages of Life. Searching Biomarkers of Nutritional Imbalance. Front. Physiol. 8:427. doi: 10.3389/fphys.2017.00427
Received: 06 March 2017; Accepted: 01 June 2017;
Published: 16 June 2017.
Edited by:
Graziano Fiorito, Stazione Zoologica Anton Dohrn, ItalyReviewed by:
Andrea Tarallo, Stazione Zoologica Anton Dohrn, ItalyClaudio Agnisola, University of Naples Federico II, Italy
Copyright © 2017 Morales, Cardenete, Hidalgo, Garrido, Martín and Almansa. This is an open-access article distributed under the terms of the Creative Commons Attribution License (CC BY). The use, distribution or reproduction in other forums is permitted, provided the original author(s) or licensor are credited and that the original publication in this journal is cited, in accordance with accepted academic practice. No use, distribution or reproduction is permitted which does not comply with these terms.
*Correspondence: Amalia E. Morales, YW1hZW5jYUB1Z3IuZXM=