- Departamento de Educação Física e Ciências do Esporte, Centro Acadêmico de Vitória, Universidade Federal de Pernambuco, Pernambuco, Brazil
Developmental origins of cardiometabolic diseases have been related to maternal nutritional conditions. In this context, the rising incidence of arterial hypertension, diabetes type II, and dyslipidemia has been attributed to genetic programming. Besides, environmental conditions during perinatal development such as maternal undernutrition or overnutrition can program changes in the integration among physiological systems leading to cardiometabolic diseases. This phenomenon can be understood in the context of the phenotypic plasticity and refers to the adjustment of a phenotype in response to environmental input without genetic change, following a novel, or unusual input during development. Experimental studies indicate that fetal exposure to an adverse maternal environment may alter the morphology and physiology that contribute to the development of cardiometabolic diseases. It has been shown that both maternal protein restriction and overnutrition alter the central and peripheral control of arterial pressure and metabolism. This review will address the new concepts on the maternal diet induced-cardiometabolic diseases that include the potential role of the perinatal malnutrition.
Introduction
Cardiovascular and metabolic diseases, such as hypertension, type II diabetes, and dyslipidemia are highly prevalent in the world and have important effects on the public health, increasing risk factors for the development of other diseases, including coronary heart disease, stroke, and heart failure (Landsberg et al., 2013). The etiology of these cardiometabolic diseases includes a complex phenotype that arises from numerous genetic, environmental, nutritional, behavioral, and ethnic origins (Landsberg et al., 2013; Ng et al., 2014). In this regard, it has been observed that the eating habits and behaviors and nutritional condition in early phases of life may play a key role on the etiology of these diseases by inducing physiological dysfunctions (Lucas, 1998; Victora et al., 2008; Wells, 2012). This phenomenon can be understood in the context of phenotypic plasticity and it refers to the ability of an organism to react to both an internal and external environmental inputs with a change in the form, state, physiology, or rate of activity without genetic changes (West-Eberhard, 2005b). Indeed the nutritional factors rise as important element in this theme and it has been highlighted since Barker (Barker, 1990, 1994, 1995, 1998, 1999a,b, 2000; Barker and Martyn, 1992; Fall and Barker, 1997; Osmond and Barker, 2000). In this context, new evidence from epidemiological and clinical studies have showed the association of the maternal under- and overnutrition with development of cardiometabolic dysfuntions (Ashton, 2000; Hemachandra et al., 2006; Antony and Laxmaiah, 2008; Conde and Monteiro, 2014; Costa-Silva et al., 2015; Parra et al., 2015). Thus, this review will address the new concepts about the involvement of the maternal protein malnutrition and overnutrition on the development of the cardiometabolic diseases.
Perinatal Origin of Cardiometabolic Diseases: The Role of Phenotypic Plasticity
Biological and medical consequences of perinatal nutritional factors have been extensively studied in the field of the “developmental origins of health and diseases” proposed by Barker and colleagues since 1986 (Barker and Osmond, 1986; Barker et al., 1989, 1993; Barker, 2007). This field of research proposes that cardiometabolic diseases can be “programmed” by the “adaptative” effects of both under- and overnutrition during early phases of growth and development on the cell physiology (Barker and Osmond, 1986; Hales and Barker, 1992; Alfaradhi and Ozanne, 2011; Chavatte-Palmer et al., 2016). As stated before, it aims to study how an organism reacts to a different environmental input, such as malnutrition, and induces changes in the phenotype, but without altering the genotype (Barker et al., 2005; West-Eberhard, 2005a; Labayen et al., 2006; Andersen et al., 2009; Biosca et al., 2011). In this context, epigenetic alterations, such as DNA methylation, histone acetylation, and microRNA expression are considered the molecular basis of the phenotypic plasticity (Wells, 2011). These modifications termed as “epigenetic” were firstly described by Conrad Waddigton in 1940 and it studies the relationship between cause and effect in the genes to produce a phenotype (Jablonka and Lamb, 2002). Nowadays, this concept is employed to describe the process of the gene expression and its linking to modifications in the cromatin structure without altering DNA sequence (Chong and Whitelaw, 2004; Egger et al., 2004). Among all epigenetic modifications, the DNA methylation is one that has been best studied and is related to addition of methyl groups on DNA cytosine residues, normally on the cytosine followed by guanine residue (CpG dinucleotides), which can produce inhibition of the gene expression by impairing transcriptional factor binding (Waterland and Michels, 2007; Mansego et al., 2013; Chango and Pogribny, 2015; Mitchell et al., 2016). In this context, it has been investigated how nutritional aspect may induce these epigenetic modifications.
Macro- and micro-nutrient compositions have been identified as important nutritional factors inducing epigenetic processes, such as DNA methylation (Mazzio and Soliman, 2014; Szarc vel Szic et al., 2015). It is considered at least three ways by which nutrients can induce DNA methylation, alter gene expression, and modify cellular phenotype: (i) by providing methyl group supply for inducing S- adenosyl-L-methionine formation (genomic DNA methylation), modifying the methyltransferase activity, or impairing DNA demethylation process; (ii) by modifying chromatin remodeling, or lysine and arginine residues in the N-terminal histone tails; and (iii) by altering microRNA expression (Chong and Whitelaw, 2004; Egger et al., 2004; Hardy and Tollefsbol, 2011; Stone et al., 2011). In this context, altered contents of amino acids, such as methionine and cysteine, as well as reduced choline and folate diet amount can modify the process of the DNA methylation leading to both DNA hyper- and hypomethylation (Fiorito et al., 2014). For example, deficiency of choline can precipitate DNA hypermethylation associated with organ dysfunction, mainly in liver metabolism (Karlic and Varga, 2011; Wei, 2013).
High fat diet (HFD) during perinatal period has been identified as risk factor to predispose and induce epigenetic processes in the parents and their offspring (Mazzio and Soliman, 2014; Szarc vel Szic et al., 2015). Both hypo- and hypermethylation processes participate in this dysregulation attributed to HFD consumption (Ng et al., 2010; Milagro et al., 2013). In adipose tissue, for example, it was observed that gene promoter of the fatty acid synthase enzyme suffered methylation (Lomba et al., 2010) and that important obesity-related genes such as leptin have disruption on their methylation status (Milagro et al., 2009).
Maternal Protein Undernutrition: Early- and Long-Term Outcomes
Maternal malnutrition is associated with the risk of developing cardiovascular disease and co-morbidities in offspring's later life including hypertension, metabolic syndrome, and type-II diabetes (Barker et al., 2007; Nuyt, 2008; Nuyt and Alexander, 2009). In humans, studies have provided support for the positive association between low birth weight and increased incidence of hypertension (Ravelli et al., 1976; Hales et al., 1991; Sawaya and Roberts, 2003; Sawaya et al., 2004).
Maternal low-protein diet model during both gestation and lactation is one of the most extensively studied animal models of phenotypic plasticity (Ozanne and Hales, 2004; Costa-Silva et al., 2009; Falcão-Tebas et al., 2012; Fidalgo et al., 2013; de Brito Alves et al., 2014; Barros et al., 2015). Feeding a low-protein diet (8% protein) during gestation and lactation is associated with growth restriction, asymmetric reduction in organ growth, elevated systolic blood pressure, dyslipidemia, and increased fasting plasma insulin concentrations in the most of studies in rodents (Ozanne and Hales, 2004; Costa-Silva et al., 2009; Falcão-Tebas et al., 2012; Fidalgo et al., 2013; Leandro et al., 2012; de Brito Alves et al., 2014, 2016; Ferreira et al., 2015; Paulino-Silva and Costa-Silva, 2016). However, it is known that the magnitude of the cardiovascular and metabolic outcomes are dependent on the both time exposure to protein restricted-diet (Zohdi et al., 2012, 2015) and growth trajectory throughout the postnatal period (Wells, 2007, 2011). A rapid and increased catch-up growth and childhood weight gain appear to augment metabolic disruption in end organs, for example liver (Tarry-Adkins et al., 2016; Wang et al., 2016).
Although, the relationship between maternal protein restriction, sympathetic overactivity and hypertension have been suggested (Johansson et al., 2007; Franco et al., 2008; Barros et al., 2015), few studies have described the physiological dysfunctions responsible for producing these effects. Nowadays, it is well accepted that perinatal protein malnutrition raise risks of hypertension by mechanisms that include abnormal vascular function (Franco Mdo et al., 2002; Brawley et al., 2003; Franco et al., 2008), altered nephron morphology and function, and stimulation of the renin-angiotensin system (RAS) (Nuyt and Alexander, 2009; Siddique et al., 2014). Recently, studies have highlighted contribution of the sympathetic overactivity associated to enhanced respiratory rhythm and O2/CO2 sensitivity on the development of the maternal low-protein diet-induced hypertension by mechanisms independent of the baroreflex function (Chen et al., 2010; Barros et al., 2015; Costa-Silva et al., 2015; de Brito Alves et al., 2015; Paulino-Silva and Costa-Silva, 2016). Offspring from dams subjected to perinatal protein restriction had relevant short-term effects on the carotid body (CB) sensitivity and respiratory control. With enhanced baseline sympathetic activity and amplified ventilatory and sympathetic responses to peripheral chemoreflex activation, prior to the establishment of hypertension (de Brito Alves et al., 2014, 2015). The underlying mechanism involved in these effects seems to be linked with up-regulation of hypoxic inducible factor (HIF-1α) in CB peripheral chemoreceptors (Ito et al., 2011, 2012; de Brito Alves et al., 2015). However, the epigenetic mechanisms in these effects are still unclear. It is hypothesized that epigenetic mechanism produced by DNA methylation could be involved (Altobelli et al., 2013; Prabhakar, 2013; Nanduri and Prabhakar, 2015).
The central nervous system (CNS) compared to other organ systems has increased vulnerability to reactive oxygen species (ROS). ROS are known to modulate the sympathetic activity and their increased production in key brainstem sites is involved in the etiology of several cardiovascular diseases, for example, diseases caused by sympathetic overexcitation, such as neurogenic hypertension (Chan et al., 2006; Essick and Sam, 2010). Ferreira and colleagues showed that perinatal protein undernutrition increased lipid peroxidation and decreased the activity of several antioxidant enzymes (superoxide dismutase, catalase, glutathione peroxidase, and glutathione reductase activities) as well as elements of the GSH system, in adult brainstem. Dysfunction in the brainstem oxidative metabolism, using the same experimental model, were observed in rats immediately after weaning associated to the increase in ROS production, with a decrease in antioxidant defense and redox status (Ferreira et al., 2015, 2016). Related to the metabolic effects on the heart, it was observed that these animals showed decreased mitochondrial oxidative phosphorylation capacity and increased ROS in the myocardium. In addition, maternal low-protein diet induced a significant decrease in enzymatic antioxidant capacity (superoxide dismutase, catalase, glutathione-S-transferase, and glutathione reductase activities) and glutathione level when compared with normoprotein group (Nascimento et al., 2014).
Regarding hepatic metabolism, studies showed that protein restricted rats had suppressed gluconeogenesis by a mechanism primarily mediated by decrease on the mRNA level of hepatic phosphoenolpyruvate carboxykinase, a key gluconeogenic enzyme, and enhancement of the insulin signals through the insulin receptor (IR)/IR substrate (IRS)/phosphatidylinositol 3-kinase (PI3K)/mammalian target of rapamycin complex 1 (mTOR) pathway in the liver (Toyoshima et al., 2010). In relation to lipid metabolism, there was decreased liver triglyceride content in adult rats exposed to protein restriction during gestation and lactation. It was suggested that this effect could be due to increased fatty-acid transport into the mitochondrial matrix or alterations in triglyceride biosynthesis (Qasem et al., 2015). A maternal protein restriction was shown to reduce the lean and increase the fat contents of 6-month old offspring with a tendency for reduced number of muscle myofibers associated with reduced expression of mRNA of Insulin-like growth factor 2 gene (IGF2 mRNA) in pigs (Chavatte-Palmer et al., 2016).
Maternal Overnutrition and Risk Factor for the Cardiometabolic Dysfuntions
Nutritional transition is a phenomenon well documented in developing countries in the twentieth and twenty-first centuries, and has induced high incidence of the chronic diseases and high prevalence of the obesity (Batista Filho and Rissin, 2003; Batista Filho and Batista, 2010; Ribeiro et al., 2015). It is evident that protein malnutrition was an health problem in the first half of the twentieth century. Now, it was replaced by a diet enriched in saturated fat or other HFDs, predisposing to overweight, and obesity (Batista et al., 2013). Nowadays, it suggested that two billion people in the world are overweight and obese individuals, with major prevalence is related to diet induced-obesity, which have been associated to cardiovascular and endocrine dysfunctions (Hotamisligil, 2006; Aubin et al., 2008; Zhang et al., 2012; Ng et al., 2014; Wensveen et al., 2015).
Recently, the obesity has been considered a physiological state of chronic inflammation, characterized by elevated levels of inflammatory markers including C-reactive protein (CRP), interleukin-6 (IL-6), and tumor necrosis factor alpha (TNF-α) (Wensveen et al., 2015; Erikci Ertunc and Hotamisligil, 2016; Lyons et al., 2016). Maternal HFD chronic consumption enhances the circulating free fatty acids and induce the activation of inflammatory pathways, enhancing chronic inflammation in offspring (Gruber et al., 2015). Studies of Roberts et al. (2015) found that cardiometabolic dysfunction was associated with changes such as elevated serum triglycerides, elevated oxidative stress levels, insulin resistance, vascular disorders, and development of hypertension (Roberts et al., 2015).
In animals on a HFD the hormone leptin has been considered one of the most important physiological mediators of the cardiometabolic dysfunction (Correia and Rahmouni, 2006; Harlan et al., 2013; Harlan and Rahmouni, 2013). Since hyperleptinemia, common in overweight and obesity conditions, produce a misbalance in autonomic system, with sympathetic overactivation (Machleidt et al., 2013; Kurajoh et al., 2015; Manna and Jain, 2015), and reduced sensitivity of vagal afferent neurons (de Lartigue, 2016). This disorder of vagal afferent signaling can activate orexigenic pathways in the CNS and drive hyperphagia, obesity, and cardiometabolic diseases at long-term (de Lartigue, 2016). Some authors have described that, at least in part, cardiovascular dysfuntion elicited by HFD or obesity may be due to changes in the neural control of respiratory and autonomic systems (Bassi et al., 2012, 2015; Hall et al., 2015; Chaar et al., 2016). Part of these effects were suggested to be influenced by atrial natriuretric peptide and renin-angiotensin pathways (Bassi et al., 2012; Gusmão, 2012).
Interestingly, it has been shown that offspring from mothers fed HFD have high risk to develop pathologic cardiac hypertrophy. This condition would be linked to re-expression of cardiac fetal genes, systolic, and diastolic dysfunction and sympathetic overactivity on the heart. These effects lead to reduced cardioprotective signaling that would predispose them to cardiac dysfunctions in adulthood (Taylor et al., 2005; Wang et al., 2010; Fernandez-Twinn et al., 2012; Blackmore et al., 2014). Regarding arterial blood pressure control, it has been described that maternal HFD induces early and persistent alterations in offspring renal and adipose RAS components (Armitage et al., 2005). These changes seem to be dependent upon the period of exposure to the maternal HFD, and contribute to increased adiposity and hypertension in offspring (Samuelsson et al., 2008; Elahi et al., 2009; Guberman et al., 2013; Mazzio and Soliman, 2014; Tan et al., 2015). Studies in baboons subjected to HFD showed that microRNA expression and putative gene targets involved in developmental disorders and cardiovascular diseases were up-regulated and others were down-regulated. The authors suggested that the epigenetic modifications caused by HFD may be involved in the developmental origins of cardiometabolic diseases (Maloyan et al., 2013).
Other metabolic outcomes induced by HFD have been pointed out in the last years and it has demonstrated that HFD displayed a drastic modification on metabolic control of the glucose metabolism and lead to increased insulin level in serum (Fan et al., 2013) and enhanced insulin action through AKT/PKB (protein kinase B) and ERK (extracellular signal-regulated kinase), and activation of mammalian target of rapamycin (mTOR) pathways in cardiac tissue (Fernandez-Twinn et al., 2012; Fan et al., 2013). Offspring from HFD mothers showed alterations in blood glucose and insulin levels, with high predisposition to insulin resistance and cardiac dysfunction (Taylor et al., 2005; Wang et al., 2010). Part of these effects are associated with enhanced production of ROS and reduction in the levels of the anti-oxidant enzymes, such as superoxide dismutase, suggesting a misbalance in the control of the oxidative stress (Fernandez-Twinn et al., 2012).
Altogether, this review addressed the new concept on the maternal diet induced-cardiometabolic diseases that include the potential role of the perinatal malnutrition. It showed that the etiology of these diseases is multifactorial involving genetic and environmental influences and their physiological integration. It is well recognized that both perinatal undernutrition and overnutrition are related with the risk of developing metabolic syndrome and hypertension in adult life (Figure 1). The underlying mechanism can be explained in the context of phenotypic plasticity during development that includes adaptive change on the CNS, heart, kidney, liver, muscle, and adipose tissue metabolisms with consequent physiology dysfunction and with subsequent cardiometabolic diseases. Moreover, maternal undernutrition or overnutrition may predispose epigenetic modifications in dams and their offspring, with predominance of DNA methylation, leading to altered gene expression during development and growth. Further, it can provide a different physiological condition which may contribute to the developmental origins of the cardiometabolic diseases. These physiological dysfunctions seem to be linked to the impaired central and peripheral control of both metabolic and cardiovascular functions by mechanisms that include enhanced sympathetic-respiratory activities and disruption in metabolism of end organs at early life. It is suggested that those effects could be associated to inflammatory conditions and impaired oxidative balance, which may contribute to adult cardiometabolic diseases.
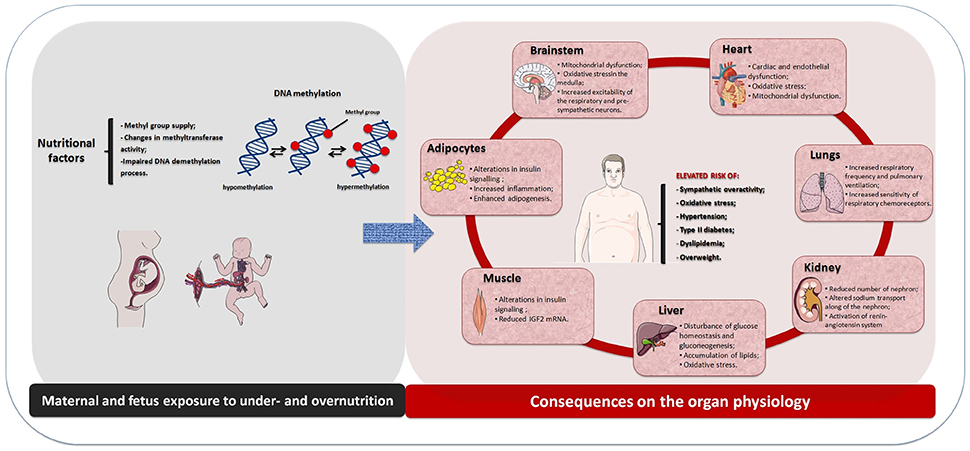
Figure 1. Schematic drawing showing the physiological effects induced by maternal and fetus exposure to under- or overnutrition through DNA methylation and their consequences on the organ physiology and increased risk of the cardiometabolic diseases in the offspring.
Author Contributions
JC, AS, and MF drafted and revised critically the work for important intellectual content and final review of the manuscript.
Conflict of Interest Statement
The authors declare that the research was conducted in the absence of any commercial or financial relationships that could be construed as a potential conflict of interest.
Abbreviations
AKT/PKB, Protein kinase B; CB, Carotid body; CNS, Central nervous system; CRP, C-reactive protein; ERK, Extracellular signal-regulated kinase; GSH, Glutathione reduced; HFD, High fat diet; HIF-1α, Hypoxic inducible factor 1 alpha; IGF2, Insulin-like growth factor 2; IL-6, Interleukin-6; IR, Insulin receptor; IRS, Insulin receptor substrate; mTOR, Mammalian target of rapamycin; PI3K, Phosphatidylinositol 3-kinase; RAS, Renin-angiotensin system; ROS, Reactive oxygen species; TNF-α, Tumor necrosis factor alpha.
References
Alfaradhi, M. Z., and Ozanne, S. E. (2011). Developmental programming in response to maternal overnutrition. Front. Genet. 2:27. doi: 10.3389/fgene.2011.00027
Altobelli, G., Bogdarina, I. G., Stupka, E., Clark, A. J., and Langley-Evans, S. (2013). Genome-wide methylation and gene expression changes in newborn rats following maternal protein restriction and reversal by folic acid. PLoS ONE 8:e82989. doi: 10.1371/journal.pone.0082989
Andersen, L. G., Angquist, L., Gamborg, M., Byberg, L., Bengtsson, C., Canoy, D., et al. (2009). Birth weight in relation to leisure time physical activity in adolescence and adulthood: meta-analysis of results from 13 nordic cohorts. PLoS ONE 4:e8192. doi: 10.1371/journal.pone.0008192
Antony, G. M., and Laxmaiah, A. (2008). Human development, poverty, health & nutrition situation in India. Indian J. Med. Res. 128, 198–205.
Armitage, J. A., Lakasing, L., Taylor, P. D., Balachandran, A. A., Jensen, R. I., Dekou, V., et al. (2005). Developmental programming of aortic and renal structure in offspring of rats fed fat-rich diets in pregnancy. J. Physiol. 565(Pt 1), 171–184. doi: 10.1113/jphysiol.2005.084947
Ashton, N. (2000). Perinatal development and adult blood pressure. Braz. J. Med. Biol. Res. 33, 731–740. doi: 10.1590/S0100-879X2000000700002
Aubin, M. C., Lajoie, C., Clément, R., Gosselin, H., Calderone, A., and Perrault, L. P. (2008). Female rats fed a high-fat diet were associated with vascular dysfunction and cardiac fibrosis in the absence of overt obesity and hyperlipidemia: therapeutic potential of resveratrol. J. Pharmacol. Exp. Ther. 325, 961–968. doi: 10.1124/jpet.107.135061
Barker, D. J. (1990). The fetal and infant origins of adult disease. BMJ 301:1111. doi: 10.1136/bmj.301.6761.1111
Barker, D. J. (1994). Maternal and fetal origins of coronary heart disease. J. R. Coll. Physicians Lond. 28, 544–551.
Barker, D. J. (1995). The wellcome foundation lecture, 1994. The fetal origins of adult disease. Proc. Biol. Sci. 262, 37–43. doi: 10.1098/rspb.1995.0173
Barker, D. J. (1998). In utero programming of chronic disease. Clin. Sci. 95, 115–128. doi: 10.1042/cs0950115
Barker, D. J. (1999b). The long-term outcome of retarded fetal growth. Schweiz. Med. Wochenschr. 129, 189–196.
Barker, D. J. (2000). In utero programming of cardiovascular disease. Theriogenology 53, 555–574. doi: 10.1016/S0093-691X(99)00258-7
Barker, D. J. (2007). The origins of the developmental origins theory. J. Intern. Med. 261, 412–417. doi: 10.1111/j.1365-2796.2007.01809.x
Barker, D. J., Gluckman, P. D., Godfrey, K. M., Harding, J. E., Owens, J. A., and Robinson, J. S. (1993). Fetal nutrition and cardiovascular disease in adult life. Lancet 341, 938–941. doi: 10.1016/0140-6736(93)91224-A
Barker, D. J., and Martyn, C. N. (1992). The maternal and fetal origins of cardiovascular disease. J. Epidemiol. Commun. Health 46, 8–11. doi: 10.1136/jech.46.1.8
Barker, D. J., and Osmond, C. (1986). Infant mortality, childhood nutrition, and ischaemic heart disease in England and Wales. Lancet 1, 1077–1081. doi: 10.1016/S0140-6736(86)91340-1
Barker, D. J., Osmond, C., Forsén, T. J., Kajantie, E., and Eriksson, J. G. (2005). Trajectories of growth among children who have coronary events as adults. N. Engl. J. Med. 353, 1802–1809. doi: 10.1056/NEJMoa044160
Barker, D. J., Osmond, C., Forsen, T. J., Kajantie, E., and Eriksson, J. G. (2007). Maternal and social origins of hypertension. Hypertension. 50, 565–571. doi: 10.1161/HYPERTENSIONAHA.107.091512
Barker, D. J., Winter, P. D., Osmond, C., Margetts, B., and Simmonds, S. J. (1989). Weight in infancy and death from ischaemic heart disease. Lancet 2, 577–580. doi: 10.1016/S0140-6736(89)90710-1
Barros, M. A., De Brito Alves, J. L., Nogueira, V. O., Wanderley, A. G., and Costa-Silva, J. H. (2015). Maternal low-protein diet induces changes in the cardiovascular autonomic modulation in male rat offspring. Nutr. Metab. Cardiovasc. Dis. 25, 123–130. doi: 10.1016/j.numecd.2014.07.011
Bassi, M., Giusti, H., Leite, C. M., Anselmo-Franci, J. A., do Carmo, J. M., da Silva, A. A., et al. (2012). Central leptin replacement enhances chemorespiratory responses in leptin-deficient mice independent of changes in body weight. Pflugers Arch. 464, 145–153. doi: 10.1007/s00424-012-1111-1
Bassi, M., Nakamura, N. B., Furuya, W. I., Colombari, D. S., Menani, J. V., do Carmo, J. M., et al. (2015). Activation of the brain melanocortin system is required for leptin-induced modulation of chemorespiratory function. Acta Physiol. (Oxf). 213, 893–901. doi: 10.1111/apha.12394
Batista, T. M., Ribeiro, R. A., da Silva, P. M., Camargo, R. L., Lollo, P. C., Boschero, A. C., et al. (2013). Taurine supplementation improves liver glucose control in normal protein and malnourished mice fed a high-fat diet. Mol. Nutr. Food Res. 57, 423–434. doi: 10.1002/mnfr.201200345
Batista Filho, M., and Batista, L. V. (2010). Transição alimentar/nutricional ou mutação antropológica? Ciênc. Cult. 62, 26–30.
Batista Filho, M., and Rissin, A. (2003). A transição nutricional no Brasil: tendências regionais e temporais. Cad. Saúde Pública 19, S181–S191. doi: 10.1590/S0102-311X2003000700019
Biosca, M., Rodríguez, G., Ventura, P., Samper, M. P., Labayen, I., Collado, M. P., et al. (2011). Central adiposity in children born small and large for gestational age. Nutr. Hosp. 26, 971–976. doi: 10.1590/S0212-16112011000500008
Blackmore, H. L., Niu, Y., Fernandez-Twinn, D. S., Tarry-Adkins, J. L., Giussani, D. A., and Ozanne, S. E. (2014). Maternal diet-induced obesity programs cardiovascular dysfunction in adult male mouse offspring independent of current body weight. Endocrinology 155, 3970–3980. doi: 10.1210/en.2014-1383
Brawley, L., Itoh, S., Torrens, C., Barker, A., Bertram, C., Poston, L., et al. (2003). Dietary protein restriction in pregnancy induces hypertension and vascular defects in rat male offspring. Pediatr. Res. 54, 83–90. doi: 10.1203/01.PDR.0000065731.00639.02
Chaar, L. J., Coelho, A., Silva, N. M., Festuccia, W. L., and Antunes, V. R. (2016). High-fat diet-induced hypertension and autonomic imbalance are associated with an upregulation of CART in the dorsomedial hypothalamus of mice. Physiol. Rep. 4:e12811. doi: 10.14814/phy2.12811
Chan, S. H., Tai, M. H., Li, C. Y., and Chan, J. Y. (2006). Reduction in molecular synthesis or enzyme activity of superoxide dismutases and catalase contributes to oxidative stress and neurogenic hypertension in spontaneously hypertensive rats. Free Radic. Biol. Med. 40, 2028–2039. doi: 10.1016/j.freeradbiomed.2006.01.032
Chango, A., and Pogribny, I. P. (2015). Considering maternal dietary modulators for epigenetic regulation and programming of the fetal epigenome. Nutrients 7, 2748–2770. doi: 10.3390/nu7042748
Chavatte-Palmer, P., Tarrade, A., and Rousseau-Ralliard, D. (2016). Diet before and during pregnancy and offspring health: the importance of animal models and what can be learned from them. Int. J. Environ. Res. Public Health 13:E586. doi: 10.3390/ijerph13060586
Chen, J. H., Tarry-Adkins, J. L., Matharu, K., Yeo, G. S., and Ozanne, S. E. (2010). Maternal protein restriction affects gene expression profiles in the kidney at weaning with implications for the regulation of renal function and lifespan. Clin. Sci. 119, 373–384. doi: 10.1042/CS20100230
Chong, S., and Whitelaw, E. (2004). Epigenetic germline inheritance. Curr. Opin. Genet. Dev. 14, 692–696. doi: 10.1016/j.gde.2004.09.001
Conde, W. L., and Monteiro, C. A. (2014). Nutrition transition and double burden of undernutrition and excess of weight in Brazil. Am. J. Clin. Nutr. 100, 1617S–1622S. doi: 10.3945/ajcn.114.084764
Correia, M. L., and Rahmouni, K. (2006). Role of leptin in the cardiovascular and endocrine complications of metabolic syndrome. Diabetes Obes. Metab. 8, 603–610. doi: 10.1111/j.1463-1326.2005.00562.x
Costa-Silva, J. H., Silva, P. A., Pedi, N., Luzardo, R., Einicker-Lamas, M., Lara, L. S., et al. (2009). Chronic undernutrition alters renal active Na+ transport in young rats: potential hidden basis for pathophysiological alterations in adulthood? Eur. J. Nutr. 48, 437–445. doi: 10.1007/s00394-009-0032-z
Costa-Silva, J. H., de Brito-Alves, J. L., Barros, M. A., Nogueira, V. O., Paulino-Silva, K. M., de Oliveira-Lira, A., et al. (2015). New insights on the maternal diet induced-hypertension: potential role of the phenotypic plasticity and sympathetic-respiratory overactivity. Front. Physiol. 6:345. doi: 10.3389/fphys.2015.00345
de Brito Alves, J. L., de Oliveira, J. M., Ferreira, D. J., de Barros, M. A., Nogueira, V. O., Alves, D. S., et al. (2016). Maternal protein restriction induced-hypertension is associated to oxidative disruption at transcriptional and functional levels in the medulla oblongata. Clin. Exp. Pharmacol. Physiol. doi: 10.1111/1440-1681.12667. [Epub ahead of print].
de Brito Alves, J. L., Nogueira, V. O., Cavalcanti Neto, M. P., Leopoldino, A. M., Curti, C., Colombari, D. S., et al. (2015). Maternal protein restriction increases respiratory and sympathetic activities and sensitizes peripheral chemoreflex in male rat offspring. J. Nutr. 145, 907–914. doi: 10.3945/jn.114.202804
de Brito Alves, J. L., Nogueira, V. O., de Oliveira, G. B., da Silva, G. S., Wanderley, A. G., Leandro, C. G., et al. (2014). Short- and long-term effects of a maternal low-protein diet on ventilation, O2/CO2 chemoreception and arterial blood pressure in male rat offspring. Br. J. Nutr. 111, 606–615. doi: 10.1017/S0007114513002833
de Lartigue, G. (2016). Role of the vagus nerve in the development and treatment of diet-induced obesity. J. Physiol. 594, 5791–5815. doi: 10.1113/JP271538
Egger, G., Liang, G., Aparicio, A., and Jones, P. A. (2004). Epigenetics in human disease and prospects for epigenetic therapy. Nature 429, 457–463. doi: 10.1038/nature02625
Elahi, M. M., Cagampang, F. R., Mukhtar, D., Anthony, F. W., Ohri, S. K., and Hanson, M. A. (2009). Long-term maternal high-fat feeding from weaning through pregnancy and lactation predisposes offspring to hypertension, raised plasma lipids and fatty liver in mice. Br. J. Nutr. 102, 514–519. doi: 10.1017/S000711450820749X
Erikci Ertunc, M., and Hotamisligil, G. S. (2016). Lipid signaling and lipotoxicity in metabolic inflammation: indications for metabolic disease pathogenesis and treatment. J. Lipid Res. doi: 10.1194/jlr.r066514. [Epub ahead of print].
Essick, E. E., and Sam, F. (2010). Oxidative stress and autophagy in cardiac disease, neurological disorders, aging and cancer. Oxid. Med. Cell Longev. 3, 168–177. doi: 10.4161/oxim.3.3.12106
Falcão-Tebas, F., Bento-Santos, A., Fidalgo, M. A., de Almeida, M. B., dos Santos, J. A., Lopes de Souza, S., et al. (2012). Maternal low-protein diet-induced delayed reflex ontogeny is attenuated by moderate physical training during gestation in rats. Br. J. Nutr. 107, 372–377. doi: 10.1017/S0007114511002947
Fall, C. H., and Barker, D. J. (1997). The fetal origins of coronary heart disease and non-insulin dependent diabetes in India. Indian Pediatr. 34, 5–8.
Fan, L., Lindsley, S. R., Comstock, S. M., Takahashi, D. L., Evans, A. E., He, G. W., et al. (2013). Maternal high-fat diet impacts endothelial function in nonhuman primate offspring. Int. J. Obes. (Lond). 37, 254–262. doi: 10.1038/ijo.2012.42
Fernandez-Twinn, D. S., Blackmore, H. L., Siggens, L., Giussani, D. A., Cross, C. M., Foo, R., et al. (2012). The programming of cardiac hypertrophy in the offspring by maternal obesity is associated with hyperinsulinemia, AKT, ERK, and mTOR activation. Endocrinology 153, 5961–5971. doi: 10.1210/en.2012-1508
Ferreira, D. J., da Silva Pedroza, A. A., Braz, G. R., da Silva-Filho, R. C., Lima, T. A., Fernandes, M. P., et al. (2016). Mitochondrial bioenergetics and oxidative status disruption in brainstem of weaned rats: immediate response to maternal protein restriction. Brain Res. 1642, 553–561. doi: 10.1016/j.brainres.2016.04.049
Ferreira, D. S., Liu, Y., Fernandes, M. P., and Lagranha, C. J. (2015). Perinatal low-protein diet alters brainstem antioxidant metabolism in adult offspring. Nutr. Neurosci. 19, 369–375. doi: 10.1179/1476830515Y.0000000030
Fidalgo, M., Falcão-Tebas, F., Bento-Santos, A., de Oliveira, E., Nogueira-Neto, J. F., de Moura, E. G., et al. (2013). Programmed changes in the adult rat offspring caused by maternal protein restriction during gestation and lactation are attenuated by maternal moderate-low physical training. Br. J. Nutr. 109, 449–456. doi: 10.1017/S0007114512001316
Fiorito, G., Guarrera, S., Valle, C., Ricceri, F., Russo, A., Grioni, S., et al. (2014). B-vitamins intake, DNA-methylation of One Carbon Metabolism and homocysteine pathway genes and myocardial infarction risk: the EPICOR study. Nutr. Metab. Cardiovasc. Dis. 24, 483–488. doi: 10.1016/j.numecd.2013.10.026
Franco, M. C., Casarini, D. E., Carneiro-Ramos, M. S., Sawaya, A. L., Barreto-Chaves, M. L., and Sesso, R. (2008). Circulating renin-angiotensin system and catecholamines in childhood: is there a role for birthweight? Clin. Sci. 114, 375–380. doi: 10.1042/CS20070284
Franco Mdo, C., Arruda, R. M., Dantas, A. P., Kawamoto, E. M., Fortes, Z. B., Scavone, C., et al. (2002). Intrauterine undernutrition: expression and activity of the endothelial nitric oxide synthase in male and female adult offspring. Cardiovasc. Res. 56, 145–153. doi: 10.1016/S0008-6363(02)00508-4
Gruber, L., Hemmerling, J., Schüppel, V., Müller, M., Boekschoten, M. V., and Haller, D. (2015). Maternal high-fat diet accelerates development of crohn's disease-like ileitis in TNFDeltaARE/WT offspring. Inflamm. Bowel Dis. 21, 2016–2025. doi: 10.1097/MIB.0000000000000465
Guberman, C., Jellyman, J. K., Han, G., Ross, M. G., and Desai, M. (2013). Maternal high-fat diet programs rat offspring hypertension and activates the adipose renin-angiotensin system. Am. J. Obstet. Gynecol. 209, 262.e1–262.e18. doi: 10.1016/j.ajog.2013.05.023
Gusmão, D. O. (2012). Relação entre Leptina, Peptídeo Natriurético Atrial e Estrógeno em um Modelo Animal de Hipertensão Associada a Obesidade. Universidade Federal da Bahia.
Hales, C. N., and Barker, D. J. (1992). Type 2 (non-insulin-dependent) diabetes mellitus: the thrifty phenotype hypothesis. Diabetologia 35, 595–601. doi: 10.1007/BF00400248
Hales, C. N., Barker, D. J., Clark, P. M., Cox, L. J., Fall, C., Osmond, C., et al. (1991). Fetal and infant growth and impaired glucose tolerance at age 64. BMJ 303, 1019–1022. doi: 10.1136/bmj.303.6809.1019
Hall, J. E., do Carmo, J. M., da Silva, A. A., Wang, Z., and Hall, M. E. (2015). Obesity-induced hypertension: interaction of neurohumoral and renal mechanisms. Circ. Res. 116, 991–1006. doi: 10.1161/CIRCRESAHA.116.305697
Hardy, T. M., and Tollefsbol, T. O. (2011). Epigenetic diet: impact on the epigenome and cancer. Epigenomics 3, 503–518. doi: 10.2217/epi.11.71
Harlan, S. M., Guo, D. F., Morgan, D. A., Fernandes-Santos, C., and Rahmouni, K. (2013). Hypothalamic mTORC1 signaling controls sympathetic nerve activity and arterial pressure and mediates leptin effects. Cell Metab. 17, 599–606. doi: 10.1016/j.cmet.2013.02.017
Harlan, S. M., and Rahmouni, K. (2013). Neuroanatomical determinants of the sympathetic nerve responses evoked by leptin. Clin. Auton. Res. 23, 1–7. doi: 10.1007/s10286-012-0168-4
Hemachandra, A. H., Klebanoff, M. A., Duggan, A. K., Hardy, J. B., and Furth, S. L. (2006). The association between intrauterine growth restriction in the full-term infant and high blood pressure at age 7 years: results from the Collaborative Perinatal Project. Int. J. Epidemiol. 35, 871–877. doi: 10.1093/ije/dyl080
Hotamisligil, G. S. (2006). Inflammation and metabolic disorders. Nature 444, 860–867. doi: 10.1038/nature05485
Ito, T., Funamoto, K., Sato, N., Nakamura, A., Tanabe, K., Hoshiai, T., et al. (2012). Maternal undernutrition induces the expression of hypoxia-related genes in the fetal brain. Tohoku J. Exp. Med. 226, 37–44. doi: 10.1620/tjem.226.37
Ito, T., Tanabe, K., Nakamura, A., Funamoto, K., Aoyagi, A., Sato, K., et al. (2011). Aberrant expression of hypoxia-inducible factor 1alpha in the fetal heart is associated with maternal undernutrition. Tohoku J. Exp. Med. 224, 163–171. doi: 10.1620/tjem.224.163
Jablonka, E., and Lamb, M. J. (2002). The changing concept of epigenetics. Ann. N.Y. Acad. Sci. 981, 82–96. doi: 10.1111/j.1749-6632.2002.tb04913.x
Johansson, S., Norman, M., Legnevall, L., Dalmaz, Y., Lagercrantz, H., and Vanpée, M. (2007). Increased catecholamines and heart rate in children with low birth weight: perinatal contributions to sympathoadrenal overactivity. J. Intern. Med. 261, 480–487. doi: 10.1111/j.1365-2796.2007.01776.x
Karlic, H., and Varga, F. (2011). Impact of vitamin D metabolism on clinical epigenetics. Clin. Epigenet. 2, 55–61. doi: 10.1007/s13148-011-0021-y
Kurajoh, M., Koyama, H., Kadoya, M., Naka, M., Miyoshi, A., Kanzaki, A., et al. (2015). Plasma leptin level is associated with cardiac autonomic dysfunction in patients with type 2 diabetes: HSCAA study. Cardiovasc. Diabetol. 14, 117. doi: 10.1186/s12933-015-0280-6
Labayen, I., Moreno, L. A., Blay, M. G., Blay, V. A., Mesana, M. I., González-Gross, M., et al. (2006). Early programming of body composition and fat distribution in adolescents. J. Nutr. 136, 147–152.
Landsberg, L., Aronne, L. J., Beilin, L. J., Burke, V., Igel, L. I., Lloyd-Jones, D., et al. (2013). Obesity-related hypertension: pathogenesis, cardiovascular risk, and treatment–a position paper of the obesity society and The American Society of Hypertension. Obesity (Silver Spring) 21, 8–24. doi: 10.1002/oby.20181
Leandro, C. G., da Silva Ribeiro, W., Dos Santos, J. A., Bento-Santos, A., Lima-Coelho, C. H., Falcão-Tebas, F., et al. (2012). Moderate physical training attenuates muscle-specific effects on fibre type composition in adult rats submitted to a perinatal maternal low-protein diet. Eur. J. Nutr. 51, 807–815. doi: 10.1007/s00394-011-0259-3
Lomba, A., Martínez, J. A., García-Díaz, D. F., Paternain, L., Marti, A., Campión, J., et al. (2010). Weight gain induced by an isocaloric pair-fed high fat diet: a nutriepigenetic study on FASN and NDUFB6 gene promoters. Mol. Genet. Metab. 101, 273–278. doi: 10.1016/j.ymgme.2010.07.017
Lucas, A. (1998). Programming by early nutrition: an experimental approach. J. Nutr. 128(2 Suppl.), 401S–406S.
Lyons, C. L., Kennedy, E. B., and Roche, H. M. (2016). Metabolic inflammation-differential modulation by dietary constituents. Nutrients 8:E247. doi: 10.3390/nu8050247
Machleidt, F., Simon, P., Krapalis, A. F., Hallschmid, M., Lehnert, H., and Sayk, F. (2013). Experimental hyperleptinemia acutely increases vasoconstrictory sympathetic nerve activity in healthy humans. J. Clin. Endocrinol. Metab. 98, E491–E496. doi: 10.1210/jc.2012-3009
Maloyan, A., Muralimanoharan, S., Huffman, S., Cox, L. A., Nathanielsz, P. W., Myatt, L., et al. (2013). Identification and comparative analyses of myocardial miRNAs involved in the fetal response to maternal obesity. Physiol. Genomics 45, 889–900. doi: 10.1152/physiolgenomics.00050.2013
Manna, P., and Jain, S. K. (2015). Obesity, oxidative stress, adipose tissue dysfunction, and the associated health risks: causes and therapeutic strategies. Metab. Syndr. Relat. Disord. 13, 423–444. doi: 10.1089/met.2015.0095
Mansego, M. L., Milagro, F. I., Campión, J., and Martínez, J. A. (2013). Techniques of DNA methylation analysis with nutritional applications. J. Nutrigenet. Nutrigenomics 6, 83–96. doi: 10.1159/000350749
Mazzio, E. A., and Soliman, K. F. (2014). Epigenetics and nutritional environmental signals. Integr. Comp. Biol. 54, 21–30. doi: 10.1093/icb/icu049
Milagro, F. I., Campión, J., García-Díaz, D. F., Goyenechea, E., Paternain, L., and Martinez, J. A. (2009). High fat diet-induced obesity modifies the methylation pattern of leptin promoter in rats. J. Physiol. Biochem. 65, 1–9. doi: 10.1007/BF03165964
Milagro, F. I., Mansego, M. L., De Miguel, C., and Martínez, J. A. (2013). Dietary factors, epigenetic modifications and obesity outcomes: progresses and perspectives. Mol. Aspects Med. 34, 782–812. doi: 10.1016/j.mam.2012.06.010
Mitchell, C., Schneper, L. M., and Notterman, D. A. (2016). DNA methylation, early life environment, and health outcomes. Pediatr. Res. 79, 212–219. doi: 10.1038/pr.2015.193
Nanduri, J., and Prabhakar, N. R. (2015). Epigenetic regulation of carotid body oxygen sensing: clinical implications. Adv. Exp. Med. Biol. 860, 1–8. doi: 10.1007/978-3-319-18440-1_1
Nascimento, L., Freitas, C. M., Silva-Filho, R., Leite, A. C., Silva, A. B., da Silva, A. I., et al. (2014). The effect of maternal low-protein diet on the heart of adult offspring: role of mitochondria and oxidative stress. Appl. Physiol. Nutr. Metab. 39, 880–887. doi: 10.1139/apnm-2013-0452
Ng, M., Fleming, T., Robinson, M., Thomson, B., Graetz, N., Margono, C., et al. (2014). Global, regional, and national prevalence of overweight and obesity in children and adults during 1980-2013: a systematic analysis for the Global Burden of Disease Study 2013. Lancet 384, 766–781. doi: 10.1016/S0140-6736(14)60460-8
Ng, S. F., Lin, R. C., Laybutt, D. R., Barres, R., Owens, J. A., and Morris, M. J. (2010). Chronic high-fat diet in fathers programs beta-cell dysfunction in female rat offspring. Nature 467, 963–966. doi: 10.1038/nature09491
Nuyt, A. M. (2008). Mechanisms underlying developmental programming of elevated blood pressure and vascular dysfunction: evidence from human studies and experimental animal models. Clin. Sci. 114, 1–17. doi: 10.1042/CS20070113
Nuyt, A. M., and Alexander, B. T. (2009). Developmental programming and hypertension. Curr. Opin. Nephrol. Hypertens. 18, 144–152. doi: 10.1097/MNH.0b013e328326092c
Osmond, C., and Barker, D. J. (2000). Fetal, infant, and childhood growth are predictors of coronary heart disease, diabetes, and hypertension in adult men and women. Environ. Health Perspect. 108(Suppl. 3), 545–553. doi: 10.1289/ehp.00108s3545
Ozanne, S. E., and Hales, C. N. (2004). Lifespan: catch-up growth and obesity in male mice. Nature 427, 411–412. doi: 10.1038/427411b
Parra, D. C., Iannotti, L., Gomez, L. F., Pachón, H., Haire-Joshu, D., Sarmiento, O. L., et al. (2015). The nutrition transition in Colombia over a decade: a novel household classification system of anthropometric measures. Arch. Public Health 73, 12. doi: 10.1186/s13690-014-0057-5
Paulino-Silva, K. M., and Costa-Silva, J. H. (2016). Hypertension in rat offspring subjected to perinatal protein malnutrition is not related to the baroreflex dysfunction. Clin. Exp. Pharmacol. Physiol. 43, 1046–1053. doi: 10.1111/1440-1681.12628
Prabhakar, N. R. (2013). Sensing hypoxia: physiology, genetics and epigenetics. J. Physiol. 591(Pt 9), 2245–2257. doi: 10.1113/jphysiol.2012.247759
Qasem, R. J., Li, J., Tang, H. M., Browne, V., Mendez-Garcia, C., Yablonski, E., et al. (2015). Decreased liver triglyceride content in adult rats exposed to protein restriction during gestation and lactation: role of hepatic triglyceride utilization. Clin. Exp. Pharmacol. Physiol. 42, 380–388. doi: 10.1111/1440-1681.12359
Ravelli, G. P., Stein, Z. A., and Susser, M. W. (1976). Obesity in young men after famine exposure in utero and early infancy. N. Engl. J. Med. 295, 349–353. doi: 10.1056/NEJM197608122950701
Ribeiro, A. M., Lima Mde, C., de Lira, P. I., and da Silva, G. A. (2015). [Low birth weight and obesity: causal or casual association?]. Rev. Paul. Pediatr. 33, 341–349. doi: 10.1016/j.rpped.2014.09.007
Roberts, V. H., Frias, A. E., and Grove, K. L. (2015). Impact of maternal obesity on fetal programming of cardiovascular disease. Physiology (Bethesda) 30, 224–231. doi: 10.1152/physiol.00021.2014
Samuelsson, A. M., Matthews, P. A., Argenton, M., Christie, M. R., McConnell, J. M., Jansen, E. H., et al. (2008). Diet-induced obesity in female mice leads to offspring hyperphagia, adiposity, hypertension, and insulin resistance: a novel murine model of developmental programming. Hypertension 51, 383–392. doi: 10.1161/HYPERTENSIONAHA.107.101477
Sawaya, A. L., Martins, P. A., Grillo, L. P., and Florêncio, T. T. (2004). Long-term effects of early malnutrition on body weight regulation. Nutr. Rev. 62(7 Pt 2), S127–S133. doi: 10.1111/j.1753-4887.2004.tb00082.x
Sawaya, A. L., and Roberts, S. (2003). Stunting and future risk of obesity: principal physiological mechanisms. Cad. Saude Publica 19(Suppl. 1), S21–S28. doi: 10.1590/S0102-311X2003000700003
Siddique, K., Guzman, G. L., Gattineni, J., and Baum, M. (2014). Effect of postnatal maternal protein intake on prenatal programming of hypertension. Reprod. Sci. 21, 1499–1507. doi: 10.1177/1933719114530186
Stone, N., Pangilinan, F., Molloy, A. M., Shane, B., Scott, J. M., Ueland, P. M., et al. (2011). Bioinformatic and genetic association analysis of microRNA target sites in one-carbon metabolism genes. PLoS ONE 6:e21851. doi: 10.1371/journal.pone.0021851
Szarc vel Szic, K., Declerck, K., Vidakovic, M., and Vanden Berghe, W. (2015). From inflammaging to healthy aging by dietary lifestyle choices: is epigenetics the key to personalized nutrition? Clin. Epigenetics 7, 33. doi: 10.1186/s13148-015-0068-2
Tan, H. C., Roberts, J., Catov, J., Krishnamurthy, R., Shypailo, R., and Bacha, F. (2015). Mother's pre-pregnancy BMI is an important determinant of adverse cardiometabolic risk in childhood. Pediatr. Diabetes 16, 419–426. doi: 10.1111/pedi.12273
Tarry-Adkins, J. L., Fernandez-Twinn, D. S., Hargreaves, I. P., Neergheen, V., Aiken, C. E., Martin-Gronert, M. S., et al. (2016). Coenzyme Q10 prevents hepatic fibrosis, inflammation, and oxidative stress in a male rat model of poor maternal nutrition and accelerated postnatal growth. Am. J. Clin. Nutr. 103, 579–588. doi: 10.3945/ajcn.115.119834
Taylor, P. D., McConnell, J., Khan, I. Y., Holemans, K., Lawrence, K. M., Asare-Anane, H., et al. (2005). Impaired glucose homeostasis and mitochondrial abnormalities in offspring of rats fed a fat-rich diet in pregnancy. Am. J. Physiol. Regul. Integr. Comp. Physiol. 288, 23. doi: 10.1152/ajpregu.00355.2004
Toyoshima, Y., Tokita, R., Ohne, Y., Hakuno, F., Noguchi, T., Minami, S., et al. (2010). Dietary protein deprivation upregulates insulin signaling and inhibits gluconeogenesis in rat liver. J. Mol. Endocrinol. 45, 329–340. doi: 10.1677/JME-10-0102
Victora, C. G., Adair, L., Fall, C., Hallal, P. C., Martorell, R., Richter, L., et al. (2008). Maternal and child undernutrition: consequences for adult health and human capital. Lancet 371, 340–357. doi: 10.1016/S0140-6736(07)61692-4
Wang, J., Cao, M., Zhuo, Y., Che, L., Fang, Z., Xu, S., et al. (2016). Catch-up growth following food restriction exacerbates adulthood glucose intolerance in pigs exposed to intrauterine undernutrition. Nutrition 32, 1275–1284. doi: 10.1016/j.nut.2016.03.010
Wang, J., Ma, H., Tong, C., Zhang, H., Lawlis, G. B., Li, Y., et al. (2010). Overnutrition and maternal obesity in sheep pregnancy alter the JNK-IRS-1 signaling cascades and cardiac function in the fetal heart. FASEB J. 24, 2066–2076. doi: 10.1096/fj.09-142315
Waterland, R. A., and Michels, K. B. (2007). Epigenetic epidemiology of the developmental origins hypothesis. Annu. Rev. Nutr. 27, 363–388. doi: 10.1146/annurev.nutr.27.061406.093705
Wei, L. N. (2013). Non-canonical activity of retinoic acid in epigenetic control of embryonic stem cell. Transcription 4, 158–161. doi: 10.4161/trns.25395
Wells, J. C. (2007). The programming effects of early growth. Early Hum. Dev. 83, 743–748. doi: 10.1016/j.earlhumdev.2007.09.002
Wells, J. C. (2011). The thrifty phenotype: an adaptation in growth or metabolism? Am. J. Hum. Biol. 23, 65–75. doi: 10.1002/ajhb.21100
Wells, J. C. (2012). Obesity as malnutrition: the role of capitalism in the obesity global epidemic. Am. J. Hum. Biol. 24, 261–276. doi: 10.1002/ajhb.22253
Wensveen, F. M., Jelencic, V., Valentic, S., Ŝestan, M., Wensveen, T. T., Theurich, S., et al. (2015). NK cells link obesity-induced adipose stress to inflammation and insulin resistance. Nat. Immunol. 16, 376–385. doi: 10.1038/ni.3120
West-Eberhard, M. J. (2005a). Phenotypic accommodation: adaptive innovation due to developmental plasticity. J. Exp. Zool. B Mol. Dev. Evol. 304, 610–618. doi: 10.1002/jez.b.21071
West-Eberhard, M. J. (2005b). Developmental plasticity and the origin of species differences. Proc. Natl. Acad. Sci. U.S.A. 102(Suppl 1), 6543–6549. doi: 10.1073/pnas.0501844102
Zhang, Y., Yuan, M., Bradley, K. M., Dong, F., Anversa, P., and Ren, J. (2012). Insulin-like growth factor 1 alleviates high-fat diet-induced myocardial contractile dysfunction: role of insulin signaling and mitochondrial function. Hypertension 59, 680–693. doi: 10.1161/HYPERTENSIONAHA.111.181867
Zohdi, V., Lim, K., Pearson, J. T., and Black, M. J. (2015). Developmental programming of cardiovascular disease following intrauterine growth restriction: findings utilising a rat model of maternal protein restriction. Nutrients 7, 119–152. doi: 10.3390/nu7010119
Keywords: developmental plasticity, perinatal nutrition, cardiometabolic control, protein restriction
Citation: Costa-Silva JH, Simões-Alves AC and Fernandes MP (2016) Developmental Origins of Cardiometabolic Diseases: Role of the Maternal Diet. Front. Physiol. 7:504. doi: 10.3389/fphys.2016.00504
Received: 21 August 2016; Accepted: 14 October 2016;
Published: 16 November 2016.
Edited by:
Camille M. Balarini, Federal University of Paraíba, BrazilReviewed by:
James Todd Pearson, National Cerebral and Cardiovascular Center, JapanAna Paula Davel, State University of Campinas, Brazil
Copyright © 2016 Costa-Silva, Simões-Alves and Fernandes. This is an open-access article distributed under the terms of the Creative Commons Attribution License (CC BY). The use, distribution or reproduction in other forums is permitted, provided the original author(s) or licensor are credited and that the original publication in this journal is cited, in accordance with accepted academic practice. No use, distribution or reproduction is permitted which does not comply with these terms.
*Correspondence: João H. Costa-Silva, am9hby5oY3NpbHZhQHVmcGUuYnI=