Commentary: Validation of a Ramp Running Protocol for Determination of the True VO2max in Mice
- Unité de Biologie Intégrative des Adaptations à l'Exercice, Université d'Evry Val d'Essonne, Evry, France
In the field of comparative physiology, it remains to be established whether the concept of VO2max is valid in the mouse and, if so, how this value can be accurately determined. In humans, VO2max is generally considered to correspond to the plateau observed when VO2 no longer rises with an increase in workload. In contrast, the concept of VO2peak tends to be used in murine studies. The objectives of the present study were to determine whether (i) a continuous ramp protocol yielded a higher VO2peak than a stepwise, incremental protocol, and (ii) the VO2peak measured in the ramp protocol corresponded to VO2max. The three protocols (based on intensity-controlled treadmill running until exhaustion with eight female FVB/N mice) were performed in random order: (a) an incremental protocol that begins at 10 m.min−1 speed and increases by 3 m.min−1 every 3 min. (b) a ramp protocol with slow acceleration (3 m.min−2), and (c) a ramp protocol with fast acceleration (12 m.min−2). Each protocol was performed with two slopes (0 and 25°). Hence, each mouse performed six exercise tests. We found that the value of VO2peak was protocol-dependent (p < 0.05) and was highest (59.0 ml.kg 0.75.min−1) for the 3 m.min−2 0° ramp protocol. In the latter, the presence of a VO2max plateau was associated with the fulfillment of two secondary criteria (a blood lactate concentration >8 mmol.l−1 and a respiratory exchange ratio >1). The total duration of the 3 m.min−2 0° ramp protocol was shorter than that of the incremental protocol. Taken as a whole, our results suggest that VO2max in the mouse is best determined by applying a ramp exercise protocol with slow acceleration and no treadmill slope.
Introduction
Although rodents are often used as models in exercise physiology, there is no consensus on the use of a standardized exercise protocol for determining the maximum oxygen uptake (VO2max) in these species. In fact, the concept of peak oxygen consumption (VO2peak) is preferred in mice. Given that VO2max is the main determinant of performance in human exercise physiology (i.e., the greatest possible oxygen uptake during physical exercise involving a large proportion of the total muscle mass (Cohn, 1987), it remains to be established whether this concept is valid in the mouse and, if so, how VO2max can be accurately determined.
It is widely acknowledged that VO2max in humans corresponds to both the cardiovascular system's functional limitation and the organism's aerobic capacity. Since the VO2max concept was introduced by Hill and Lupton (1923), the use of exercise protocols with progressive or stepped increments has been validated in human - although the optimal choice of exercise protocol is still subject to debate. In stepwise protocols, the height of the step (i.e., the magnitude of the increment) and the duration of each workload level are left to the investigator's discretion.Since the 1960s, a number of different incremental protocols (with variations in running speed, treadmill slope or both) have been tested for their reliability in determining VO2max (Balke and Ware, 1959; Bruce et al., 1963; Froelicher et al., 1974). In contrast, ramp protocol are characterized by a continuous, gradual increase in the workload (i.e., power, speed or slope) up to maximum values. Many researchers have compared incremental protocols with ramp protocols, in order to establish the most efficient method for determining VO2max (Whipp et al., 1981; Astorino et al., 2005; Yoon et al., 2007). These studies have shown that the ramp exercise protocol is well suited to the human's aerobic metabolism and thus enables VO2max to be accurately determined. However, ramp protocols take longer to complete, and incremental protocols are preferred for the routine measurement of VO2max because they allow other performance indicators (such as the ventilatory threshold and the lactate threshold) to be determined. In humans, VO2max is generally considered to correspond to the plateau observed when VO2 no longer increases with speed. However, about half of tested subjects do not reach a plateau before they abandon the protocol; secondary criteria then have to be used to establish when the last (peak) VO2 value indeed corresponds to VO2max. Three secondary criteria have been proposed: (i) the maximum heart rate at the end of the test, which corresponds to an estimate of the theoretical maximum (Åstrand, 1952; Astrand, 1960; Maritz et al., 1961); (ii) an end-of-exercise respiratory exchange ratio (RER) >1.15 (Issekutz et al., 1962); and (iii) an end-of-exercise blood lactate concentration >8 mmol.l−1.
For the purposes of comparative physiology, VO2max has also been determined in rodents. This parameter can be used in studies of exercise training or in descriptive studies of genetically modified animals (Kemi et al., 2002; Hoydal et al., 2007; Mouisel et al., 2014). As in humans, the relationship between running intensity and oxygen uptake is linear in mice (as demonstrated during steady-state, fixed-intensity running (Fernando et al., 1993; Schefer and Talan, 1996; Wisløff et al., 2001); this enables the use of incremental protocols. However, various strains of mouse have been used, and an effect of strain on treadmill performance has been evidenced. FVB mice achieve high maximum and critical speeds during forced treadmill exercise (Lightfoot et al., 2001; Lerman et al., 2002; Billat et al., 2005). Furthermore, age (Schefer and Talan, 1996) gender (Hoydal et al., 2007) may affect VO2max. VO2peak decreases in old age, although female and male mice appear to have similar levels of performance (Kemi et al., 2002; Billat et al., 2005). Consequently, the disparities in the literature data on VO2peak can be explained (at least in part) by differences in age and strain.
Although, the mouse has been widely used to study the biochemical and molecular adaptations to exercise, a number of different protocols have been applied; this may explain (at least in part) the broad range of values obtained for VO2peak. Furthermore, it has been reported that VO2peak in mice is slope-dependent (Kemi et al., 2002). The incremental protocols described in the literature differ in their duration, increment size and the criteria used to determine exhaustion (usually the animal's behavior or the shape of the VO2/time curve) (Dohm et al., 1994; Rezende et al., 2005; Hawkins et al., 2007). It is not known whether a ramp protocol is suitable for determining VO2peak in mice or whether this value is protocol-dependent. Kemi et al. (2002) were the first to estimate the animal's level of exhaustion by applying secondary criteria (i.e., the RER and blood lactate levels) (Kemi et al., 2002). However, the presence or absence of a VO2 plateau, the latter's characteristics and the relationship between VO2peak and VO2max have not previously been studied in the mouse. We hypothesized that VO2peak and VO2max in mice are protocol-dependent and that (as in humans) a ramp exercise protocol would be suitable for determining VO2max. Thus, the objective of the present study in mice was to determine whether (i) a continuous ramp protocol yielded a higher VO2peak than a stepwise, incremental protocol, and (ii) the VO2peak measured in the ramp protocol corresponded to VO2max.
Methods
Animal
One-year-old male FVB mice (n = 8) were selected for use in this study by virtue of their high level of performance on a treadmill (Lerman et al., 2002). The mice were kept in a specific and opportunistic pathogen-free animal facility (CERFE, Genopole, Evry, France) at a temperature of 22°C and with light-dark cycles 12/12-h. The animals were fed a standard diet ad libitum. Our protocol was approved by our institutions Animal Care and Use Committee on Care and complied with the European Convention of the Council of Europe for the protection of vertebrate animals used for experimental and other scientific purposes.
Familiarization
Mice were familiarized with the single-lane, motorized treadmill (adjustable belt speed: 0–99.9 m.min−1; Columbus Instruments, Columbus, OH, USA) during four 10-min running sessions (at 0, 3, 6, and 9 m.min−1), with a 48-h interval between each session. All mice subsequently included in the study were able to run for the required time at 9 m.min−1. The running speed was not increased further, in order to avoid a training effect.
The Exercise Protocol
The treadmill was set up in a metabolic chamber. Three different protocols were applied: an incremental protocol (IP) with a starting speed of 10 m.min−1 and an increment of 3 m.min−1 every 3 min; a ramp protocol with a starting speed of 3 m.min−1 and an acceleration of 0.05 m.min−1.s−1 (corresponding to 3 m.min−2), hereafter referred to as “Ramp3”; and a ramp protocol with a starting speed of 3 m.min−1 and an acceleration of 0.2 m.min−1.s−1 (corresponding to 12 m.min−2), hereafter referred to as “Ramp12.” Each of the three protocols was performed with two different slopes (0 and 25°); hence, each mouse performed six sessions. To avoid conditioning bias, the test sequence was randomized and there was 24-h interval between each session. The exercise session lasted until exhaustion, which was defined as the mouse's inability to maintain running speed despite being in contact with the electrical grid for more than 5 consecutive seconds (Mille-Hamard et al., 2012). All mice were compliant in all tests. The resting blood lactate concentration was measured at the start of the test ([Lac]rest) and 2 min after the end of each run ([La]max). To this end, a blood drop was collected at the tail vein (using the tail snip method), placed on a test strip and inserted into a lactate analyzer (Lactate Pro, Arkray, Inc., Kyoto, Japan).
Gas Measurements
Ambient air was fed through the metabolic chamber at a rate of 0.66 l.min−1; the flow was chosen such that the incoming vs. outgoing difference in O2 fraction was within the sensor's range of measurement (−0.3 to −0.8% O2). A fan was used to mix the incoming air with the air around the treadmill and blow it toward the animal. The air flowed from the front of the treadmill to the rear of the treadmill and then returned toward the front under the belt. This created a rapid, circular “loop” of mixed gases (i.e., incoming “fresh” air mixed with the accumulated, exhaled gases), from which a sample was drawn for analysis every 5 s. Samples were dried prior to measurement of the O2 and CO2 fractions. The gas analyzers were calibrated with standardized gas mixtures (Air Liquide Santé, Paris, France) before each test session, as recommended by the manufacturer. To allow rapid comparisons over a wide range of body weights (especially with human data), dimensional analyses and empirical studies have shown that VO2 should be divided by the body mass raised to the power of 0.75 (Taylor et al., 1981; Hoydal et al., 2007; Mille-Hamard et al., 2012).
Data Analysis
VO2peak was defined as the highest observed value of VO2 when averaged over successive 15 s periods. VO2max was defined as in humans (i.e., the highest VO2peak value recorded during a set of different test protocols, and the occurrence of a VO2 plateau). The VO2 plateau was determined when the VO2 did not increase by more than 1% of the difference between the VO2 at rest and VO2peak over a 30 s period, despite an increase in running speed. The mouse's maximum speed (Vmax) was defined as the running speed at the end of the protocol. The RER was defined as the ratio between the amount of oxygen (O2) consumed and the amount of carbon dioxide (CO2) produced in the metabolic chamber. The maximum respiratory exchange ratio (RERmax) was defined as the highest observed value of the RER when averaged over successive 15 s periods.
Statistics
Data are expressed as the mean ± standard deviation (SD). Statistical analysis was carried out with a two-way repeated measures ANOVA, followed by a Holm-Sidak post-hoc test. The threshold for statistical significance was set to p < 0.05. All statistical analyses were performed using STATISTICA software (version 9.0, Statsoft, Berkeley, CA, USA).
Results
VO2peak in Each Exercise Protocol
The highest observed VO2peak (59.0 ± 0.61 ml.kg−0.75.min−1, Figure 1) was obtained during the Ramp3 0° protocol. This value was significantly greater than those obtained in the other protocols. The presence of a slope influenced the value of VO2peak, which was higher in IP 25° than in IP 0° but lower in Ramp3 25° and Ramp12 25° than in Ramp3 0° and Ramp12 0°. The minimum VO2 determined at the beginning of the protocol (referred to as the VO2 at rest) was essentially the same in all protocols (mean: 43.6 ± 3.9 ml.kg−0.75.min−1).
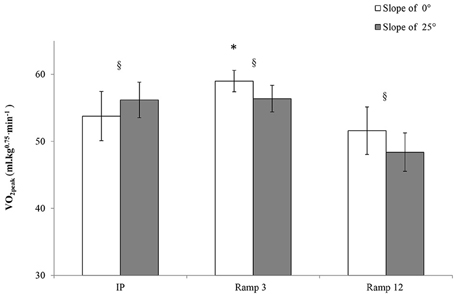
Figure 1. VO2peak in each exercise protocol : one-year-old sedentary FVB/N mice (n = 8) performed six exhaustive exercise protocols with a treadmill slope of 25 or 0°. IP, an incremental protocol with a starting speed of 10 m.min−1 and an increment of 3 m.min−1 every 3 min; Ramp3, a ramp protocol with a starting speed of 3 m.min−1 and an acceleration of 3 m.min−2 (0.05 m.min−1.s−1); Ramp12, a ramp protocol with a starting speed of 3 m.min−1 and an acceleration of 12 m.min−2 (0.2 m.min−1.s−1); §, a significant difference between 25 and 0° for the same protocol (p < 0.05); *, differs significantly from all other protocols (p < 0.05).
Observation of a VO2peak Plateau as a Function of the Exercise Protocol
As shown in Table 1, all mice displayed a VO2 plateau for at least 30 s during the Ramp3 0° and IP 25° protocols (mean plateau duration: 57.5 s ± 11.3 and 75 ± 11.24 s, respectively). During other protocols, some (but not all) mice reached a VO2 plateau for at least 30 s (Table 1)
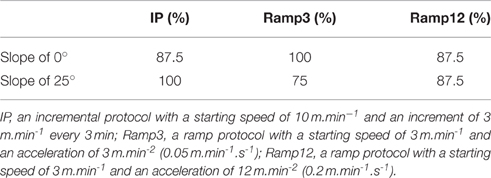
Table 1. Percentages of mice reaching a VO2 plateau for least 30 s, as defined in the Methods section.
Maximal Respiratory Exchange Ratio: RERmax
There were no inter-test differences in RERmax (Figure 2). For Ramp3 0°, the mean RERmax value was 1.06 ± 0.01, and RERmax was greater than 1.05 for seven of the eight mice.
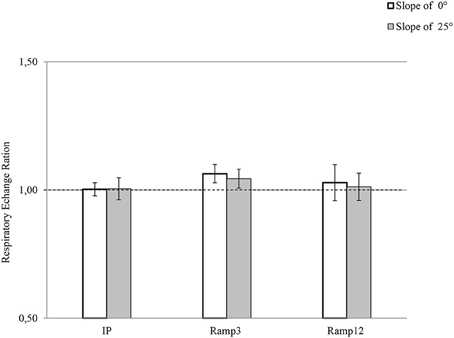
Figure 2. RERmax in each exercise protocol. IP, an incremental protocol with a starting speed of 10 m.min−1 and an increment of 3 m.min−1 every 3 min; Ramp3, a ramp protocol with a starting speed of 3 m.min−1 and an acceleration of 3 m.min−2 (0.05 m.min−1.s−1); Ramp12, a ramp protocol with a starting speed of 3 m.min−1 and an acceleration of 12 m.min−2 (0.2 m.min−1.s−1).
Maximum Blood Lactate Concentration
[La]max was above 6 mmol.l−1 for all mice and all protocols (Figure 3). In the Ramp3 0° protocol, the mean [La]max was 13.80 ± 0.34 and [La]max was greater than 12 mol.l−1 for all mice.
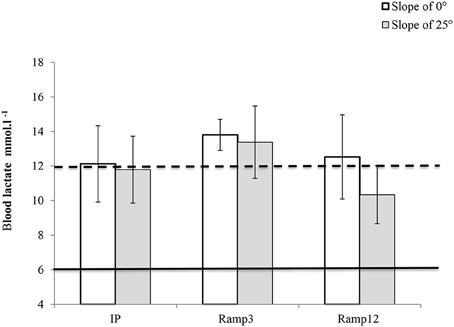
Figure 3. [La]max (measured 2 min after the end of the run) in each exercise protocol. IP, an incremental protocol with a starting speed of 10 m.min−1 and an increment of 3 m.min−1 every 3 min; Ramp3, a ramp protocol with a starting speed of 3 m.min−1 and an acceleration of 3 m.min−2 (0.05 m.min−1.s−1); Ramp12, a ramp protocol with a starting speed of 3 m.min−1 and an acceleration of 12 m.min−2 (0.2 m.min−1.s−1).
Maximal Speed: Vmax
The Vmax of the mice was higher in the ramp protocols (54.88 ± 4.57 m.min−1 for Ramp12 0°; 46.34 ± 2.45 m.min−1 for Ramp3 0°) than in the step protocol (IP 0°: 38.13 ± 1.79 m.min−1) (Figure 4). For all three protocols, Vmax was higher with 0° than with 25°.
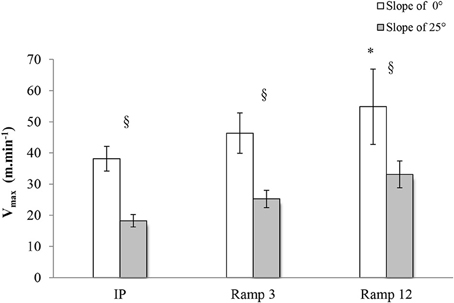
Figure 4. Vmax in each exercise protocol. IP, an incremental protocol with a starting speed of 10 m.min−1 and an increment of 3 m.min−1 every 3 min; Ramp3, a ramp protocol with a starting speed of 3 m.min−1 and an acceleration of 3 m.min−2 (0.05 m.min−1.s−1); Ramp12, a ramp protocol with a starting speed of 3 m.min−1 and an acceleration of 12 m.min−2 (0.2 m.min−1.s−1); §, a significant difference between 25 and 0° for the same protocol (p < 0.05); *, differs significantly from all other protocols (p < 0.05).
Time to Exhaustion
As shown in Figure 5, the time to exhaustion was significantly longer in IP 0° (29.33 ± 1.58 min) than in the two ramp protocols. For example, the time to exhaustion in Ramp3 0° (15.43 ± 0.8 min) was almost half that observed in IP 0°.
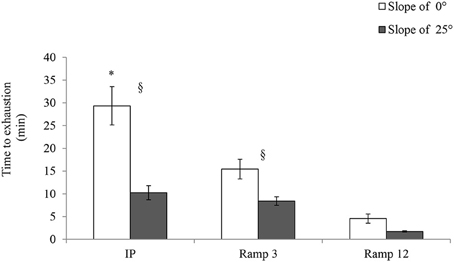
Figure 5. Time to exhaustion. IP, an incremental protocol with a starting speed of 10 m.min−1 and an increment of 3 m.min−1 every 3 min; Ramp3, a ramp protocol with a starting speed of 3 m.min−1 and an acceleration of 3 m.min−2 (0.05 m.min−1.s−1); Ramp12, a ramp protocol with a starting speed of 3 m.min−1 and an acceleration of 12 m.min−2 (0.2 m.min−1.s−1); §, a significant difference between 25 and 0° for the same protocol (p < 0.05); *, differs significantly from all other protocols (p < 0.05).
Discussion
The present study in mice was designed to determine whether (i) a continuous ramp protocol yielded a higher VO2peak than a stepwise, incremental protocol, and (ii) the VO2peak measured in the ramp protocol corresponded to VO2max.
This is an important issue, given that mice are frequently studied models in exercise physiology and that a variety of exercise protocols have been applied in this context. Our main findings were that a ramp protocol (with an acceleration of 3 m.min−2 and no treadmill slope) elicited a higher VO2peak than an incremental protocol (regardless of slope), and that the VO2peak does appear to correspond to the VO2max (given that a VO2 plateau was observed and the secondary criteria were met). The Ramp3 0° protocol is therefore relevant for the determination of VO2max inmice.
According to the literature data, VO2peak in sedentary male mice ranges from 47 to 94 ml.kg−0.75.min−1 (Dohm et al., 1994; Schefer and Talan, 1996; Desai et al., 1999; Niebauer et al., 1999; Kemi et al., 2002). Furthermore, no major gender differences have been reported. Although gender differences have been observed for voluntary exercise (with young female mice running farther and faster than young males Lightfoot et al., 2004; Bartling et al., 2016), studies of forced exercise on a treadmill have not evidenced gender differences for critical speed, maximum distance (Billat et al., 2005; Lightfoot et al., 2007), or VO2peak in untrained mice (Kemi et al., 2002). Hence, we conclude that aerobic capacity does not depend on gender in untrained mice. Along with heterogeneity in the test protocols, several other factors may influence the observed VO2peak. It has been reported that VO2peak falls from 79 ml.kg−0.75.min−1 in young adult (12-month-old) mice to 56 ml.kg−0.75.min−1 in elderly (24-month-old) mice (Schefer and Talan, 1996). Thus, age differences in various studies may account for some of the discrepancies between reported VO2peak values. Moreover, the mouse's level of performance is known to depend on the strain (Lightfoot et al., 2001; Billat et al., 2005). Given that VO2peak is considered to be an indicator of performance, one can legitimately hypothesize that this variable is also influenced by the strain of mouse studied. The only study to date of VO2peak in FVB mice reported a value corresponding to 60 ml.kg−0.75.min−1 (Chow et al., 2007) which falls within the range of values observed in the present study. Hence, the choice of different strains may also account for some of the discrepancies in VO2peak values.
Furthermore, the impact of the exercise protocol used to determine VO2peak values in mice has not previously been assessed. To the best of our knowledge, the only previous study in this field focused on the effect of treadmill slope on VO2peak in an incremental protocol (Wisløff et al., 2001). We hypothesized that the choice of exercise protocol would have a critical impact on the measured VO2peak. For example, Kemi et al.'s (2002) study used an incremental protocol with an increment of 1.8 m.min−1 every 2 min. They reported a mean VO2peak value of 47 ml.kg−0.75.min−1 and a mean time to exhaustion of 30 min. In contrast, Dohm et al. (1994) study used an incremental protocol with an increment of 8.4 m.min−1 every 2 min to obtain a mean VO2peak value of 94 ml.kg−0.75.min−1 and a time to exhaustion of 16 min. The results of the present study showed that the VO2peak value is protocol-dependent (p < 0.05). The highest value was obtained in the Ramp3 0° protocol; hence, ramp protocols are suitable for determining VO2peak in mice. Indeed, the ramp protocol was associated with a shorter time to exhaustion (15 ± 0.82 min in Ramp3 0° and 30 ± 1.51 min in IP 0°). This may explain why VO2peak was higher in the Ramp3 0° protocol than in the IP 0° protocol. In humans, a shorter time to exhaustion is associated with a higher VO2max (Froelicher et al., 1974); this also appears to be true in the mouse.
It has been demonstrated that VO2peak is highest when the treadmill slope is between 15 and 35° (Kemi et al., 2002). Accordingly, we chose a value of 25°. This slope was associated with significant differences in the measured VO2peak (relative to the 0° condition, and for both the incremental protocol and the ramp protocols). Interestingly, the IP 25° protocol yielded a higher VO2peak value that the IP 0° protocol. This confirmed the results of Kemi et al.'s study of an incremental protocol (2002). In contrast, VO2peak was lower for Ramp3 25° than for Ramp3 0°. In exercising human (in whom energy expenditure is mainly related to muscle work), concentric work requires 3- to 5-fold more energy than the same amount of eccentric work. The energy cost of running therefore depends on the relative proportions of these two types of work, which in turn depends on the slope; the steeper the slope at a given speed, the greater the proportion of concentric work and thus the greater the energy expenditure. (Minetti et al., 1993, 1994; Pringle et al., 2002). This phenomenon seems to have occurred in the ramp protocols because the mice attained a lower Vmax when the treadmill was inclined. Furthermore, running on a sloping treadmill may recruit a greater muscle mass (Kemi et al., 2002). Consequently, involvement of a greater muscle mass and a greater proportion of concentric work in ramp protocols with slope might be responsible for fatigue and thus a lower VO2peak. However, the data collected in the present study did not enable us to confirm this hypothesis. Furthermore, it is possible that use of a shallower slope would have increased the concentric work without leading to too much fatigue and thus would have yielded a higher VO2peak value.
As well as being associated with the highest VO2peak value, the Ramp3 0° protocol produced a VO2max plateau for which two secondary criteria (the blood lactate concentration and the RER) were fulfilled. Thus, a ramp protocol with an acceleration of 3 m.min−2 and no slope enables the determination of the VO2max in mice, according to the definition usually applied in humans. Over the last 15 years, a number of researchers have evaluated the influence of data sampling on changes over time in VO2 and the determination of VO2max in human (Astorino et al., 2005; Midgley et al., 2006, 2007; Astorino, 2009). These studies showed that averaging VO2 over successive 15 s periods provided a more accurate measurement of VO2max and increased the likelihood of observing a VO2 plateau. As breath-by-breath sampling is not possible for mice in a metabolic chamber, we used the device's shortest sampling time (5 s, i.e., below the maximum recommended value of 15 s). Furthermore, very few studies have focused on whether a VO2 plateau (which defines VO2max) can be observed in mice. Many researchers have not distinguished between VO2peak and VO2max, and have defined VO2max in different ways. For example, Gebczynski defined VO2max as the highest mean VO2 value over 1 min (Gebczynski and Konarzewski, 2009), and Ferreira et al. (2007) considered that VO2max was equivalent to VO2peak (Ferreira et al., 2007). In contrast, some researchers have stated that VO2max corresponds to the VO2 plateau; unfortunately, the researchers evaluated the VO2 curve visually and did not define criteria for detecting a plateau (Niebauer et al., 1999; Kemi et al., 2002). In 1955, Taylor et al. stated that the change in VO2 (ΔVO2) should be below 2.1 ml.kg−1.min−1 or 150 ml min−1 for more than 30 s if it is to be considered as a VO2max plateau: (Billat et al., 2013). For a sedentary subject, this ΔVO2 represents around 5% of the difference between the VO2 measured at rest and VO2max. In view of our previous data in mice, (Mille-Hamard et al., 2012; Mouisel et al., 2014) and studies indicating that there is not much difference between VO2 at rest and VO2peak in mice (Ferreira et al., 2007; Mazzucatto et al., 2014), we decided to reduce the value of ΔVO2. Hence, in the present study, the VO2 plateau was determined when the VO2 did not increase by more than 1% of the difference between the VO2 at rest and VO2peak over a 30 s period, despite an increase in running speed.
Furthermore, Kemi et al. considered two of the secondary criteria applied in human exercise tests. Given that non-invasive measurement of the heart rate is not practical in mice, Kemi et al. suggested that an RER > 1 and an [La]max > 6 mmol.l−1 can be used to confirm the value of VO2max when a VO2 plateau is not observed (Kemi et al., 2002). Our present data on RERmax and [La]max suggest that the VO2max was attained by all the mice during the Ramp3 0° protocol. The recorded values of RERmax (mean: 1.06 ± 0.01) and [La]max (>12 mmol.l−1) indicated that exercise was strenuous. (Astorino, 2009).
In humans, a standardized stepwise protocol is usually preferred because it enables the determination of other performance indices (blood lactate, ventilatory thresholds, heart rate, etc.) as well as VO2max. In mice, these indices cannot be calculated without using non-routine equipment (an implanted heart rate sensor and a mouthpiece, for example), and so the ramp protocol suggested here (which enables the true VO2max to be determined rapidly) should be preferred. However, it remains to be seen whether the ramp protocol is suitable for all strains and age groups and for both sexes.
Conclusion
The principal findings of this study in the mouse were that (i) the VO2peak observed at the end of exhaustive exercise is protocol-dependent, and (ii) a ramp exercise protocol with an acceleration of 3 m.min−2 (i.e., 0.05 m.min−1.s−1) and no treadmill slope is suitable for determining VO2max as defined in humans.
Author Contributions
MA, RN contributed to the design of the work, the acquisition, analysis, and interpretation of data, drafted the work; LM, IM contributed to the design of the work, the acquisition, analysis, and interpretation of data, drafted the work and revisited it critically for important intellectual content; VB contributed to the design of the work, the interpretation of data, revisited the work critically for important intellectual content. All authors approved the version to be published and agreed to be accountable for all aspects of the work in ensuring that questions related to the accuracy or integrity of any part of the work are appropriately investigated and resolved.
Conflict of Interest Statement
The authors declare that the research was conducted in the absence of any commercial or financial relationships that could be construed as a potential conflict of interest.
References
Astorino, T. A. (2009). Alterations in VOmax and the VO plateau with manipulation of sampling interval. Clin. Physiol. Funct. Imaging 29, 60–67. doi: 10.1111/j.1475-097X.2008.00835.x
Astorino, T. A., Willey, J., Kinnahan, J., Larsson, S. M., Welch, H., and Dalleck, L. C. (2005). Elucidating determinants of the plateau in oxygen consumption at VO2max. Br. J. Sports Med. 39, 655–660. discussion: 660. doi: 10.1136/bjsm.2004.016550
Astrand, I. (1960). Aerobic work capacity in men and women with special reference to age. Acta Physiol. Scand. Suppl. 49, 1–92.
Åstrand, P. O. (1952). Experimental Studies of Physical Working Capacity in Relation to Sex and Age. Copenhagen: E. Munksgaard.
Balke, B., and Ware, R. W. (1959). An experimental study of physical fitness of Air Force personnel. U. S. Armed Forces Med. J. 10, 675–688.
Bartling, B., Al-Robaiy, S., Lehnich, H., Binder, L., Hiebl, B., and Simm, A. (2016). Sex-related differences in the wheel-running activity of mice decline with increasing age. Exp. Gerontol. doi: 10.1016/j.exger.2016.04.011. [Epub ahead of print].
Billat, V. L., Mouisel, E., Roblot, N., and Melki, J. (2005). Inter- and intrastrain variation in mouse critical running speed. J. Appl. Physiol. (1985) 98, 1258–1263. doi: 10.1152/japplphysiol.00991.2004
Billat, V., Petot, H., Karp, J. R., Sarre, G., Morton, R. H., and Mille-Hamard, L. (2013). The sustainability of VO2max: effect of decreasing the workload. Eur. J. Appl. Physiol. 113, 385–394. doi: 10.1007/s00421-012-2424-7
Bruce, R. A., Blackmon, J. R., Jones, J. W., and Strait, G. (1963). Exercising testing in adult normal subjects and cardiac patients. Pediatrics 32(Suppl), 742–756.
Chow, L. S., Greenlund, L. J., Asmann, Y. W., Short, K. R., McCrady, S. K., Levine, J. A., et al. (2007). Impact of endurance training on murine spontaneous activity, muscle mitochondrial DNA abundance, gene transcripts, and function. J. Appl. Physiol. (1985) 102, 1078–1089. doi: 10.1152/japplphysiol.00791.2006
Cohn, J. N. (1987). Quantitative exercise testing for the cardiac patient: the value of monitoring gas exchange. Atlanta, Georgia, March 7–8, 1986. Proceedings. Circulation 76, VI1-58.
Desai, K. H., Schauble, E., Luo, W., Kranias, E., and Bernstein, D. (1999). Phospholamban deficiency does not compromise exercise capacity. Am. J. Physiol. 276, H1172–H1177.
Dohm, M. R., Richardson, C. S., and Garland, T. Jr. (1994). Exercise physiology of wild and random-bred laboratory house mice and their reciprocal hybrids. Am. J. Physiol. 267, R1098–R1108.
Fernando, P., Bonen, A., and Hoffman-Goetz, L. (1993). Predicting submaximal oxygen consumption during treadmill running in mice. Can. J. Physiol. Pharmacol. 71, 854–857. doi: 10.1139/y93-128
Ferreira, J. C., Rolim, N. P., Bartholomeu, J. B., Gobatto, C. A., Kokubun, E., and Brum, P. C. (2007). Maximal lactate steady state in running mice: effect of exercise training. Clin. Exp. Pharmacol. Physiol. 34, 760–765. doi: 10.1111/j.1440-1681.2007.04635.x
Froelicher, V. F. Jr., Brammell, H., Davis, G., Noguera, I., Stewart, A., and Lancaster, M. C. (1974). A comparison of the reproducibility and physiologic response to three maximal treadmill exercise protocols. Chest 65, 512–517. doi: 10.1378/chest.65.5.512
Gebczynski, A. K., and Konarzewski, M. (2009). Metabolic correlates of selection on aerobic capacity in laboratory mice: a test of the model for the evolution of endothermy. J. Exp. Biol. 212, 2872–2878. doi: 10.1242/jeb.030874
Hawkins, M. N., Raven, P. B., Snell, P. G., Stray-Gundersen, J., and Levine, B. D. (2007). Maximal oxygen uptake as a parametric measure of cardiorespiratory capacity. Med. Sci. Sports Exerc. 39, 103–107. doi: 10.1249/01.mss.0000241641.75101.64
Hill, A. V., and Lupton, H. (1923). Muscular exercise, lactic acid, and the supply and utilization of oxygen. QJM 16, 135–171. doi: 10.1093/qjmed/os-16.62.135
Høydal, M. A., Wisløff, U., Kemi, O. J., and Ellingsen, O. (2007). Running speed and maximal oxygen uptake in rats and mice: practical implications for exercise training. Eur. J. Cardiovasc. Prev. Rehabil. 14, 753–760. doi: 10.1097/HJR.0b013e3281eacef1
Issekutz, B., Birkhead, N. C., and Rodahl, K. (1962). Use of respiratory quotients in assessment of aerobic work capacity. J. Appl. Physiol. 17, 47–50.
Kemi, O. J., Loennechen, J. P., Wisloff, U., and Ellingsen, Ø. (2002). Intensity-controlled treadmill running in mice: cardiac and skeletal muscle hypertrophy. J. Appl. Physiol. (1985) 93, 1301–1309. doi: 10.1152/japplphysiol.00231.2002
Lerman, I., Harrison, B. C., Freeman, K., Hewett, T. E., Allen, D. L., Robbins, J., et al. (2002). Genetic variability in forced and voluntary endurance exercise performance in seven inbred mouse strains. J. Appl. Physiol. (1985) 92, 2245–2255. doi: 10.1152/japplphysiol.01045.2001
Lightfoot, J. T., Turner, M. J., Daves, M., Vordermark, A., and Kleeberger, S. R. (2004). Genetic influence on daily wheel running activity level. Physiol. Genomics 19, 270–276. doi: 10.1152/physiolgenomics.00125.2004
Lightfoot, J. T., Turner, M. J., Debate, K. A., and Kleeberger, S. R. (2001). Interstrain variation in murine aerobic capacity. Med. Sci. Sports Exerc. 33, 2053–2057. doi: 10.1097/00005768-200112000-00012
Lightfoot, J. T., Turner, M. J., Knab, A. K., Jedlicka, A. E., Oshimura, T., Marzec, J., et al. (2007). Quantitative trait loci associated with maximal exercise endurance in mice. J. Appl. Physiol. (1985) 103, 105–110. doi: 10.1152/japplphysiol.01328.2006
Maritz, J. S., Morrison, J. F., Peter, J., Strydom, N. B., and Wyndham, C. H. (1961). A practical method of estimating an individual's maximal oxygen intake. Ergonomics 4, 97–122. doi: 10.1080/00140136108930512
Mazzucatto, F., Higa, T. S., Fonseca-Alaniz, M. H., and Evangelista, F. S. (2014). Reversal of metabolic adaptations induced by physical training after two weeks of physical detraining. Int. J. Clin. Exp. Med. 7, 2000–2008.
Midgley, A. W., McNaughton, L. R., and Carroll, S. (2006). Verification phase as a useful tool in the determination of the maximal oxygen uptake of distance runners. Appl. Physiol. Nutr. Metab. 31, 541–548. doi: 10.1139/h06-023
Midgley, A. W., McNaughton, L. R., and Carroll, S. (2007). Physiological determinants of time to exhaustion during intermittent treadmill running at vV(.-)O(2max). Int. J. Sports Med. 28, 273–280. doi: 10.1055/s-2006-924336
Mille-Hamard, L., Billat, V. L., Henry, E., Bonnamy, B., Joly, F., Benech, P., et al. (2012). Skeletal muscle alterations and exercise performance decrease in erythropoietin-deficient mice: a comparative study. BMC Med. Genomics 5:29. doi: 10.1186/1755-8794-5-29
Minetti, A. E., Ardigò, L. P., and Saibene, F. (1993). Mechanical determinants of gradient walking energetics in man. J. Physiol. 472, 725–735. doi: 10.1113/jphysiol.1993.sp019969
Minetti, A. E., Ardigò, L. P., and Saibene, F. (1994). Mechanical determinants of the minimum energy cost of gradient running in humans. J. Exp. Biol. 195, 211–225.
Mouisel, E., Relizani, K., Mille-Hamard, L., Denis, R., Hourdé, C., Agbulut, O., et al. (2014). Myostatin is a key mediator between energy metabolism and endurance capacity of skeletal muscle. Am. J. Physiol. Regul. Integr. Comp. Physiol. 307, R444–R454. doi: 10.1152/ajpregu.00377.2013
Niebauer, J., Maxwell, A. J., Lin, P. S., Tsao, P. S., Kosek, J., Bernstein, D., et al. (1999). Impaired aerobic capacity in hypercholesterolemic mice: partial reversal by exercise training. Am. J. Physiol. 276, H1346–H1354.
Pringle, J. S., Carter, H., Doust, J. H., and Jones, A. M. (2002). Oxygen uptake kinetics during horizontal and uphill treadmill running in humans. Eur. J. Appl. Physiol. 88, 163–169. doi: 10.1007/s00421-002-0687-0
Rezende, E. L., Chappell, M. A., Gomes, F. R., Malisch, J. L., and Garland, T. Jr. (2005). Maximal metabolic rates during voluntary exercise, forced exercise, and cold exposure in house mice selectively bred for high wheel-running. J. Exp. Biol. 208, 2447–2458. doi: 10.1242/jeb.01631
Schefer, V., and Talan, M. I. (1996). Oxygen consumption in adult and AGED C57BL/6J mice during acute treadmill exercise of different intensity. Exp. Gerontol. 31, 387–392. doi: 10.1016/0531-5565(95)02032-2
Taylor, C. R., Maloiy, G. M., Weibel, E. R., Langman, V. A., Kamau, J. M., Seeherman, H. J., et al. (1981). Design of the mammalian respiratory system. III Scaling maximum aerobic capacity to body mass: wild and domestic mammals. Respir Physiol. 44, 25–37. doi: 10.1016/0034-5687(81)90075-X
Whipp, B. J., Davis, J. A., Torres, F., and Wasserman, K. (1981). A test to determine parameters of aerobic function during exercise. J. Appl. Physiol. 50, 217–221.
Wisløff, U., Helgerud, J., Kemi, O. J., and Ellingsen, O. (2001). Intensity-controlled treadmill running in rats: VO(2 max) and cardiac hypertrophy. Am. J. Physiol. Heart Circ. Physiol. 280, H1301–H1310. Available online at: http://ajpheart.physiology.org/content/280/3/H1301.full.pdf+html
Keywords: VO2max, mice, exercise protocol, comparative physiology, performance
Citation: Ayachi M, Niel R, Momken I, Billat VL and Mille-Hamard L (2016) Validation of a Ramp Running Protocol for Determination of the True VO2max in Mice. Front. Physiol. 7:372. doi: 10.3389/fphys.2016.00372
Received: 24 May 2016; Accepted: 12 August 2016;
Published: 29 August 2016.
Edited by:
Gary Iwamoto, University of Illinois at Urbana–Champaign, USAReviewed by:
Amanda Nelson, University of Wisconsin–Green Bay, USAThomas Lowder, University of Central Arkansas, USA
Copyright © 2016 Ayachi, Niel, Momken, Billat and Mille-Hamard. This is an open-access article distributed under the terms of the Creative Commons Attribution License (CC BY). The use, distribution or reproduction in other forums is permitted, provided the original author(s) or licensor are credited and that the original publication in this journal is cited, in accordance with accepted academic practice. No use, distribution or reproduction is permitted which does not comply with these terms.
*Correspondence: Laurence Mille-Hamard, bGF1cmVuY2UuaGFtYXJkQHVuaXYtZXZyeS5mcg==