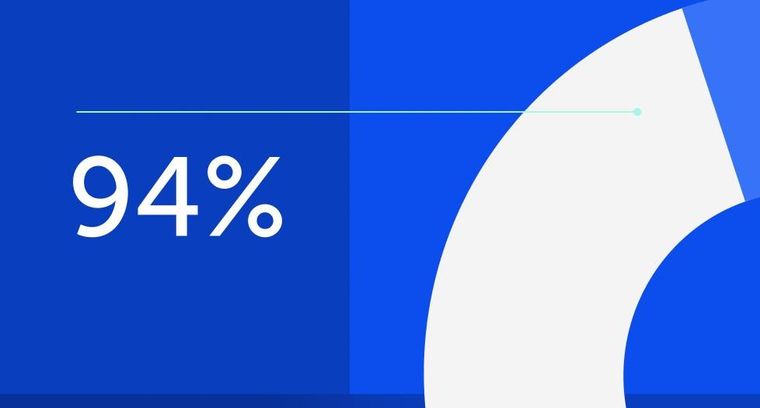
94% of researchers rate our articles as excellent or good
Learn more about the work of our research integrity team to safeguard the quality of each article we publish.
Find out more
REVIEW article
Front. Physiol., 24 June 2016
Sec. Integrative Physiology
Volume 7 - 2016 | https://doi.org/10.3389/fphys.2016.00251
This article is part of the Research TopicCelebrating Twenty Years of the Brazilian Symposium on Cardiovascular PhysiologyView all 23 articles
Emotional stress has been recognized as a modifiable risk factor for cardiovascular diseases. The impact of stress on physiological and psychological processes is determined by characteristics of the stress stimulus. For example, distinct responses are induced by acute vs. chronic aversive stimuli. Additionally, the magnitude of stress responses has been reported to be inversely related to the degree of predictability of the aversive stimulus. Therefore, the purpose of the present review was to discuss experimental research in animal models describing the influence of stressor stimulus characteristics, such as chronicity and predictability, in cardiovascular dysfunctions induced by emotional stress. Regarding chronicity, the importance of cardiovascular and autonomic adjustments during acute stress sessions and cardiovascular consequences of frequent stress response activation during repeated exposure to aversive threats (i.e., chronic stress) is discussed. Evidence of the cardiovascular and autonomic changes induced by chronic stressors involving daily exposure to the same stressor (predictable) vs. different stressors (unpredictable) is reviewed and discussed in terms of the impact of predictability in cardiovascular dysfunctions induced by stress.
Convergent clinical and preclinical studies have provided evidence on the important role of psychosocial factors in the etiology and progression of cardiovascular diseases (Friedman and Rosenman, 1959; Rosenman et al., 1975; Kawachi et al., 1994; Ford et al., 1998; Rozanski et al., 1999; Rugulies, 2002; Smith et al., 2004; Grippo and Johnson, 2009; Roest et al., 2010; Carnevali et al., 2013a; Sgoifo et al., 2014). Among several psychosocial factors, psychological stress has been recognized as a modifiable risk factor for several cardiovascular dysfunctions (Steptoe and Kivimaki, 2012; von Känel, 2012; Inoue, 2014). Indeed, epidemiological and experimental results on humans and animals have demonstrated the influence of psychosocial stress on cardiovascular health (Rosengren et al., 2004; Kivimaki et al., 2006b; Grippo and Johnson, 2009; Steptoe and Kivimaki, 2012; Jarczok et al., 2013; Sgoifo et al., 2014). The association between stress and cardiovascular diseases has been shown to be independent of traditional cardiovascular risk factors (e.g., age, sex, smoking, diabetes mellitus, and obesity; Rosengren et al., 2004; Kivimaki et al., 2006b; Steptoe and Kivimaki, 2012).
The impact of stress on physiological and psychological processes is determined by stressor characteristics, such as chronicity, predictability, and severity (Nalivaiko, 2011; Steptoe and Kivimaki, 2012; Herman, 2013). Indeed, distinct cardiovascular and neuroendocrine changes have been reported following acute vs. chronic stress regimens (Nalivaiko, 2011; Herman, 2013). Furthermore, studies in animals comparing the impact of predictable vs. unpredictable stressor stimuli demonstrated that the latter induces more severe behavioral and physiological consequences (Bassett et al., 1973; Magariños and McEwen, 1995; Marin et al., 2007; Flak et al., 2012; Kopp et al., 2013; Smith et al., 2013; Duarte et al., 2015a). A dose–response pattern of stress-induced cardiovascular dysfunctions has also been reported, and stress severity may be modulated by the duration and/or frequency of the exposure to stress stimulus (Marmot et al., 1991; Kivimaki et al., 2006a; Chandola et al., 2008; Steptoe and Kivimaki, 2012).
The purpose of the present review is to discuss experimental research in animal models describing the impact of emotional stress on cardiovascular function. The primary focus of this review is to discuss the influence of stress type in stress-induced cardiovascular changes. Therefore, the first section discusses the influence of chronicity by summarizing the cardiovascular responses during acute stress sessions and the cardiovascular dysfunctions following repeated exposure to aversive stimuli (i.e., chronic stress). The second section discusses the impact of the predictability of stressor stimulus in cardiovascular and autonomic changes induced by chronic stress.
A coordinated and complex set of physiological changes is generated during acute stress sessions. Changes in the autonomic nervous system activity promote immediate responses during aversive threats, which are mainly characterized by changes in the cardiovascular function. Cardiovascular responses typically consist of increase in blood pressure, heart rate (HR), and cardiac output (Hubbard et al., 1986; Schadt and Hasser, 1998; Dos Reis et al., 2014). However, these responses are consequences of more complex changes in cardiovascular function. Below is discussed the blood flow redistribution, changes of cardiac autonomic activity, and modulation of baroreflex function observed during an acute stress session. Additionally, the recovery of cardiovascular function after ending the stress session is described.
Blood flow redistribution occurs from the visceral and cutaneous beds toward the skeletal muscle vasculature during aversive threats (Kirby et al., 1987; Kapusta et al., 1989; Knardahl and Hendley, 1990; Viken et al., 1991; Zhang et al., 1996; Schadt and Hasser, 1998; Blessing, 2003). Blood flow redistribution is mediated mainly by vasoconstriction of the splanchnic, renal, cutaneous, and celiac vascular beds and by vasodilatation of the skeletal muscle vasculature (Iriuchijima et al., 1982; Kirby et al., 1987; Kapusta et al., 1989; Zhang et al., 1992, 1996; Anderson and Overton, 1994; Schadt and Hasser, 1998; Blessing, 2003; Mohammed et al., 2014). The increase in skeletal muscle blood flow is primarily due to an increase in the level of circulating catecholamines and activation of β2-adrenoceptors in the skeletal muscle beds, whereas vasoconstriction in the visceral vasculature is mediated by catecholamine released from both the adrenal medulla and sympathetic nerve fibers and activation of α-adrenoceptors (Iriuchijima et al., 1982; Knardahl and Hendley, 1990; Zhang et al., 1992, 1996).
An increase in the total peripheral resistance during stress was reported in borderline hypertensive rats (Hatton et al., 1997). However, studies in normotensive animals documented little or no change in this parameter (Hubbard et al., 1986; Martin et al., 1996; Zhang et al., 1996; Schadt and Hasser, 1998), indicating that vasodilation mostly balanced vasoconstriction. Therefore, the increase in cardiac output is possibly the main mechanism mediating the moderate increase in arterial pressure during aversive threats in rodents. In this regard, hemodynamic changes during stress also include an increase in venomotor tone via activation of α2-adrenoceptors (Martin et al., 1996; Schadt and Hasser, 1998), which increase venous return and contribute to stress-induced increase in the cardiac output.
The tachycardic response during aversive threats is mediated by an increase in the sympathetic tone to the heart (Iwata and LeDoux, 1988; Baudrie et al., 1997; Sgoifo et al., 1999; van den Buuse et al., 2001; Carrive, 2006; Crestani et al., 2010b; Dos Reis et al., 2014). However, stress-induced tachycardic response was enhanced following systemic treatment with muscarinic cholinergic receptor antagonists (Iwata and LeDoux, 1988; Baudrie et al., 1997; Nijsen et al., 1998; Carrive, 2006; Crestani et al., 2009; Dos Reis et al., 2014), indicating an increase in the parasympathetic tone to the heart. Indeed, coactivation of cardiac sympathetic and parasympathetic activities has been documented during aversive situations (Iwata and LeDoux, 1988; Baudrie et al., 1997; Carrive, 2006; Dos Reis et al., 2014). The increase in the parasympathetic tone to the heart allows precise response control, reducing the amplitude of the response, and counteracting excessive cardiac activation associated with cardiac sympathetic system activation alone. The parasympathetic activation and the consequent reduction of cardiac response possibly contributes to a functional state stabilization of the heart (Paton et al., 2005).
Change in baroreflex function is a relevant mechanism controlling cardiovascular and autonomic responses during aversive threats. The baroreflex is an important mechanism for arterial pressure regulation. For example, an increase in arterial pressure activates baroreceptors within the wall of the carotid arteries and aorta that in turn increase cardiac parasympathetic activity and decrease sympathetic activity, leading to decreased HR, vascular resistance, and venous return (Michelini, 1994; Sved and Gordon, 1994). However, as stated above, the increase in arterial pressure during stress is followed by a parallel enhancement in sympathetic activity and HR. Hilton (1963) addressed first the question regarding how parallel increase in blood pressure, HR, and sympathetic activity occurs during aversive situations. Based on the responses obtained by stimulation of the hypothalamic defense area, which mimics the cardiovascular responses induced by stress, baroreflex function was first claimed to be suppressed during defensive behavior (Hilton, 1963; Coote et al., 1979). However, further studies consistently demonstrated that pressor, tachycardic, sympathetic activation, and regional vasoconstrictor responses observed during emotional stress are enhanced in sinoaortic baroreceptor-denervated rats (Norman et al., 1981; Buchholz et al., 1986; Zhang et al., 1996; Dos Reis et al., 2014), indicating an active role of the baroreflex counteracting stress-induced autonomic and cardiovascular responses during defensive responses. Indeed, baroreflex is clearly functional during aversive threats, but it curves for both renal sympathetic nerve activity and HR shifts to the right and upward (Shammas et al., 1988; Hatton et al., 1997; Schadt and Hasser, 1998; Kanbar et al., 2007; Burke and Head, 2009; Crestani et al., 2010b; Miki and Yoshimoto, 2013), allowing a parallel increase in arterial pressure, HR, and sympathetic activity. A schematic representation of the right and upward shift of the baroreflex curve during aversive situations is presented in Figure 1.
Figure 1. Schematic representation sketching the modulation of baroreflex function during emotional stress. Figure schematically represents sigmoid baroreflex curves correlating mean arterial pressure (MAP) and sympathetic nerve activity (SNA) or heart rate (HR) in basal condition (basal) and during an aversive situation (stress). Note that baroreflex curves shifts to the right (green arrow) and upward (red arrow). The rightward shift represents a resetting of the operating point of baroreflex toward higher arterial pressure values, whereas the upward shift is due to increase in SNA and HR during stress (possibly mediated by mechanisms independent of baroreflex resetting). For details, see text in the section “Cardiovascular responses during acute sessions of stress.”
The rightward shift represents a resetting of the operating point of the baroreflex toward higher arterial pressure values (Figure 1). Therefore, the baroreflex operates under higher arterial pressure values during stress. This idea is supported by evidence that a decrease in the increase in arterial pressure due to treatment with α-adrenoceptor antagonists (i.e., maintaining arterial pressure values below the new operating point of the baroreflex) enhanced the tachycardic response during aversive situations (Baudrie et al., 1997; Carrive, 2002; Dos Reis et al., 2014), possibly to increase arterial pressure to some preset value. The baroreflex resetting seems to be a mechanism allowing the simultaneous increase in arterial pressure and sympathetic activity/HR rather than generating the cardiovascular responses to stress, once sinoaortic baroreceptor denervation facilitated stress-induced cardiovascular and autonomic adjustments, as stated above (Norman et al., 1981; Buchholz et al., 1986; Zhang et al., 1996; Dos Reis et al., 2014). However, Zhang et al. (1996) reported that vasodilatation in the skeletal muscle vasculature was reduced in sinoaortic baroreceptor-denervated rats, indicating that baroreflex activity resetting may contribute to blood flow redistribution during aversive threats.
The abovementioned evidence indicates that autonomic and cardiovascular responses to stress are mediated by mechanisms acting independent of the changes in baroreflex function. However, the increase in sympathetic activity and HR is responsible for the upward shift of the baroreflex curves (Schadt and Hasser, 1998; Crestani et al., 2010b; Miki and Yoshimoto, 2013; Figure 1). Indeed, the organism operates at higher values of HR and sympathetic activity during stress, inducing a vertical change in the baroreflex curves (Schadt and Hasser, 1998; Crestani et al., 2010b; Miki and Yoshimoto, 2013; Figure 1).
Arterial pressure and HR progressively decrease toward baseline values during recovery from an aversive stimulus, returning to pre-stress levels ~30–60 min after ending stress (McDougall et al., 2000; Vianna and Carrive, 2005; Igosheva et al., 2007; Porter et al., 2007; Crestani et al., 2010b; Krause et al., 2011). Nevertheless, we have previously reported that besides arterial pressure and HR had already returned to pre-stress values 30 min after ending an acute session of restraint stress, the baroreflex activity remained similar to that observed during stress, returning to control values only 60 min after ending the stress session (Crestani et al., 2010b). These findings indicate non-persistent effects of stress on cardiovascular function, but suggest a period of vulnerability during the recovery period, once the baroreflex function is reset to higher arterial pressure values. Nevertheless, persistence of the stress effects on baroreflex activity may be important to counteract excessive activation of depressor mechanisms during the recovery period, thus allowing adequate return of blood pressure to normal values without abrupt falls. Indeed, central and peripheral depressor mechanisms are activated during the recovery period and mediate the return of arterial pressure to resting values (Yip and Krukoff, 2002; D'Angelo et al., 2006).
The physiological and behavioral responses during acute stress sessions constitute important adaptive responses, maintaining homeostasis and ensuring survival (Sterling and Eyer, 1988; Sterling, 2012). However, the frequent/prolonged activation (i.e., over-exposure to stress changes) or generation of inadequate responses (i.e., insufficient responses to individual needs) during stressful events can result in disease development (McEwen and Stellar, 1993; McEwen, 1998; Danese and McEwen, 2012). A situation of over-exposure to stress responses occurs during repeated exposure to aversive threats (i.e., chronic stress; McEwen, 1998). Regarding the cardiovascular consequences of stress, besides reports of acute cardiovascular events such as ventricular arrhythmias, myocardial infarction, and cardiomyopathy (potentially fatal) after exposure to acute stressful situations (Cannon, 1942; Lown et al., 1973; Natelson and Cagin, 1979; Sgoifo et al., 1997, 1999; Ueyama et al., 2002; Mostofsky et al., 2014; Lagraauw et al., 2015), enduring cardiovascular changes (days to years) are mainly provoked by long-term exposure to stressful events (Nalivaiko, 2011). Therefore, the frequency of exposure to stress (chronicity) is an important factor in determining the pattern of stress-induced cardiovascular diseases. Below, we discuss the evidence of enduring changes in arterial pressure, HR, cardiac function, autonomic activity, and baroreflex function that lasts beyond the duration of chronic stress protocols.
Despite of pronounced neuroendocrine (e.g., increased hypothalamic–pituitary–adrenal, HPA, axis activity), somatic (e.g., reduced body weight gain, adrenal hypertrophy, and thymus atrophy), and behavioral (e.g., anxiety- and depression-like behavior) changes following long-term exposure to stressful events, preclinical studies have failed to consistently reproduce epidemiological findings demonstrating a relationship between chronically stressful life events and hypertension in animals (Timio et al., 1988; Markovitz et al., 2004). Indeed, inconsistent findings have been reported regarding the effects of chronic stress on arterial pressure in animal models. Nalivaiko (2011) reviewed in details publications that investigated the effect of different chronic stress models on arterial pressure in several rat strains. An important consideration raised was the influence of the method used to assess arterial pressure. For example, most studies reviewed by Nalivaiko (2011) that identified a significant hypertensive effect following exposure to chronic stressors used the tail-cuff method (indirect) to measure the arterial pressure. Even in studies that validated the findings obtained through the tail-cuff method by direct methods (through implantation of a catheter into the femoral or carotid artery at the end of the stress protocol), the arterial pressure increase obtained in the direct method was lower than that in the tail-cuff method (Nalivaiko, 2011). Similar analysis of studies published after 2007 (date of the more recent study included in the Nalivaiko's review; Table 1) further reinforces observation regarding the influence of the method used to assess arterial pressure in the identification of stress-induced hypertension.
Table 1. Studies examining the effect of the exposure to chronic stressors in basal parameters of mean arterial pressure (MAP).
Animals need to be restrained (similar to the restraint stress model) in the tail-cuff method, and marked pressor and tachycardic responses are observed, even following recommended habituation (Grundt et al., 2009). Based on this, Nalivaiko (2011) proposed that the identification of hypertensive effect preferentially by the tail-cuff method would be due to a more vigorous reaction of the animals stressed to the tail-cuff procedure. Hence, it has been reported that exposure to chronic stressors increases pressor response during acute aversive stimuli (Grippo et al., 2002, 2006; Cudnoch-Jedrzejewska et al., 2010, 2014; Maslova et al., 2010).
Another factor mentioned by Nalivaiko (2011) could be the possible influence of coping strategies in models providing actual or perceived control over a stressful situation, which may occur, for example, in highly predictable protocols (e.g., during repeated exposure to the same stressor). We recently addressed this issue by comparing the impact on arterial pressure (measured by the direct method) of a predictable (repeated restraint stress, RRS) vs. an unpredictable (chronic variable stress, CVS) chronic stress protocol (Duarte et al., 2015a) (see the next section for details). We observed a similar small pressor effect (+10–15 mm Hg) following exposure to either RRS or CVS for 10 days (Duarte et al., 2015a). Other studies using longer CVS protocols (4–8weeks) reported either similar effect (~+8 mm Hg) (Grippo et al., 2008) or absence of changes on arterial pressure measured by either direct methods or telemetry (Grippo et al., 2002, 2006; Cudnoch-Jedrzejewska et al., 2010; Demirtas et al., 2014; Stanley et al., 2014). In addition, Bobrovskaya et al. (2013) did not identify changes in mean arterial pressure measured by telemetry (only a small increase in systolic arterial pressure was identified) following repeated exposure to footshock (more intense aversive stimulus) for 6 weeks in an unpredictable schedule. Thus, the small hypertensive effect obtained by direct measurement of arterial pressure seems to be independent of intensity, predictability, and duration of chronic stress protocol.
An additional factor considered in our review (Table 1) was an elevated baseline arterial pressure in the control group, which would indicate some degree of stress induced by the experimental procedure (independent of chronic stressor) that in turn could buffer effects of chronic stress. However, independently of the chronic stress paradigm, a similar small hypertensive effect (~10 mm Hg) was observed following long-term stress exposure in studies wherein baseline mean arterial pressure in control groups ranged from 95 to 120 mmHg (Grippo et al., 2008; Daubert et al., 2012; Duarte et al., 2015a; Cruz et al., 2016) (Table 1). It has also been documented that cardiovascular dysfunctions induced by chronic stressors may be related to animals age (Crestani, 2016); hence, an influence of age (e.g., young vs. adult) was considered in this review (Table 1). However, the influence of age seems to be related to the type of chronic stressor. For example, social isolation for 3 weeks increased arterial pressure in adolescent rats, without affecting this parameter in adult animals (Cruz et al., 2016). In contrast, RRS induced a mild hypertensive effect in adult animals alone (Duarte et al., 2015a).
More consistent results have been reported for HR. Table 2 summarizes the main findings of changes in baseline HR following exposure to chronic stressors. Increase in baseline HR has been reported in many studies, but this effect seems to be related to the chronic stressor type. Indeed, resting tachycardia has been consistently documented following exposure to two animal models of chronic stress: the CVS and chronic social isolation in prairie voles (a highly social rodent species; Table 2). Resting tachycardia induced by both these models was independent of the protocol length. For example, increased baseline HR was observed following exposure to protocols ranging from 10 days to 8 weeks of CVS (Grippo et al., 2002, 2008; Mercanoglu et al., 2008; Bouzinova et al., 2012; Duarte et al., 2015a) and 5 days to 8 weeks of social isolation in prairie voles (Grippo et al., 2007, 2011; McNeal et al., 2014). Moreover, the increase in HR was not affected by baseline HR in control animals (indicative of some degree of stress of the experimental procedure, see discussion above) and by animal age (Table 2).
Table 2. Studies examining the effect of the exposure to chronic stressors in basal parameters of heart rate (HR).
Although, most studies investigating the effect of chronic social isolation in prairie voles have used female animals (Table 2), resting tachycardia was also reported in male prairie voles following a period of social isolation (McNeal et al., 2014). CVS-induced increase in HR was evaluated only in males animals, but was documented in both Wistar and Sprague–Dawley rats (Grippo et al., 2002, 2006, 2008; Bouzinova et al., 2012; Duarte et al., 2015a; Matchkov et al., 2015), indicating that the effect is independent of the rat strain. As discussed for the effect of stress on arterial pressure, we note higher responses in studies that measured HR by the tail-cuff method compared with data obtained by other methods (Table 2). As discussed above, it can be related to a more vigorous reaction of the stressed animals to the tail-cuff procedure (Nalivaiko, 2011).
Despite the absence of changes in baseline HR, some models induce profound changes in cardiac function. For example, repeated social defeat episodes caused accumulation of fibrous tissue in the left ventricular myocardium, maladaptive cardiac hypertrophy, changes in electrical conduction system of the heart (e.g., reduced myocardial refractoriness and impaired conduction), and increased susceptibility to cardiac arrhythmias (Gelsema et al., 1994; Costoli et al., 2004; Carnevali et al., 2013b, 2015; Sgoifo et al., 2014). Disruption of the circadian rhythm for HR has also been reported following repeated exposure to social defeat (Tornatzky and Miczek, 1993; Sgoifo et al., 2002; Carnevali et al., 2015).
RRS is another chronic stressor that does not induce significant changes in baseline HR (Table 2), but cardiac hypertrophy (Nagaraja and Jeganathan, 1999; Bruder-Nascimento et al., 2012; Duarte et al., 2015a), electrocardiogram (ECG) abnormality, myocardium injury, and cardiac dysfunction (Zhao et al., 2007; Roth et al., 2015) have been reported in animals exposed to this experimental model of stress. Moreover, RRS increased the size of infarction and the incidence of potentially fatal arrhythmias induced by myocardial ischemia (Scheuer and Mifflin, 1998), increased plaque formation in the coronary arteries and occurrence of myocardial infarctions in mice susceptible to atherosclerosis (Roth et al., 2015), and exacerbated hypertension and left ventricular hypertrophy, fibrosis, and diastolic dysfunction related to metabolic syndrome (Habib et al., 2015; Matsuura et al., 2015). These results indicate that RRS may affect the outcome of cardiac diseases.
Consistent findings have also indicated that crowding stress (i.e., social stress that causes competition for resources such as space, food, and water) does not affect baseline HR (Table 2). Nevertheless, analysis of responses to myocardial ischemia indicated an impairment of post-ischemic recovery of cardiac mechanical function and aggravation of tachyarrhythmia and ventricular fibrillation in animals subjected to crowding stress (Ravingerova et al., 2011; Ledvenyiova-Farkasova et al., 2015), suggesting that exposure to this social stressor reduces the tolerance to cardiac ischemia. Maladaptive cardiac hypertrophy, ECG abnormality, and impairment of coronary perfusion of the myocardium have also been reported following exposure to crowding stress (Nagaraja and Jeganathan, 1999; Ravingerova et al., 2011).
In addition to causing tachycardia, cardiac dysfunctions have also been reported following exposure to either CVS or chronic social isolation in prairie voles. Indeed, increased susceptibility to experimentally and stress-induced cardiac arrhythmias was reported in animals subjected to either stressor (Grippo et al., 2004, 2010, 2012a; Liang et al., 2015), and CVS increased the infarcted area after myocardial ischemia (Mercanoglu et al., 2008). These findings indicate that these experimental models of stress may increase the vulnerability and severity of cardiac complications. In addition, cardiac contractile dysfunction was reported in animals subjected to CVS protocols (Grippo et al., 2006; Xie et al., 2012).
Studies in the literature also investigated a possible impact of chronic stressors in the pacemaker activity of the sinoatrial node, referred as intrinsic HR. It can be assessed experimentally by dual blockade of sympathetic and parasympathetic activities of the heart via combined systemic treatment with β-adrenoceptor antagonists (i.e., sympathetic blocker; e.g., propranolol or atenolol) and muscarinic cholinergic receptors (i.e., parasympathetic blocker; e.g., methylatropine). However, intrinsic HR was not affected after exposure to either CVS (Grippo et al., 2002; Almeida et al., 2015; Duarte et al., 2015a), chronic social isolation in prairie voles (Grippo et al., 2007, 2009; McNeal et al., 2014), or RRS (Duarte et al., 2015a). This finding seems to be independent of the duration of chronic stress protocol. For example, intrinsic HR was investigated in CVS protocols ranged from 10 days to 4 weeks (Grippo et al., 2002; Almeida et al., 2015; Duarte et al., 2015a), whereas social isolation in prairie voles ranged from 5 days to 4 weeks (Grippo et al., 2007; McNeal et al., 2014). To the best of my knowledge, a possible impact of other animal models in cardiac pacemaker activity has never been evaluated.
Reduction in HR variability following exposure to different animal models of chronic stress has been described (Grippo et al., 2002, 2009; Wood et al., 2012; Sévoz-Couche et al., 2013), which indicates changes in cardiac autonomic activity. Indeed, analysis of cardiac autonomic activity by either power spectral analysis of oscillatory components of HR or pharmacological blockade of cardiac sympathetic (e.g., treatment with propranolol) and parasympathetic (e.g., treatment with methylatropine) activities indicated significant stress-induced changes in cardiac autonomic activity. Nevertheless, these alterations seem to be specific to the chronic stressor type. For example, pharmacological blockade of cardiac autonomic activity revealed that CVS increased the sympathetic tone to the heart, without significantly affecting cardiac parasympathetic activity (Grippo et al., 2002; Duarte et al., 2015a). It was also evidenced by demonstration of an increase in LF/HF ratio of HR variability (Bundzikova-Osacka et al., 2015), which indicates a change in cardiac sympathovagal balance toward a sympathetic predominance. In contrast, the changes in cardiac autonomic activity induced by chronic social isolation in prairie voles, evidenced by pharmacological autonomic blockade, were characterized by both a decrease in parasympathetic and an increase in sympathetic tone to the heart (Grippo et al., 2007, 2009; McNeal et al., 2014). Despite specific changes in sympathetic and parasympathetic activities, the changes induced by either CVS or social isolation in prairie voles were characterized by a change in cardiac sympathovagal balance toward a sympathetic predominance, which is in line with the resting tachycardia induced by these experimental models. Moreover, the increase in sympathetic contribution of cardiac autonomic balance corroborates the increased susceptibility to cardiac arrhythmias induced by both models (Grippo et al., 2004, 2006, 2012a), as well as with cardiac contractile dysfunction (Grippo et al., 2006; Xie et al., 2012) and increased severity of myocardial ischemia (Mercanoglu et al., 2008) observed after exposure to CVS. Studies have not reported changes in cardiac sympathovagal balance following exposure to RRS protocols (Daubert et al., 2012; Duarte et al., 2015a,b), which is in line with the absence of changes in baseline HR.
Cardiac autonomic imbalance has been reported in Sprague–Dawley rats subjected to repeated sessions of social defeat (Wood et al., 2012; Sévoz-Couche et al., 2013). By analyzing HR variability, results indicated a shift in sympathovagal balance toward sympathetic predominance (Wood et al., 2012; Sévoz-Couche et al., 2013), which was likely mediated by both a decrease in parasympathetic activity and increase in sympathetic tone to the heart (Sévoz-Couche et al., 2013). This finding is in line with the increase in baseline HR observed in Sprague–Dawley rats following repeated exposure to social defeat (Sévoz-Couche et al., 2013). However, Sprague–Dawley rats seem to be selectively susceptible to resting tachycardia induced by repeated social defeat once studies did not identify changes in baseline HR in either mice (Bartolomucci et al., 2003; Costoli et al., 2004; Carnevali et al., 2012) or wide-type Groningen (Sgoifo et al., 2001; Carnevali et al., 2013b), Long-Evans (Tornatzky and Miczek, 1993), Wistar-Kyoto (Carnevali et al., 2015), Lister hooded (Chung et al., 1999), and Dahl-s (Adams and Blizard, 1986; Adams et al., 1987) rats. Nevertheless, differences in experimental protocol may also account for the differences in findings of autonomic/cardiovascular changes because exposures to social defeat in different studies ranged from 5 to 25 days (Table 2). Moreover, the defeated animal cohabited with its aggressor in some protocols, being subjected to intermittent episodes of aggressive interaction but with continuous sensorial contact (Bartolomucci et al., 2003; Costoli et al., 2004), whereas animals were exposed only to intermittent episodes of social defeat in other studies (Sgoifo et al., 2001; Carnevali et al., 2013b), including those that identified changes in HR (Wood et al., 2012; Sévoz-Couche et al., 2013).
The increase in sympathetic activity induced by chronic stressors seems not to be restricted to the heart. For instance, Grippo et al. (2008) reported that a 4-week CVS protocol increased lumbar sympathetic nerve activity. In addition, an increase in tyrosine hydroxylase expression and activity has been reported in the sympathetic ganglia and adrenal medulla following exposure to chronic stressors (Nankova et al., 1994, 1996; Bobrovskaya et al., 2013). The relevance of the response in the adrenal medulla is unclear since changes in plasma concentration of adrenaline and noradrenaline were not identified following exposure to several chronic stress protocols, including RRS, footshock, social isolation, crowding, and CVS (Konarska et al., 1989; Dronjak et al., 2004; Gavrilovic et al., 2005; Spasojevic et al., 2009). Therefore, sympathoexcitation induced by chronic stressors seems to be mediated by an increase in activity of sympathetic nerves rather than changes in the sympatho-adrenomedullary system.
Long-term potentiation was reported in the sympathetic ganglia of animals subjected to cage-switch stress for 4 weeks (Alkadhi et al., 2005). It is an activity-dependent sustained increase in ganglionic transmission, which is similar to that described in the brain. Therefore, this sustained enhancement of synaptic efficacy may constitute an important mechanism underlying the increase in sympathetic tone following long-term exposure to aversive stimuli (Alkadhi et al., 2005).
Changes in the baroreflex control of HR were reported following exposure to several chronic stressors, including CVS, RRS, repeated social defeat stress, and chronic social isolation (Conti et al., 2001; Porter et al., 2004; Daubert et al., 2012; Xie et al., 2012; Sévoz-Couche et al., 2013; Almeida et al., 2015; Duarte et al., 2015a; Cruz et al., 2016). However, the effects in the baroreflex activity seem to be dependent on the duration of chronic stress. For example, exposure to CVS protocols of either 10 (Duarte et al., 2015a) or 14 days (Xie et al., 2012; Almeida et al., 2015) induced changes in the baroreflex control of HR, but a 4-week protocol did not affect baroreflex HR responses (Grippo et al., 2008). Nevertheless, the reflex increase in lumbar sympathetic nerve activity induced by hypotension was decreased in animals following exposure to a CVS protocol for 4 weeks (Grippo et al., 2008), indicating that lumbar sympathetic nerve and cardiac autonomic innervation are affected differently by CVS. In addition, different effects of CVS in reflex bradycardia induced by increased blood pressure were obtained when baroreflex function was evaluated in animals anesthetized (facilitation; Xie et al., 2012) and unanesthetized rats (impairment; Almeida et al., 2015; Duarte et al., 2015a), indicating that anesthesia may affect the analysis of cardiovascular changes induced by chronic stressors.
Most studies describing stress effects evaluated the baroreflex activity using the classical pharmacological approach (i.e., arterial pressure changes were induced by intravenous infusion of vasoactive agents). However, analysis of the baroreflex responses over the physiological range of fluctuations in arterial pressure without any pharmacological manipulations (i.e., spontaneous baroreflex) has also provided evidence of an impact of chronic stressors, but the effects are stress-specific type. For example, changes in spontaneous baroreflex activity were reported in animals subjected to repeated exposure to social defeat (Sévoz-Couche et al., 2013) and CVS (Almeida et al., 2015), but not following RRS (Daubert et al., 2012; Duarte et al., 2015b). Therefore, an impact of RRS in baroreflex activity was evidenced by the classical pharmacological approach alone (Conti et al., 2001; Porter et al., 2004; Duarte et al., 2015a). Acute ablation of specific central nervous system regions has different effects on the baroreflex responses assessed by the classical pharmacological approach and the sequence analysis technique (Crestani et al., 2010a; de Andrade et al., 2014), indicating that differences in the neural circuitry of reflex responses within the narrow range of physiological variations and during more pronounced arterial pressure changes could explain the specific influence of RRS on the baroreflex responses over the full range of arterial pressure changes. Nevertheless, further studies are necessary to clarify this issue.
The studies that evaluated cardiovascular function in unanesthetized animals provided evidence that both CVS (Grippo et al., 2008; Almeida et al., 2015; Duarte et al., 2015a) and repeated social defeat stress (Sévoz-Couche et al., 2013) impaired the baroreflex function. Impairment of the baroreflex function is proposed to be involved in the physiopathology of hypertension (Grassi et al., 2006; Honzikova and Fiser, 2009), and is associated with overactivity of sympathetic activity (Grassi et al., 2004). Therefore, sympathoexcitation and mild hypertension (reported in some studies, Table 1) induced by these chronic stressors may be mediated by changes in the baroreflex activity. In contrast, an increase in baroreflex sensitivity was observed following exposure to RRS (Conti et al., 2001; Duarte et al., 2015a). Moreover, a recent study did not identify an impact of chronic social isolation in baroreflex function in adult rats (Cruz et al., 2016). Therefore, an involvement of baroreflex changes in the physiopathology of stress-induced cardiovascular complications is dependent on the chronic stress type.
Most studies that characterized the influence of predictability of stressor in its responses evaluated alterations in neuroendocrine function, behavioral responses, somatic parameters, and brain morphology/function. Indeed, a possible difference in the impact of predictable vs. unpredictable stressors in cardiovascular function and autonomic activity was addressed only recently (Duarte et al., 2015a,b). Therefore, before discussing the influence of predictability in cardiovascular/autonomic responses to stress, a summary of the impact of predictable vs. unpredictable stressors in the HPA axis, anxiety- and depression-like behaviors, and somatic parameters is presented in order to discuss experimental results in rodents that characterized the influence of predictability of stressor stimulus and its consequences.
The impact of predictability of stressor stimulus was initially examined by comparing responses to signaled (e.g., aversive stimulus preceded by light) vs. unsignaled footshock. Some of these studies indicated that signaling minimized stress reactions (e.g., corticosterone release) and stress-induced pathology (e.g., stomach ulceration; Perkins, 1955; Seligman, 1968; Weiss, 1970), which supported the hypothesis that a reliable predictor of an aversive stimulus minimizes its responses (Seligman, 1968). However, these results were not consistent (Brady et al., 1962; Paré, 1971; Bassett et al., 1973), and the definition of predictability as identical with the presence or absence of a signal preceding a regularly occurring aversive stimulus was criticized (Bassett et al., 1973). Indeed, the predictability of a stressor in terms of time has been proposed as an important dimension of its consequences. For example, repeated stress applied varying the interval between each exposure (i.e., irregular) induced greater and more persistent increase in plasma corticosterone, fatty acid, and glucose levels than regular exposure (Bassett et al., 1973; Quirce et al., 1981; Smith et al., 2013). Increase in anxiety- and depression-like behaviors and somatic changes such as adrenal hypertrophy and reduction in body weight gain were also rather observed following stressor applied irregularly than regularly (Martí and Armario, 1997; Smith et al., 2013), besides some of these effects may be related to stressor intensity (Martí and Armario, 1997). Studies have also used protocols that vary the duration of each stress session, but with constant interval between the sessions (Rockman et al., 1987; Ortiz et al., 2015). Nevertheless, a comparison of the responses induced by this stress paradigm with those induced by a stressor applied regularly has never been investigated.
Studies have also evaluated the predictability by varying the type of stressor. These studies have compared the impact of daily exposure to the same stressor type (i.e., homotypic) vs. the exposure to different aversive stimuli (i.e., heterotypic). Typically, these studies have compared the effects of the RRS applied in a predictable schedule vs. the CVS, which is a widely used paradigm that involves daily exposure of rodents to different stressors at unpredictable times (Willnér, 2005; Grippo, 2009; Frisbee et al., 2015). In this regard, the CVS has been demonstrated to induce more severe somatic changes such as adrenal hypertrophy and thymus involution (Magariños and McEwen, 1995; Zucchi et al., 2009; Flak et al., 2012; Kopp et al., 2013; Duarte et al., 2015a), which is possibly related to an increase in baseline HPA axis activity observed mainly following exposure to heterotypic stressors (Magariños and McEwen, 1995; Marin et al., 2007). Increase in anxiety- and depression-like behaviors was also more severe following exposure to CVS than to RRS (Haile et al., 2001; Pastor-Ciurana et al., 2014; Yoon et al., 2014; Zhu et al., 2014; Gao et al., 2016). Although some studies report that CVS induces increased reduction in body weight gain (Marin et al., 2007; Gao et al., 2016), several studies have demonstrated that homotypic and heterotypic stressors similarly affect this parameter (Magariños and McEwen, 1995; Vyas et al., 2002; Flak et al., 2012; Yoon et al., 2014; Duarte et al., 2015a).
The lesser impact of homotypic stressors in neuroendocrine, behavioral, and some somatic parameters is possibly related to a habituation process upon repeated exposure to the same stressor, which is reduced during exposure to heterotypic stressors (Herman, 2013). This habituation process is mainly evidenced by a progressive reduction in HPA axis activation (Grissom and Bhatnagar, 2009). Indeed, adaptation to chronic stress determined by habituation of responses upon repeated stress exposure has been proposed, which limits the long-term impact of stress (Grissom and Bhatnagar, 2009; Herman, 2013). In this regard, the habituation process has been demonstrated to be impaired when homotypic stressors are applied irregularly (De Boer et al., 1989; Martí and Armario, 1997; Smith et al., 2013), which is in line with evidence discussed above wherein stressors applied irregularly induce more severe responses.
Cardiovascular and autonomic changes have been reported following exposure to both predictable and unpredictable stressors. However, few studies to date have investigated the influence of predictability by directly comparing cardiovascular and autonomic changes induced by predictable vs. unpredictable stress protocols. De Boer et al. (1989) initially demonstrated that noise applied regularly, but not irregularly, increased plasma noradrenaline concentration, whereas noradrenaline response to noise was decreased in animals subjected to irregular protocol. Plasma adrenaline response to noise was reduced by both regular and irregular protocols (De Boer et al., 1989). These results do not support an influence of predictability in the sympatho-adrenomedullary response to stress. However, a possible influence of regular vs. irregular aversive stimuli on cardiovascular parameters, such as blood pressure and HR, has never been evaluated. Indeed, an impact of predictable vs. unpredictable stressors in cardiovascular function was addressed only recently. In this regard, Duarte et al. (2015a) compared the effect of the homotypic stressor RRS (predictable) vs. the heterotypic stressor CVS (unpredictable) on the baseline values of arterial pressure and HR, baroreflex function, and cardiac autonomic activity. They observed that both chronic stressors increased the baseline arterial pressure values (Duarte et al., 2015a). However, increase in baseline HR values and cardiac sympathetic activity as well as impaired baroreflex function was observed only in animals subjected to CVS (Duarte et al., 2015a). Although, these results provide evidence of a more severe impact of unpredictable vs. predictable stressors in cardiovascular function and autonomic activity, relevant cardiovascular changes were also detected following exposure to the predictable stressor RRS.
As stated above, adaptation to chronic stress determined by habituation of physiological responses upon repeated exposure to the same stressor has been proposed, which limits its long-term impact (Grissom and Bhatnagar, 2009; Herman, 2013). This idea has been supported mainly by consistent findings of habituation of HPA axis activation (Grissom and Bhatnagar, 2009). Table 3 summarizes the studies that compared cardiovascular and autonomic responses during an acute stress session and after repeated exposure to the stressor. We note that studies have not consistently demonstrated a habituation of cardiovascular responses upon repeated exposure to a stressor (Table 3). For example, some findings indicated a decrease in pressor and tachycardiac response upon repeated exposure to restraint stress (Chen and Herbert, 1995; Bechtold et al., 2009), but several other studies reported similar cardiovascular responses to this stressor during both acute and repeated exposures (McDougall et al., 2000, 2005; Conti et al., 2001; Daubert et al., 2012). Analysis of cardiovascular and autonomic responses during repeated exposure to social defeat has also identified inconsistent results. Indeed, some results evidenced habituation (Adams et al., 1987; Chung et al., 1999; Costoli et al., 2004), whereas several other studies reported similar responses during repeated exposure to social defeat (Adams and Blizard, 1986; Meehan et al., 1995; Sgoifo et al., 2001, 2002; Carnevali et al., 2012, 2013b). Regardless of inconsistency of the results, evidence that cardiovascular responses do not readily habituate indicates a reduced process of adaptation (Grissom and Bhatnagar, 2009; Herman, 2013), which supports the findings of Duarte et al. (2015a) and other researchers (see above sections) demonstrating significant cardiovascular dysfunctions following exposure to predictable stressors (e.g., RRS and repeated social defeat).
Table 3. Studies comparing arterial pressure, heart rate (HR), and autonomic responses during an acute session of stress and after repeated exposure to the stressor.
Reasons for the discrepancy in findings of habituation of cardiovascular responses are unclear, but may be the result of methodological differences. For example, the protocol of one of the studies that identified habituation included some stress-free days (i.e., animals were subjected to restraint stress 5–7 days/week for 21–25 days; Bechtold et al., 2009), which is different from the studies that did not identify habituation to restraint stress wherein animals were continuously subjected to stress. In this regard, stress-free periods between periods of repeated stress exposure has been proposed to facilitate the habituation process (Grissom and Bhatnagar, 2009). Differences between species and strains may also contribute. For example, more consistent evidence of habituation to social defeat was observed in mice (Bartolomucci et al., 2003; Costoli et al., 2004), whereas similar responses upon repeated exposure to social defeat was observed in rats (Adams and Blizard, 1986; Meehan et al., 1995; Sgoifo et al., 2001, 2002; Carnevali et al., 2013b). A study using rats reported habituation upon repeated exposure to social defeat (Chung et al., 1999), whereas another report in mice did not identify habituation (Carnevali et al., 2012). However, strains of mice and rats used in these studies were different from those wherein a habituation process was observed in mice rather than in rats (Table 3), indicating that the strain may also affect the habituation process. Nevertheless, further studies are necessary to better address the habituation of the cardiovascular responses upon repeated exposure to the same stressor.
A significant increase in research using animal models on the impact of emotional stress in cardiovascular function and autonomic activity has occurred in last years. These studies have provided evidence that the impact of stress on the cardiovascular function is determined by stressor stimulus characteristics, such as chronicity and predictability. Regarding chronicity, changes in cardiovascular function and autonomic activity are well-documented during acute stress sessions. These responses constitute important short-term adaptive mechanisms, maintaining homeostasis and ensuring survival. However, enduring autonomic imbalance and cardiovascular dysfunctions are provoked mainly by long-term exposure to stressful events (i.e., chronic stress). In this sense, studies have provided consistent results regarding the effect of chronic emotional stress in baseline HR values, autonomic activity, and baroreflex function. Nevertheless, the chronic stress paradigm seems to be an important factor that determines the pattern of changes in these parameters. A detailed analysis of the effects of chronic stress in arterial pressure reveals inconsistent results; hence, an animal model of stress-induced hypertension is still missing.
Analysis of neuroendocrine and behavioral responses to stress has clearly demonstrated an influence of predictability of stressor in its effects. Nevertheless, only a limited number of studies compared the cardiovascular and autonomic changes following exposure to predictable vs. unpredictable stressors. These studies have not demonstrated a clear influence of predictability in cardiovascular dysfunctions induced by chronic stress. However, a possible influence of predictability in stress-induced cardiovascular complications is only beginning to be investigated, and a number of issues need to be addressed before more conclusive analysis can be performed. For example, adaptation to chronic stress determined by habituation of physiological responses upon repeated exposure to the same stressor has been proposed (Grissom and Bhatnagar, 2009; Herman, 2013), which explains the more severe impact of homotypic vs. heterotypic stressors in HPA axis activity, behavioral responses, and somatic parameters. However, habituation of the cardiovascular and autonomic responses is still a matter of debate. Furthermore, evaluation of cardiovascular changes following exposure to homotypic stressors applied regularly vs. irregularly is a relevant unvalued aspect in determining the influence of predictability, because this approach compares responses with an aversive stimulus of similar intensity applied at predictable vs. unpredictable schedules. Therefore, further studies are necessary to elucidate the influence of predictability of stressor in its cardiovascular responses.
CC designed, drafted, and revised the manuscript; and prepared the figures and tables.
The author declares that the research was conducted in the absence of any commercial or financial relationships that could be construed as a potential conflict of interest.
The author wishes to acknowledge the financial support from FAPESP (grants # 2012/14376-0 and 2015/05922-9), CNPq (grant #456405/2014-3), and Programa de Apoio ao Desenvolvimento Científico da Faculdade de Ciências Farmacêuticas da UNESP – PADC. CC is a CNPq research fellow (process #305583/2015-8).
Adams, N., and Blizard, D. A. (1986). Effects of social defeat on acute cardiovascular response in salt-sensitive and salt-resistant rats. Behav. Neural Biol. 46, 325–336.
Adams, N., Lins, M. D., and Blizard, D. A. (1987). Contrasting effects of social stress and foot-shock on acute cardiovascular response in salt-sensitive rats. Behav. Neural Biol. 48, 368–381.
Alkadhi, K. A., Alzoubi, K. H., Aleisa, A. M., Tanner, F. L., and Nimer, A. S. (2005). Psychosocial stress-induced hypertension results from in vivo expression of long-term potentiation in rat sympathetic ganglia. Neurobiol. Dis. 20, 849–857. doi: 10.1016/j.nbd.2005.05.020
Almeida, J., Duarte, J. O., Oliveira, L. A., and Crestani, C. C. (2015). Effects of nitric oxide synthesis inhibitor or fluoxetine treatment on depression-like state and cardiovascular changes induced by chronic variable stress in rats. Stress 18, 462–474. doi: 10.3109/10253890.2015.1038993
Anderson, G. A., and Overton, J. M. (1994). Acute exercise and cardiovascular responses to stress in rats. Physiol. Behav. 56, 639–644.
Bartolomucci, A., Palanza, P., Costoli, T., Savani, E., Laviola, G., Parmigiani, S., et al. (2003). Chronic psychosocial stress persistently alters autonomic function and physical activity in mice. Physiol. Behav. 80, 57–67. doi: 10.1016/S0031-9384(03)00209-9
Bassett, J. R., Cairncross, K. D., and King, M. G. (1973). Parameters of novelty, shock predictability and response contigency in corticosterone release in the rat. Physiol. Behav. 10, 901–907.
Baudrie, V., Tulen, J. H., Blanc, J., and Elghozi, J. L. (1997). Autonomic components of the cardiovascular responses to an acoustic startle stimulus in rats. J. Auton. Pharmacol. 17, 303–309.
Bayramgurler, D., Karson, A., Yazir, Y., Celikyurt, I. K., Kurnaz, S., and Utkan, T. (2013). The effect of etanercept on aortic nitric oxide-dependent vasorelaxation in an unpredictable chronic, mild stress model of depression in rats. Eur. J. Pharmacol. 710, 67–72. doi: 10.1016/j.ejphar.2013.04.007
Bechtold, A. G., Patel, G., Hochhaus, G., and Scheuer, D. A. (2009). Chronic blockade of hindbrain glucocorticoid receptors reduces blood pressure responses to novel stress and attenuates adaptation to repeated stress. Am. J. Physiol. Regul. Integr. Comp. Physiol. 296, R1445–R1454. doi: 10.1152/ajpregu.00095.2008
Bernatova, I., and Csizmadiova, Z. (2006). Effect of chronic social stress on nitric oxide synthesis and vascular function in rats with family history of hypertension. Life Sci. 78, 1726–1732. doi: 10.1016/j.lfs.2005.08.005
Bernatova, I., Puzserova, A., and Dubovicky, M. (2010). Sex differences in social stress-induced pressor and behavioral responses in normotensive and prehypertensive rats. Gen. Physiol. Biophys. 29, 346–354. doi: 10.4149/gpb_2010_04_346
Bernatova, I., Puzserova, A., Navarova, J., Csizmadiova, Z., and Zeman, M. (2007). Crowding-induced alterations in vascular system of Wistar-Kyoto rats: role of nitric oxide. Physiol. Res. 56, 667–669.
Bernatowa, I., Csizmadiova, Z., Kopincova, J., and Puzserova, A. (2007). Vascular function and nitric oxide production in chronic social-stress-exposed rats with various family history of hypertension. J. Physiol. Pharmacol. 58, 487–501.
Blanc, J., Baudrie, V., Tulen, J., Ponchon, P., Gaudet, E., and Elghozi, J. L. (1997). Social isolation affects the pattern of cardiovascular responses to repetitive acoustic startle stimuli. Clin. Exp. Pharmacol. Physiol. 24, 40–45.
Blessing, W. W. (2003). Lower brainstem pathways regulating sympathetically mediated changes in cutaneous blood flow. Cell. Mol. Neurobiol. 23, 527–538. doi: 10.1023/A:1025020029037
Bobrovskaya, L., Beard, D., Bondarenko, E., Beig, M. I., Jobling, P., Walker, F. R., et al. (2013). Does exposure to chronic stress influence blood pressure in rats? Auton. Neurosci. 177, 217–223. doi: 10.1016/j.autneu.2013.05.001
Bouzinova, E. V., Moller-Nielsen, N., Boedtkjer, D. B., Broegger, T., Wiborg, O., Aalkjaer, C., et al. (2012). Chronic mild stress-induced depression-like symptoms in rats and abnormalities in catecholamine uptake in small arteries. Psychosom. Med. 74, 278–287. doi: 10.1097/PSY.0b013e31824c40a9
Brady, J. P., Thornton, D. R., and De Fisher, D. (1962). Deleterious effects of anxiety elicited by conditioned pre-aversive stimuli in the rat. Psychosom. Med. 24, 590–595.
Bruder-Nascimento, T., Campos, D. H., Cicogna, A. C., and Cordellini, S. (2015). Chronic stress improves NO− and Ca2+ flux-dependent vascular function: a pharmacological study. Arq. Bras. Cardiol. 104, 226–233. doi: 10.5935/abc.20140207
Bruder-Nascimento, T., Campos, D. H., Leopoldo, A. S., Lima-Leopoldo, A. P., Okoshi, K., Cordellini, S., et al. (2012). Chronic stress improves the myocardial function without altering L-type Ca+2 channel activity in rats. Arq. Bras. Cardiol. 99, 907–914. doi: 10.1590/S0066-782X2012005000082
Buchholz, R. A., Hubbard, J. W., Keeton, T. K., and Nathan, M. A. (1986). Cardiovascular and neuroendocrine responses to behavioral stress after central or peripheral barodenervation in rats. Brain Res. 365, 360–364.
Bundzikova-Osacka, J., Ghosal, S., Packard, B. A., Ulrich-Lai, Y. M., and Herman, J. P. (2015). Role of nucleus of the solitary tract noradrenergic neurons in post-stress cardiovascular and hormonal control in male rats. Stress 18, 221–232. doi: 10.3109/10253890.2015.1013531
Burke, S. L., and Head, G. A. (2009). Cardiac and renal baroreflex control during stress in conscious renovascular hypertensive rabbits: effect of rilmenidine. J. Hypertens. 27, 132–141. doi: 10.1097/HJH.0b013e328317a7a7
Carnevali, L., Bondarenko, E., Sgoifo, A., Walker, F. R., Head, G. A., Lukoshkova, E. V., et al. (2011). Metyrapone and fluoxetine suppress enduring behavioral but not cardiac effects of subchronic stress in rats. Am. J. Physiol. Regul. Integr. Comp. Physiol. 301, R1123–R1131. doi: 10.1152/ajpregu.00273.2011
Carnevali, L., Mastorci, F., Audero, E., Graiani, G., Rossi, S., Macchi, E., et al. (2012). Stress-induced susceptibility to sudden cardiac death in mice with altered serotonin homeostasis. PLoS ONE 7:e41184. doi: 10.1371/journal.pone.0041184
Carnevali, L., Trombini, M., Porta, A., Montano, N., de Boer, S. F., and Sgoifo, A. (2013a). Vagal withdrawal and susceptibility to cardiac arrhythmias in rats with high trait aggressiveness. PLoS ONE 8:e68316. doi: 10.1371/journal.pone.0068316
Carnevali, L., Trombini, M., Rossi, S., Graiani, G., Manghi, M., Koolhaas, J. M., et al. (2013b). Structural and electrical myocardial remodeling in a rodent model of depression. Psychosom. Med. 75, 42–51. doi: 10.1097/PSY.0b013e318276cb0d
Carnevali, L., Vacondio, F., Rossi, S., Callegari, S., Macchi, E., Spadoni, G., et al. (2015). Antidepressant-like activity and cardioprotective effects of fatty acid amide hydrolase inhibitor URB694 in socially stressed Wistar Kyoto rats. Eur. Neuropsychopharmacol. 25, 2157–2169. doi: 10.1016/j.euroneuro.2015.07.015
Carrive, P. (2002). Cardiovascular and behavioural components of conditioned fear to context after ganglionic and alpha-adrenergic blockade. Auton. Neurosci. 98, 90–93. doi: 10.1016/S1566-0702(02)00039-5
Carrive, P. (2006). Dual activation of cardiac sympathetic and parasympathetic components during conditioned fear to context in the rat. Clin. Exp. Pharmacol. Physiol. 33, 1251–1254. doi: 10.1111/j.1440-1681.2006.04519.x
Chandola, T., Britton, A., Brunner, E., Hemingway, H., Malik, M., Kumari, M., et al. (2008). Work stress and coronary heart disease: what are the mechanisms? Eur. Heart J. 29, 640–648. doi: 10.1093/eurheartj/ehm584
Chen, X., and Herbert, J. (1995). Regional changes in c-fos expression in the basal forebrain and brainstem during adaptation to repeated stress: correlations with cardiovascular, hypothermic and endocrine responses. Neuroscience 64, 675–685.
Chung, K. K., Martinez, M., and Herbert, J. (1999). Central serotonin depletion modulates the behavioural, endocrine and physiological responses to repeated social stress and subsequent c-fos expression in the brains of male rats. Neuroscience 92, 613–625.
Conti, L. H., Shannon, M. H., Murry, J. D., and Printz, M. P. (2001). Repeated restraint stress-induced increase in baroreceptor reflex sensitivity: role of corticotropin-releasing factor. Neuropeptides 35, 71–81. doi: 10.1054/npep.2001.0847
Coote, J. H., Hilton, S. M., and Perez-Gonzales, J. F. (1979). Inhibition of the baroreceptor reflex on stimulation in the brain stem defence centre. J. Physiol. 288, 549–560.
Costoli, T., Bartolomucci, A., Graiani, G., Stilli, D., Laviola, G., and Sgoifo, A. (2004). Effects of chronic psychosocial stress on cardiac autonomic responsiveness and myocardial structure in mice. Am. J. Physiol. Heart Circ. Physiol. 286, H2133–H2140. doi: 10.1152/ajpheart.00869.2003
Crestani, C. C., Alves, F. H., Busnardo, C., Resstel, L. B., and Corrêa, F. M. (2010a). N-methyl-D-aspartate glutamate receptors in the hypothalamic paraventricular nucleus modulate cardiac component of the baroreflex in unanesthetized rats. Neurosci. Res. 67, 317–326. doi: 10.1016/j.neures.2010.05.001
Crestani, C. C., Alves, F. H., Tavares, R. F., and Correa, F. M. (2009). Role of the bed nucleus of the stria terminalis in the cardiovascular responses to acute restraint stress in rats. Stress 12, 268–278. doi: 10.1080/10253890802331477
Crestani, C. C., Tavares, R. F., Alves, F. H., Resstel, L. B., and Correa, F. M. (2010b). Effect of acute restraint stress on the tachycardiac and bradycardiac responses of the baroreflex in rats. Stress 13, 61–72. doi: 10.3109/10253890902927950
Crestani, C. C. (2016). Adolescent vulnerability to cardiovascular consequences of chronic emotional stress: review and perspectives for future research. Neurosci. Biobehav. Rev. doi: 10.1016/j.neubiorev.2016.03.027. [Epub ahead of print].
Cruz, F. C., Duarte, J. O., Leão, R. M., Hummel, L. F., Planeta, C. S., and Crestani, C. C. (2016). Adolescent vulnerability to cardiovascular consequences of chronic social stress: immediate and long-term effects of social isolation during adolescence. Dev. Neurobiol. 76, 34–46. doi: 10.1002/dneu.22297
Cudnoch-Jedrzejewska, A., Czarzasta, K., Puchalska, L., Dobruch, J., Borowik, O., Pachucki, J., et al. (2014). Angiotensin converting enzyme inhibition reduces cardiovascular responses to acute stress in myocardially infarcted and chronically stressed rats. Biomed Res. Int. 2014:385082. doi: 10.1155/2014/385082
Cudnoch-Jedrzejewska, A., Szczepanska-Sadowska, E., Dobruch, J., Gomolka, R., and Puchalska, L. (2010). Brain vasopressin V(1) receptors contribute to enhanced cardiovascular responses to acute stress in chronically stressed rats and rats with myocardial infarcton. Am. J. Physiol. Regul. Integr. Comp. Physiol. 298, R672–R680. doi: 10.1152/ajpregu.00543.2009
Danese, A., and McEwen, B. S. (2012). Adverse childhood experiences, allostasis, allostatic load, and age-related disease. Physiol. Behav. 106, 29–39. doi: 10.1016/j.physbeh.2011.08.019
D'Angelo, G., Mintz, J. D., Tidwell, J. E., Schreihofer, A. M., Pollock, D. M., and Stepp, D. W. (2006). Exaggerated cardiovascular stress responses and impaired beta-adrenergic-mediated pressor recovery in obese Zucker rats. Hypertension 48, 1109–1115. doi: 10.1161/01.HYP.0000247306.53547.d4
Daubert, D. L., McCowan, M., Erdos, B., and Scheuer, D. A. (2012). Nucleus of the solitary tract catecholaminergic neurons modulate the cardiovascular response to psychological stress in rats. J. Physiol. 590(Pt 19), 4881–4895. doi: 10.1113/jphysiol.2012.232314
de Andrade, O., Borghi, S. M., de Souza, H. C., Fontes, M. A., and Martins-Pinge, M. C. (2014). Paraventricular nucleus of hypothalamus participates in the sympathetic modulation and spontaneous fluctuation of baroreflex during head up tilt in unanesthetized rats. Neurosci. Lett. 558, 1–7. doi: 10.1016/j.neulet.2013.09.039
De Boer, S. F., Van der Gugten, J., and Slangen, J. L. (1989). Plasma catecholamine and corticosterone responses to predictable and unpredictable noise stress in rats. Physiol. Behav. 45, 789–795.
Demirtas, T., Utkan, T., Karson, A., Yazir, Y., Bayramgurler, D., and Gacar, N. (2014). The link between unpredictable chronic mild stress model for depression and vascular inflammation? Inflammation 37, 1432–1438. doi: 10.1007/s10753-014-9867-4
Dos Reis, D. G., Fortaleza, E. A., Tavares, R. F., and Corrêa, F. M. (2014). Role of the autonomic nervous system and baroreflex in stress-evoked cardiovascular responses in rats. Stress 17, 362–372. doi: 10.3109/10253890.2014.930429
Dronjak, S., Gavrilovic, L., Filipovic, D., and Radojcic, M. B. (2004). Immobilization and cold stress affect sympatho-adrenomedullary system and pituitary-adrenocortical axis of rats exposed to long-term isolation and crowding. Physiol. Behav. 81, 409–415. doi: 10.1016/j.physbeh.2004.01.011
Duarte, J. O., Cruz, F. C., Leao, R. M., Planeta, C. S., and Crestani, C. C. (2015a). Stress vulnerability during adolescence: comparison of chronic stressors in adolescent and adult rats. Psychosom. Med. 77, 186–199. doi: 10.1097/PSY.0000000000000141
Duarte, J. O., Planeta, C. S., and Crestani, C. C. (2015b). Immediate and long-term effects of psychological stress during adolescence in cardiovascular function: comparison of homotypic vs heterotypic stress regimens. Int. J. Dev. Neurosci. 40, 52–59. doi: 10.1016/j.ijdevneu.2014.11.004
Flak, J. N., Jankord, R., Solomon, M. B., Krause, E. G., and Herman, J. P. (2011). Opposing effects of chronic stress and weight restriction on cardiovascular, neuroendocrine and metabolic function. Physiol. Behav. 104, 228–234. doi: 10.1016/j.physbeh.2011.03.002
Flak, J. N., Solomon, M. B., Jankord, R., Krause, E. G., and Herman, J. P. (2012). Identification of chronic stress-activated regions reveals a potential recruited circuit in rat brain. Eur. J. Neurosci. 36, 2547–2555. doi: 10.1111/j.1460-9568.2012.08161.x
Ford, D. E., Mead, L. A., Chang, P. P., Cooper-Patrick, L., Wang, N. Y., and Klag, M. J. (1998). Depression is a risk factor for coronary artery disease in men: the precursors study. Arch. Intern. Med. 158, 1422–1426.
Friedman, M., and Rosenman, R. H. (1959). Association of specific overt behavior pattern with blood and cardiovascular findings; blood cholesterol level, blood clotting time, incidence of arcus senilis, and clinical coronary artery disease. J. Am. Med. Assoc. 169, 1286–1296.
Frisbee, J. C., Brooks, S. D., Stanley, S. C., and d'Audiffret, A. C. (2015). An unpredictable chronic mild stress protocol for instigating depressive symptoms, behavioral changes and negative health outcomes in rodents. J. Vis. Exp. doi: 10.3791/53109. [Epub ahead of print].
Gao, Y., Chen, F., Kong, Q. Q., Ning, S. F., Yuan, H. J., Lian, H. Y., et al. (2016). Stresses on female mice impair oocyte developmental potential: effects of stress severity and duration on oocytes at the growing follicle stage. Reprod. Sci. doi: 10.1177/1933719116630416. [Epub ahead of print].
Gardiner, S. M., and Bennett, T. (1983). The cardiovascular and renal responses to short-term isolation in Brattleboro rats. Clin. Sci. 64, 377–382.
Gavrilovic, L., Spasojevic, N., and Dronjak, S. (2005). Novel stressors affected catecholamine stores in socially isolated normotensive and spontaneously hypertensive rats. Auton. Neurosci. 122, 38–44. doi: 10.1016/j.autneu.2005.07.010
Gelsema, A. J., Schoemaker, R. G., Ruzicka, M., and Copeland, N. E. (1994). Cardiovascular effects of social stress in borderline hypertensive rats. J. Hypertens. 12, 1019–1028.
Goodson, M. L., Packard, A. E., Buesing, D. R., Maney, M., Myers, B., Fang, Y., et al. (2016). Chronic stress and rosiglitazone increase indices of vascular stiffness in male rats. Physiol. Behav. doi: 10.1016/j.physbeh.2016.03.031. [Epub ahead of print].
Grassi, G., Seravalle, G., Dell'Oro, R., Facchini, A., Ilardo, V., and Mancia, G. (2004). Sympathetic and baroreflex function in hypertensive or heart failure patients with ventricular arrhythmias. J. Hypertens. 22, 1747–1753. doi: 10.1097/00004872-200409000-00019
Grassi, G., Trevano, F. Q., Seravalle, G., Scopelliti, F., and Mancia, G. (2006). Baroreflex function in hypertension: consequences for antihypertensive therapy. Prog. Cardiovasc. Dis. 48, 407–415. doi: 10.1016/j.pcad.2006.03.002
Grippo, A. J., Beltz, T. G., and Johnson, A. K. (2003). Behavioral and cardiovascular changes in the chronic mild stress model of depression. Physiol. Behav. 78, 703–710. doi: 10.1016/S0031-9384(03)00050-7
Grippo, A. J., Beltz, T. G., Weiss, R. M., and Johnson, A. K. (2006). The effects of chronic fluoxetine treatment on chronic mild stress-induced cardiovascular changes and anhedonia. Biol. Psychiatry 59, 309–316. doi: 10.1016/j.biopsych.2005.07.010
Grippo, A. J., Carter, C. S., McNeal, N., Chandler, D. L., Larocca, M. A., Bates, S. L., et al. (2011). 24-hour autonomic dysfunction and depressive behaviors in an animal model of social isolation: implications for the study of depression and cardiovascular disease. Psychosom. Med. 73, 59–66. doi: 10.1097/PSY.0b013e31820019e4
Grippo, A. J., and Johnson, A. K. (2009). Stress, depression and cardiovascular dysregulation: a review of neurobiological mechanisms and the integration of research from preclinical disease models. Stress 12, 1–21. doi: 10.1080/10253890802046281
Grippo, A. J., Lamb, D. G., Carter, C. S., and Porges, S. W. (2007). Social isolation disrupts autonomic regulation of the heart and influences negative affective behaviors. Biol. Psychiatry 62, 1162–1170. doi: 10.1016/j.biopsych.2007.04.011
Grippo, A. J., Moffitt, J. A., and Johnson, A. K. (2002). Cardiovascular alterations and autonomic imbalance in an experimental model of depression. Am. J. Physiol. Regul. Integr. Comp. Physiol. 282, R1333–R1341. doi: 10.1152/ajpregu.00614.2001
Grippo, A. J., Moffitt, J. A., and Johnson, A. K. (2008). Evaluation of baroreceptor reflex function in the chronic mild stress rodent model of depression. Psychosom. Med. 70, 435–443. doi: 10.1097/PSY.0b013e31816ff7dd
Grippo, A. J., Moffitt, J. A., Sgoifo, A., Jepson, A. J., Bates, S. L., Chandler, D. L., et al. (2012a). The integration of depressive behaviors and cardiac dysfunction during an operational measure of depression: investigating the role of negative social experiences in an animal model. Psychosom. Med. 74, 612–619. doi: 10.1097/PSY.0b013e31825ca8e5
Grippo, A. J., Pournajafi-Nazarloo, H., Sanzenbacher, L., Trahanas, D. M., McNeal, N., Clarke, D. A., et al. (2012b). Peripheral oxytocin administration buffers autonomic but not behavioral responses to environmental stressors in isolated prairie voles. Stress 15, 149–161. doi: 10.3109/10253890.2011.605486
Grippo, A. J., Santos, C. M., Johnson, R. F., Beltz, T. G., Martins, J. B., Felder, R. B., et al. (2004). Increased susceptibility to ventricular arrhythmias in a rodent model of experimental depression. Am. J. Physiol. Heart Circ. Physiol. 286, H619–H626. doi: 10.1152/ajpheart.00450.2003
Grippo, A. J., Sgoifo, A., Mastorci, F., McNeal, N., and Trahanas, D. M. (2010). Cardiac dysfunction and hypothalamic activation during a social crowding stressor in prairie voles. Auton. Neurosci. 156, 44–50. doi: 10.1016/j.autneu.2010.03.003
Grippo, A. J., Trahanas, D. M., Zimmerman, R. R. II, Porges, S. W., and Carter, C. S. (2009). Oxytocin protects against negative behavioral and autonomic consequences of long-term social isolation. Psychoneuroendocrinology 34, 1542–1553. doi: 10.1016/j.psyneuen.2009.05.017
Grippo, A. J. (2009). Mechanisms underlying altered mood and cardiovascular dysfunction: the value of neurobiological and behavioral research with animal models. Neurosci. Biobehav. Rev. 33, 171–180. doi: 10.1016/j.neubiorev.2008.07.004
Grissom, N., and Bhatnagar, S. (2009). Habituation to repeated stress: get used to it. Neurobiol. Learn. Mem. 92, 215–224. doi: 10.1016/j.nlm.2008.07.001
Grundt, A., Grundt, C., Gorbey, S., Thomas, M. A., and Lemmer, B. (2009). Strain-dependent differences of restraint stress-induced hypertension in WKY and SHR. Physiol. Behav. 97, 341–346. doi: 10.1016/j.physbeh.2009.02.029
Habib, M., Shaker, S., El-Gayar, N., and Aboul-Fotouh, S. (2015). The effects of antidepressants “fluoxetine and imipramine” on vascular abnormalities and Toll like receptor-4 expression in diabetic and non-diabetic rats exposed to chronic stress. PLoS ONE 10:e0120559. doi: 10.1371/journal.pone.0120559
Haile, C. N., GrandPre, T., and Kosten, T. A. (2001). Chronic unpredictable stress, but not chronic predictable stress, enhances the sensitivity to the behavioral effects of cocaine in rats. Psychopharmacology 154, 213–220. doi: 10.1007/s002130000650
Hatton, D. C., Brooks, V., Qi, Y., and McCarron, D. A. (1997). Cardiovascular response to stress: baroreflex resetting and hemodynamics. Am. J. Physiol. 272(5 Pt 2), R1588–R1594.
Herman, J. P. (2013). Neural control of chronic stress adaptation. Front. Behav. Neurosci. 7:61. doi: 10.3389/fnbeh.2013.00061
Hilton, S. M. (1963). Inhibition of baroreceptor reflexes on hypothalamic stimulation. J. Physiol. 165, 56–57.
Honzíková, N., and Fiser, B. (2009). Baroreflex sensitivity and essential hypertension in adolescents. Physiol. Res. 58, 605–612.
Hubbard, J. W., Cox, R. H., Sanders, B. J., and Lawler, J. E. (1986). Changes in cardiac output and vascular resistance during behavioral stress in the rat. Am. J. Physiol. 251(1 Pt 2), R82–R90.
Igosheva, N., Taylor, P. D., Poston, L., and Glover, V. (2007). Prenatal stress in the rat results in increased blood pressure responsiveness to stress and enhanced arterial reactivity to neuropeptide Y in adulthood. J. Physiol. 582(Pt 2), 665–674. doi: 10.1113/jphysiol.2007.130252
Inoue, N. (2014). Stress and atherosclerotic cardiovascular disease. J. Atheroscler. Thromb. 21, 391–401. doi: 10.5551/jat.21709
Iriuchijima, J., Kawaue, Y., and Teranishi, Y. (1982). Blood flow redistribution in the transposition response of the rat. Jpn. J. Physiol. 32, 807–816.
Ismail, B., Aboul-Fotouh, S., Mansour, A. A., Shehata, H. H., Salman, M. I., Ibrahim, E. A., et al. (2014). Behavioural, metabolic, and endothelial effects of the TNF-alpha suppressor thalidomide on rats subjected to chronic mild stress and fed an atherogenic diet. Can. J. Physiol. Pharmacol. 92, 375–385. doi: 10.1139/cjpp-2013-0446
Iwata, J., and LeDoux, J. E. (1988). Dissociation of associative and nonassociative concomitants of classical fear conditioning in the freely behaving rat. Behav. Neurosci. 102, 66–76.
Jarczok, M. N., Jarczok, M., Mauss, D., Koenig, J., Li, J., Herr, R. M., et al. (2013). Autonomic nervous system activity and workplace stressors–a systematic review. Neurosci. Biobehav. Rev. 37, 1810–1823. doi: 10.1016/j.neubiorev.2013.07.004
Kanbar, R., Orea, V., Barres, C., and Julien, C. (2007). Baroreflex control of renal sympathetic nerve activity during air-jet stress in rats. Am. J. Physiol. Regul. Integr. Comp. Physiol. 292, R362–R367. doi: 10.1152/ajpregu.00413.2006
Kapusta, D. R., Knardahl, S., Koepke, J. P., Johnson, A. K., and DiBona, G. F. (1989). Selective central alpha-2 adrenoceptor control of regional haemodynamic responses to air jet stress in conscious spontaneously hypertensive rats. J. Hypertens. 7, 189–194.
Kawachi, I., Sparrow, D., Vokonas, P. S., and Weiss, S. T. (1994). Symptoms of anxiety and risk of coronary heart disease. The normative aging study. Circulation 90, 2225–2229.
Kirby, R. F., Callahan, M. F., and Johnson, A. K. (1987). Regional vascular responses to an acute stressor in spontaneously hypertensive and Wistar-Kyoto rats. J. Auton. Nerv. Syst. 20, 185–188.
Kivimäki, M., Head, J., Ferrie, J. E., Brunner, E., Marmot, M. G., Vahtera, J., et al. (2006a). Why is evidence on job strain and coronary heart disease mixed? An illustration of measurement challenges in the Whitehall II study. Psychosom. Med. 68, 398–401. doi: 10.1097/01.psy.0000221252.84351.e2
Kivimaki, M., Virtanen, M., Elovainio, M., Kouvonen, A., Vaananen, A., and Vahtera, J. (2006b). Work stress in the etiology of coronary heart disease–a meta-analysis. Scand. J. Work Environ. Health 32, 431–442. doi: 10.5271/sjweh.1049
Knardahl, S., and Hendley, E. D. (1990). Association between cardiovascular reactivity to stress and hypertension or behavior. Am. J. Physiol. 259(1 Pt 2), H248–H257.
Konarska, M., Stewart, R. E., and McCarty, R. (1989). Sensitization of sympathetic-adrenal medullary responses to a novel stressor in chronically stressed laboratory rats. Physiol. Behav. 46, 129–135.
Kopp, B. L., Wick, D., and Herman, J. P. (2013). Differential effects of homotypic vs. heterotypic chronic stress regimens on microglial activation in the prefrontal cortex. Physiol. Behav. 122, 246–252. doi: 10.1016/j.physbeh.2013.05.030
Krause, E. G., de Kloet, A. D., Flak, J. N., Smeltzer, M. D., Solomon, M. B., Evanson, N. K., et al. (2011). Hydration state controls stress responsiveness and social behavior. J. Neurosci. 31, 5470–5476. doi: 10.1523/JNEUROSCI.6078-10.2011
Lagraauw, H. M., Kuiper, J., and Bot, I. (2015). Acute and chronic psychological stress as risk factors for cardiovascular disease: insights gained from epidemiological, clinical and experimental studies. Brain Behav. Immun. 50, 18–30. doi: 10.1016/j.bbi.2015.08.007
Ledvenyiova-Farkasova, V., Bernatova, I., Balis, P., Puzserova, A., Bartekova, M., Gablovsky, I., et al. (2015). Effect of crowding stress on tolerance to ischemia-reperfusion injury in young male and female hypertensive rats: molecular mechanisms. Can. J. Physiol. Pharmacol. 93, 793–802. doi: 10.1139/cjpp-2015-0026
Liang, J., Yuan, X., Shi, S., Wang, F., Chen, Y., Qu, C., et al. (2015). Effect and mechanism of fluoxetine on electrophysiology in vivo in a rat model of postmyocardial infarction depression. Drug Des. Devel. Ther. 9, 763–772. doi: 10.2147/DDDT.S75863
Lown, B., Verrier, R., and Corbalan, R. (1973). Psychologic stress and threshold for repetitive ventricular response. Science 182, 834–836.
Lu, X. T., Liu, Y. F., Zhang, L., Yang, R. X., Liu, X. Q., Yan, F. F., et al. (2012). Unpredictable chronic mild stress promotes atherosclerosis in high cholesterol-fed rabbits. Psychosom. Med. 74, 604–611. doi: 10.1097/PSY.0b013e31825d0b71
Magariños, A. M., and McEwen, B. S. (1995). Stress-induced atrophy of apical dendrites of hippocampal CA3c neurons: comparison of stressors. Neuroscience 69, 83–88.
Marin, M. T., Cruz, F. C., and Planeta, C. S. (2007). Chronic restraint or variable stresses differently affect the behavior, corticosterone secretion and body weight in rats. Physiol. Behav. 90, 29–35. doi: 10.1016/j.physbeh.2006.08.021
Markovitz, J. H., Matthews, K. A., Whooley, M., Lewis, C. E., and Greenlund, K. J. (2004). Increases in job strain are associated with incident hypertension in the CARDIA Study. Ann. Behav. Med. 28, 4–9. doi: 10.1207/s15324796abm2801_2
Marmot, M. G., Smith, G. D., Stansfeld, S., Patel, C., North, F., Head, J., et al. (1991). Health inequalities among British civil servants: the Whitehall II study. Lancet 337, 1387–1393.
Martí, O., and Armario, A. (1997). Influence of regularity of exposure to chronic stress on the pattern of habituation of pituitary-adrenal hormones, prolactin and glucose. Stress 1, 179–189.
Martin, D. S., Appelt, C., Rodrigo, M. C., and Egland, M. C. (1996). Acute stress increases venomotor tone in conscious rats. Am. J. Physiol. 271(4 Pt 2), H1375–H1383.
Maslova, L. N., Bulygina, V. V., and Amstislavskaya, T. G. (2010). Prolonged social isolation and social instability in adolescence in rats: immediate and long-term physiological and behavioral effects. Neurosci. Behav. Physiol. 40, 955–963. doi: 10.1007/s11055-010-9352-y
Matchkov, V. V., Kravtsova, V. V., Wiborg, O., Aalkjaer, C., and Bouzinova, E. V. (2015). Chronic selective serotonin reuptake inhibition modulates endothelial dysfunction and oxidative state in rat chronic mild stress model of depression. Am. J. Physiol. Regul. Integr. Comp. Physiol. 309, R814–R823. doi: 10.1152/ajpregu.00337.2014
Matsuura, N., Nagasawa, K., Minagawa, Y., Ito, S., Sano, Y., Yamada, Y., et al. (2015). Restraint stress exacerbates cardiac and adipose tissue pathology via beta-adrenergic signaling in rats with metabolic syndrome. Am. J. Physiol. Heart Circ. Physiol. 308, H1275–H1286. doi: 10.1152/ajpheart.00906.2014
McDougall, S. J., Lawrence, A. J., and Widdop, R. E. (2005). Differential cardiovascular responses to stressors in hypertensive and normotensive rats. Exp. Physiol. 90, 141–150. doi: 10.1113/expphysiol.2004.028308
McDougall, S. J., Paull, J. R., Widdop, R. E., and Lawrence, A. J. (2000). Restraint stress: differential cardiovascular responses in Wistar-Kyoto and spontaneously hypertensive rats. Hypertension 35(1 Pt 1), 126–129. doi: 10.1161/01.HYP.35.1.126
McEwen, B. S., and Stellar, E. (1993). Stress and the individual. Mechanisms leading to disease. Arch. Intern. Med. 153, 2093–2101.
McEwen, B. S. (1998). Protective and damaging effects of stress mediators. N. Engl. J. Med. 338, 171–179. doi: 10.1056/NEJM199801153380307
McNeal, N., Scotti, M. A., Wardwell, J., Chandler, D. L., Bates, S. L., Larocca, M., et al. (2014). Disruption of social bonds induces behavioral and physiological dysregulation in male and female prairie voles. Auton. Neurosci. 180, 9–16. doi: 10.1016/j.autneu.2013.10.001
Meehan, W. P., Tornatzky, W., and Miczek, K. A. (1995). Blood pressure via telemetry during social confrontations in rats: effects of clonidine. Physiol. Behav. 58, 81–88.
Mercanoglu, G., Safran, N., Uzun, H., and Eroglu, L. (2008). Chronic emotional stress exposure increases infarct size in rats: the role of oxidative and nitrosative damage in response to sympathetic hyperactivity. Methods Find. Exp. Clin. Pharmacol. 30, 745–752. doi: 10.1358/mf.2008.30.10.1316822
Michelini, L. C. (1994). Vasopressin in the nucleus tractus solitarius: a modulator of baroreceptor reflex control of heart rate. Braz. J. Med. Biol. Res. 27, 1017–1032.
Miki, K., and Yoshimoto, M. (2013). Sympathetic nerve activity during sleep, exercise, and mental stress. Auton. Neurosci. 174, 15–20. doi: 10.1016/j.autneu.2012.12.007
Mohammed, M., Ootsuka, Y., and Blessing, W. (2014). Brown adipose tissue thermogenesis contributes to emotional hyperthermia in a resident rat suddenly confronted with an intruder rat. Am. J. Physiol. Regul. Integr. Comp. Physiol. 306, R394–R400. doi: 10.1152/ajpregu.00475.2013
Mostofsky, E., Penner, E. A., and Mittleman, M. A. (2014). Outbursts of anger as a trigger of acute cardiovascular events: a systematic review and meta-analysis. Eur. Heart J. 35, 1404–1410. doi: 10.1093/eurheartj/ehu033
Nagaraja, H. S., and Jeganathan, P. S. (1999). Influence of different types of stress on selected cardiovascular parameters in rats. Indian J. Physiol. Pharmacol. 43, 296–304.
Nalivaiko, E. (2011). Animal models of psychogenic cardiovascular disorders: what we can learn from them and what we cannot. Clin. Exp. Pharmacol. Physiol. 38, 115–125. doi: 10.1111/j.1440-1681.2010.05465.x
Nankova, B., Kvetnanské, R., Hiremagalur, B., Sabban, B., Rusnak, M., and Sabban, E. L. (1996). Immobilization stress elevates gene expression for catecholamine biosynthetic enzymes and some neuropeptides in rat sympathetic ganglia: effects of adrenocorticotropin and glucocorticoids. Endocrinology 137, 5597–5604. doi: 10.1210/endo.137.12.8940389
Nankova, B., Kvetnansky, R., McMahon, A., Viskupic, E., Hiremagalur, B., Frankle, G., et al. (1994). Induction of tyrosine hydroxylase gene expression by a nonneuronal nonpituitary-mediated mechanism in immobilization stress. Proc. Natl. Acad. Sci. U.S.A. 91, 5937–5941.
Natelson, B. H., and Cagin, N. A. (1979). Stress-induced ventricular arrhythmias. Psychosom. Med. 41, 259–262.
Nijsen, M. J., Croiset, G., Diamant, M., Stam, R., Delsing, D., de Wied, D., et al. (1998). Conditioned fear-induced tachycardia in the rat: vagal involvement. Eur. J. Pharmacol. 350, 211–222.
Norman, R. A. Jr., Coleman, T. G., and Dent, A. C. (1981). Continuous monitoring of arterial pressure indicates sinoaortic denervated rats are not hypertensive. Hypertension 3, 119–125.
Ortiz, J. B., Taylor, S. B., Hoffman, A. N., Campbell, A. N., Lucas, L. R., and Conrad, C. D. (2015). Sex-specific impairment and recovery of spatial learning following the end of chronic unpredictable restraint stress: potential relevance of limbic GAD. Behav. Brain Res. 282, 176–184. doi: 10.1016/j.bbr.2014.12.051
Paré, W. P. (1971). Six-hour escape-avoidance work shift and production of stomach ulcers. J. Comp. Physiol. Psychol. 74, 459–466.
Pastor-Ciurana, J., Rabasa, C., Ortega-Sánchez, J. A., Sanchis-Ollé, M., Gabriel-Salazar, M., Ginesta, M., et al. (2014). Prior exposure to repeated immobilization or chronic unpredictable stress protects from some negative sequels of an acute immobilization. Behav. Brain Res. 265, 155–162. doi: 10.1016/j.bbr.2014.02.028
Paton, J. F., Boscan, P., Pickering, A. E., and Nalivaiko, E. (2005). The yin and yang of cardiac autonomic control: vago-sympathetic interactions revisited. Brain Res. Brain Res. Rev. 49, 555–565. doi: 10.1016/j.brainresrev.2005.02.005
Perkins, C. C. Jr. (1955). The stimulus conditions which follow learned responses. Psychol. Rev. 62, 341–348.
Porter, J. P., King, S. H., and Honeycutt, A. D. (2007). Prenatal high-salt diet in the Sprague-Dawley rat programs blood pressure and heart rate hyperresponsiveness to stress in adult female offspring. Am. J. Physiol. Regul. Integr. Comp. Physiol. 293, R334–R342. doi: 10.1152/ajpregu.00887.2006
Porter, J. P., Phillips, A., Rich, J., and Wright, D. (2004). Effect of chronic stress on the cardiac baroreflex in the post-weanling rat. Life Sci. 75, 1595–1607. doi: 10.1016/j.lfs.2004.03.018
Puzserova, A., and Bernatova, I. (2010). Chronic social stress increases nitric oxide-dependent vasorelaxation in normotensive rats. Interdiscip. Toxicol. 3, 109–117. doi: 10.2478/v10102-010-0049-4
Puzserova, A., Slezak, P., Balis, P., and Bernatova, I. (2013). Long-term social stress induces nitric oxide-independent endothelial dysfunction in normotensive rats. Stress 16, 331–339. doi: 10.3109/10253890.2012.725116
Puzserova, A., Torok, J., Sotnikova, R., Zemancikova, A., and Bernatova, I. (2012). Reactivity of the mesenteric bed arteries of normotensive rats exposed to chronic social stress. Gen. Physiol. Biophys. 31, 279–290. doi: 10.4149/gpb_2012_032
Quirce, C. M., Odio, M., and Solano, J. M. (1981). The effects of predictable and unpredictable schedules of physical restraint upon rats. Life Sci. 28, 1897–1902.
Rakhshan, K., Imani, A., Faghihi, M., Nabavizadeh, F., Golnazari, M., and Karimian, S. (2015). Evaluation of Chronic physical and psychological stress induction on cardiac ischemia / reperfusion injuries in isolated male rat heart: the role of sympathetic nervous system. Acta Med. Iran. 53, 482–490.
Ravingerova, T., Bernatova, I., Matejikova, J., Ledvenyiova, V., Nemcekova, M., Pechanova, O., et al. (2011). Impaired cardiac ischemic tolerance in spontaneously hypertensive rats is attenuated by adaptation to chronic and acute stress. Exp. Clin. Cardiol. 16, e23–e29.
Rockman, G. E., Hall, A., Hong, J., and Glavin, G. B. (1987). Unpredictable cold-immobilization stress effects on voluntary ethanol consumption in rats. Life Sci. 40, 1245–1251.
Roest, A. M., Martens, E. J., de Jonge, P., and Denollet, J. (2010). Anxiety and risk of incident coronary heart disease: a meta-analysis. J. Am. Coll. Cardiol. 56, 38–46. doi: 10.1016/j.jacc.2010.03.034
Rosengren, A., Hawken, S., Ounpuu, S., Sliwa, K., Zubaid, M., Almahmeed, W. A., et al. (2004). Association of psychosocial risk factors with risk of acute myocardial infarction in 11119 cases and 13648 controls from 52 countries (the INTERHEART study): case-control study. Lancet 364, 953–962. doi: 10.1016/S0140-6736(04)17019-0
Rosenman, R. H., Brand, R. J., Jenkins, D., Friedman, M., Straus, R., and Wurm, M. (1975). Coronary heart disease in Western Collaborative Group Study. Final follow-up experience of 8 1/2 years. JAMA 233, 872–877.
Roth, L., Rombouts, M., Schrijvers, D. M., Lemmens, K., De Keulenaer, G. W., Martinet, W., et al. (2015). Chronic intermittent mental stress promotes atherosclerotic plaque vulnerability, myocardial infarction and sudden death in mice. Atherosclerosis 242, 288–294. doi: 10.1016/j.atherosclerosis.2015.07.025
Rozanski, A., Blumenthal, J. A., and Kaplan, J. (1999). Impact of psychological factors on the pathogenesis of cardiovascular disease and implications for therapy. Circulation 99, 2192–2217.
Rugulies, R. (2002). Depression as a predictor for coronary heart disease. a review and meta-analysis. Am. J. Prev. Med. 23, 51–61. doi: 10.1016/S0749-3797(02)00439-7
Schadt, J. C., and Hasser, E. M. (1998). Hemodynamic effects of acute stressors in the conscious rabbit. Am. J. Physiol. 274(3 Pt 2), R814–R821.
Scheuer, D. A., and Mifflin, S. W. (1998). Repeated intermittent stress exacerbates myocardial ischemia-reperfusion injury. Am. J. Physiol. 274(2 Pt 2), R470–R475.
Seligman, M. E. (1968). Chronic fear produced by unpredictable electric shock. J. Comp. Physiol. Psychol. 66, 402–411.
Sévoz-Couche, C., Brouillard, C., Camus, F., Laude, D., De Boer, S. F., Becker, C., et al. (2013). Involvement of the dorsomedial hypothalamus and the nucleus tractus solitarii in chronic cardiovascular changes associated with anxiety in rats. J. Physiol. 591(Pt 7), 1871–1887. doi: 10.1113/jphysiol.2012.247791
Sgoifo, A., Carnevali, L., and Grippo, A. J. (2014). The socially stressed heart. Insights from studies in rodents. Neurosci. Biobehav. Rev. 39, 51–60. doi: 10.1016/j.neubiorev.2013.12.005
Sgoifo, A., de Boer, S. F., Westenbroek, C., Maes, F. W., Beldhuis, H., Suzuki, T., et al. (1997). Incidence of arrhythmias and heart rate variability in wild-type rats exposed to social stress. Am. J. Physiol. 273(4 Pt 2), H1754–H1760.
Sgoifo, A., Koolhaas, J. M., Musso, E., and De Boer, S. F. (1999). Different sympathovagal modulation of heart rate during social and nonsocial stress episodes in wild-type rats. Physiol. Behav. 67, 733–738.
Sgoifo, A., Pozzato, C., Costoli, T., Manghi, M., Stilli, D., Ferrari, P. F., et al. (2001). Cardiac autonomic responses to intermittent social conflict in rats. Physiol. Behav. 73, 343–349. doi: 10.1016/S0031-9384(01)00455-3
Sgoifo, A., Pozzato, C., Meerlo, P., Costoli, T., Manghi, M., Stilli, D., et al. (2002). Intermittent exposure to social defeat and open-field test in rats: acute and long-term effects on ECG, body temperature and physical activity. Stress 5, 23–35. doi: 10.1080/102538902900012387
Shammas, R. A., Denison, A. L., Pfennig, T. W., Hemker, D. P., and Stephenson, R. B. (1988). Baroreflex unimpaired by operant avoidance or classical aversive conditioning in dogs. Am. J. Physiol. 254(6 Pt 2), R1025–R1034.
Slezak, P., Puzserova, A., Balis, P., Sestakova, N., Majzunova, M., Dovinova, I., et al. (2014). Genotype-related effect of crowding stress on blood pressure and vascular function in young female rats. Biomed Res. Int. 2014:413629. doi: 10.1155/2014/413629
Smith, A. S., Lieberwirth, C., and Wang, Z. (2013). Behavioral and physiological responses of female prairie voles (Microtus ochrogaster) to various stressful conditions. Stress 16, 531–539. doi: 10.3109/10253890.2013.794449
Smith, T. W., Glazer, K., Ruiz, J. M., and Gallo, L. C. (2004). Hostility, anger, aggressiveness, and coronary heart disease: an interpersonal perspective on personality, emotion, and health. J. Pers. 72, 1217–1270. doi: 10.1111/j.1467-6494.2004.00296.x
Spasojevic, N., Gavrilovic, L., Kovacevic, I., and Dronjak, S. (2009). Effects of antidepressants maprotiline and fluxilan on sympatho-adrenomedullary system in stressed rats. Auton. Neurosci. 145, 104–107. doi: 10.1016/j.autneu.2008.11.002
Stanley, S. C., Brooks, S. D., Butcher, J. T., d'Audiffret, A. C., Frisbee, S. J., and Frisbee, J. C. (2014). Protective effect of sex on chronic stress- and depressive behavior-induced vascular dysfunction in BALB/cJ mice. J. Appl. Physiol. (1985) 117, 959–970. doi: 10.1152/japplphysiol.00537.2014
Steptoe, A., and Kivimaki, M. (2012). Stress and cardiovascular disease. Nat. Rev. Cardiol. 9, 360–370. doi: 10.1038/nrcardio.2012.45
Sterling, P., and Eyer, P. (1988). “Allostasis: a new paradigm to explain arousal pathology,” in Handbook of Life Stress, Cognition and Health, eds S. Fisher and J. Reason (New York, NY: John Wiley & Sons), 629–649.
Sterling, P. (2012). Allostasis: a model of predictive regulation. Physiol. Behav. 106, 5–15. doi: 10.1016/j.physbeh.2011.06.004
Sved, A. F., and Gordon, F. J. (1994). Amino acids as central neurotransmitter in the baroreceptor reflex pathway. News Physiol. Sci. 9, 243–246.
Timio, M., Verdecchia, P., Venanzi, S., Gentili, S., Ronconi, M., Francucci, B., et al. (1988). Age and blood pressure changes. A 20-year follow-up study in nuns in a secluded order. Hypertension 12, 457–461.
Toot, J. D., Reho, J. J., Novak, J., Dunphy, G., Ely, D. L., and Ramirez, R. J. (2011). Colony social stress differentially alters blood pressure and resistance-sized mesenteric artery reactivity in SHR/y and WKY male rats. Stress 14, 33–41. doi: 10.3109/10253890.2010.491876
Tornatzky, W., and Miczek, K. A. (1993). Long-term impairment of autonomic circadian rhythms after brief intermittent social stress. Physiol. Behav. 53, 983–993.
Tsvirkun, D., Bourreau, J., Mieuset, A., Garo, F., Vinogradova, O., Larina, I., et al. (2012). Contribution of social isolation, restraint, and hindlimb unloading to changes in hemodynamic parameters and motion activity in rats. PLoS ONE 7:e39923. doi: 10.1371/journal.pone.0039923
Ueyama, T., Kasamatsu, K., Hano, T., Yamamoto, K., Tsuruo, Y., and Nishio, I. (2002). Emotional stress induces transient left ventricular hypocontraction in the rat via activation of cardiac adrenoceptors: a possible animal model of “tako-tsubo” cardiomyopathy. Circ. J. 66, 712–713. doi: 10.1253/circj.66.712
van den Buuse, M., Van Acker, S. A., Fluttert, M., and De Kloet, E. R. (2001). Blood pressure, heart rate, and behavioral responses to psychological “novelty” stress in freely moving rats. Psychophysiology 38, 490–499. doi: 10.1111/1469-8986.3830490
Vianna, D. M., and Carrive, P. (2005). Changes in cutaneous and body temperature during and after conditioned fear to context in the rat. Eur. J. Neurosci. 21, 2505–2512. doi: 10.1111/j.1460-9568.2005.04073.x
Viken, R. J., Johnson, A. K., and Knutson, J. F. (1991). Blood pressure, heart rate, and regional resistance in behavioral defense. Physiol. Behav. 50, 1097–1101.
von Känel, R. (2012). Psychosocial stress and cardiovascular risk: current opinion. Swiss Med. Wkly. 142, w13502. doi: 10.4414/smw.2012.13502
Vyas, A., Mitra, R., Shankaranarayana Rao, B. S., and Chattarji, S. (2002). Chronic stress induces contrasting patterns of dendritic remodeling in hippocampal and amygdaloid neurons. J. Neurosci. 22, 6810–6818.
Weiss, J. M. (1970). Somatic effects of predictable and unpredictable shock. Psychosom. Med. 32, 397–408.
Willnér, P. (2005). Chronic mild stress (CMS) revisited: consistency and behavioural-neurobiological concordance in the effects of CMS. Neuropsychobiology 52, 90–110. doi: 10.1159/000087097
Wood, S. K., McFadden, K. V., Grigoriadis, D., Bhatnagar, S., and Valentino, R. J. (2012). Depressive and cardiovascular disease comorbidity in a rat model of social stress: a putative role for corticotropin-releasing factor. Psychopharmacology (Berl). 222, 325–336. doi: 10.1007/s00213-012-2648-6
Xie, F., Sun, L., Su, X., Wang, Y., Liu, J., Zhang, R., et al. (2012). Neuropeptide Y reverses chronic stress-induced baroreflex hypersensitivity in rats. Cell. Physiol. Biochem. 29, 463–474. doi: 10.1159/000338500
Yang, H. J., Kim, K. Y., Kang, P., Lee, H. S., and Seol, G. H. (2014). Effects of Salvia sclarea on chronic immobilization stress induced endothelial dysfunction in rats. BMC Complement. Altern. Med. 14:396. doi: 10.1186/1472-6882-14-396
Yip, A. W., and Krukoff, T. L. (2002). Endothelin-A receptors and NO mediate decrease in arterial pressure during recovery from restraint. Am. J. Physiol. Regul. Integr. Comp. Physiol. 282, R881–R889. doi: 10.1152/ajpregu.00308.2001
Yoon, S. H., Kim, B. H., Ye, S. K., and Kim, M. H. (2014). Chronic non-social stress affects depressive behaviors but not anxiety in mice. Korean J. Physiol. Pharmacol. 18, 263–268. doi: 10.4196/kjpp.2014.18.3.263
Zhang, Z. Q., Julien, C., and Barres, C. (1996). Baroreceptor modulation of regional haemodynamic responses to acute stress in rat. J. Auton. Nerv. Syst. 60, 23–30.
Zhang, Z. Q., Julien, C., Cerutti, C., Paultre, C., and Barres, C. (1992). Role of sympathetic nerve fibers in hemodynamic responses to stress in rats. Arch. Mal. Coeur Vaiss. 85, 1141–1144.
Zhao, Y., Wang, W., and Qian, L. (2007). Hsp70 may protect cardiomyocytes from stress-induced injury by inhibiting Fas-mediated apoptosis. Cell Stress Chaperones 12, 83–95. doi: 10.1379/CSC-231R.1
Zhu, S., Shi, R., Wang, J., Wang, J. F., and Li, X. M. (2014). Unpredictable chronic mild stress not chronic restraint stress induces depressive behaviours in mice. Neuroreport 25, 1151–1155. doi: 10.1097/WNR.0000000000000243
Keywords: psychological stress, restraint stress, chronic variable stress, social isolation, social defeat, crowding stress, baroreflex, autonomic activity
Citation: Crestani CC (2016) Emotional Stress and Cardiovascular Complications in Animal Models: A Review of the Influence of Stress Type. Front. Physiol. 7:251. doi: 10.3389/fphys.2016.00251
Received: 13 May 2016; Accepted: 09 June 2016;
Published: 24 June 2016.
Edited by:
Valdir Andrade Braga, Federal University of Paraiba, BrazilReviewed by:
Eugene Nalivaiko, University of Newcastle, AustraliaCopyright © 2016 Crestani. This is an open-access article distributed under the terms of the Creative Commons Attribution License (CC BY). The use, distribution or reproduction in other forums is permitted, provided the original author(s) or licensor are credited and that the original publication in this journal is cited, in accordance with accepted academic practice. No use, distribution or reproduction is permitted which does not comply with these terms.
*Correspondence: Carlos C. Crestani, Y2NjcmVzdGFuaUB5YWhvby5jb20uYnI=
Disclaimer: All claims expressed in this article are solely those of the authors and do not necessarily represent those of their affiliated organizations, or those of the publisher, the editors and the reviewers. Any product that may be evaluated in this article or claim that may be made by its manufacturer is not guaranteed or endorsed by the publisher.
Research integrity at Frontiers
Learn more about the work of our research integrity team to safeguard the quality of each article we publish.