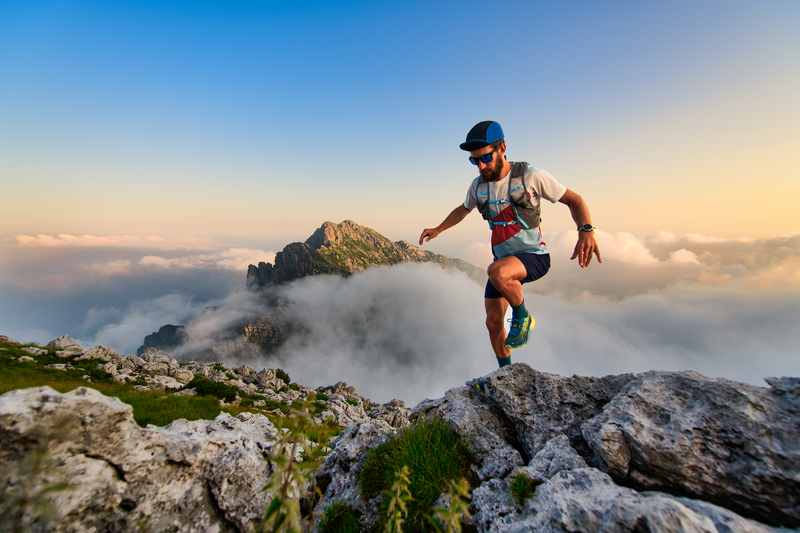
95% of researchers rate our articles as excellent or good
Learn more about the work of our research integrity team to safeguard the quality of each article we publish.
Find out more
REVIEW article
Front. Physiol. , 02 June 2016
Sec. Membrane Physiology and Membrane Biophysics
Volume 7 - 2016 | https://doi.org/10.3389/fphys.2016.00195
This article is part of the Research Topic P-type ATPases in health and disease View all 19 articles
Decreased Na+, K+-ATPase (NKA) activity causes energy deficiency, which is commonly observed in neurodegenerative diseases. The NKA is constituted of three subunits: α, β, and γ, with four distinct isoforms of the catalytic α subunit (α1−4). Genetic mutations in the ATP1A2 gene and ATP1A3 gene, encoding the α2 and α3 subunit isoforms, respectively can cause distinct neurological disorders, concurrent to impaired NKA activity. Within the central nervous system (CNS), the α2 isoform is expressed mostly in glial cells and the α3 isoform is neuron-specific. Mutations in ATP1A2 gene can result in familial hemiplegic migraine (FHM2), while mutations in the ATP1A3 gene can cause Rapid-onset dystonia-Parkinsonism (RDP) and alternating hemiplegia of childhood (AHC), as well as the cerebellar ataxia, areflexia, pescavus, optic atrophy and sensorineural hearing loss (CAPOS) syndrome. Data indicates that the central glutamatergic system is affected by mutations in the α2 isoform, however further investigations are required to establish a connection to mutations in the α3 isoform, especially given the diagnostic confusion and overlap with glutamate transporter disease. The age-related decline in brain α2∕3 activity may arise from changes in the cyclic guanosine monophosphate (cGMP) and cGMP-dependent protein kinase (PKG) pathway. Glutamate, through nitric oxide synthase (NOS), cGMP and PKG, stimulates brain α2∕3 activity, with the glutamatergic N-methyl-D-aspartate (NMDA) receptor cascade able to drive an adaptive, neuroprotective response to inflammatory and challenging stimuli, including amyloid-β. Here we review the NKA, both as an ion pump as well as a receptor that interacts with NMDA, including the role of NKA subunits mutations. Failure of the NKA-associated adaptive response mechanisms may render neurons more susceptible to degeneration over the course of aging.
In 1957, the Danish physician Jens C. Skou discovered the mechanism behind active ion transport in homogenates of leg nerve from shore crabs: a Mg2+-dependent ATPase stimulated by Na+ and K+, speculated to be located at the plasma membrane (Skou, 1957). In the same year that Skou published his ATPase work, Robert L. Post and Philip Jolly showed that interdependent active Na+ efflux and K+ influx followed an electrogenic stoichiometry of 3:2 (Post and Jolly, 1957), an exchange previously shown to be blocked by the cardiotonic steroid (CTS) isolated from plant species of the genus Strophanthus, strophantin, widely known as ouabain (OUA) (Schatzmann, 1953; Schatzmann and Witt, 1954). In 1960, Post and colleagues demonstrated the presence of Na+, K+-ATPase (NKA) in the plasma membrane of human red blood cells (Post et al., 1960), including its transient phosphorylation by ATP on an aspartyl residue (Post et al., 1965; Post and Kume, 1973). Subsequent work indicated a feed-forward reaction, whereby as Na+ binds to the enzyme, it stimulates a Mg2+-dependent phosphorylation via ATP, while K+ binding facilitates dephosphorylation, giving a transition of two conformations named E1 and E2, respectively (Post et al., 1972). Later work made it clear that activity of this molecular motor is vital for cell integrity, given its maintenance of the osmotic balance and its powerful role in cell homeostasis, including preserving and contributing to the resting membrane potential, electrolyte constitution of the cerebrospinal fluid (CSF) and influencing the cellular and/or transcellular transport of other ions, energy substrates and neurotransmitters (Blanco and Mercer, 1998; Skou, 1998; Blanco, 2005).
The enzyme is composed of two distinct polypeptides (Kyte, 1971), with equimolar stoichiometry (Craig and Kyte, 1980). The larger one is the α subunit, with an amino acid sequence (>1010 amino acids) that was first deduced from sheep kidney complementary DNA in 1985 by Jerry Lingrel's group (Shull et al., 1985). The α subunit has a mass of 110–112 kDa, with 10 transmembrane domains, mostly with protein resides in the cytoplasm side and with less than 10% exposed to the extracellular milieu (Blanco, 2005). The α subunit is also known as the catalytic or functional subunit, since it contains the binding sites for Na+ and K+, as well as for CTS. The α subunit is subject to transient phosphorylation and breaks down Mg2+-ATPase (Kyte, 1975; Blanco, 2005). The smaller β subunit is a type II (one transmembrane domain with intracellular N-terminal) glycoprotein of 302–304 amino acids that noncovalently binds to the α subunit (Shull et al., 1985). Although the α subunit can display independent ATP catalysis (Blanco et al., 1994), it is only when it is associated with the β subunit that normal pumping activity is achieved. The β subunit also influences the NKA kinetic characteristics, assisting the correct assembly and membrane delivery of the enzyme (chaperone function), as well as helping in cell adhesion and cell polarity (Blanco, 2005; Geering, 2008; Cereijido et al., 2012; Vagin et al., 2012). A third partner comprises the αβ protomer (formerly termed the γ subunit) (Forbush et al., 1978). This type I (one transmembrane domain with intracellular C-terminal) protein is small—around 65 amino acids, with a mass of 7 kDa—and belongs to the FXYD family of ion transport regulators. FXYD represents the signature motif found in all 7 members. FXYD2 is the original γ subunit, although all can interact with NKA (Geering, 2008). However, they are not necessary for enzymatic activity, modulating, usually decreasing, pump affinity for cations (Geering, 2006, 2008). The electrophoresis technique has unveiled puzzling pharmacological data that demonstrate differences in the potency and affinity of CTS for NKA, when NKA is derived from different tissues and species (Akera et al., 1972; Tobin and Brody, 1972; De Pover and Godfraind, 1976, 1979; Marks and Seeds, 1978; Noel and Godfraind, 1984). By the end of 1970's, Kathlyn Sweadner demonstrated in brain preparations of several mammalian species that two protein bands of slightly different molecular weights could be clearly defined, with the biochemical characteristics of NKA (Sweadner, 1979). The kidney α subunit co-migrated with the brain lower migrating (lighter) band in gel and was designated α (now α1), with the other brain band, heavier and not present in kidney, designated α(+) (Sweadner and Gilkeson, 1985). A third form was revealed from rat brain cDNA sequence analysis and named αIII (now α3), a major form in the central nervous system (CNS) (Shull et al., 1986; Schneider et al., 1988; Sweadner, 1989). α(+) was indeed a mixture of α2 and α3. Years later, a fourth α isoform was discovered and characterized (Shamraj and Lingrel, 1994; Woo et al., 1999; Blanco et al., 2000). All isoforms are encoded by different genes, have a high degree of sequence homology and are expressed in a cell/tissue-specific manner (Broude et al., 1989; Mobasheri et al., 2000; Blanco, 2005): α1 is expressed in all mammalian tissue, being virtually the only α isoform expressed in the kidney across species; α2 is found in striated and smooth muscles, adipose tissue, nervous tissue and some other tissues; α3 and α4 have a more restricted distribution, with the former being primarily found in nervous tissue, more specifically in neurons, where it may be considered a brain neuronal marker (Dobretsov and Stimers, 2005), and the latter is only expressed in the midpiece of the sperm, where it is important for sperm motility (Sanchez et al., 2006).
NKA has been classically conceptualized as a pump, with its specific inhibition by CTS that increases intracellular Na+ and Ca2+ concentrations leading to cell ionic imbalance (Akera and Brody, 1977). This was challenged by a series of elegant studies headed by Zi-Jian Xie and Amir Askari 20 years ago. These researchers observed that OUA induced myocyte hypertrophy by activating proto-oncogenes and late response genes, with effects mediated by the Ras-Raf-ERK1/2-pathway (Peng et al., 1996; Huang L. et al., 1997; Kometiani et al., 1998). This was preceded by EGFR transactivation through the nonreceptor Tyr kinase, Src, steps not dependent on Ca2+(Haas et al., 2000). Such signal transducer pump effects arise from activity in restricted areas of the plasma membrane, within a subset of lipid rafts known as caveolae (Liu et al., 2003; Wang et al., 2004; Quintas et al., 2010). NKA (E1 state) can interact with Src at the kinase and SH3 domain with OUA, by arresting the E2 conformation of the ATPase, unleashing the kinase domain of Src, thereby inducing protein-protein signaling (Liang et al., 2006; Tian et al., 2006; Ye et al., 2013). As such, NKA-Src forms a binary receptor that transduces signals after CTS binding.
After the first reports on the novel signaling role of NKA, a number of different intracellular pathways involved in distinct cell fates has been unveiled, confirming NKA as more than an ion pump (Nesher et al., 2007; Cai et al., 2008; Riganti et al., 2011; Arnaud-Batista et al., 2012; Lucas et al., 2012) and helping to clarify how endogenous CTS can produce their effects in vivo, even at very low concentrations (Xie and Xie, 2005; Aperia et al., 2016).
Considering the importance of NKA in basic cellular functions, it has been suggested that NKA mutations affecting α1 (ATP1A1), α2 (ATP1A2), and α3 (ATP1A3) genes can contribute to the pathogenesis of several CNS diseases.
Mutation in the ATP1A1 gene leads to primary aldosteronism (Azizan et al., 2013), which is the main cause of secondary hypertension. Aldosterone production is elevated and non-suppressible by sodium loading (Duan and Mete, 2015). The mutation causes a decrease in NKA activity and in K+ affinity, consequently leading to an inappropriate cellular depolarization (Beuschlein et al., 2013). Primary aldosteronism can also be caused by mutations in ATP2B3 (Ca2+-ATPase), CACNA1D (Cav1.3), and KCNJ5 (K+ channel) (Azizan et al., 2013; Zennaro et al., 2015). In a study with 474 patients, ATP1A1 mutation was found in 5.3% of the sample, although the relationship between the disorder and the ATP1A1 mutation was only discovered recently, requiring further investigations as to the mechanism involved (Fernandes-Rosa et al., 2014).
ATP1A3 is only expressed in CNS neurons, mostly in the cerebellum and basal ganglia, key structures in the regulation of a range of functions, including motor activity, memory and spatial learning. The ATP1A3 mutations are common in the conserved transmembrane or N-terminus domain of NKA and are related to rare disorders, such as rapid-onset dystonia-parkinsonism (RDP), alternating hemiplegia of childhood (AHC), and cerebellar ataxia, areflexia, pescavus, optic atrophy, and sensorineural hearing loss (CAPOS) syndrome. Although having many common features, these three diseases have quite distinct phenotypes (Sweney et al., 2015).
RDP (or DYT12) is a type of dystonia, being classed as a hyperkinetic movement disorder. RDP onset can be highly variable, occurring from 18 months to 55 years, suggesting considerable heterogeneity in its pathophysiology (Sweney et al., 2015). The main features are involuntary muscle contractions, abnormal posture and repetitive movements. RDP was first linked to ATP1A3 mutations by De Carvalho Aguiar and colleagues in 2004 (de Carvalho Aguiar et al., 2004), having an autosomal dominant inheritance. However, this disorder can also be sporadic or not related to any mutation in ATP13A (Kabakci et al., 2005). Although some RDP symptoms resemble Parkinson's disease, with both disorders showing evidence of abnormal CSF dopamine metabolites, RDP patients are unresponsive to deep brain stimulation (Charlesworth et al., 2013) or to L-DOPA treatment (Asmus and Gasser, 2010). RDP differentiation from Parkinson's disease is based on: triggering by physical or emotional stress, abrupt onset, bulbar involvement and normal computed tomography in the striatum (Zanotti-Fregonara et al., 2008; Asmus and Gasser, 2010).
Twelve mutations are associated with RDP, each being related to different severity levels. RDP treatment is only symptomatic, mostly utilizing benzodiazepines (Sweney et al., 2015). The T613M mutation is the most common and with the most severe outcome. Psychiatric conditions, such as bipolar disorder and anxiety, seem to be related to RDP (Barbano et al., 2012). A growing variety of clinical presentations have been reported in association with these mutations, including episodes of flaccidity and lack of motion for hours leading to stiffness (Anselm et al., 2009) or delayed motor development and hypotonia that lead to a uncoordinated gait, as well as speech and swallowing difficulties in R756H and D823N mutations.
Recent studies on a family with RDP, where only women present with a symptomatic phenotype, indicate a new mutation that causes a deletion (c.443_445delGAG, p.Ser148del). A male member from the same family also carries the p.Ser148del mutation, but he does not have any symptoms, suggesting a reduced penetrance of this mutation (Wilcox et al., 2015). RDP patients are still commonly misdiagnosed, indicating the need to incorporate new features in order to make an appropriate diagnosis (Brashear et al., 2012).
The onset of motor symptoms in RDP patients occurs usually by 25 years of age, with initial symptoms occurring in the upper body. Following initial diagnosis, patients have been shown to have poor learning, memory and psychomotor speed vs. controls (Cook et al., 2014). This would seem to arise from α3 being highly expressed in all nuclei of the basal ganglia and cerebellum, which are important regions for the control of movement, and in the hippocampus, which is crucial to spatial learning and memory (Oblak et al., 2014). Experiments utilizing in vitro and in vivo models have investigated the alterations driven by these mutations, including the role of impaired NKA activity. A C-terminus protein mutation decreases Na+ affinity, although no abnormality in biogenesis or plasma membrane targeting was found. Also, a decreased survival in these neuronal cells was observed when they were treated with OUA (Blanco-Arias et al., 2009).
Other mutations were also tested in COS7 cells, with no phosphorylation from ATP and low Na+ affinity being detected in most mutations. Consequently, increased intracellular Na+ and decreased extracellular K+ were observed. Thus, the affinity of Na+ may be an important concern in RDP (Toustrup-Jensen et al., 2014) (Figure 1). In mutated α3 mice (Het mice), stress exposure was capable of causing motor and balance deficits characteristic of RDP (DeAndrade et al., 2011). These authors also showed significant alterations in monoamine metabolism and thermal sensitivity in this model (DeAndrade et al., 2011).
Figure 1. Schematic representation of the influence of ATP1A2 mutations in glia and ATP1A3 mutations in neurons on glutamatergic system activity. Mutations cause a dysfunctional NKA activity, (A) ATP1A3 mutation in neurons decreases the NKA activity and increases intracellular Na+, which increases the cellular excitability, thereby affecting neuronal functions. Flumarazine and topiramate could be used as treatment in some cases. (B) ATP1A2 in astrocytes results in change of metabolism, more K+, and glutamate in the extracellular space and rendering the CNS more vulnerable to migraine, seizures, and neurodegenerative process.
AHC is a neurodevelopmental disorder described initially in 1971 by Verret and Steele (1971). However, it was only in 2012 that AHC was discovered to be a heterozygous ATP1A3 de novo mutation (Heinzen et al., 2012; Rosewich et al., 2014). AHC patients present with an onset before the age of 18 months and following an environmental or physiological stimulus, which is also present in RDP. AHC patients present with motor delay and attacks of hemiplegia, as well as cognitive and intellectual deficits (Sweney et al., 2015). AHC disorder can be difficult to differential diagnosis especially in relation to familial hemiplegic migraine (FHM) and glutamate transport disease (Jen et al., 2005). Sleep can stop AHC attacks, although even after sleep, attacks can return. Avoidance of the possible triggers is also important to prophylaxis, whilst antiepileptic drugs should only be used in patients showing status epilepticus (Rosewich et al., 2014; Paciorkowski et al., 2015). Flunarizine is a Ca2+ and Na+ channel blocker, which was developed to treat migraine and is currently the main AHC treatment, although the mechanism of action is unknown. Similarly, topiramate's mode of efficacy is unknown and may include its inhibition of 2-amino-3-(3-hydroxyl-5-methyl-4-isoxazolyl) propionic acid (AMPA) receptors and carbonic anhydrase, thereby lowering extracellular pH (Hoei-Hansen Et al., 2014; Yang et al., 2014) (Figure 1).
Another strategy used in AHC patients is the ketogenic diet/modified Atkins diet, given that a family with the p.Asp923Asn mutation was initially misdiagnosed as GLUT1DS (glucose transporter deficiency syndrome), with 15 months of diet management leading to no further attacks. A ketogenic diet provides an alternative energy source for the brain and may decrease neuroexcitability (Roubergue et al., 2015). In some cases, when a patient has two mutations in SLC2A1 and ATP1A3, flunarizine provides an incomplete response, with concurrent use of a ketogenic diet improving the utility of flunarizine (Ulate-Campos et al., 2014). A patient with c.2401G>A; p.D801N mutation failed to respond to all the treatment strategies and only the use of a corticosteroid achieved complete remission (Wong and Kwong, 2015).
In Japan, the more severe phenotype arising from the E815K mutation was found to be more common than in other countries, occurring in 50% of investigated patients. The more severe phenotype evident in these mutations includes: earlier age of onset, more epileptic episodes and increased motor impairment (Ishii et al., 2013). Usually patients with no detectable mutation have less symptomatology (Hoei-Hansen et al., 2014), whilst an early onset of AHC symptoms seems positively correlated with a more severe clinical course (Viollet et al., 2015).
A Danish study showed bilateral episodes to be common, with hemiplegic episodes not always alternating. Consequently, the term AHC may not be the most appropriate (Hoei-Hansen Et al., 2014). A recent study of 155 patients showed 34 mutations to be related to AHC, with at least one being detected in 85% of patients. The three major mutations were found p.Glu815Lys, p.Asp801Asn, and p.Gly947Arg, with the first having the most severe phenotype, as indicated by greater intellectual impairment and motor disability. In contrast, p.Asp801Asn presents with a milder phenotype, whilst p.Gly947Arg appears to have the most favorable prognosis, in comparison to other mutations (Panagiotakaki et al., 2015).
Some mutations show a symptomatology that indicates a combination of RDP and AHC, including c.2600G>A, D923N and R756 (Rosewich et al., 2014). The E277K and D923N mutations have been described in both (Boelman et al., 2014). The difference between RDP and AHC may be due to different regions and cells affected by the mutations in ATP13A, with differential impacts arising from effects within a developmental context (Ozelius, 2012). Patients with epilepsy had an earlier onset, although no relationship with a specific mutation (Panagiotakaki et al., 2015). AHC patients have electrocardiogram and repolarization abnormalities compared to people with epilepsy, contributing to episodes of collapse unrelated to seizures, as well as to some premature deaths (Novy et al., 2014; Jaffer et al., 2015). Activated platelets from AHC patients have functional and structural abnormalities, with 93 proteins related to metabolism being differentially expressed in comparison to healthy controls. Cathepsin B was also more highly expressed, indicating an important role of apoptotic pathway activation in driving mutation-associated cell death (Di Michele et al., 2013). An AHC patient showing a mutation in SLC2A1 p.Gly18Arg, which leads to the loss of GLUT1 expression, was also diagnosed with hemiplegic migraine, which can be related to increases in cortex glutamate levels. As such, glutamate levels should also be investigated in mutation-associated AHC patients (Weller et al., 2015).
Mutations of ATP1A3 that can be evident in AHC patients, have been tested in Sf9 cells. OUA binding, NKA activity and phosphorylation were measured for each mutant and were found to be abnormal in I274N, E815K, and G947R mutations. Some mutations showed normal bind to OUA, although no NKA activity or phosphorylation were found. Such differences may be associated with phenotype severity (Weigand et al., 2014). In a murine AHC model, the D801N mutant mouse (Mashlool, Mashl+/), a similar symptomatology is found as that evident in AHC patients, including abnormalities in gait and motor coordination, as well as spontaneous recurrent seizures and hemiplegia. The study of hippocampal slices showed increased excitability, which contributes to abnormal plasticity and a predisposition to more spontaneous seizures (Hunanyan et al., 2015).
Another animal model, the Myshkin (Myk) model, carries the I810N mutation, which presents with a reduced threshold for hippocampal seizures and neuronal loss. Although α3 is normally expressed, NKA activity is reduced with a consequent reduction in thalamocortical functional connectivity (Clapcote et al., 2009; Kirshenbaum et al., 2013). This murine model is also used as a model of bipolar disorder, with symptoms being improved by melatonin and physical exercise (Kirshenbaum et al., 2014). An α3 mutation is evident in the Atp1a3tm1Ling murine model, leading to a reduction in hippocampal α3 expression, thereby lowering NKA activity. Dystonia is only induced in this model after kainate injection into the cerebellum (Ikeda et al., 2013).
In 1996, CAPOS syndrome was described, with only the c.2452G> mutation in ATP1A3 being found in four families studied. This is a gain-of-function mutation, which differs from the loss mutations evident in RDP and AHC (Demos et al., 2014). CAPOS is a rare syndrome associated with febrile illness, with each patient differing in regard to severity and outcome (Sweney et al., 2015). Recently, CAPOS syndrome has been shown to cause hemiplegic migraine in a single case study (Potic et al., 2015).
Migraine is a brain disorder that affects over 10% of the population, characterized by a headache associated with neurological and autonomic symptoms. Moreover, it frequently occurs as a pulsating and unilateral headache, usually followed by phono/photophobia and nausea (Russell et al., 1994). Migraine is thought to be initiated by brain dysregulation, leading to activation and increased vulnerability of the trigeminovascular system, mainly the trigeminal nociceptive afferents innervating the meninges (Goadsby et al., 2002; Pietrobon and Striessnig, 2003; Pietrobon, 2005). Migraine disorders may be preceded, or not, by neurological symptoms that are often visual, and can therefore be classified as migraine with or without aura (Pietrobon and Striessnig, 2003; Pietrobon, 2005). Clinical studies indicate that migraine aura is related with a wave of cortical spreading depression (CSD). CSD is a strong wave of short duration of neuronal and glial cell depolarization, which extends over the cortex and is accompanied by long-lasting depression of neuronal activity (Lauritzen, 1994; Cutrer et al., 1998; Bowyer et al., 2001; Hadjikhani et al., 2001). Studies have shown a relationship between CSD and migraine aura in humans and animal models and its importance to trigeminal activation (Russell et al., 1994; Bowyer et al., 2001; Hadjikhani et al., 2001; Bolay et al., 2002; Goadsby, 2002; Dalkara et al., 2006; Zhang et al., 2010).
FHM is a special autosomal dominant subtype of migraine with aura, characterized by some degree of motor frailty or paralysis, often hemiparesis (Thomsen et al., 2002). Furthermore, severe cases have been associated with symptoms such as progressive cerebellar ataxia, coma, fever, and/or epileptic seizures (Ducros et al., 1997). FHM is a genetically heterogeneous disease, in which mutations can occur in the ion transportation genes CACNA1A, ATP1A2, and SCN1A (Rogawski, 2008), promoting the different types of familial migraines, FHM1, FHM2 and FHM3 (Russell et al., 1994; Pierelli et al., 2006; Deprez et al., 2008). FHM1 is induced by mutations in CACNA1A at chromosome 19p13, which encodes the Cav2.1 (P/Q-type) (Ophoff et al., 1996). FHM2 is occasioned by mutations in the ATP1A2 at chromosome 1q23 (De Fusco et al., 2003). The last gene is SCN1A, encoding the α1 subunit of the neuronal sodium channel, the mutation in which leads to FHM3 (Dichgans et al., 2005). In this review, we describe only mutations in ATP1A2, as well as the role of glutamate in the development of FHM. FHM was the first human disease linked to mutations of the α2 catalytic subunit, which was first demonstrated in two Italian families (De Fusco et al., 2003). There is also a linkage between sporadic hemiplegic migraine (SHM) and mutations in α2 (Thomsen and Olesen, 2004; Kirchmann et al., 2006), with other brain disorders also associated with α2 mutations.
In the mouse, α2 is expressed in cardiac and skeletal muscle throughout life. However, in neurons, Atp1a2 is only expressed during embryonic development (Moseley et al., 2003), being present in astrocytes and meningeal tissues in adulthood (McGrail et al., 1991; Watts et al., 1991; Peng et al., 1997). During neuronal activity, NKA is important for removing K+ from the synaptic cleft. Moreover, it is fundamental for the re-uptake of released glutamate from the extracellular space, since active transport of glutamate into neurons and astrocytes is regulated by Na+ and K+ gradients, emphasizing the importance of NKA in synaptic regulation (Ransom et al., 2000; D'Ambrosio et al., 2002; Jorgensen et al., 2003). Atp1a2-deficient embryos have impaired neurotransmitter clearance, as well as potentiated neural excitation and cell death, specifically in the amygdala and piriform cortex. Consequently, heterozygous adult mice show increased levels of anxiety/fear behaviors, concurrent to raised levels of c-Fos and increased neuronal activity in the piriform cortex and amygdala after fear stimuli. This indicates a role for α2 in regulating not only neural activity (Ikeda et al., 2003), but also affective processing, including in the role of the amygdala in cortex development.
Kawakami et al. (2005) reported that heterozygous Atp1a2 mice, KOE21, show spontaneous epileptic seizures. In addition, their data demonstrated neuronal apoptosis in the piriform cortex and amygdala (Ikeda et al., 2003), being a possible explanation as to the manifestation of epilepsy in 20% of FHM2/SHM families (Bianchin et al., 2010). These epileptic seizures arise when neurons situated in temporal lobe regions, including the amygdala, piriform cortex and hippocampus become hyperexcitable (Aroniadou-Anderjaska et al., 2008). However, histological changes were not observed in the piriform cortex and amygdala in the brains of KOE21 knockout mice (Ikeda et al., 2004). A colocalization of the NKA α2 with NCX occurs in astrocyte cultures, suggesting a central NKA role in the modulation of intracellular Ca2+ of the endoplasmic reticulum (Juhaszova and Blaustein, 1997). In addition, high levels of intracellular and intra-endoplasmic reticulum Ca2+ ions were detected in cultured astrocytes from Atp1a2-deficient mice (Golovina et al., 2003), linking NKA α2 to the manifold consequences of Ca2+ dysregulation.
Mutation in astrocyte NKA α2 facilitates both neuronal paroxysmal depolarizing shifts and hyper-excitability, triggering seizures as well as CSD induction and propagation in FHM/SHM patients (Pietrobon, 2005). Bolay et al. (2002), using an animal model of migraine, observed that CSD activates a N-methyl-D-aspartate (NMDA)-dependent pathway leading to an activation of nociceptive trigeminal afferents in the meninges, resulting in headache. Additionally, a specific role for NKA in glutamate clearance during synaptic transmission has also been established, with NKA being stimulated by glutamate in astrocyte cultures (Pellerin and Magistretti, 1997). Furthermore, a colocalization and functional coupling between NKA and glutamate transporters has also been demonstrated (Rose et al., 2009), with α2 having a similar perisynaptic glial localization to the glutamate transporters GLAST and GLT-1in the rat somatosensory cortex (Cholet et al., 2002; Rose et al., 2009). As such, inefficient astrocyte glutamate reuptake resulting in increased cortical excitatory neurotransmission, specifically as driven by NMDA receptor-dependent transmission during high-frequency action potential sequences, may enhance the sensibility to CSD in FHM2 (Tzingounis and Wadiche, 2007). Wider ionic dysregulation may also be evident, with the increase of extracellular K+ levels resulting from the impaired clearance by NKA, and an increase in the intracellular Na+ resulting in an elevation in the intracellular Ca2+ level through the NCX (Pietrobon, 2005), contributing to wider central disruption. Moreover, recent data indicates that impaired glutamate clearance by astrocytes, and involving the α2, is an important molecular mechanism of FHM2 (Figure 1). This in vitro study, in a hippocampal mixed astrocyte and neuronal culture derived from homozygous E17 embryonic mice, demonstrated reduced glutamate uptake due to the loss of functional NKAα2 in astrocytes. Interestingly, in vivo data in the same study showed a relationship between the glutamate system and the female sex hormone in psychiatric manifestations of FHM2. Furthermore, higher glutamate levels were evident in different brain regions from adult female mice, compared to male mice (Bøttger et al., 2016).
In support of a role for glutamatergic dysregulation in migraine, MTDH, a regulator of glutamate transporters, associates with the frequent form of migraine with aura. Furthermore, a mutation in the SLCA3 gene that encodes the glial excitatory amino acid transporter type 1 (EAAT1) has also been found, leading to neuronal hyper-excitability and consequent hemiplegia, as well as seizures and episodic ataxia, which arise as a consequence of impaired glutamate uptake (Jen et al., 2005).
Recent studies have demonstrated a link between long-term memory formation in the rat hippocampus and the astrocyte-neuron lactate transport (Suzuki et al., 2011). Furthermore, there is a relationship between NKA and the astrocyte specific glutamate-dependent transport of lactate (Kleene et al., 2007). This interesting finding may contribute to the pathophysiological processes underlying language disorder, cognitive impairment and mental retardation evident in FHM2/SHM patients (Le Pira et al., 2004; Kalaydjian et al., 2007). As such, evidence suggests that the central glutamatergic system has an important role in driving the consequences of ATP1A2 mutations and should also be more explored in ATP13A mutations, due to the diagnostic confusion and overlap with glutamate transporter diseases.
Glutamate is an amino acid, which is considered the main central excitatory neurotransmitter, being highly abundant in the majority of animals, especially in the cortex, hippocampus and caudate nucleus (reviewed in Erecinska and Silver, 1990). Glutamate's cellular actions are mediated through the activation of metabotropic and ionotropic receptors. The ionotropic receptors are divided according to their affinities for specific agonists, namely NMDA, AMPA, and kainate (McLennan, 1981). The glutamatergic metabotropic receptors were first investigated in late 1980's (Nicoletti et al., 1986). There are eight types of metabotropic receptors divided into three groups (Groups I, II, and III) depending on DNA sequence and the signaling pathway they activate (reviewed in Wieronska et al., 2016).
Glutamate has many roles, including being part of intermediary metabolism in the brain (Krebs, 1935; Berl et al., 1961) as well as acting as a precursor for different compounds, such as the inhibitory neurotransmitter gamma-aminobutyric acid (GABA) (Roberts and Frankel, 1950). By acting through metabotropic and/or ionotropic receptors, glutamate is also an important element in a variety of core processes such as learning and memory, partly by regulating synaptic plasticity via long-term potentiation (LTP) (Bliss and Collingridge, 1993; Vickery et al., 1997), long-term depression (LTD) (Dudek and Bear, 1992; Yokoi et al., 1996; Nicoll et al., 1998; Dong et al., 2013) or both (Manahan-Vaughan, 1997; Huang L. Q. et al., 1997; Wang et al., 2015). The hippocampus and cortex are the major brain regions investigated in studies of cognition, with these regions also showing the highest concentrations of brain glutamate. Glutamate is crucial to many other brain processes, including motor behavior (Conquet et al., 1994; Pekhletski et al., 1996; Coesmans et al., 2003; Talpalar and Kiehn, 2010; Ohtani et al., 2014; Guimaraes et al., 2015), as well as in brain development (Deisseroth et al., 2004; Poulsen et al., 2005; DeSilva et al., 2012; Yamasaki et al., 2014; Jantzie et al., 2015).
Abnormalities in glutamate function can arise from alterations in a variety of factors, including: glutamate transporters and glutamatergic receptors, as well as glutamate metabolism and synthesis. Also, changes in the levels of glutamate's precursor, glutamine, may drive alterations in the extracellular glutamate concentration. Inflammation is linked to a host of medical conditions, with effects linked to sustained glutamate elevations (Takaki et al., 2012) or changes in glutamatergic receptor expression (Drouin-Ouellet et al., 2011). Increased levels of glutamate contribute to inflammatory process, as indicated by the efficacy of glutamatergic receptor antagonist in reducing inflammation (Bonnet et al., 2015).
Lack of glutamate could lead to memory deficits, given that LTP in some brain areas is NMDA receptor-dependent (Malenka and Bear, 2004). However, excess glutamate can be excitotoxic to neurons. A number of studies show a strong correlation between abnormal glutamatergic signaling pathway and neurodegenerative/psychiatric diseases, including Alzheimer's disease (Takahashi et al., 2015; Haas et al., 2016), Parkinson's disease (Alvarsson et al., 2015; Morin et al., 2015), Huntington's disease (Behrens et al., 2002; Estrada-Sánchez et al., 2009), amyotrophic lateral sclerosis (Plaitakis et al., 1988; Veyrat-Durebex et al., 2015), epilepsy (Wong et al., 2015), ischemia (Dhami et al., 2013), migraine (Peres et al., 2004; Campos et al., 2013), schizophrenia (Spangaro et al., 2012; Matsuno et al., 2015), depression (Berman et al., 2000; Duric et al., 2013; Peng et al., 2016), addiction (Aitta-aho et al., 2012; Perry et al., 2016), and autism spectrum disorder (Bristot Silvestrin et al., 2013).
In the mammalian CNS, astrocytes are the most abundant glial cells. Astrocytes show two different NKA α isoforms, α1 and α2, with α1 being ubiquitously expressed (Geering, 2008). Under physiological conditions, during excitatory synaptic activity, astrocytes are responsible for glutamate uptake from the synaptic space, keeping extracellular levels of glutamate at nanomolar concentrations, and thereby avoiding the harmful consequences of glutamate receptor over-stimulation (Rothstein et al., 1996; Herman and Jahr, 2007; Kirischuk et al., 2012; Illarionova et al., 2014; Zhang et al., 2016). Astrocytes are therefore considered significant regulators of glutamatergic synaptic transmission (Amara and Fontana, 2002; Zhang et al., 2016). The glial glutamate transporters (GluTs) that mediate glutamate uptake rely on the NKA electrochemical gradient (Levy et al., 1998; Anderson and Swanson, 2000; Chatton et al., 2000; Kanner, 2006). The family of excitatory amino acid transporters (EAAT) are composed of the glutamate transporters, with GLAST (glutamate/aspartate transporter or EAAT1) and GLT-1 (glutamate transporter 1, or EAAT2) being considered the most important (Tanaka, 2000; Zhang et al., 2016) and the predominant type of GluT in adult astrocytes (Rothstein et al., 1996; Dunlop, 2006; Matos et al., 2013). As such, an alteration in the NKA ionic and electrochemical gradient is intimately associated with astrocyte regulation of glutamatergic activity.
In the cell, ATP depletion can lead to a reversal (Longuemare et al., 1999), or inhibition, of glutamate uptake (Sheean et al., 2013; Shan et al., 2014), as shown by inhibitory doses of OUA, which impairs glutamate transport (Pellerin and Magistretti, 1997; Rose et al., 2009; Genda et al., 2011) and leads to the redistribution and clustering of GluTs (Nakagawa et al., 2008; Nguyen et al., 2010). Some studies indicate a co-localization and physical interaction between the α2 subunit and GluTs (Cholet et al., 2002; Rose et al., 2009; Genda et al., 2011; Matos et al., 2013; Illarionova et al., 2014), suggesting NKA regulation of GluTs by direct contact, as well as via the electrochemical gradient.
Elevated glutamate concentrations (up to 50 μM) may stimulate NKA activity (Gegelashvili et al., 2007). This was observed in a study of human fetal astrocytes, which showed that during synaptic activity, when glutamate is elevated, there is an increase in cell membrane expression of the NKA FXYD2/γ subunit and GLAST, suggesting a possible interaction between them (Gegelashvili et al., 2007; Nguyen et al., 2010; Zhang et al., 2016). Furthermore, the inhibition of Src in forebrain synaptosomes with PP2 or SU6656, as well as the inhibition of NKA by OUA, both decreased glutamate transport activity to the same level (Rose et al., 2009), suggesting the existence of a possible protein complex formed between NKA/Src/GluT (Rose et al., 2009). Moreover, adenosine can modulate glutamate uptake through activation of astrocyte adenosine A2A receptors (A2ARs), which can interact with the α2 isoform in the adult mouse brain, leading to the inhibition of astrocyte glutamate uptake (Matos et al., 2013). This data strengthens the idea of a macromolecular complex in astrocytic membranes encompassing A2ARs, α2 and GLT-I (Matos et al., 2013; Reinhard et al., 2013), with parallels in neurons. In 2009, Rose and colleagues showed that in the rat hippocampus GLT-1b isoform is co-expressed with α2 in astrocytes, whereas GLT-1a isoform was co-expressed with α3, a neuronal specific isoform (Chen et al., 2004; Bassan et al., 2008; Furness et al., 2008; González-González et al., 2008; Rose et al., 2009; Petr et al., 2015).
Notwithstanding the existence of a well described physical interaction between α2 and GluTs (Cholet et al., 2002; Porras et al., 2008; Rose et al., 2009; Genda et al., 2011; Bauer et al., 2012; Matos et al., 2013; Rose and Chatton, 2016), the substantial Na+ influx that is driven into astrocytes during synaptic activity has been related to a possible functional interaction, whereby astrocytes increase the local availability of metabolic substrates (Rose and Chatton, 2016). During glutamate stimulation, there is a dramatic reduction in neuronal ATP mediated by NMDA receptor activation followed by an increase in NKA activity (Foo et al., 2012).
In the 1990's, Inoue and Matsui observed an increase in K+ uptake by α2 and α3 after stimulation of rat embryonic neurons with glutamate (100 μM) (Inoue and Matsui, 1990). Similar results were observed some years later in Wistar adult rat slices (Nathanson et al., 1995; Munhoz et al., 2005) by Munhoz et al. (2005), which described a pathway whereby glutamate stimulation of NMDA receptor-NOS leads to increased NKA activity, possibly due to increased cyclic GMP (cGMP) synthesis. Glutamate-induced Ca2+ influx leads to the activation of neuronal nitric oxide synthase (nNOS) and increased production of nitric oxide (NO). NO, by activating soluble guanylylcyclase (sGC) enzyme, increases concentrations of the second messenger cGMP, thereby up-regulating cGMP-dependent protein kinase (PKG). PKG modulates NKA, altering the activity of NKA isoforms, both centrally and peripherally (McKee et al., 1994; de Oliveira Elias et al., 1999; Munhoz et al., 2005; Scavone et al., 2005). Accordingly, a strong correspondence occurs between pharmacologically induced cGMP and NKA modulation in the cerebellum, reinforcing the role of the glutamate-NO-cGMP pathway in NKA regulation (Nathanson et al., 1995).
In 1999, Inoue and colleagues showed that when primary neuronal cultures from rat embryos were treated with three typical agonists of ionotropic glutamate receptors, namely kainic acid (KA), AMPA and NMDA, a remarkable increase in the activity of α2 and α3 isoforms and a slight decreased activity of α1 was observed, suggesting that glutamate effects on NKA may be mainly mediated by ionotropic glutamate receptors (Inoue et al., 1999; Kawamoto et al., 2012), with the neuronal NKA isoforms differing in physiological functions and playing a crucial role in restoring ion gradients after neuronal excitation (Inoue et al., 1999). Meanwhile, Brines and Robbins (1992) observed that prolonged inhibition of α2 and α3 could lead to the potentiation of glutamate neurotoxicity, with NKA activity also regulating the cell surface expression and turnover of AMPA receptors (Zhang et al., 2009). Interestingly, when primary cultured cerebellar neurons were treated with 0.1 μM glutamate a 37% increase in NKA activity was observed, while a concentration of 100 μM glutamate was able to increase NKA activity by 85%. However, when pre-treated with the NMDA receptor antagonist, MK-801, NKA activity increase was abrogated, suggesting that glutamate-driven NKA activation is mediated by NMDA receptors (Marcaida et al., 1996). Once NMDA receptors are activated, the subsequent Ca2+ influx can activate calcineurin, a calcium/calmodulin-dependent protein phosphatase (Marcaida et al., 1996; Rambo et al., 2012; Unoki et al., 2012; de Lores Arnaiz and Bersier, 2014), which, through NKA dephosphorylation, restores NKA enzyme activity (Bertorello et al., 1991; Aperia et al., 1992; Marcaida et al., 1996). It is well proven that protein kinase C (PKC) phosphorylates the α subunit, leading to a decrease in its enzyme activity (Bertorello and Aperia, 1989; Fukuda et al., 1991; Beguin et al., 1994; Marcaida et al., 1996; Cheng et al., 1997; Chibalin et al., 1998; Nishi et al., 1999; Taub et al., 2010; Liu et al., 2016). Calcineurin can desensitize NMDA receptors (Lieberman and Mody, 1994) via the C-terminus of the NR2A subunit (Krupp et al., 2002) and regulates calmodulin effects, reducing the open time of the channel (Rycroft and Gibb, 2004).
During learning processes PKC is active and phosphorylates some Src family kinases (SFK), which in turn induces tyrosine phosphorylation of NMDA receptors, leading to a rapid up-regulation of NMDA receptor membrane expression (Groveman et al., 2012). Some studies have proposed that Src protein forms a complex with the NMDA receptor through the binding of its SH2 domain with the N-terminal region of postsynaptic density protein 95 (PSD-95). Furthermore, PSD-95 seems to negatively regulate Src (Kalia et al., 2006; Groveman et al., 2012). Indeed, co-activation of mGluR5 and the NMDA receptor with low concentrations of their agonists (CHPG and NMDA, respectively), in rat hippocampal slices, leads to the phosphorylation of NR2B (Tyr1472) by Src kinase (Sarantis et al., 2015), in turn increasing NMDA-mediated excitability.
It is important to note that NKA acts as a specific receptor for CTS. The interplay between the NKA and NMDA receptors is proposed to arise from the initial administration of CTS leading to conformational changes that enhance NMDA subunit expression as a compensatory mechanism (de Lores Arnaiz and Bersier, 2014). OUA is also able to regulate NMDA binding and receptor activity in the adult rat brain (Reines et al., 2001, 2004; Bersier and Rodríguez de Lores Arnaiz, 2009), in a concentration-dependent manner (Bersier and Rodríguez de Lores Arnaiz, 2009). Akkuratov et al. (2015), in rat cerebellar neurons, showed that both α1 and α3 subunits can functionally interact with NMDA receptors whereas Sibarov and colleagues demonstrated by patch-clamp and Ca2+ imaging experiments that rat cortical neurons treated with 30 μM NMDA or KA with 1 nM OUA or digoxin for 4 or 6 h, were protected from apoptosis (Sibarov et al., 2012; Abushik et al., 2013). Similar protection was observed in vivo (Golden and Martin, 2006). One possible explanation is that an up-regulation of the plasma membrane NCX leads to more Ca2+ extrusion preventing the increase of sEPSC frequency, which is typically found in excitotoxicity process. This up-regulation of NCX activity seems to be due to an interaction between NKA and NCX (Sibarov et al., 2012).
Furthermore, a study published in 2013, in primary cerebellar cell culture treated with OUA in different concentrations (1, 10, and 100 mM) for different times (1, 2, and 4 h), suggested nuclear factor-kappaB (NF-κB) activation in a time and concentration-dependent manner. However, this effect was not observed when neurons were pre-treated with MK-801, PP1 (Src-family tyrosine kinase inhibitor), manumycin A (farnesyltransferase inhibitor), and PD98059 (mitogen-activated protein kinase (MAPK) inhibitor). As such, this study described a pathway whereby OUA activates NF-κB through the NMDA receptor-Src-Ras-MAPK pathways (de Sá Lima et al., 2013). Strong evidence indicates that NMDA can activate NF-κB in the rat hippocampus (Lipsky et al., 2001; Kawamoto et al., 2012), with intra-hippocampal injection of 1 μM NMDA 1 h before the injection of 10 nM OUA potentiating NF-κB activity (Kawamoto et al., 2012). This activation was reduced when cells were pre-treated with an NMDA receptor antagonist (MK-801), suggesting that NF-κB activation by OUA, in part, arises from NMDA receptor modulation (Kawamoto et al., 2012). Overall, the above results indicate that OUA, through the action of NKA as a pump or as a receptor, can modulate many signals important in neurotransmission.
Several studies have shown age-related modifications in the NMDA receptor-NO-cGMP cascade in the CNS (Peterson and Cotman, 1989; Tamaru et al., 1991; Wenk et al., 1991; Vallebuona and Raiteri, 1995; Wardas et al., 1997; Ossowska et al., 2001; Chepkova et al., 2015). In addition, the aging process is associated with a progressive decline in NKA activity, both centrally and peripherally (Scavone et al., 2000; Kawamoto et al., 2008; Vasconcelos et al., 2015). In the CNS, this reduction is due to the modulation of α2 and α3 isoforms with no change in α1 and Mg2+-ATPase activities, as reported in the rat cerebellum and hippocampus (Kawamoto et al., 2008; Vasconcelos et al., 2015). As no differences in the α2 and α3 protein levels are evident, the age-related decrease in NKA activity is linked to modifications in glutamate-cGMP-PKG pathway (Kawamoto et al., 2008). The age-related failure of NKA modulation by glutamate through cGMP-PKG signaling may be connected to ionic disturbances during senescence, which may predispose to neurodegenerative diseases (Scavone et al., 2005). Interestingly, while inflammation, closely associated with aging and neurodegeneration, worsens aging-associated glutamate signaling and NKA defects, intermittent fasting counteracts this effect (Vasconcelos et al., 2015) (Figure 2).
Figure 2. Schematic model for molecular mechanisms underlying aging modulatory effects on NKA isoforms. Aging can either increase the production of ROS, such as superoxide radical and hydrogen peroxide, or induce NO• release by impairing Ca2+ homeostasis and subsequently increasing intracellular Ca2+ (nNOS-mediated NO• production). NO• is a free radical and can generate peroxynitrite, which may cause neurotoxicity by lipid peroxidation, mitochondria disruption, mutations of DNA and proteins, apoptosis and impairment of α1 activity. Strategies that induce NMDA activation can also activate the cGMP pathway, which, in turn, may lead to neuroprotective signaling, partly by upregulating α2 and α3 (cGMP, cyclic GMP; GLU, glutamate; NKA, Na+,K+-ATPase).
DNA repair defects (Garinis et al., 2008; Schumacher et al., 2008; Végh et al., 2012), and consequent mitochondrial dysfunction (Zahn et al., 2007; Yankner et al., 2008), are considered important causes of aging and, in turn, with neurodegenerative disorders (Lin and Beal, 2006; Johri and Beal, 2012). Likewise, ROS-induced mitochondria impairment may contribute to the etiology of neurodegenerative disorders (Lin and Beal, 2006). Compromised mitochondria lead to impaired energy supply, producing less ATP and even more free radicals, as a consequence of inefficient oxidative phosphorylation, which, in turn, can directly or indirectly affect NKA activity (Nathanson et al., 1995). In support of this, a number of studies show the α subunit to be sensitive to free radical attack (Kim and Akera, 1987; Mense et al., 1997; Xie and Xie, 2005), with the oxidized α subunit being degraded (Zolotarjova et al., 1994; Thevenod and Friedmann, 1999). Furthermore, age-related increases in the levels of the free radical superoxide () lead to ONOO− formation via reaction with the NO generated by glutamate signaling (Wink et al., 1996). ONOO− also impairs NKA activity (Boldyrev et al., 1997; Sato et al., 1997; Muriel and Sandoval, 2000; Kocak-Toker et al., 2005; Reifenberger et al., 2008). NKA inhibition also increases neuronal susceptibility to glutamate excitotoxicity, at least in part by reducing the Na+ gradient necessary for glutamate transport and clearance from the synapse cleft, leading to a positive feedback loop (Lees et al., 1990; Brines and Robbins, 1992). Both cGMP and PKG are well-established as important mediators of LTP, which NKA also modulates (Stevens and Wang, 1993; Zhuo et al., 1994; Nathanson et al., 1995). Moreover, NKA impairment downregulates the synaptic AMPA receptor, contributing to synaptic transmission defects, and consequently cognitive decline (Zhang et al., 2009). Since cognitive impairment is a hallmark of aging and neurodegenerative diseases, NKA-mediated glutamate signaling dysfunction may be an important contributory factor to neurodegenerative disorders (Végh et al., 2012).
EAAT downregulation has been associated with many neurodegenerative diseases (Sheldon and Robinson, 2007). As described above, the resulting elevation in glutamate levels in the synapse cleft and consequent excitotoxicity may interfere in NKA functioning by increasing NO release and free-radical production (Dawson et al., 1991).
Glutamatergic signaling dysfunction and its associated consequences are critical contributors to the pathophysiological underpinnings of Alzheimer's disease (AD) (Lewerenz and Maher, 2015). AD is the most frequent form of dementia, being characterized by memory and cognitive impairments. The AD brain shows two classical changes: increased Aβ peptide deposition in senile plaques; and tau hyperphosphorylation that leads to neurofibrillary tangles (Khachaturian, 1985; Mattson, 2004). AD is also associated with increased ROS production (Butterfield et al., 2001), compromised glutamate clearance (Lauderback et al., 2001; Vitvitsky et al., 2012) and increased immune-inflammatory activity, in association with raised levels of glutamate, which regulates tryptophan catabolites (Maes and Anderson, 2016).
ATPase activities play a key role in protecting neurons from excitotoxic insults (Hattori et al., 1998). Hattori and colleagues showed reduced NKA activity in postmortem AD patients' brains (77.2% of the controls) with no change in Mg2+-ATPase activities (Hattori et al., 1998). The protein levels of alpha and beta subunits were also decreased in AD brains (Hattori et al., 1998). However, although this study showed a clear reduction of NKA activity in the frontal lobes of AD brains (Hattori et al., 1998), a previous study found no change in NKA enzyme activity in 4 AD brains vs. 5 control brains (Liguri et al., 1990).
In an in vitro study, the acute administration of Aβ25−35 or Aβ1−40 oligomers in rat hippocampal neurons resulted in lower NKA activity (Mark et al., 1995), suggesting a role of Aβ toxicity in the NKA activity impairment. Also, a disruption in NKA activity leads to Na+ intracellular accumulation, increasing the influx of calcium through voltage-dependent calcium channels (Mark et al., 1995), suggesting an NKA role in AD cellular toxicity and apoptosis (Hattori et al., 1998). In an AD model, the APP+PS1 transgenic mouse, a decrease in hippocampal NKA activity and protein levels is also evident (Dickey et al., 2005), further implicating a role for Aβ regulation of NKA in AD.
Supporting the NKA dysfunction in AD, Vitvitsky and colleagues showed an imbalance of Na+ and K+ ion concentrations in both postmortem AD brain tissue and in vitro primary astrocytes treated with Aβ25−35 or Aβ1−40peptides (Vitvitsky et al., 2012). The electrochemical Na+ gradient maintained by NKA is the driving force for glutamate re-uptake by EAATs (Rose et al., 2009). In the astrocyte cell culture, ion homeostasis disruption was associated with reduced levels of NKA and Na+-dependent EAATs (Vitvitsky et al., 2012). Furthermore, homocysteine, a protein involved in many CNS disorders, including Parkinson's disease (Kuhn et al., 1998) and AD (Gallucci et al., 2004), was shown to reduce NKA activity and consequently impair glutamate reuptake in the rat hippocampus (Machado et al., 2011). Hence, NKA impairment associated with glutamate signaling imbalance may contribute to the pathophysiology of AD and other neurodegenerative diseases by significantly deregulating membrane transport, brain electrophysiological activity and other important cellular processes (Vitvitsky et al., 2012). Huntington's disease (HD) is another neurodegenerative disorder associated with cognitive decline and synapse impairment (DiFiglia et al., 1995; Smith et al., 2005; Rozas et al., 2010; Nithianantharajah and Hannan, 2013; Valencia et al., 2013). Many studies show glutamate signaling to be defective in HD, which is proposed to play a role in its pathophysiology (Coyle and Schwarcz, 1976; Beal et al., 1986; Zeron et al., 2002; Shehadeh et al., 2006). In Hdh140Q∕140Q, a knock-in mouse model of HD, a reduction of NKA and glutamate transporters, VGlut1 and Vglut2, by more than 30% is evident at 12 months vs. controls (Valencia et al., 2010, 2013).
Amyotrophic lateral sclerosis (ALS) is an age-related fatal disease characterized by progressive motoneuron degeneration. The loss of Cu/Zn superoxide dismutase 1 (SOD1) activity, an antioxidant enzyme, following increased oxidative stress, is widely thought to have a role in the etiology and/or course of ALS (Julien, 2001). Other possible contributing factors include increases in iNOS (Almer et al., 1999), glutamate excitotoxicity due to EAATs dysfunction (Rothstein et al., 1995; Bruijn et al., 1997; Trotti et al., 1999) and mitochondrial defect (Beal, 1995). Importantly, Ellis et al. showed that the NO-sGC-cGMP pathway was significantly impaired in the transgenic SOD1 ALS murine model, with this cascade being unable to modulate NKA activity (Ellis et al., 2003). Furthermore, in this study, a marked reduction in NKA activity accompanied by a decrease in the protein levels of the α subunits was evident. As to whether such changes are causal or a consequence of other pathophysiological processes in ALS requires further investigation (Ellis et al., 2003).
NKA is present in the membranes of most eukaryotic cells and acts as ion pump. NKA can be modulated by hormones and neurotransmitters, allowing these factors to regulate NKA's diverse important effects, including in the regulation of neurotransmission. NKA can also act as a receptor for steroids, as typified by the effects of OUA, with this being another mechanism whereby it contributes to the regulation of many essential cellular functions, centrally and peripherally. Changes in NKA activity, as occurs in aging as well as when arising from mutations, will play a role in a host of CNS diseases, partly via deficits in energy and glutamatergic regulation. Central energy deficiency has been proposed to be a key factor in many, currently poorly managed, neurodegenerative diseases, which are linked to changes in brain metabolism and increased levels of apoptosis. Given that NKA is fundamental for optimizing central synaptic functioning, as well as energy regulation, its further study, including its environmental, epigenetic and genetic regulation, are likely to be important in the development of new pharmacological and non-pharmaceutical treatments for a host of medical conditions that are currently poorly managed, as well as for the study and regulation of aging per se.
Conceived and designed the manuscript: EK, LQ, and CS. Wrote the manuscript: AO, AV, PK, JL, and LQ have contributed equally to this work. Final revision: LQ, EK, CS, AO, PK, AV, JL.
The authors declare that the research was conducted in the absence of any commercial or financial relationships that could be construed as a potential conflict of interest.
AO, AV, JL, and PK are supported by Ph.D. fellowship from Fundação de Amparo à Pesquisa do Estado de São Paulo (FAPESP). CS and LQ are research fellows of Conselho Nacional de Desenvolvimento Científico e Tecnológico (CNPq). This publication was made possible by Grants from FAPESP to CS; FAPESP Young Investigators Grants to EK; CNPq to CS and LQ; and the Neuroscience Research Suport Centers (NAPNA). We thank George Anderson, CRC Scotland and London for English Editing.
Abushik, P. A., Sibarov, D. A., Eaton, M. J., Skatchkov, S. N., and Antonov, S. M. (2013). Kainate-induced calcium overload of cortical neurons in vitro: dependence on expression of AMPAR GluA2-subunit and down-regulation by subnanomolar ouabain. Cell Calcium 54, 95–104. doi: 10.1016/j.ceca.2013.05.002
Aitta-aho, T., Möykkynen, T. P., Panhelainen, A. E., Vekovischeva, O. Y., Bäckström, P., and Korpi, E. R. (2012). Importance of GluA1 subunit-containing AMPA glutamate receptors for morphine state-dependency. PLoS ONE 7:e38325. doi: 10.1371/journal.pone.0038325
Akera, T., and Brody, T. M. (1977). The role of Na+, K+-ATPase in the inotropic action of digitalis. Pharmacol. Rev. 29, 187–220.
Akera, T., Hook, J. B., Tobin, T., and Brody, T. M. (1972). Cardiac glycoside sensitivity of (Na + +K +)-activated ATPase in new-born rats. Res. Commun. Chem. Pathol. Pharmacol. 4, 699–706.
Akkuratov, E. E., Lopacheva, O. M., Kruusmägi, M., Lopachev, A. V., Shah, Z. A., Boldyrev, A. A., et al. (2015). Functional interaction between Na/K-ATPase and NMDA receptor in cerebellar neurons. Mol. Neurobiol. 52, 1726–1734. doi: 10.1007/s12035-014-8975-3
Almer, G., Vukosavic, S., Romero, N., and Przedborski, S. (1999). Inducible nitric oxide synthase up-regulation in a transgenic mouse model of familial amyotrophic lateral sclerosis. J. Neurochem. 72, 2415–2425. doi: 10.1046/j.1471-4159.1999.0722415.x
Alvarsson, A., Zhang, X., Stan, T. L., Schintu, N., Kadkhodaei, B., Millan, M. J., et al. (2015). Modulation by trace amine-associated receptor 1 of experimental Parkinsonism, L-DOPA responsivity, and glutamatergic neurotransmission. J. Neurosci. 35, 14057–14069. doi: 10.1523/JNEUROSCI.1312-15.2015
Amara, S. G., and Fontana, A. C. (2002). Excitatory amino acid transporters: keeping up with glutamate. Neurochem. Int. 41, 313–318. doi: 10.1016/S0197-0186(02)00018-9
Anderson, C. M., and Swanson, R. A. (2000). Astrocyte glutamate transport: review of properties, regulation, and physiological functions. Glia 32, 1–14. doi: 10.1002/1098-1136(200010)32:1<1::AID-GLIA10>3.0.CO;2-W
Anselm, I. A., Sweadner, K. J., Gollamudi, S., Ozelius, L. J., and Darras, B. T. (2009). Rapid-onset dystonia-parkinsonism in a child with a novel atp1a3 gene mutation. Neurology 73, 400–401. doi: 10.1212/WNL.0b013e3181b04acd
Aperia, A. C., Akkuratov, E. E., Fontana, J. M., and Brismar, H. (2016). Na+-K+-ATPase, a new class of plasma membrane receptors. Am. J. Physiol. Cell Physiol. 310, C491–C495. doi: 10.1152/ajpcell.00359.2015
Aperia, A., Ibarra, F., Svensson, L. B., Klee, C., and Greengard, P. (1992). Calcineurin mediates alpha-adrenergic stimulation of Na+, K(+)-ATPase activity in renal tubule cells. Proc. Natl. Acad. Sci. U.S.A. 89, 7394–7397. doi: 10.1073/pnas.89.16.7394
Arnaud-Batista, F. J., Costa, G. T., Oliveira, I. M., Costa, P. P., Santos, C. F., Fonteles, M. C., et al. (2012). Natriuretic effect of bufalin in isolated rat kidneys involves activation of the Na+-K+-ATPase-Src kinase pathway. Am. J. Physiol. Renal Physiol. 302, F959–F966. doi: 10.1152/ajprenal.00130.2011
Aroniadou-Anderjaska, V., Fritsch, B., Qashu, F., and Braga, M. F. (2008). Pathology and pathophysiology of the amygdala in epileptogenesis and epilepsy. Epilepsy Res. 78, 102–116. doi: 10.1016/j.eplepsyres.2007.11.011
Asmus, F., and Gasser, T. (2010). Dystonia-plus syndromes. Eur. J. Neurol. 17 (Suppl. 1), 37–45. doi: 10.1111/j.1468-1331.2010.03049.x
Azizan, E. A., Poulsen, H., Tuluc, P., Zhou, J., Clausen, M. V., Lieb, A., et al. (2013). Somatic mutations in ATP1A1 and CACNA1D underlie a common subtype of adrenal hypertension. Nat. Genet. 45, 1055–1060. doi: 10.1038/ng.2716
Barbano, R. L., Hill, D. F., Snively, B. M., Light, L. S., Boggs, N., McCall, W. V., et al. (2012). New triggers and non-motor findings in a family with rapid-onset dystonia-parkinsonism. Parkinsonism Relat. Disord. 18, 737–741. doi: 10.1016/j.parkreldis.2012.03.020
Bassan, M., Liu, H., Madsen, K. L., Armsen, W., Zhou, J., Desilva, T., et al. (2008). Interaction between the glutamate transporter GLT1b and the synaptic PDZ domain protein PICK1. Eur. J. Neurosci. 27, 66–82. doi: 10.1111/j.1460-9568.2007.05986.x
Bauer, D. E., Jackson, J. G., Genda, E. N., Montoya, M. M., Yudkoff, M., and Robinson, M. B. (2012). The glutamate transporter, GLAST, participates in a macromolecular complex that supports glutamate metabolism. Neurochem. Int. 61, 566–574. doi: 10.1016/j.neuint.2012.01.013
Beal, M. F. (1995). Aging, energy, and oxidative stress in neurodegenerative diseases. Ann. Neurol. 38, 357–366. doi: 10.1002/ana.410380304
Beal, M. F., Kowall, N. W., Ellison, D. W., Mazurek, M. F., Swartz, K. J., and Martin, J. B. (1986). Replication of the neurochemical characteristics of Huntington's disease by quinolinic acid. Nature 321, 168–171. doi: 10.1038/321168a0
Beguin, P., Beggah, A. T., Chibalin, A. V., Burgener-Kairuz, P., Jaisser, F., Mathews, P. M., et al. (1994). Phosphorylation of the Na,K-ATPase alpha-subunit by protein kinase A and C in vitro and in intact cells. Identification of a novel motif for PKC-mediated phosphorylation. J. Biol. Chem. 269, 24437–24445.
Behrens, P. F., Franz, P., Woodman, B., Lindenberg, K. S., and Landwehrmeyer, G. B. (2002). Impaired glutamate transport and glutamate-glutamine cycling: downstream effects of the Huntington mutation. Brain 125(Pt 8), 1908–1922. doi: 10.1093/brain/awf180
Berl, S., Lajtha, A., and Waelsch, H. (1961). Amino acid and protein metabolism. VI. Cerebral compartments of glutamic acid metabolism. J. Neurochem. 7, 186–197. doi: 10.1111/j.1471-4159.1961.tb13503.x
Berman, R. M., Cappiello, A., Anand, A., Oren, D. A., Heninger, G. R., Charney, D. S., et al. (2000). Antidepressant effects of ketamine in depressed patients. Biol. Psychiatry 47, 351–354. doi: 10.1016/S0006-3223(99)00230-9
Bersier, M. G., and Rodríguez de Lores Arnaiz, G. (2009). Intracerebroventricular administration of ouabain to rats changes the expression of NMDA receptor subunits in cerebral cortex and hippocampus. Neurochem. Res. 34, 1650–1657. doi: 10.1007/s11064-009-9956-1
Bertorello, A., and Aperia, A. (1989). Na+-K+-ATPase is an effector protein for protein kinase C in renal proximal tubule cells. Am. J. Physiol. 256(2 Pt 2), F370–F373.
Bertorello, A. M., Aperia, A., Walaas, S. I., Nairn, A. C., and Greengard, P. (1991). Phosphorylation of the catalytic subunit of Na+, K(+)-ATPase inhibits the activity of the enzyme. Proc. Natl. Acad. Sci. U.S.A. 88, 11359–11362. doi: 10.1073/pnas.88.24.11359
Beuschlein, F., Boulkroun, S., Osswald, A., Wieland, T., Nielsen, H. N., Lichtenauer, U. D., et al. (2013). Somatic mutations in ATP1A1 and ATP2B3 lead to aldosterone-producing adenomas and secondary hypertension. Nat. Genet. 45, 440–4, 4e1–2. doi: 10.1038/ng.2550
Bianchin, M. M., Londero, R. G., Lima, J. E., and Bigal, M. E. (2010). Migraine and epilepsy: a focus on overlapping clinical, pathophysiological, molecular, and therapeutic aspects. Curr. Pain Headache Rep. 14, 276–283. doi: 10.1007/s11916-010-0121-y
Blanco, G. (2005). Na,K-ATPase subunit heterogeneity as a mechanism for tissue-specific ion regulation. Semin. Nephrol. 25, 292–303. doi: 10.1016/j.semnephrol.2005.03.004
Blanco, G., DeTomaso, A. W., Koster, J., Xie, Z. J., and Mercer, R. W. (1994). The alpha-subunit of the Na, K-ATPase has catalytic activity independent of the beta-subunit. J. Biol. Chem. 269, 23420–23425.
Blanco, G., and Mercer, R. W. (1998). Isozymes of the Na-K-ATPase: heterogeneity in structure, diversity in function. Am. J. Physiol. 275(5 Pt 2), F633–F650.
Blanco, G., Sánchez, G., Melton, R. J., Tourtellotte, W. G., and Mercer, R. W. (2000). The alpha4 isoform of the Na,K-ATPase is expressed in the germ cells of the testes. J. Histochem. Cytochem. 48, 1023–1032. doi: 10.1177/002215540004800801
Blanco-Arias, P., Einholm, A. P., Mamsa, H., Concheiro, C., Gutiérrez-de-Terán, H., Romero, J., et al. (2009). A C-terminal mutation of ATP1A3 underscores the crucial role of sodium affinity in the pathophysiology of rapid-onset dystonia-parkinsonism. Hum. Mol. Genet. 18, 2370–2377. doi: 10.1093/hmg/ddp170
Bliss, T. V., and Collingridge, G. L. (1993). A synaptic model of memory: long-term potentiation in the hippocampus. Nature 361, 31–39. doi: 10.1038/361031a0
Boelman, C., Lagman-Bartolome, A. M., MacGregor, D. L., McCabe, J., Logan, W. J., and Minassian, B. A. (2014). Identical ATP1A3 mutation causes alternating hemiplegia of childhood and rapid-onset dystonia parkinsonism phenotypes. Pediatr. Neurol. 51, 850–853. doi: 10.1016/j.pediatrneurol.2014.08.015
Bolay, H., Reuter, U., Dunn, A. K., Huang, Z., Boas, D. A., and Moskowitz, M. A. (2002). Intrinsic brain activity triggers trigeminal meningeal afferents in a migraine model. Nat. Med. 8, 136–142. doi: 10.1038/nm0202-136
Boldyrev, A. A., Bulygina, E. R., Kramarenko, G. G., and Vanin, A. F. (1997). Effect of nitroso compounds on Na/K-ATPase. Biochim. Biophys. Acta 1321, 243–251. doi: 10.1016/S0005-2728(97)00053-4
Bonnet, C. S., Williams, A. S., Gilbert, S. J., Harvey, A. K., Evans, B. A., and Mason, D. J. (2015). AMPA/kainate glutamate receptors contribute to inflammation, degeneration and pain related behaviour in inflammatory stages of arthritis. Ann. Rheum. Dis. 74, 242–251. doi: 10.1136/annrheumdis-2013-203670
Bøttger, P., Glerup, S., Gesslein, B., Illarionova, N. B., Isaksen, T. J., Heuck, A., et al. (2016). Glutamate-system defects behind psychiatric manifestations in a familial hemiplegic migraine type 2 disease-mutation mouse model. Sci. Rep. 6, 22047. doi: 10.1038/srep22047
Bowyer, S. M., Aurora, K. S., Moran, J. E., Tepley, N., and Welch, K. M. (2001). Magnetoencephalographic fields from patients with spontaneous and induced migraine aura. Ann. Neurol. 50, 582–587. doi: 10.1002/ana.1293
Brashear, A., Mink, J. W., Hill, D. F., Boggs, N., McCall, W. V., Stacy, M. A., et al. (2012). ATP1A3 mutations in infants: a new rapid-onset dystonia-Parkinsonism phenotype characterized by motor delay and ataxia. Dev. Med. Child Neurol. 54, 1065–1067. doi: 10.1111/j.1469-8749.2012.04421.x
Brines, M. L., and Robbins, R. J. (1992). Inhibition of alpha 2/alpha 3 sodium pump isoforms potentiates glutamate neurotoxicity. Brain Res. 591, 94–102. doi: 10.1016/0006-8993(92)90982-F
Bristot Silvestrin, R., Bambini-Junior, V., Galland, F., Daniele Bobermim, L., Quincozes-Santos, A., Torres Abib, R., et al. (2013). Animal model of autism induced by prenatal exposure to valproate: altered glutamate metabolism in the hippocampus. Brain Res. 1495, 52–60. doi: 10.1016/j.brainres.2012.11.048
Broude, N. E., Modyanov, N. N., Monastyrskaya, G. S., and Sverdlov, E. D. (1989). Advances in Na+, K+-ATPase studies: from protein to gene and back to protein. FEBS Lett. 257, 1–9. doi: 10.1016/0014-5793(89)81773-9
Bruijn, L. I., Becher, M. W., Lee, M. K., Anderson, K. L., Jenkins, N. A., Copeland, N. G., et al. (1997). ALS-linked SOD1 mutant G85R mediates damage to astrocytes and promotes rapidly progressive disease with SOD1-containing inclusions. Neuron 18, 327–338. doi: 10.1016/S0896-6273(00)80272-X
Butterfield, D. A., Drake, J., Pocernich, C., and Castegna, A. (2001). Evidence of oxidative damage in Alzheimer's disease brain: central role for amyloid beta-peptide. Trends Mol. Med. 7, 548–554. doi: 10.1016/S1471-4914(01)02173-6
Cai, T., Wang, H., Chen, Y., Liu, L., Gunning, W. T., Quintas, L. E., et al. (2008). Regulation of caveolin-1 membrane trafficking by the Na/K-ATPase. J. Cell Biol. 182, 1153–1169. doi: 10.1083/jcb.200712022
Campos, F., Sobrino, T., Pérez-Mato, M., Rodríguez-Osorio, X., Leira, R., Blanco, M., et al. (2013). Glutamate oxaloacetate transaminase: a new key in the dysregulation of glutamate in migraine patients. Cephalalgia 33, 1148–1154. doi: 10.1177/0333102413487444
Cereijido, M., Contreras, R. G., Shoshani, L., and Larre, I. (2012). The Na+-K+-ATPase as self-adhesion molecule and hormone receptor. Am. J. Physiol. Cell Physiol. 302, C473–C481. doi: 10.1152/ajpcell.00083.2011
Charlesworth, G., Bhatia, K. P., and Wood, N. W. (2013). The genetics of dystonia: new twists in an old tale. Brain 136(Pt 7), 2017–2037. doi: 10.1093/brain/awt138
Chatton, J. Y., Marquet, P., and Magistretti, P. J. (2000). A quantitative analysis of L-glutamate-regulated Na+ dynamics in mouse cortical astrocytes: implications for cellular bioenergetics. Eur. J. Neurosci. 12, 3843–3853. doi: 10.1046/j.1460-9568.2000.00269.x
Chen, W., Mahadomrongkul, V., Berger, U. V., Bassan, M., DeSilva, T., Tanaka, K., et al. (2004). The glutamate transporter GLT1a is expressed in excitatory axon terminals of mature hippocampal neurons. J. Neurosci. 24, 1136–1148. doi: 10.1523/JNEUROSCI.1586-03.2004
Cheng, X. J., Höög, J. O., Nairn, A. C., Greengard, P., and Aperia, A. (1997). Regulation of rat Na(+)-K(+)-ATPase activity by PKC is modulated by state of phosphorylation of Ser-943 by PKA. Am. J. Physiol. 273(6 Pt 1), C1981–C1986.
Chepkova, A. N., Schönfeld, S., and Sergeeva, O. A. (2015). Age-related alterations in the expression of genes and synaptic plasticity associated with nitric oxide signaling in the mouse dorsal striatum. Neural Plast. 2015, 458123. doi: 10.1155/2015/458123
Chibalin, A. V., Pedemonte, C. H., Katz, A. I., Féraille, E., Berggren, P. O., and Bertorello, A. M. (1998). Phosphorylation of the catalyic alpha-subunit constitutes a triggering signal for Na+, K+-ATPase endocytosis. J. Biol. Chem. 273, 8814–8819. doi: 10.1074/jbc.273.15.8814
Cholet, N., Pellerin, L., Magistretti, P. J., and Hamel, E. (2002). Similar perisynaptic glial localization for the Na+, K+-ATPase alpha 2 subunit and the glutamate transporters GLAST and GLT-1 in the rat somatosensory cortex. Cereb. Cortex 12, 515–525. doi: 10.1093/cercor/12.5.515
Clapcote, S. J., Duffy, S., Xie, G., Kirshenbaum, G., Bechard, A. R., Rodacker Schack, V., et al. (2009). Mutation I810N in the alpha3 isoform of Na+, K+-ATPase causes impairments in the sodium pump and hyperexcitability in the CNS. Proc. Natl. Acad. Sci. U.S.A. 106, 14085–14090. doi: 10.1073/pnas.0904817106
Coesmans, M., Smitt, P. A., Linden, D. J., Shigemoto, R., Hirano, T., Yamakawa, Y., et al. (2003). Mechanisms underlying cerebellar motor deficits due to mGluR1-autoantibodies. Ann. Neurol. 53, 325–336. doi: 10.1002/ana.10451
Conquet, F., Bashir, Z. I., Davies, C. H., Daniel, H., Ferraguti, F., Bordi, F., et al. (1994). Motor deficit and impairment of synaptic plasticity in mice lacking mGluR1. Nature 372, 237–243. doi: 10.1038/372237a0
Cook, J. F., Hill, D. F., Snively, B. M., Boggs, N., Suerken, C. K., Haq, I., et al. (2014). Cognitive impairment in rapid-onset dystonia-parkinsonism. Movem. Disorders 29, 344–350. doi: 10.1002/mds.25790
Coyle, J. T., and Schwarcz, R. (1976). Lesion of striatal neurones with kainic acid provides a model for Huntington's chorea. Nature 263, 244–246. doi: 10.1038/263244a0
Craig, W. S., and Kyte, J. (1980). Stoichiometry and molecular weight of the minimum asymmetric unit of canine renal sodium and potassium ion-activated adenosine triphosphatase. J. Biol. Chem. 255, 6262–6269.
Cutrer, F. M., Sorensen, A. G., Weisskoff, R. M., Ostergaard, L., Sanchez del Rio, M., Lee, E. J., et al. (1998). Perfusion-weighted imaging defects during spontaneous migrainous aura. Ann. Neurol. 43, 25–31. doi: 10.1002/ana.410430108
Dalkara, T., Zervas, N. T., and Moskowitz, M. A. (2006). From spreading depression to the trigeminovascular system. Neurol. Sci. 27 (Suppl. 2), S86–S90. doi: 10.1007/s10072-006-0577-z
D'Ambrosio, R., Gordon, D. S., and Winn, H. R. (2002). Differential role of KIR channel and Na(+)/K(+)-pump in the regulation of extracellular K(+) in rat hippocampus. J. Neurophysiol. 87, 87–102. doi: 10.1152/jn.00240.2001
Dawson, V. L., Dawson, T. M., London, E. D., Bredt, D. S., and Snyder, S. H. (1991). Nitric oxide mediates glutamate neurotoxicity in primary cortical cultures. Proc. Natl. Acad. Sci. U.S.A. 88, 6368–6371. doi: 10.1073/pnas.88.14.6368
DeAndrade, M. P., Yokoi, F., van Groen, T., Lingrel, J. B., and Li, Y. (2011). Characterization of Atp1a3 mutant mice as a model of rapid-onset dystonia with parkinsonism. Behav. Brain Res. 216, 659–665. doi: 10.1016/j.bbr.2010.09.009
de Carvalho Aguiar, P., Sweadner, K. J., Penniston, J. T., Zaremba, J., Liu, L., Caton, M., et al. (2004). Mutations in the Na+/K+ -ATPase alpha3 gene ATP1A3 are associated with rapid-onset dystonia parkinsonism. Neuron 43, 169–175. doi: 10.1016/j.neuron.2004.06.028
De Fusco, M., Marconi, R., Silvestri, L., Atorino, L., Rampoldi, L., Morgante, L., et al. (2003). Haploinsufficiency of ATP1A2 encoding the Na+/K+ pump alpha2 subunit associated with familial hemiplegic migraine type 2. Nat. Genet. 33, 192–196. doi: 10.1038/ng1081
Deisseroth, K., Singla, S., Toda, H., Monje, M., Palmer, T. D., and Malenka, R. C. (2004). Excitation-neurogenesis coupling in adult neural stem/progenitor cells. Neuron 42, 535–552. doi: 10.1016/S0896-6273(04)00266-1
de Lores Arnaiz, G. R., and Bersier, M. G. (2014). Relationship between Na+, K+-ATPase and NMDA receptor at central synapses. Curr. Protein Pept. Sci. 15, 761–777. doi: 10.2174/1389203715666140903145608
Demos, M. K., van Karnebeek, C. D., Ross, C. J., Adam, S., Shen, Y., Zhan, S. H., et al. (2014). A novel recurrent mutation in ATP1A3 causes CAPOS syndrome. Orphanet J. Rare Dis. 9, 15. doi: 10.1186/1750-1172-9-15
de Oliveira Elias, M., Tavares de Lima, W., Vannuchi, Y. B., Marcourakis, T., da Silva, Z. L., Trezena, A. G., et al. (1999). Nitric oxide modulates Na+, K+-ATPase activity through cyclic GMP pathway in proximal rat trachea. Eur. J. Pharmacol. 367, 307–314. doi: 10.1016/S0014-2999(98)00928-5
De Pover, A., and Godfraind, T. (1976). Sensitivity to cardiac glycosides of (Na + K) ATPase prepared from human heart, guinea-pig heart and guinea-pig brain. Arch. Int. Pharmacodyn. Ther. 221, 339–341.
De Pover, A., and Godfraind, T. (1979). Interaction of ouabain with (Na+ + K+)ATPase from human heart and from guinea-pig heart. Biochem. Pharmacol. 28, 3051–3056. doi: 10.1016/0006-2952(79)90612-9
Deprez, L., Weckhuysen, S., Peeters, K., Deconinck, T., Claeys, K. G., Claes, L. R., et al. (2008). Epilepsy as part of the phenotype associated with ATP1A2 mutations. Epilepsia 49, 500–508. doi: 10.1111/j.1528-1167.2007.01415.x
de Sá Lima, L., Kawamoto, E. M., Munhoz, C. D., Kinoshita, P. F., Orellana, A. M., Curi, R., et al. (2013). Ouabain activates NFkappaB through an NMDA signaling pathway in cultured cerebellar cells. Neuropharmacology 73, 327–336. doi: 10.1016/j.neuropharm.2013.06.006
DeSilva, T. M., Borenstein, N. S., Volpe, J. J., Kinney, H. C., and Rosenberg, P. A. (2012). Expression of EAAT2 in neurons and protoplasmic astrocytes during human cortical development. J. Comp. Neurol. 520, 3912–3932. doi: 10.1002/cne.23130
Dhami, K. S., Churchward, M. A., Baker, G. B., and Todd, K. G. (2013). Fluoxetine and citalopram decrease microglial release of glutamate and D-serine to promote cortical neuronal viability following ischemic insult. Mol. Cell. Neurosci. 56, 365–374. doi: 10.1016/j.mcn.2013.07.006
Dichgans, M., Freilinger, T., Eckstein, G., Babini, E., Lorenz-Depiereux, B., Biskup, S., et al. (2005). Mutation in the neuronal voltage-gated sodium channel SCN1A in familial hemiplegic migraine. Lancet 366, 371–377. doi: 10.1016/S0140-6736(05)66786-4
Dickey, C. A., Gordon, M. N., Wilcock, D. M., Herber, D. L., Freeman, M. J., and Morgan, D. (2005). Dysregulation of Na+/K+ ATPase by amyloid in APP+PS1 transgenic mice. BMC Neurosci. 6:7. doi: 10.1186/1471-2202-6-7
DiFiglia, M., Sapp, E., Chase, K., Schwarz, C., Meloni, A., Young, C., et al. (1995). Huntingtin is a cytoplasmic protein associated with vesicles in human and rat brain neurons. Neuron 14, 1075–1081. doi: 10.1016/0896-6273(95)90346-1
Di Michele, M., Goubau, C., Waelkens, E., Thys, C., De Vos, R., Overbergh, L., et al. (2013). Functional studies and proteomics in platelets and fibroblasts reveal a lysosomal defect with increased cathepsin-dependent apoptosis in ATP1A3 defective alternating hemiplegia of childhood. J. Proteomics 86, 53–69. doi: 10.1016/j.jprot.2013.05.005
Dobretsov, M., and Stimers, J. R. (2005). Neuronal function and alpha3 isoform of the Na/K-ATPase. Front. Biosci. 10, 2373–2396. doi: 10.2741/1704
Dong, Z., Bai, Y., Wu, X., Li, H., Gong, B., Howland, J. G., et al. (2013). Hippocampal long-term depression mediates spatial reversal learning in the Morris water maze. Neuropharmacology 64, 65–73. doi: 10.1016/j.neuropharm.2012.06.027
Drouin-Ouellet, J., Brownell, A. L., Saint-Pierre, M., Fasano, C., Emond, V., Trudeau, L. E., et al. (2011). Neuroinflammation is associated with changes in glial mGluR5 expression and the development of neonatal excitotoxic lesions. Glia 59, 188–199. doi: 10.1002/glia.21086
Duan, K., and Mete, O. (2015). Clinicopathologic correlates of primary aldosteronism. Arch. Pathol. Lab. Med. 139, 948–954. doi: 10.5858/arpa.2014-0156-RS
Ducros, A., Joutel, A., Vahedi, K., Cecillon, M., Ferreira, A., Bernard, E., et al. (1997). Mapping of a second locus for familial hemiplegic migraine to 1q21-q23 and evidence of further heterogeneity. Ann. Neurol. 42, 885–890. doi: 10.1002/ana.410420610
Dudek, S. M., and Bear, M. F. (1992). Homosynaptic long-term depression in area CA1 of hippocampus and effects of N-methyl-D-aspartate receptor blockade. Proc. Natl. Acad. Sci. U.S.A. 89, 4363–4367. doi: 10.1073/pnas.89.10.4363
Dunlop, J. (2006). Glutamate-based therapeutic approaches: targeting the glutamate transport system. Curr. Opin. Pharmacol. 6, 103–107. doi: 10.1016/j.coph.2005.09.004
Duric, V., Banasr, M., Stockmeier, C. A., Simen, A. A., Newton, S. S., Overholser, J. C., et al. (2013). Altered expression of synapse and glutamate related genes in post-mortem hippocampus of depressed subjects. Int. J. Neuropsychopharmacol. 16, 69–82. doi: 10.1017/S1461145712000016
Ellis, D. Z., Rabe, J., and Sweadner, K. J. (2003). Global loss of Na, K-ATPase and its nitric oxide-mediated regulation in a transgenic mouse model of amyotrophic lateral sclerosis. J. Neurosci. 23, 43–51.
Erecinska, M., and Silver, I. A. (1990). Metabolism and role of glutamate in mammalian brain. Progress Neurobiol. 35, 245–296. doi: 10.1016/0301-0082(90)90013-7
Estrada-Sánchez, A. M., Montiel, T., Segovia, J., and Massieu, L. (2009). Glutamate toxicity in the striatum of the R6/2 Huntington's disease transgenic mice is age-dependent and correlates with decreased levels of glutamate transporters. Neurobiol. Dis. 34, 78–86. doi: 10.1016/j.nbd.2008.12.017
Fernandes-Rosa, F. L., Williams, T. A., Riester, A., Steichen, O., Beuschlein, F., Boulkroun, S., et al. (2014). Genetic spectrum and clinical correlates of somatic mutations in aldosterone-producing adenoma. Hypertension 64, 354–361. doi: 10.1161/HYPERTENSIONAHA.114.03419
Foo, K., Blumenthal, L., and Man, H. Y. (2012). Regulation of neuronal bioenergy homeostasis by glutamate. Neurochem. Int. 61, 389–396. doi: 10.1016/j.neuint.2012.06.003
Forbush, B. III., Kaplan, J. H., and Hoffman, J. F. (1978). Characterization of a new photoaffinity derivative of ouabain: labeling of the large polypeptide and of a proteolipid component of the Na, K-ATPase. Biochemistry 17, 3667–3676. doi: 10.1021/bi00610a037
Fukuda, Y., Bertorello, A., and Aperia, A. (1991). Ontogeny of the regulation of Na+, K(+)-ATPase activity in the renal proximal tubule cell. Pediatr. Res. 30, 131–134. doi: 10.1203/00006450-199108000-00001
Furness, D. N., Dehnes, Y., Akhtar, A. Q., Rossi, D. J., Hamann, M., Grutle, N. J., et al. (2008). A quantitative assessment of glutamate uptake into hippocampal synaptic terminals and astrocytes: new insights into a neuronal role for excitatory amino acid transporter 2 (EAAT2). Neuroscience 157, 80–94. doi: 10.1016/j.neuroscience.2008.08.043
Gallucci, M., Zanardo, A., De Valentin, L., and Vianello, A. (2004). Homocysteine in Alzheimer disease and vascular dementia. Archiv. Gerontol. Geriatr. (Suppl. 9), 38, 195–200. doi: 10.1016/j.archger.2004.04.027
Garinis, G. A., van der Horst, G. T., Vijg, J., and Hoeijmakers, J. H. (2008). DNA damage and ageing: new-age ideas for an age-old problem. Nat. Cell Biol. 10, 1241–1247. doi: 10.1038/ncb1108-1241
Geering, K. (2006). FXYD proteins: new regulators of Na-K-ATPase. Am. J. Physiol. Renal Physiol. 290, F241–F250. doi: 10.1152/ajprenal.00126.2005
Geering, K. (2008). Functional roles of Na, K-ATPase subunits. Curr. Opin. Nephrol. Hypertens. 17, 526–532. doi: 10.1097/MNH.0b013e3283036cbf
Gegelashvili, M., Rodriguez-Kern, A., Sung, L., Shimamoto, K., and Gegelashvili, G. (2007). Glutamate transporter GLAST/EAAT1 directs cell surface expression of FXYD2/gamma subunit of Na, K-ATPase in human fetal astrocytes. Neurochem. Int. 50, 916–920. doi: 10.1016/j.neuint.2006.12.015
Genda, E. N., Jackson, J. G., Sheldon, A. L., Locke, S. F., Greco, T. M., O'Donnell, J. C., et al. (2011). Co-compartmentalization of the astroglial glutamate transporter, GLT-1, with glycolytic enzymes and mitochondria. J. Neurosci. 31, 18275–18288. doi: 10.1523/JNEUROSCI.3305-11.2011
Goadsby, P. J. (2002). New directions in migraine research. J. Clin. Neurosci. 9, 368–373. doi: 10.1054/jocn.2001.0967
Goadsby, P. J., Lipton, R. B., and Ferrari, M. D. (2002). Migraine–current understanding and treatment. N. Engl. J. Med. 346, 257–270. doi: 10.1056/NEJMra010917
Golden, W. C., and Martin, L. J. (2006). Low-dose ouabain protects against excitotoxic apoptosis and up-regulates nuclear Bcl-2 in vivo. Neuroscience 137, 133–144. doi: 10.1016/j.neuroscience.2005.10.004
Golovina, V. A., Song, H., James, P. F., Lingrel, J. B., and Blaustein, M. P. (2003). Na+ pump alpha 2-subunit expression modulates Ca2+ signaling. Am. J. Physiol. Cell Physiol. 284, C475–C486. doi: 10.1152/ajpcell.00383.2002
González-González, I. M., García-Tardon, N., Cubelos, B., Giménez, C., and Zafra, F. (2008). The glutamate transporter GLT1b interacts with the scaffold protein PSD-95. J. Neurochem. 105, 1834–1848. doi: 10.1111/j.1471-4159.2008.05281.x
Groveman, B. R., Feng, S., Fang, X. Q., Pflueger, M., Lin, S. X., Bienkiewicz, E. A., et al. (2012). The regulation of N-methyl-D-aspartate receptors by Src kinase. FEBS J. 279, 20–28. doi: 10.1111/j.1742-4658.2011.08413.x
Guimaraes, I. M., Carvalho, T. G., Ferguson, S. S., Pereira, G. S., and Ribeiro, F. M. (2015). The metabotropic glutamate receptor 5 role on motor behavior involves specific neural substrates. Mol. Brain 8, 24. doi: 10.1186/s13041-015-0113-2
Haas, L. T., Salazar, S. V., Kostylev, M. A., Um, J. W., Kaufman, A. C., and Strittmatter, S. M. (2016). Metabotropic glutamate receptor 5 couples cellular prion protein to intracellular signalling in Alzheimer's disease. Brain 139(Pt 2), 526–546. doi: 10.1093/brain/awv356
Haas, M., Askari, A., and Xie, Z. (2000). Involvement of Src and epidermal growth factor receptor in the signal-transducing function of Na+/K+-ATPase. J. Biol. Chem. 275, 27832–27837. doi: 10.1074/jbc.m002951200
Hadjikhani, N., Sanchez Del Rio, M., Wu, O., Schwartz, D., Bakker, D., Fischl, B., et al. (2001). Mechanisms of migraine aura revealed by functional MRI in human visual cortex. Proc. Natl. Acad. Sci. U.S.A. 98, 4687–4692. doi: 10.1073/pnas.071582498
Hattori, N., Kitagawa, K., Higashida, T., Yagyu, K., Shimohama, S., Wataya, T., et al. (1998). CI-ATPase and Na+/K(+)-ATPase activities in Alzheimer's disease brains. Neurosci. Lett. 254, 141–144. doi: 10.1016/S0304-3940(98)00654-5
Heinzen, E. L., Swoboda, K. J., Hitomi, Y., Gurrieri, F., Nicole, S., de Vries, B., et al. (2012). De novo mutations in ATP1A3 cause alternating hemiplegia of childhood. Nat. Genet. 44, 1030–1034. doi: 10.1038/ng.2358
Herman, M. A., and Jahr, C. E. (2007). Extracellular glutamate concentration in hippocampal slice. J. Neurosci. 27, 9736–9741. doi: 10.1523/JNEUROSCI.3009-07.2007
Hoei-Hansen, C. E., Dali, C. Í., Lyngbye, T. J., Duno, M., and Uldall, P. (2014). Alternating hemiplegia of childhood in Denmark: clinical manifestations and ATP1A3 mutation status. Eur. J. Paediatric Neurol. 18, 50–54. doi: 10.1016/j.ejpn.2013.08.007
Huang, L., Li, H., and Xie, Z. (1997). Ouabain-induced hypertrophy in cultured cardiac myocytes is accompanied by changes in expression of several late response genes. J. Mol. Cell. Cardiol. 29, 429–437. doi: 10.1006/jmcc.1996.0320
Huang, L. Q., Rowan, M. J., and Anwyl, R. (1997). mGluR II agonist inhibition of LTP induction, and mGluR II antagonist inhibition of LTD induction, in the dentate gyrus in vitro. Neuroreport 8, 687–693. doi: 10.1097/00001756-199702100-00022
Hunanyan, A. S., Fainberg, N. A., Linabarger, M., Arehart, E., Leonard, A. S., Adil, S. M., et al. (2015). Knock-in mouse model of alternating hemiplegia of childhood: behavioral and electrophysiologic characterization. Epilepsia 56, 82–93. doi: 10.1111/epi.12878
Ikeda, K., Onaka, T., Yamakado, M., Nakai, J., Ishikawa, T. O., Taketo, M. M., et al. (2003). Degeneration of the amygdala/piriform cortex and enhanced fear/anxiety behaviors in sodium pump α2 subunit (Atp1a2)-deficient mice. J. Neurosci. 23, 4667–4676.
Ikeda, K., Onimaru, H., Yamada, J., Inoue, K., Ueno, S., Onaka, T., et al. (2004). Malfunction of respiratory-related neuronal activity in Na+, K+-ATPase alpha2 subunit-deficient mice is attributable to abnormal Cl- homeostasis in brainstem neurons. J. Neurosci. 24, 10693–10701. doi: 10.1523/JNEUROSCI.2909-04.2004
Ikeda, K., Satake, S., Onaka, T., Sugimoto, H., Takeda, N., Imoto, K., et al. (2013). Enhanced inhibitory neurotransmission in the cerebellar cortex of Atp1a3-deficient heterozygous mice. J. Physiol. (Lond). 591, 3433–3449. doi: 10.1113/jphysiol.2012.247817
Illarionova, N. B., Brismar, H., Aperia, A., and Gunnarson, E. (2014). Role of Na,K-ATPase alpha1 and alpha2 isoforms in the support of astrocyte glutamate uptake. PLoS ONE 9:e98469. doi: 10.1371/journal.pone.0098469
Inoue, N., and Matsui, H. (1990). Activation of a brain type Na pump after glutamate excitation of cerebral neurons. Brain Res. 534, 309–312. doi: 10.1016/0006-8993(90)90146-3
Inoue, N., Soga, T., and Kato, T. (1999). Glutamate receptors mediate regulation of Na pump isoform activities in neurons. Neuroreport 10, 3289–3293. doi: 10.1097/00001756-199911080-00008
Ishii, A., Saito, Y., Mitsui, J., Ishiura, H., Yoshimura, J., Arai, H., et al. (2013). Identification of ATP1A3 mutations by exome sequencing as the cause of alternating hemiplegia of childhood in Japanese patients. PLoS ONE 8:e56120. doi: 10.1371/journal.pone.0056120
Jaffer, F., Avbersek, A., Vavassori, R., Fons, C., Campistol, J., Stagnaro, M., et al. (2015). Faulty cardiac repolarization reserve in alternating hemiplegia of childhood broadens the phenotype. Brain 138(Pt 10), 2859–2874. doi: 10.1093/brain/awv243
Jantzie, L. L., Talos, D. M., Jackson, M. C., Park, H. K., Graham, D. A., Lechpammer, M., et al. (2015). Developmental expression of N-methyl-D-aspartate (NMDA) receptor subunits in human white and gray matter: potential mechanism of increased vulnerability in the immature brain. Cereb. Cortex 25, 482–495. doi: 10.1093/cercor/bht246
Jen, J. C., Wan, J., Palos, T. P., Howard, B. D., and Baloh, R. W. (2005). Mutation in the glutamate transporter EAAT1 causes episodic ataxia, hemiplegia, and seizures. Neurology 65, 529–534. doi: 10.1212/01.WNL.0000172638.58172.5a
Johri, A., and Beal, M. F. (2012). Mitochondrial dysfunction in neurodegenerative diseases. J. Pharmacol. Exp. Ther. 342, 619–630. doi: 10.1124/jpet.112.192138
Jorgensen, P. L., Hakansson, K. O., and Karlish, S. J. (2003). Structure and mechanism of Na,K-ATPase: functional sites and their interactions. Annu. Rev. Physiol. 65, 817–849. doi: 10.1146/annurev.physiol.65.092101.142558
Juhaszova, M., and Blaustein, M. P. (1997). Na+ pump low and high ouabain affinity alpha subunit isoforms are differently distributed in cells. Proc. Natl. Acad. Sci. U.S.A. 94, 1800–1805. doi: 10.1073/pnas.94.5.1800
Julien, J. P. (2001). Amyotrophic lateral sclerosis. unfolding the toxicity of the misfolded. Cell 104, 581–591. doi: 10.1016/S0092-8674(01)00244-6
Kabakci, K., Isbruch, K., Schilling, K., Hedrich, K., de Carvalho Aguiar, P., Ozelius, L. J., et al. (2005). Genetic heterogeneity in rapid onset dystonia-parkinsonism: description of a new family. J. Neurol. Neurosurg. Psychiatr. 76, 860–862. doi: 10.1136/jnnp.2004.046730
Kalaydjian, A., Zandi, P. P., Swartz, K. L., Eaton, W. W., and Lyketsos, C. (2007). How migraines impact cognitive function: findings from the Baltimore, E. C. A. Neurology 68, 1417–1424. doi: 10.1212/01.wnl.0000268250.10171.b3
Kalia, L. V., Pitcher, G. M., Pelkey, K. A., and Salter, M. W. (2006). PSD-95 is a negative regulator of the tyrosine kinase Src in the NMDA receptor complex. EMBO J. 25, 4971–4982. doi: 10.1038/sj.emboj.7601342
Kanner, B. I. (2006). Structure and function of sodium-coupled GABA and glutamate transporters. J. Membr. Biol. 213, 89–100. doi: 10.1007/s00232-006-0877-5
Kawakami, K., Onaka, T., Iwase, M., Homma, I., and Ikeda, K. (2005). Hyperphagia and obesity in Na,K-ATPase alpha2 subunit-defective mice. Obes. Res. 13, 1661–1671. doi: 10.1038/oby.2005.204
Kawamoto, E. M., Lima, L. S., Munhoz, C. D., Yshii, L. M., Kinoshita, P. F., Amara, F. G., et al. (2012). Influence of N-methyl-D-aspartate receptors on ouabain activation of nuclear factor-kappaB in the rat hippocampus. J. Neurosci. Res. 90, 213–228. doi: 10.1002/jnr.22745
Kawamoto, E. M., Munhoz, C. D., Lepsch, L. B., de Sá Lima, L., Glezer, I., Markus, R. P., et al. (2008). Age-related changes in cerebellar phosphatase-1 reduce Na,K-ATPase activity. Neurobiol. Aging 29, 1712–1720. doi: 10.1016/j.neurobiolaging.2007.04.008
Khachaturian, Z. S. (1985). Diagnosis of Alzheimer's disease. Arch. Neurol. 42, 1097–1105. doi: 10.1001/archneur.1985.04060100083029
Kim, M. S., and Akera, T. (1987). O2 free radicals: cause of ischemia-reperfusion injury to cardiac Na+-K+-ATPase. Am. J. Physiol. 252(2 Pt 2), H252–H257.
Kirchmann, M., Thomsen, L. L., and Olesen, J. (2006). The CACNA1A and ATP1A2 genes are not involved in dominantly inherited migraine with aura. Am. J. Med. Genetics Part B Neuropsychiatric Genetics 141B, 250–256. doi: 10.1002/ajmg.b.30277
Kirischuk, S., Parpura, V., and Verkhratsky, A. (2012). Sodium dynamics: another key to astroglial excitability? Trends Neurosci. 35, 497–506. doi: 10.1016/j.tins.2012.04.003
Kirshenbaum, G. S., Burgess, C. R., Déry, N., Fahnestock, M., Peever, J. H., and Roder, J. C. (2014). Attenuation of mania-like behavior in Na(+),K(+)-ATPase alpha3 mutant mice by prospective therapies for bipolar disorder: melatonin and exercise. Neuroscience 260, 195–204. doi: 10.1016/j.neuroscience.2013.12.011
Kirshenbaum, G. S., Dawson, N., Mullins, J. G., Johnston, T. H., Drinkhill, M. J., Edwards, I. J., et al. (2013). Alternating hemiplegia of childhood-related neural and behavioural phenotypes in Na+,K+-ATPase alpha3 missense mutant mice. PLoS ONE 8:e60141. doi: 10.1371/journal.pone.0060141
Kleene, R., Loers, G., Langer, J., Frobert, Y., Buck, F., and Schachner, M. (2007). Prion protein regulates glutamate-dependent lactate transport of astrocytes. J. Neurosci. 27, 12331–12340. doi: 10.1523/JNEUROSCI.1358-07.2007
Kocak-Toker, N., Giris, M., Tülübas, F., Uysal, M., and Aykac-Toker, G. (2005). Peroxynitrite induced decrease in Na+, K+-ATPase activity is restored by taurine. World J. Gastroenterol. 11, 3554–3557. doi: 10.3748/wjg.v11.i23.3554
Kometiani, P., Li, J., Gnudi, L., Kahn, B. B., Askari, A., and Xie, Z. (1998). Multiple signal transduction pathways link Na+/K+-ATPase to growth-related genes in cardiac myocytes. The roles of Ras and mitogen-activated protein kinases. J. Biol. Chem. 273, 15249–15256. doi: 10.1074/jbc.273.24.15249
Krebs, H. A. (1935). Metabolism of amino-acids: The synthesis of glutamine from glutamic acid and ammonia, and the enzymic hydrolysis of glutamine in animal tissues. Biochem. J. 29, 1951–1969. doi: 10.1042/bj0291951
Krupp, J. J., Vissel, B., Thomas, C. G., Heinemann, S. F., and Westbrook, G. L. (2002). Calcineurin acts via the C-terminus of NR2A to modulate desensitization of NMDA receptors. Neuropharmacology 42, 593–602. doi: 10.1016/S0028-3908(02)00031-X
Kuhn, W., Roebroek, R., Blom, H., van Oppenraaij, D., Przuntek, H., Kretschmer, A., et al. (1998). Elevated plasma levels of homocysteine in Parkinson's disease. Eur. Neurol. 40, 225–227. doi: 10.1159/000007984
Kyte, J. (1971). Purification of the sodium- and potassium-dependent adenosine triphosphatase from canine renal medulla. J. Biol. Chem. 246, 4157–4165.
Kyte, J. (1975). Structural studies of sodium and potassium ion-activated adenosine triphosphatase. The relationship between molecular structure and the mechanism of active transport. J. Biol. Chem. 250, 7443–7449.
Lauderback, C. M., Hackett, J. M., Huang, F. F., Keller, J. N., Szweda, L. I., Markesbery, W. R., et al. (2001). The glial glutamate transporter, GLT-1, is oxidatively modified by 4-hydroxy-2-nonenal in the Alzheimer's disease brain: the role of Abeta1-42. J. Neurochem. 78, 413–416. doi: 10.1046/j.1471-4159.2001.00451.x
Lauritzen, M. (1994). Pathophysiology of the migraine aura. The spreading depression theory. Brain 117, 199–210. doi: 10.1093/brain/117.1.199
Lees, G. J., Lehmann, A., Sandberg, M., and Hamberger, A. (1990). The neurotoxicity of ouabain, a sodium-potassium ATPase inhibitor, in the rat hippocampus. Neurosci. Lett. 120, 159–162. doi: 10.1016/0304-3940(90)90027-7
Le Pira, F., Lanaia, F., Zappalà, G., Morana, R., Panetta, M. R., Reggio, E., et al. (2004). Relationship between clinical variables and cognitive performances in migraineurs with and without aura. Funct. Neurol. 19, 101–105.
Levy, L. M., Warr, O., and Attwell, D. (1998). Stoichiometry of the glial glutamate transporter GLT-1 expressed inducibly in a Chinese hamster ovary cell line selected for low endogenous Na+-dependent glutamate uptake. J. Neurosci. 18, 9620–9628.
Lewerenz, J., and Maher, P. (2015). Chronic glutamate toxicity in neurodegenerative diseases-what is the evidence? Front. Neurosci. 9:469. doi: 10.3389/fnins.2015.00469
Liang, M., Cai, T., Tian, J., Qu, W., and Xie, Z. J. (2006). Functional characterization of Src-interacting Na/K-ATPase using RNA interference assay. J. Biol. Chem. 281, 19709–19719. doi: 10.1074/jbc.M512240200
Lieberman, D. N., and Mody, I. (1994). Regulation of NMDA channel function by endogenous Ca(2+)-dependent phosphatase. Nature 369, 235–239. doi: 10.1038/369235a0
Liguri, G., Taddei, N., Nassi, P., Latorraca, S., Nediani, C., and Sorbi, S. (1990). Changes in Na+,K(+)-ATPase, Ca2(+)-ATPase and some soluble enzymes related to energy metabolism in brains of patients with Alzheimer's disease. Neurosci. Lett. 112, 338–342. doi: 10.1016/0304-3940(90)90227-Z
Lin, M. T., and Beal, M. F. (2006). Mitochondrial dysfunction and oxidative stress in neurodegenerative diseases. Nature 443, 787–795. doi: 10.1038/nature05292
Lipsky, R. H., Xu, K., Zhu, D., Kelly, C., Terhakopian, A., Novelli, A., et al. (2001). Nuclear factor kappaB is a critical determinant in N-methyl-D-aspartate receptor-mediated neuroprotection. J. Neurochem. 78, 254–264. doi: 10.1046/j.1471-4159.2001.00386.x
Liu, L., Mohammadi, K., Aynafshar, B., Wang, H., Li, D., Liu, J., et al. (2003). Role of caveolae in signal-transducing function of cardiac Na+/K+-ATPase. Am. J. Physiol. Cell Physiol. 284, C1550–C1560. doi: 10.1152/ajpcell.00555.2002
Liu, T., Konkalmatt, P. R., Yang, Y., and Jose, P. A. (2016). Gastrin decreases Na+, K+-ATPase activity via a PI3 kinase- and PKC-dependent pathway in human renal proximal tubule cells. Am. J. Physiol. Endocrinol. Metab. 310, E565–E571. doi: 10.1152/ajpendo.00360.2015
Longuemare, M. C., Rose, C. R., Farrell, K., Ransom, B. R., Waxman, S. G., and Swanson, R. A. (1999). K(+)-induced reversal of astrocyte glutamate uptake is limited by compensatory changes in intracellular Na+. Neuroscience 93, 285–292. doi: 10.1016/S0306-4522(99)00152-9
Lucas, T. F., Amaral, L. S., Porto, C. S., and Quintas, L. E. (2012). Na+/K+-ATPase alpha1 isoform mediates ouabain-induced expression of cyclin D1 and proliferation of rat sertoli cells. Reproduction 144, 737–745. doi: 10.1530/REP-12-0232
Machado, F. R., Ferreira, A. G., da Cunha, A. A., Tagliari, B., Mussulini, B. H., Wofchuk, S., et al. (2011). Homocysteine alters glutamate uptake and Na+,K+-ATPase activity and oxidative status in rats hippocampus: protection by vitamin C. Metab. Brain Dis. 26, 61–67. doi: 10.1007/s11011-011-9232-3
Maes, M., and Anderson, G. (2016). Overlapping the Tryptophan Catabolite (TRYCAT) and melatoninergic pathways in Alzheimer's Disease. Curr. Pharm. Des. 22, 1074–1085. doi: 10.2174/1381612822666151214125804
Malenka, R. C., and Bear, M. F. (2004). LTP and LTD: an embarrassment of riches. Neuron 44, 5–21. doi: 10.1016/j.neuron.2004.09.012
Manahan-Vaughan, D. (1997). Group 1 and 2 metabotropic glutamate receptors play differential roles in hippocampal long-term depression and long-term potentiation in freely moving rats. J. Neurosci. 17, 3303–3311.
Marcaida, G., Kosenko, E., Miñana, M. D., Grisolía, S., and Felipo, V. (1996). Glutamate induces a calcineurin-mediated dephosphorylation of Na+,K(+)-ATPase that results in its activation in cerebellar neurons in culture. J. Neurochem. 66, 99–104. doi: 10.1046/j.1471-4159.1996.66010099.x
Mark, R. J., Hensley, K., Butterfield, D. A., and Mattson, M. P. (1995). Amyloid beta-peptide impairs ion-motive ATPase activities: evidence for a role in loss of neuronal Ca2+ homeostasis and cell death. J. Neurosci. 15, 6239–6249.
Marks, M. J., and Seeds, N. W. (1978). A heterogeneous ouabain-ATPase interaction in mouse brain. Life Sci. 23, 2735–2744. doi: 10.1016/0024-3205(78)90654-9
Matos, M., Augusto, E., Agostinho, P., Cunha, R. A., and Chen, J. F. (2013). Antagonistic interaction between adenosine A2A receptors and Na+/K+-ATPase-alpha2 controlling glutamate uptake in astrocytes. J. Neurosci. 33, 18492–18502. doi: 10.1523/JNEUROSCI.1828-13.2013
Matsuno, H., Ohi, K., Hashimoto, R., Yamamori, H., Yasuda, Y., Fujimoto, M., et al. (2015). A naturally occurring null variant of the NMDA type glutamate receptor NR3B subunit is a risk factor of schizophrenia. PLoS ONE 10:e0116319. doi: 10.1371/journal.pone.0116319
Mattson, M. P. (2004). Pathways towards and away from Alzheimer's disease. Nature 430, 631–639. doi: 10.1038/nature02621
McGrail, K. M., Phillips, J. M., and Sweadner, K. J. (1991). Immunofluorescent localization of three Na,K-ATPase isozymes in the rat central nervous system: both neurons and glia can express more than one Na,K-ATPase. J. Neurosci. 11, 381–391.
McKee, M., Scavone, C., and Nathanson, J. A. (1994). Nitric oxide, cGMP, and hormone regulation of active sodium transport. Proc. Natl. Acad. Sci. U.S.A. 91, 12056–12060. doi: 10.1073/pnas.91.25.12056
McLennan, H. (1981). On the nature of the receptors for various excitatory amino acids in the mammalian central nervous system. Adv. Biochem. Psychopharmacol. 27, 253–262.
Mense, M., Stark, G., and Apell, H. J. (1997). Effects of free radicals on partial reactions of the Na, K-ATPase. J. Membr. Biol. 156, 63–71. doi: 10.1007/s002329900188
Mobasheri, A., Avila, J., Cózar-Castellano, I., Brownleader, M. D., Trevan, M., Francis, M. J., et al. (2000). Na+, K+-ATPase isozyme diversity; comparative biochemistry and physiological implications of novel functional interactions. Biosci. Rep. 20, 51–91. doi: 10.1023/A:1005580332144
Morin, N., Morissette, M., Grégoire, L., and Di Paolo, T. (2015). Effect of a chronic treatment with an mGlu5 receptor antagonist on brain serotonin markers in parkinsonian monkeys. Prog. Neuropsychopharmacol. Biol. Psychiatry 56, 27–38. doi: 10.1016/j.pnpbp.2014.07.006
Moseley, A. E., Lieske, S. P., Wetzel, R. K., James, P. F., He, S., Shelly, D. A., et al. (2003). The Na,K-ATPase alpha 2 isoform is expressed in neurons, and its absence disrupts neuronal activity in newborn mice. J. Biol. Chem. 278, 5317–5324. doi: 10.1074/jbc.M211315200
Munhoz, C. D., Kawamoto, E. M., de Sá Lima, L., Lepsch, L. B., Glezer, I., Marcourakis, T., et al. (2005). Glutamate modulates sodium-potassium-ATPase through cyclic GMP and cyclic GMP-dependent protein kinase in rat striatum. Cell Biochem. Funct. 23, 115–123. doi: 10.1002/cbf.1217
Muriel, P., and Sandoval, G. (2000). Nitric oxide and peroxynitrite anion modulate liver plasma membrane fluidity and Na(+)/K(+)-ATPase activity. Nitric Oxide 4, 333–342. doi: 10.1006/niox.2000.0285
Nakagawa, T., Otsubo, Y., Yatani, Y., Shirakawa, H., and Kaneko, S. (2008). Mechanisms of substrate transport-induced clustering of a glial glutamate transporter GLT-1 in astroglial-neuronal cultures. Eur. J. Neurosci. 28, 1719–1730. doi: 10.1111/j.1460-9568.2008.06494.x
Nathanson, J. A., Scavone, C., Scanlon, C., and McKee, M. (1995). The cellular Na+ pump as a site of action for carbon monoxide and glutamate: a mechanism for long-term modulation of cellular activity. Neuron 14, 781–794. doi: 10.1016/0896-6273(95)90222-8
Nesher, M., Shpolansky, U., Rosen, H., and Lichtstein, D. (2007). The digitalis-like steroid hormones: new mechanisms of action and biological significance. Life Sci. 80, 2093–2107. doi: 10.1016/j.lfs.2007.03.013
Nguyen, K. T., Buljan, V., Else, P. L., Pow, D. V., and Balcar, V. J. (2010). Cardiac glycosides ouabain and digoxin interfere with the regulation of glutamate transporter GLAST in astrocytes cultured from neonatal rat brain. Neurochem. Res. 35, 2062–2069. doi: 10.1007/s11064-010-0274-4
Nicoletti, F., Wroblewski, J. T., Novelli, A., Alho, H., Guidotti, A., and Costa, E. (1986). The activation of inositol phospholipid metabolism as a signal-transducing system for excitatory amino acids in primary cultures of cerebellar granule cells. J. Neurosci. 6, 1905–1911.
Nicoll, R. A., Oliet, S. H., and Malenka, R. C. (1998). NMDA receptor-dependent and metabotropic glutamate receptor-dependent forms of long-term depression coexist in CA1 hippocampal pyramidal cells. Neurobiol. Learn. Mem. 70, 62–72. doi: 10.1006/nlme.1998.3838
Nishi, A., Fisone, G., Snyder, G. L., Dulubova, I., Aperia, A., Nairn, A. C., et al. (1999). Regulation of Na+, K+-ATPase isoforms in rat neostriatum by dopamine and protein kinase C. J. Neurochem. 73, 1492–1501. doi: 10.1046/j.1471-4159.1999.0731492.x
Nithianantharajah, J., and Hannan, A. J. (2013). Dysregulation of synaptic proteins, dendritic spine abnormalities and pathological plasticity of synapses as experience-dependent mediators of cognitive and psychiatric symptoms in Huntington's disease. Neuroscience 251, 66–74. doi: 10.1016/j.neuroscience.2012.05.043
Noel, F., and Godfraind, T. (1984). Heterogeneity of ouabain specific binding sites and (Na+ + K+)-ATPase inhibition in microsomes from rat heart. Biochem. Pharmacol. 33, 47–53. doi: 10.1016/0006-2952(84)90369-1
Novy, J., McWilliams, E., and Sisodiya, S. M. (2014). Asystole in alternating hemiplegia with de novo ATP1A3 mutation. Eur. J. Med. Genet. 57, 37–39. doi: 10.1016/j.ejmg.2013.11.003
Oblak, A. L., Hagen, M. C., Sweadner, K. J., Haq, I., Whitlow, C. T., Maldjian, J. A., et al. (2014). Rapid-onset dystonia-parkinsonism associated with the I758S mutation of the ATP1A3 gene: a neuropathologic and neuroanatomical study of four siblings. Acta Neuropathol. 128, 81–98. doi: 10.1007/s00401-014-1279-x
Ohtani, Y., Miyata, M., Hashimoto, K., Tabata, T., Kishimoto, Y., Fukaya, M., et al. (2014). The synaptic targeting of mGluR1 by its carboxyl-terminal domain is crucial for cerebellar function. J. Neurosci. 34, 2702–2712. doi: 10.1523/JNEUROSCI.3542-13.2014
Ophoff, R. A., Terwindt, G. M., Vergouwe, M. N., van Eijk, R., Oefner, P. J., Hoffman, S. M., et al. (1996). Familial hemiplegic migraine and episodic ataxia type-2 are caused by mutations in the Ca2+ channel gene CACNL1A4. Cell 87, 543–552. doi: 10.1016/S0092-8674(00)81373-2
Ossowska, K., Wolfarth, S., Schulze, G., Wardas, J., Pietraszek, M., Lorenc-Koci, E., et al. (2001). Decline in motor functions in aging is related to the loss of NMDA receptors. Brain Res. 907, 71–83. doi: 10.1016/S0006-8993(01)02601-4
Ozelius, L. J. (2012). Clinical spectrum of disease associated with ATP1A3 mutations. Lancet Neurol. 11, 741–743. doi: 10.1016/S1474-4422(12)70185-0
Paciorkowski, A. R., McDaniel, S. S., Jansen, L. A., Tully, H., Tuttle, E., Ghoneim, D. H., et al. (2015). Novel mutations in ATP1A3 associated with catastrophic early life epilepsy, episodic prolonged apnea, and postnatal microcephaly. Epilepsia 56, 422–430. doi: 10.1111/epi.12914
Panagiotakaki, E., De Grandis, E., Stagnaro, M., Heinzen, E. L., Fons, C., Sisodiya, S., et al. (2015). Clinical profile of patients with ATP1A3 mutations in alternating hemiplegia of childhood-a study of 155 patients. Orphanet J. Rare Dis. 10, 123. doi: 10.1186/s13023-015-0335-5
Pekhletski, R., Gerlai, R., Overstreet, L. S., Huang, X. P., Agopyan, N., Slater, N. T., et al. (1996). Impaired cerebellar synaptic plasticity and motor performance in mice lacking the mGluR4 subtype of metabotropic glutamate receptor. J. Neurosci. 16, 6364–6373.
Pellerin, L., and Magistretti, P. J. (1997). Glutamate uptake stimulates Na+,K+-ATPase activity in astrocytes via activation of a distinct subunit highly sensitive to ouabain. J. Neurochem. 69, 2132–2137. doi: 10.1046/j.1471-4159.1997.69052132.x
Peng, L., Martin-Vasallo, P., and Sweadner, K. J. (1997). Isoforms of Na,K-ATPase alpha and beta subunits in the rat cerebellum and in granule cell cultures. J. Neurosci. 17, 3488–3502.
Peng, M., Huang, L., Xie, Z., Huang, W. H., and Askari, A. (1996). Partial inhibition of Na+/K+-ATPase by ouabain induces the Ca2+-dependent expressions of early-response genes in cardiac myocytes. J. Biol. Chem. 271, 10372–10378. doi: 10.1074/jbc.271.17.10372
Peng, W. F., Ding, J., Li, X., Fan, F., Zhang, Q. Q., and Wang, X. (2016). N-methyl-d-aspartate receptor NR2B subunit involved in depression-like behaviours in lithium chloride-pilocarpine chronic rat epilepsy model. Epilepsy Res. 119, 77–85. doi: 10.1016/j.eplepsyres.2015.09.013
Peres, M. F., Zukerman, E., Senne Soares, C. A., Alonso, E. O., Santos, B. F., and Faulhaber, M. H. (2004). Cerebrospinal fluid glutamate levels in chronic migraine. Cephalalgia 24, 735–739. doi: 10.1111/j.1468-2982.2004.00750.x
Perry, C. J., Reed, F., Zbukvic, I. C., Kim, J. H., and Lawrence, A. J. (2016). The metabotropic glutamate 5 receptor is necessary for extinction of cocaine-associated cues. Br. J. Pharmacol. 173, 1085–1094. doi: 10.1111/bph.13437
Peterson, C., and Cotman, C. W. (1989). Strain-dependent decrease in glutamate binding to the N-methyl-D-aspartic acid receptor during aging. Neurosci. Lett. 104, 309–313. doi: 10.1016/0304-3940(89)90594-6
Petr, G. T., Sun, Y., Frederick, N. M., Zhou, Y., Dhamne, S. C., Hameed, M. Q., et al. (2015). Conditional deletion of the glutamate transporter GLT-1 reveals that astrocytic GLT-1 protects against fatal epilepsy while neuronal GLT-1 contributes significantly to glutamate uptake into synaptosomes. J. Neurosci. 35, 5187–5201. doi: 10.1523/JNEUROSCI.4255-14.2015
Pierelli, F., Grieco, G. S., Pauri, F., Pirro, C., Fiermonte, G., Ambrosini, A., et al. (2006). A novel ATP1A2 mutation in a family with FHM type II. Cephalalgia 26, 324–328. doi: 10.1111/j.1468-2982.2006.01002.x
Pietrobon, D. (2005). Migraine: new molecular mechanisms. Neuroscientist 11, 373–386. doi: 10.1177/1073858405275554
Pietrobon, D., and Striessnig, J. (2003). Neurobiology of migraine. Nat. Rev. Neurosci. 4, 386–398. doi: 10.1038/nrn1102
Plaitakis, A., Constantakakis, E., and Smith, J. (1988). The neuroexcitotoxic amino acids glutamate and aspartate are altered in the spinal cord and brain in amyotrophic lateral sclerosis. Ann. Neurol. 24, 446–449. doi: 10.1002/ana.410240314
Porras, O. H., Ruminot, I., Loaiza, A., and Barros, L. F. (2008). Na(+)-Ca(2+) cosignaling in the stimulation of the glucose transporter GLUT1 in cultured astrocytes. Glia 56, 59–68. doi: 10.1002/glia.20589
Post, R. L., Hegyvary, C., and Kume, S. (1972). Activation by adenosine triphosphate in the phosphorylation kinetics of sodium and potassium ion transport adenosine triphosphatase. J. Biol. Chem. 247, 6530–6540.
Post, R. L., and Jolly, P. C. (1957). The linkage of sodium, potassium, and ammonium active transport across the human erythrocyte membrane. Biochim. Biophys. Acta 25, 118–128. doi: 10.1016/0006-3002(57)90426-2
Post, R. L., and Kume, S. (1973). Evidence for an aspartyl phosphate residue at the active site of sodium and potassium ion transport adenosine triphosphatase. J. Biol. Chem. 248, 6993–7000.
Post, R. L., Merritt, C. R., Kinsolving, C. R., and Albright, C. D. (1960). Membrane adenosine triphosphatase as a participant in the active transport of sodium and potassium in the human erythrocyte. J. Biol. Chem. 235, 1796–1802.
Post, R. L., Sen, A. K., and Rosenthal, A. S. (1965). A phosphorylated intermediate in adenosine triphosphate-dependent sodium and potassium transport across kidney membranes. J. Biol. Chem. 240, 1437–1445.
Potic, A., Nmezi, B., and Padiath, Q. S. (2015). CAPOS syndrome and hemiplegic migraine in a novel pedigree with the specific ATP1A3 mutation. J. Neurol. Sci. 358, 453–456. doi: 10.1016/j.jns.2015.10.002
Poulsen, F. R., Blaabjerg, M., Montero, M., and Zimmer, J. (2005). Glutamate receptor antagonists and growth factors modulate dentate granule cell neurogenesis in organotypic, rat hippocampal slice cultures. Brain Res. 1051, 35–49. doi: 10.1016/j.brainres.2005.05.050
Quintas, L. E., Pierre, S. V., Liu, L., Bai, Y., Liu, X., and Xie, Z. J. (2010). Alterations of Na+/K+-ATPase function in caveolin-1 knockout cardiac fibroblasts. J. Mol. Cell. Cardiol. 49, 525–531. doi: 10.1016/j.yjmcc.2010.04.015
Rambo, L. M., Ribeiro, L. R., Schramm, V. G., Berch, A. M., Stamm, D. N., Della-Pace, I. D., et al. (2012). Creatine increases hippocampal Na(+),K(+)-ATPase activity via NMDA-calcineurin pathway. Brain Res. Bull. 88, 553–559. doi: 10.1016/j.brainresbull.2012.06.007
Ransom, C. B., Ransom, B. R., and Sontheimer, H. (2000). Activity-dependent extracellular K+ accumulation in rat optic nerve: the role of glial and axonal Na+ pumps. J. Physiol. 522, 427–442. doi: 10.1111/j.1469-7793.2000.00427.x
Reifenberger, M. S., Arnett, K. L., Gatto, C., and Milanick, M. A. (2008). The reactive nitrogen species peroxynitrite is a potent inhibitor of renal Na-K-ATPase activity. Am. J. Physiol. Ren. Physiol. 295, F1191–F1198. doi: 10.1152/ajprenal.90296.2008
Reinés, A., Peña, C., and Rodríguez de Lores Arnaiz, G. (2001). [3H]dizocilpine binding to N-methyl-D-aspartate (NMDA) receptor is modulated by an endogenous Na+, K+-ATPase inhibitor. Comparison with ouabain. Neurochem. Int. 39, 301–310. doi: 10.1016/S0197-0186(01)00034-1
Reines, A., Pena, C., and Rodriguez de Lores Arnaiz, G. (2004). Allosteric modulation of [3H]dizocilpine binding to N-methyl-D-aspartate receptor by an endogenous Na+, K+-ATPase inhibitor: dependence on receptor activation. Brain Res. 996, 117–125. doi: 10.1016/j.brainres.2003.10.018
Reinhard, L., Tidow, H., Clausen, M. J., and Nissen, P. (2013). Na(+),K (+)-ATPase as a docking station: protein-protein complexes of the Na(+),K (+)-ATPase. Cell. Mol. Life Sci. 70, 205–222. doi: 10.1007/s00018-012-1039-9
Riganti, C., Campia, I., Kopecka, J., Gazzano, E., Doublier, S., Aldieri, E., et al. (2011). Pleiotropic effects of cardioactive glycosides. Curr. Med. Chem. 18, 872–885. doi: 10.2174/092986711794927685
Roberts, E., and Frankel, S. (1950). gamma-Aminobutyric acid in brain: its formation from glutamic acid. J. Biol. Chem. 187, 55–63.
Rogawski, M. A. (2008). Common pathophysiologic mechanisms in migraine and epilepsy. Arch. Neurol. 65, 709–714. doi: 10.1001/archneur.65.6.709
Rose, C. R., and Chatton, J. Y. (2016). Astrocyte sodium signaling and neuro-metabolic coupling in the brain. Neuroscience 323, 121–134. doi: 10.1016/j.neuroscience.2015.03.002
Rose, E. M., Koo, J. C., Antflick, J. E., Ahmed, S. M., Angers, S., and Hampson, D. R. (2009). Glutamate transporter coupling to Na,K-ATPase. J. Neurosci. 29, 8143–8155. doi: 10.1523/JNEUROSCI.1081-09.2009
Rosewich, H., Baethmann, M., Ohlenbusch, A., Gärtner, J., and Brockmann, K. (2014). A novel ATP1A3 mutation with unique clinical presentation. J. Neurol. Sci. 341, 133–135. doi: 10.1016/j.jns.2014.03.034
Rothstein, J. D., Dykes-Hoberg, M., Pardo, C. A., Bristol, L. A., Jin, L., Kuncl, R. W., et al. (1996). Knockout of glutamate transporters reveals a major role for astroglial transport in excitotoxicity and clearance of glutamate. Neuron 16, 675–686. doi: 10.1016/S0896-6273(00)80086-0
Rothstein, J. D., Van Kammen, M., Levey, A. I., Martin, L. J., and Kuncl, R. W. (1995). Selective loss of glial glutamate transporter GLT-1 in amyotrophic lateral sclerosis. Ann. Neurol. 38, 73–84. doi: 10.1002/ana.410380114
Roubergue, A., Philibert, B., Gautier, A., Kuster, A., Markowicz, K., Billette de Villemeur, T., et al. (2015). Excellent response to a ketogenic diet in a patient with alternating hemiplegia of childhood. JIMD Rep. 15, 7–12. doi: 10.1007/8904_2013_292
Rozas, J. L., Gómez-Sánchez, L., Tomás-Zapico, C., Lucas, J. J., and Fernández-Chacón, R. (2010). Presynaptic dysfunction in Huntington's disease. Biochem. Soc. Trans. 38, 488–492. doi: 10.1042/BST0380488
Russell, M. B., Iversen, H. K., and Olesen, J. (1994). Improved description of the migraine aura by a diagnostic aura diary. Cephalalgia 14, 107–117. doi: 10.1046/j.1468-2982.1994.1402107.x
Rycroft, B. K., and Gibb, A. J. (2004). Inhibitory interactions of calcineurin (phosphatase 2B) and calmodulin on rat hippocampal NMDA receptors. Neuropharmacology 47, 505–514. doi: 10.1016/j.neuropharm.2004.06.001
Sanchez, G., Nguyen, A. N., Timmerberg, B., Tash, J. S., and Blanco, G. (2006). The Na,K-ATPase alpha4 isoform from humans has distinct enzymatic properties and is important for sperm motility. Mol. Hum. Reprod. 12, 565–576. doi: 10.1093/molehr/gal062
Sarantis, K., Tsiamaki, E., Kouvaros, S., Papatheodoropoulos, C., and Angelatou, F. (2015). Adenosine A(2)A receptors permit mGluR5-evoked tyrosine phosphorylation of NR2B (Tyr1472) in rat hippocampus: a possible key mechanism in NMDA receptor modulation. J. Neurochem. 135, 714–726. doi: 10.1111/jnc.13291
Sato, T., Kamata, Y., Irifune, M., and Nishikawa, T. (1997). Inhibitory effect of several nitric oxide-generating compounds on purified Na+,K(+)-ATPase activity from porcine cerebral cortex. J. Neurochem. 68, 1312–1318. doi: 10.1046/j.1471-4159.1997.68031312.x
Scavone, C., Glezer, I., Demarchi Munhoz, C., de Sena Bernardes, C., and Pekelmann Markus, R. (2000). Influence of age on nitric oxide modulatory action on Na(+), K(+)-ATPase activity through cyclic GMP pathway in proximal rat trachea. Eur. J. Pharmacol. 388, 1–7. doi: 10.1016/S0014-2999(99)00850-X
Scavone, C., Munhoz, C. D., Kawamoto, E. M., Glezer, I., de Sá Lima, L., Marcourakis, T., et al. (2005). Age-related changes in cyclic GMP and PKG-stimulated cerebellar Na,K-ATPase activity. Neurobiol. Aging 26, 907–916. doi: 10.1016/j.neurobiolaging.2004.08.013
Schatzmann, H. J. (1953). [Cardiac glycosides as inhibitors of active potassium and sodium transport by erythrocyte membrane]. Helv. Physiol. Pharmacol. Acta 11, 346–354.
Schatzmann, H. J., and Witt, P. N. (1954). Action of k-strophanthin on potassium leakage from frog sartorius muscle. J. Pharmacol. Exp. Ther. 112, 501–508.
Schneider, J. W., Mercer, R. W., Gilmore-Hebert, M., Utset, M. F., Lai, C., Greene, A., et al. (1988). Tissue specificity, localization in brain, and cell-free translation of mRNA encoding the A3 isoform of Na+,K+-ATPase. Proc. Natl. Acad. Sci. U.S.A. 85, 284–288. doi: 10.1073/pnas.85.1.284
Schumacher, B., Garinis, G. A., and Hoeijmakers, J. H. (2008). Age to survive: DNA damage and aging. Trends Genet. 24, 77–85. doi: 10.1016/j.tig.2007.11.004
Shamraj, O. I., and Lingrel, J. B. (1994). A putative fourth Na+,K(+)-ATPase alpha-subunit gene is expressed in testis. Proc. Natl. Acad. Sci. U.S.A. 91, 12952–12956. doi: 10.1073/pnas.91.26.12952
Shan, D., Mount, D., Moore, S., Haroutunian, V., Meador-Woodruff, J. H., and McCullumsmith, R. E. (2014). Abnormal partitioning of hexokinase 1 suggests disruption of a glutamate transport protein complex in schizophrenia. Schizophr. Res. 154, 1–13. doi: 10.1016/j.schres.2014.01.028
Sheean, R. K., Lau, C. L., Shin, Y. S., O'shea, R. D., and Beart, P. M. (2013). Links between L-glutamate transporters, Na+/K+-ATPase and cytoskeleton in astrocytes: evidence following inhibition with rottlerin. Neuroscience 254, 335–346. doi: 10.1016/j.neuroscience.2013.09.043
Shehadeh, J., Fernandes, H. B., Zeron Mullins, M. M., Graham, R. K., Leavitt, B. R., Hayden, M. R., et al. (2006). Striatal neuronal apoptosis is preferentially enhanced by NMDA receptor activation in YAC transgenic mouse model of Huntington disease. Neurobiol. Dis. 21, 392–403. doi: 10.1016/j.nbd.2005.08.001
Sheldon, A. L., and Robinson, M. B. (2007). The role of glutamate transporters in neurodegenerative diseases and potential opportunities for intervention. Neurochem. Int. 51, 333–355. doi: 10.1016/j.neuint.2007.03.012
Shull, G. E., Greeb, J., and Lingrel, J. B. (1986). Molecular cloning of three distinct forms of the Na+,K+-ATPase alpha-subunit from rat brain. Biochemistry 25, 8125–8132. doi: 10.1021/bi00373a001
Shull, G. E., Schwartz, A., and Lingrel, J. B. (1985). Amino-acid sequence of the catalytic subunit of the (Na+ + K+)ATPase deduced from a complementary DNA. Nature 316, 691–695. doi: 10.1038/316691a0
Sibarov, D. A., Bolshakov, A. E., Abushik, P. A., Krivoi, I. I., and Antonov, S. M. (2012). Na+,K+-ATPase functionally interacts with the plasma membrane Na+,Ca2+ exchanger to prevent Ca2+ overload and neuronal apoptosis in excitotoxic stress. J. Pharmacol. Exp. Ther. 343, 596–607. doi: 10.1124/jpet.112.198341
Skou, J. C. (1957). The influence of some cations on an adenosine triphosphatase from peripheral nerves. Biochim. Biophys. Acta 23, 394–401. doi: 10.1016/0006-3002(57)90343-8
Skou, J. C. (1998). Nobel Lecture. The identification of the sodium pump. Biosci. Rep. 18, 155–169. doi: 10.1023/A:1020196612909
Smith, R., Brundin, P., and Li, J. Y. (2005). Synaptic dysfunction in Huntington's disease: a new perspective. Cell. Mol. Life Sci. 62, 1901–1912. doi: 10.1007/s00018-005-5084-5
Spangaro, M., Bosia, M., Zanoletti, A., Bechi, M., Cocchi, F., Pirovano, A., et al. (2012). Cognitive dysfunction and glutamate reuptake: effect of EAAT2 polymorphism in schizophrenia. Neurosci. Lett. 522, 151–155. doi: 10.1016/j.neulet.2012.06.030
Stevens, C. F., and Wang, Y. (1993). Reversal of long-term potentiation by inhibitors of haem oxygenase. Nature 364, 147–149. doi: 10.1038/364147a0
Suzuki, A., Stern, S. A., Bozdagi, O., Huntley, G. W., Walker, R. H., Magistretti, P. J., et al. (2011). Astrocyte-neuron lactate transport is required for long-term memory formation. Cell 144, 810–823. doi: 10.1016/j.cell.2011.02.018
Sweadner, K. J. (1979). Two molecular forms of (Na+ + K+)-stimulated ATPase in brain. Separation, and difference in affinity for strophanthidin. J. Biol. Chem. 254, 6060–6067.
Sweadner, K. J. (1989). Isozymes of the Na+/K+-ATPase. Biochim. Biophys. Acta 988, 185–220. doi: 10.1016/0304-4157(89)90019-1
Sweadner, K. J., and Gilkeson, R. C. (1985). Two isozymes of the Na,K-ATPase have distinct antigenic determinants. J. Biol. Chem. 260, 9016–9022.
Sweney, M. T., Newcomb, T. M., and Swoboda, K. J. (2015). The expanding spectrum of neurological phenotypes in children with ATP1A3 mutations, Alternating Hemiplegia of Childhood, Rapid-onset Dystonia-Parkinsonism, CAPOS and beyond. Pediatr. Neurol. 52, 56–64. doi: 10.1016/j.pediatrneurol.2014.09.015
Takahashi, K., Kong, Q., Lin, Y., Stouffer, N., Schulte, D. A., Lai, L., et al. (2015). Restored glial glutamate transporter EAAT2 function as a potential therapeutic approach for Alzheimer's disease. J. Exp. Med. 212, 319–332. doi: 10.1084/jem.20140413
Takaki, J., Fujimori, K., Miura, M., Suzuki, T., Sekino, Y., and Sato, K. (2012). L-glutamate released from activated microglia downregulates astrocytic L-glutamate transporter expression in neuroinflammation: the ‘collusion’ hypothesis for increased extracellular L-glutamate concentration in neuroinflammation. J. Neuroinflammation 9, 275. doi: 10.1186/1742-2094-9-275
Talpalar, A. E., and Kiehn, O. (2010). Glutamatergic mechanisms for speed control and network operation in the rodent locomotor CpG. Front. Neural Circuits 4:19. doi: 10.3389/fncir.2010.00019
Tamaru, M., Yoneda, Y., Ogita, K., Shimizu, J., and Nagata, Y. (1991). Age-related decreases of the N-methyl-D-aspartate receptor complex in the rat cerebral cortex and hippocampus. Brain Res. 542, 83–90. doi: 10.1016/0006-8993(91)91001-H
Tanaka, K. (2000). Functions of glutamate transporters in the brain. Neurosci. Res. 37, 15–19. doi: 10.1016/S0168-0102(00)00104-8
Taub, M., Springate, J. E., and Cutuli, F. (2010). Targeting of renal proximal tubule Na,K-ATPase by salt-inducible kinase. Biochem. Biophys. Res. Commun. 393, 339–344. doi: 10.1016/j.bbrc.2010.02.037
Thevenod, F., and Friedmann, J. M. (1999). Cadmium-mediated oxidative stress in kidney proximal tubule cells induces degradation of Na+/K(+)-ATPase through proteasomal and endo-/lysosomal proteolytic pathways. FASEB J. 13, 1751–1761.
Thomsen, L. L., Eriksen, M. K., Roemer, S. F., Andersen, I., Olesen, J., and Russell, M. B. (2002). A population-based study of familial hemiplegic migraine suggests revised diagnostic criteria. Brain 125. 1379–1391. doi: 10.1093/brain/awf132
Thomsen, L. L., and Olesen, J. (2004). Sporadic hemiplegic migraine. Cephalalgia 24, 1016–1023. doi: 10.1111/j.1468-2982.2004.00788.x
Tian, J., Cai, T., Yuan, Z., Wang, H., Liu, L., Haas, M., et al. (2006). Binding of Src to Na+/K+-ATPase forms a functional signaling complex. Mol. Biol. Cell 17, 317–326. doi: 10.1091/mbc.E05-08-0735
Tobin, T., and Brody, T. M. (1972). Rates of dissociation of enzyme-ouabain complexes and K 0.5 values in (Na + + K +) adenosine triphosphatase from different species. Biochem. Pharmacol. 21, 1553–1560. doi: 10.1016/0006-2952(72)90305-X
Toustrup-Jensen, M. S., Einholm, A. P., Schack, V. R., Nielsen, H. N., Holm, R., Sobrido, M. J., et al. (2014). Relationship between intracellular Na+ concentration and reduced Na+ affinity in Na+,K+-ATPase mutants causing neurological disease. J. Biol. Chem. 289, 3186–3197. doi: 10.1074/jbc.M113.543272
Trotti, D., Rolfs, A., Danbolt, N. C., Brown, R. H. Jr., and Hediger, M. A. (1999). SOD1 mutants linked to amyotrophic lateral sclerosis selectively inactivate a glial glutamate transporter. Nat. Neurosci. 2, 848. doi: 10.1038/12227
Tzingounis, A. V., and Wadiche, J. I. (2007). Glutamate transporters: confining runaway excitation by shaping synaptic transmission. Nat. Rev. Neurosci. 8, 935–947. doi: 10.1038/nrn2274
Ulate-Campos, A., Fons, C., Artuch, R., Castejón, E., Martorell, L., Ozelius, L., et al. (2014). Alternating hemiplegia of childhood with a de novo mutation in ATP1A3 and changes in SLC2A1 responsive to a ketogenic diet. Pediatr. Neurol. 50, 377–379. doi: 10.1016/j.pediatrneurol.2013.11.017
Unoki, T., Matsuda, S., Kakegawa, W., Van, N. T., Kohda, K., Suzuki, A., et al. (2012). NMDA receptor-mediated PIP5K activation to produce PI(4,5)P(2) is essential for AMPA receptor endocytosis during LTD. Neuron 73, 135–148. doi: 10.1016/j.neuron.2011.09.034
Vagin, O., Dada, L. A., Tokhtaeva, E., and Sachs, G. (2012). The Na-K-ATPase alpha(1)beta(1) heterodimer as a cell adhesion molecule in epithelia. Am. J. Physiol. Cell Physiol. 302, C1271–C1281. doi: 10.1152/ajpcell.00456.2011
Valencia, A., Reeves, P. B., Sapp, E., Li, X., Alexander, J., Kegel, K. B., et al. (2010). Mutant huntingtin and glycogen synthase kinase 3-beta accumulate in neuronal lipid rafts of a presymptomatic knock-in mouse model of Huntington's disease. J. Neurosci. Res. 88, 179–190. doi: 10.1002/jnr.22184
Valencia, A., Sapp, E., Kimm, J. S., McClory, H., Ansong, K. A., Yohrling, G., et al. (2013). Striatal synaptosomes from Hdh140Q∕140Q knock-in mice have altered protein levels, novel sites of methionine oxidation, and excess glutamate release after stimulation. J. Huntingtons Dis. 2, 459–475. doi: 10.3233/JHD-130080
Vallebuona, F., and Raiteri, M. (1995). Age-related changes in the NMDA receptor/nitric oxide/cGMP pathway in the hippocampus and cerebellum of freely moving rats subjected to transcerebral microdialysis. Eur. J. Neurosci. 7, 694–701. doi: 10.1111/j.1460-9568.1995.tb00673.x
Vasconcelos, A. R., Kinoshita, P. F., Yshii, L. M., Marques Orellana, A. M., Böhmer, A. E., de Sá Lima, L., et al. (2015). Effects of intermittent fasting on age-related changes on Na,K-ATPase activity and oxidative status induced by lipopolysaccharide in rat hippocampus. Neurobiol. Aging 36, 1914–1923. doi: 10.1016/j.neurobiolaging.2015.02.020
Végh, M. J., de Waard, M. C., van der Pluijm, I., Ridwan, Y., Sassen, M. J., van Nierop, P., et al. (2012). Synaptic proteome changes in a DNA repair deficient ercc1 mouse model of accelerated aging. J. Proteome Res. 11, 1855–1867. doi: 10.1021/pr201203m
Verret, S., and Steele, J. C. (1971). Alternating hemiplegia in childhood: a report of eight patients with complicated migraine beginning in infancy. Pediatrics 47, 675–680.
Veyrat-Durebex, C., Corcia, P., Piver, E., Devos, D., Dangoumau, A., Gouel, F., et al. (2015). Disruption of TCA cycle and glutamate metabolism identified by metabolomics in an in vitro model of amyotrophic lateral sclerosis. Mol. Neurobiol. doi: 10.1007/s12035-015-9567-6. [Epub ahead of print].
Vickery, R. M., Morris, S. H., and Bindman, L. J. (1997). Metabotropic glutamate receptors are involved in long-term potentiation in isolated slices of rat medial frontal cortex. J. Neurophysiol. 78, 3039–3046.
Viollet, L., Glusman, G., Murphy, K. J., Newcomb, T. M., Reyna, S. P., Sweney, M., et al. (2015). Alternating hemiplegia of childhood: retrospective genetic study and genotype-phenotype correlations in 187 subjects from the US AHCF registry. PLoS ONE 10:e0127045. doi: 10.1371/journal.pone.0127045
Vitvitsky, V. M., Garg, S. K., Keep, R. F., Albin, R. L., and Banerjee, R. (2012). Na+ and K+ ion imbalances in Alzheimer's disease. Biochim. Biophys. Acta 1822, 1671–1681. doi: 10.1016/j.bbadis.2012.07.004
Wang, H., Haas, M., Liang, M., Cai, T., Tian, J., Li, S., et al. (2004). Ouabain assembles signaling cascades through the caveolar Na+/K+-ATPase. J. Biol. Chem. 279, 17250–17259. doi: 10.1074/jbc.M313239200
Wang, Z., Neely, R., and Landisman, C. E. (2015). Activation of GROUP, I., and Group II metabotropic glutamate receptors causes, L. T. D., and LTP of electrical synapses in the rat thalamic reticular nucleus. J. Neurosci. 35, 7616–7625. doi: 10.1523/JNEUROSCI.3688-14.2015
Wardas, J., Pietraszek, M., Schulze, G., Ossowska, K., and Wolfarth, S. (1997). Age-related changes in glutamate receptors: an autoradiographic analysis. Pol. J. Pharmacol. 49, 401–410.
Watts, A. G., Sanchez-Watts, G., Emanuel, J. R., and Levenson, R. (1991). Cell-specific expression of mRNAs encoding Na+,K(+)-ATPase alpha- and beta-subunit isoforms within the rat central nervous system. Proc. Natl. Acad. Sci. U.S.A. 88, 7425–7429. doi: 10.1073/pnas.88.16.7425
Weigand, K. M., Messchaert, M., Swarts, H. G., Russel, F. G., and Koenderink, J. B. (2014). Alternating Hemiplegia of Childhood mutations have a differential effect on Na(+),K(+)-ATPase activity and ouabain binding. Biochim. Biophys. Acta 1842, 1010–1016. doi: 10.1016/j.bbadis.2014.03.002
Weller, C. M., Leen, W. G., Neville, B. G., Duncan, J. S., de Vries, B., Geilenkirchen, M. A., et al. (2015). A novel SLC2A1 mutation linking hemiplegic migraine with alternating hemiplegia of childhood. Cephalalgia 35, 10–15. doi: 10.1177/0333102414532379
Wenk, G. L., Walker, L. C., Price, D. L., and Cork, L. C. (1991). Loss of NMDA, but not GABA-A, binding in the brains of aged rats and monkeys. Neurobiol. Aging 12, 93–98. doi: 10.1016/0197-4580(91)90047-N
Wieronska, J. M., Zorn, S. H., Doller, D., and Pilc, A. (2016). Metabotropic glutamate receptors as targets for new antipsychotic drugs: historical perspective and critical comparative assessment. Pharmacol. Ther. 157, 10–27. doi: 10.1016/j.pharmthera.2015.10.007
Wilcox, R., Brænne, I., Brüggemann, N., Winkler, S., Wiegers, K., Bertram, L., et al. (2015). Genome sequencing identifies a novel mutation in ATP1A3 in a family with dystonia in females only. J. Neurol. 262, 187–193. doi: 10.1007/s00415-014-7547-9
Wink, D. A., Cook, J. A., Pacelli, R., DeGraff, W., Gamson, J., Liebmann, J., et al. (1996). The effect of various nitric oxide-donor agents on hydrogen peroxide-mediated toxicity: a direct correlation between nitric oxide formation and protection. Arch. Biochem. Biophys. 331, 241–248. doi: 10.1006/abbi.1996.0304
Wong, S. B., Cheng, S. J., Hung, W. C., Lee, W. T., and Min, M. Y. (2015). Rosiglitazone suppresses in vitro seizures in hippocampal slice by inhibiting presynaptic glutamate release in a model of temporal lobe epilepsy. PLoS ONE 10:e0144806. doi: 10.1371/journal.pone.0144806
Wong, V. C., and Kwong, A. K. (2015). ATP1A3 mutation in a Chinese girl with alternating hemiplegia of childhood–Potential target of treatment? Brain Dev. 37, 907–910. doi: 10.1016/j.braindev.2015.01.003
Woo, A. L., James, P. F., and Lingrel, J. B. (1999). Characterization of the fourth alpha isoform of the Na,K-ATPase. J. Membr. Biol. 169, 39–44. doi: 10.1007/PL00005899
Xie, Z., and Xie, J. (2005). The Na/K-ATPase-mediated signal transduction as a target for new drug development. Front. Biosci. 10, 3100–3109. doi: 10.2741/1766
Yamasaki, M., Okada, R., Takasaki, C., Toki, S., Fukaya, M., Natsume, R., et al. (2014). Opposing role of NMDA receptor GluN2B and GluN2D in somatosensory development and maturation. J. Neurosci. 34, 11534–11548. doi: 10.1523/JNEUROSCI.1811-14.2014
Yang, X., Gao, H., Zhang, J., Xu, X., Liu, X., Wu, X., et al. (2014). ATP1A3 mutations and genotype-phenotype correlation of alternating hemiplegia of childhood in Chinese patients. PLoS ONE 9:e97274. doi: 10.1371/journal.pone.0097274
Yankner, B. A., Lu, T., and Loerch, P. (2008). The aging brain. Annu. Rev. Pathol. 3, 41–66. doi: 10.1146/annurev.pathmechdis.2.010506.092044
Ye, Q., Lai, F., Banerjee, M., Duan, Q., Li, Z., Si, S., et al. (2013). Expression of mutant alpha1 Na/K-ATPase defective in conformational transition attenuates Src-mediated signal transduction. J. Biol. Chem. 288, 5803–5814. doi: 10.1074/jbc.M112.442608
Yokoi, M., Kobayashi, K., Manabe, T., Takahashi, T., Sakaguchi, I., Katsuura, G., et al. (1996). Impairment of hippocampal mossy fiber LTD in mice lacking mGluR2. Science 273, 645–647. doi: 10.1126/science.273.5275.645
Zahn, J. M., Poosala, S., Owen, A. B., Ingram, D. K., Lustig, A., Carter, A., et al. (2007). AGEMAP: a gene expression database for aging in mice. PLoS Genet. 3:e201. doi: 10.1371/journal.pgen.0030201
Zanotti-Fregonara, P., Vidailhet, M., Kas, A., Ozelius, L. J., Clot, F., Hindie, E., et al. (2008). [123I]-FP-CIT and [99mTc]-HMPAO single photon emission computed tomography in a new sporadic case of rapid-onset dystonia-parkinsonism. J. Neurol. Sci. 273, 148–151. doi: 10.1016/j.jns.2008.06.033
Zennaro, M. C., Boulkroun, S., and Fernandes-Rosa, F. (2015). An update on novel mechanisms of primary aldosteronism. J. Endocrinol. 224, R63–R77. doi: 10.1530/joe-14-0597
Zeron, M. M., Hansson, O., Chen, N., Wellington, C. L., Leavitt, B. R., Brundin, P., et al. (2002). Increased sensitivity to N-methyl-D-aspartate receptor-mediated excitotoxicity in a mouse model of Huntington's disease. Neuron 33, 849–860. doi: 10.1016/S0896-6273(02)00615-3
Zhang, D., Hou, Q., Wang, M., Lin, A., Jarzylo, L., Navis, A., et al. (2009). Na,K-ATPase activity regulates AMPA receptor turnover through proteasome-mediated proteolysis. J. Neurosci. 29, 4498–4511. doi: 10.1523/JNEUROSCI.6094-08.2009
Zhang, L. N., Sun, Y. J., Wang, L. X., and Gao, Z. B. (2016). Glutamate Transporters/Na(+), K(+)-ATPase Involving in the Neuroprotective Effect as a Potential Regulatory Target of Glutamate Uptake. Mol. Neurobiol. 53, 1124–1131. doi: 10.1007/s12035-014-9071-4
Zhang, X., Levy, D., Noseda, R., Kainz, V., Jakubowski, M., and Burstein, R. (2010). Activation of meningeal nociceptors by cortical spreading depression: implications for migraine with aura. J. Neurosci. 30, 8807–8814. doi: 10.1523/JNEUROSCI.0511-10.2010
Zhuo, M., Hu, Y., Schultz, C., Kandel, E. R., and Hawkins, R. D. (1994). Role of guanylyl cyclase and cGMP-dependent protein kinase in long-term potentiation. Nature 368, 635–639. doi: 10.1038/368635a0
Keywords: Na+, K+-ATPase, glutamate, aging, ATP1A2 and ATP1A3 mutations, neurodegenerative diseases
Citation: Kinoshita PF, Leite JA, Orellana AMM, Vasconcelos AR, Quintas LEM, Kawamoto EM and Scavone C (2016) The Influence of Na+, K+-ATPase on Glutamate Signaling in Neurodegenerative Diseases and Senescence. Front. Physiol. 7:195. doi: 10.3389/fphys.2016.00195
Received: 09 March 2016; Accepted: 17 May 2016;
Published: 02 June 2016.
Edited by:
Sigrid A. Langhans, Nemours/Alfred I. duPont Hospital for Children, USAReviewed by:
Habibeh Khoshbouei, Univeristy of Florida, USACopyright © 2016 Kinoshita, Leite, Orellana, Vasconcelos, Quintas, Kawamoto and Scavone. This is an open-access article distributed under the terms of the Creative Commons Attribution License (CC BY). The use, distribution or reproduction in other forums is permitted, provided the original author(s) or licensor are credited and that the original publication in this journal is cited, in accordance with accepted academic practice. No use, distribution or reproduction is permitted which does not comply with these terms.
*Correspondence: Cristoforo Scavone, Y3Jpc2Nhdm9uZUB1c3AuYnI=; Y3Jpc3RvZm9yby5zY2F2b25lQGdtYWlsLmNvbQ==
†These authors have contributed equally to this work.
Disclaimer: All claims expressed in this article are solely those of the authors and do not necessarily represent those of their affiliated organizations, or those of the publisher, the editors and the reviewers. Any product that may be evaluated in this article or claim that may be made by its manufacturer is not guaranteed or endorsed by the publisher.
Research integrity at Frontiers
Learn more about the work of our research integrity team to safeguard the quality of each article we publish.