- Division of Oncology/Hematology, Department of Internal Medicine, College of Medicine, Korea University, Seoul, South Korea
Gastric cancer and colorectal cancer are the leading cause of cancer mortality and have a dismal prognosis. The introduction of biological agents to treat these cancers has resulted in improved outcomes, and combination chemotherapy with targeted agents and conventional chemotherapeutic agents is regarded as standard therapy. Additional newly clarified mechanisms of oncogenesis and resistance to targeted agents require the development of new biologic agents. Aberrant activation of the inositide signaling pathway by a loss of function PTEN mutation or gain of function mutation/amplification of PIK3CA is an oncogenic mechanism in gastric cancer and colorectal cancer. Clinical trials with biologic agents that target the inositide signaling pathway are being performed to further improve treatment outcomes of patients with advanced gastric cancer and metastatic colorectal cancer (CRC). In this review we summarize the inositide signaling pathway, the targeted agents that inhibit abnormal activation of this signaling pathway and the clinical trials currently being performed in patients with advanced or metastatic gastric cancer and metastatic CRC using these targeted agents.
Introduction
Phosphoinositides are ubiquitous signaling molecules in eukaryotes that are modified by phosphorylation at multiple positions on phosphatidylinositol (PtdIns). PtdIns is a membrane phospholipid containing a hydrophobic diacylglycerol and water soluble inositol ring. Phosphorylation at different positions on the inositol ring results in seven phosphoinositides (Sasaki et al., 2009). The phosphoinositide signaling system is essential for regulating cellular processes, including cell proliferation, vesicle transport, and cytoskeletal remodeling. Deregulation of phosphoinositide signaling by altering the genes encoding phosphoinositide-modifying enzymes leads to many diseases, such as cancers, inflammatory bowel disease, rheumatic disease, diabetes, and others (Bunney and Katan, 2010; Raimondi and Falasca, 2012).
In this article, we focus on the phosphoinositide signaling system and possible targeted therapies in gastric cancer (GC) and colorectal cancer (CRC).
The Phosphoinositide Signaling System
The parent PtdIns synthesized in the endoplasmic reticulum is phosphorylated into several different PtdIns monophosphates in the plasma membrane. The phosphorylated PtdIns4P further undergoes phosphorylation to PtdIns(4,5)P2 by PtdIns4P-5 kinases in the plasma membrane. Subsequent conversion to PtdIns (3,4,5)P3, a key lipid with signaling functions controlling cell growth and proliferation, is carried out by PI3K. The protein kinases, Akt/protein kinase B (PKB), 3-phosphoinositide-dependent protein kinase 1 (PDK1), and BTK cooperate to transduce different downstream signaling pathways. Dephosphorylation of PtdIns (3,4,5)P3 by PtdIns-3 phosphatase (PTEN) and PtdIns-5 phosphatase generate PtdIns (4,5)P2 and PtdIns (3,4)P2, respectively(Di Paolo and De Camilli, 2006; Engelman et al., 2006; Leslie et al., 2008; Ooms et al., 2009; Bunney and Katan, 2010). Phosphorylation of Akt/PKB on threonine 308 (Thr308) and serine 473 (Ser473) results in activation of the kinase and subsequent inactivation of tuberous sclerosis complex2 (TSC2) via phosphorylation. The inactivation of TSC2 leads to proteasomal degradation of the TSC1/TSC2 protein complex which in turn activates the mammalian target of rapamycin (mTOR) activation (Inoki et al., 2002; Potter et al., 2002; Figure 1).
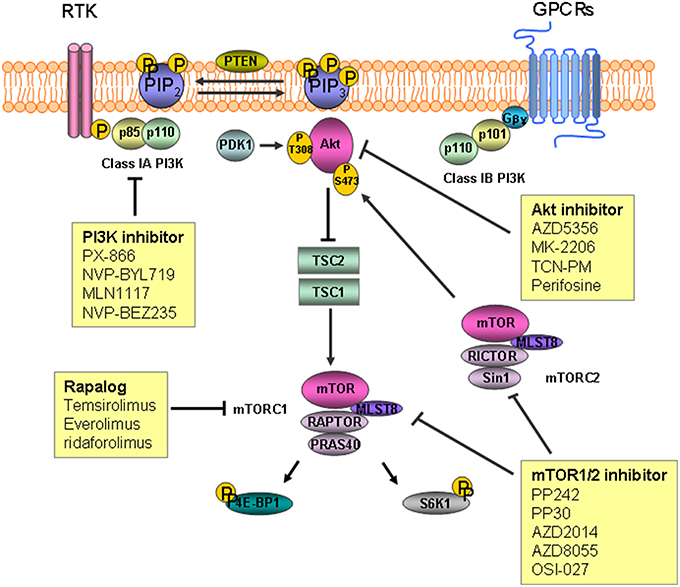
Figure 1. The inositide signaling pathway and target agents used to treat gastric cancer and colorectal cancer. Phosphatidylinosityo-4,5-bisphosphate [PtdIns(4,5)P2] is converted to phosphatidylinosityo-3,4,5-triphosphate [PtdIns(3,4,5)P3] by phosphoinositide 3-kinase (PI3K) as a result of phosphorylation. Akt/PKB is recruited to the plasma membrane followed by phosphorylation by mammalian target of rapamycin (mTOR) complex2 and 3-phosphoinositide-dependent protein kinase 1 (PDK1). Inactivation of tuberous sclerosis complex (TSC) 2 by Akt leads to degradation of TSC1/TSC2, which permits activation of mTOR. mTOR complex 1 phosphorylates downstream substrates S6 kinase 1(S6K1) and 4E-binding protein 1(4E-BP1), leading to mRNA translation initiation. RTK, receptor tyrosine kinase; GPCR, G-protein coupled receptor.
PI3K and PTEN
PI3K is a family of lipid kinases known to phosphorylate PtdIns(4,5)P2 at position 3 resulting in PtdIns(3,4,5)P3. PtdIns(3,4,5)P3 is a second messenger involved in regulating cell survival, proliferation, and growth, and its enhancement frequently leads to cancers (Engelman et al., 2006; Yuan and Cantley, 2008). PI3Ks are categorized into classes I–III based on their structures and substrate preference (Cantley, 2002). Class I PI3Ks are further grouped into two sub-families of class 1A and class1B according to their receptors. Class IA PI3Ks are heterodimeric proteins comprised of a regulatory subunit (p85α, p85β, or p55γ) and a catalytic subunit (p110α, p110β, or p110δ). Signaling from receptor tyrosine kinases (RTKs) is mediated through class IA PI3Ks by binding of the p85 regulatory subunit to phospho-tyrosine residues in the activated RTKs. The amino (N)-terminal p85-binding domain of the p110 catalytic subunit binds to the p85 regulatory subunit, and this interaction recruits the p85-p110 heterodimer to PtdIns(4,5)P2, which is a substrate located in the plasma membrane (Fruman et al., 1998; Engelman et al., 2006). Class IB PI3K is a heterodimer comprised of the p110 γ catalytic subunit and two regulatory subunits (p84 and p101). Class IB PI3K is activated by G-protein coupled receptors through interactions with the p101 regulatory subunit and the Gβγ subunit of the receptor (Stephens et al., 1997; Wymann et al., 2003). Class II PI3Ks consist of a p110-like catalytic subunit that preferentially phosphorylates PtdIns (Katso et al., 2001). Class III PI3Ks, or human vacuolar protein-sorting defective 34 (Vps34), regulates mTOR but little is known about their functions (Nobukuni et al., 2005). PTEN, which dephosphorylates PtdIns (3,4,5)P3, is a phosphatase that acts on phosphoinositides.
Akt
Serine/threonine protein kinase Akt/PKB plays a role regulating cellular growth, survival, proliferation, and metabolism. Activation of Akt downstream of PI3K is mediated by phosphorylation with protein kinases. Following phosphorylation to PtdIns(3,4,5)P3 by PI3K, Akt/PKB is recruited to the plasma membrane enriched with PtdIns(3,4,5)P3 through its N-terminal pleckstrin homology (PH) domain. PDK1 then phosphorylates the recruited Akt at Thr308 on the central kinase catalytic domain (CAT). mTOR complex2 (mTORC2) is responsible for phosphorylation of the other Ser 473 site on the carboxyl terminal extension domain (EXT). Activated AKt/PKB then phosphorylates downstream substrates such as mTOR to control cellular functions (Alessi et al., 1997; Sabatini et al., 2005; Manning and Cantley, 2007; Gonzalez and McGraw, 2009).
mTOR
mTOR is a 289-kDa serine/threonine kinase that plays a central role regulating cellular processes including cell growth and proliferation. mTOR exerts its effects by forming two distinct complexes distinguished according to the binding proteins mTORC1 and mTOR2. mTORC1 consists of mTOR, proline-rich Akt substrate 40 kDa, mammalian lethal with SEC13 protein8 (MLST8), and the regulatory-associated protein of mTOR. Activation of mTORC1 downstream of Akt/PKB subsequently phosphorylates substrates S6 kinase 1 (S6K1) and 4E-binding protein 1 (4E-BP1), which leads to initiation of mRNA translation and progression and stimulates protein synthesis. mTORC2 consists of mTOR, rapamycin-insensitive companion of mTOR, MLST8, and mammalian stress-activated protein kinase interacting protein 1. Activated mTORC2 phosphorylates Akt/PKB on Ser473, which is then fully activated with additional phosphorylation on Thr308 by PDK1 (Fingar et al., 2004; Sarbassov et al., 2005; Yang and Guan, 2007; Zoncu et al., 2011; Al-Batran et al., 2012).
The Role of Phosphoinositide Pathway Dysregulation in the Biology of Stem Cells
Tissue regeneration in the gut is ultimately regulated and sustained by intestinal stem cells (ISCs) that proliferate constantly and live long (Barker et al., 2010; Merlos-Suárez et al., 2011). In an animal study, ISCs were shown to initiate crypt expansion resulting in the formation of polyps, a precancerous neoplasm, but it is unclear which mutations in ISCs result in primary tumor initiation (He et al., 2007). Impaired bone morphogenic protein (BMP) signaling and activating mutations of Wnt signaling in ISCs can result in polyposis (Howe et al., 2001; Haramis et al., 2004; Sancho et al., 2004). There is evidence that PTEN and Akt could play crucial roles in the interaction between BMP and Wnt signals (He et al., 2007). Inactivation of PTEN was reported to cause Akt activation and activated Akt phosphorylates β-catenin, a main effector of the Wnt pathway, resulting in β-catenin's nuclear localization (Persad et al., 2001; He et al., 2007). In several organ systems including the intestine, nuclear translocation of β-catenin is considered to be crucial in activation of ISCs (Lowry et al., 2005). Bone morphogenetic protein4 (BMP4) treatment caused a substantial rise in PTEN levels in conjunction with the induction of differentiation and loss of tumorigenicity in CRC stem cells, but was not effective in stem cells harboring a PI3KCA mutation or with complete PTEN loss (Lombardo et al., 2011). These results show a coordination among BMP, PI3K/Akt, and Wnt signaling pathways in the biology of ISCs.
Genetic/Epigenetic Aberrations of Phosphoinositide Signaling System in GI Cancers
Mutations in the p110α, a catalytic subunit of class IA PI3K, are reported in 14–32% of patients with CRC (Samuels et al., 2004; Velho et al., 2005; Yuan and Cantley, 2008). Samuels et al. evaluated functional effects of the mutation of PIK3CA in CRC by inactivation of PIK3CA mutation in CRC cell lines. They reported PIK3CA mutations facilitate tumor invasion and attenuate apoptosis (Samuels et al., 2005). Studies on the prognosis of patients with CRC harboring PIK3CA mutations have reported controversial results, and the impact of the mutation has been regarded as insignificant (Cathomas, 2014). In GC, the PIK3CA mutation is reported in 4–25% (Samuels et al., 2004; Li et al., 2005; Velho et al., 2005). A study concerning the role of amplification of PIK3CA gene in GC reported a high frequency (67%) of amplification in GC and that amplification of PIK3CA is associated with poor prognosis (Shi et al., 2012) (Table 1).
Preclinical Studies of Phosphoinositide Pathway
Several studies have demonstrated that the phosphoinositide pathway is associated with proliferation, apoptosis, and metastasis. A preclinical study demonstrated activation of Akt1 promoted cell survival by showing inhibition of Akt1 phosphorylation and subsequent inhibited cell growth with LY294002, a PI3K inhibitor, in GC. In this study, dominant negative Akt also showed inhibition of proliferation and induction of cell cycle arrest in GC cells (Han et al., 2008). Another study showed upregulated PIK3CA expression is associated with lymph node metastasis in GC (Liu et al., 2010). Xing et al. investigated the effects of LY294002 on invasiveness with a GC mouse xenograft model. They found that LY294002 inhibited tumor growth and promoted apoptosis (Xing et al., 2009). The role of PIK3CA mutations was also demonstrated in CRC by showing inhibition of growth in PIK3CA mutant CRC cell lines by treatment with LY294002 (Samuels et al., 2005).
Next Druggable Target Candidate
PI3K expression of metastatic tumors in CRC is higher than that of primary tumors (Zhu et al., 2012), suggesting that PI3K might contribute to the progression and distant metastasis of CRC as in other advanced stage cancers. As activating PIK3CA mutations are observed in up to 20% of CRCs, many PI3K inhibitors have been studied (DeVita et al., 2008). Three types of PI3K inhibitors are now available for targeted therapy of solid tumors, such as Pan-class I inhibitors, isoform specific PI3K inhibitors, and dual PI3K/mTOR inhibitors (Vadas et al., 2011; Martini et al., 2013).
Pan- Class I Inhibitors
Pan-class I inhibitors are active against all p110 isoforms. These inhibitors include quecertin, the first non-specific PI3K inhibitor, wortmannin, LY294002, PX-866, NVP-BKM120, ZSTK474, BKM120, GDC0941, XL147, and BAY80-6946 (Singh et al., 2015). Wortmannin is a potent and specific PI3K inhibitor that binds covalently to Lys802 on the catalytic subunit of p110α and to Lys883 on the p110γ subunit (Powis et al., 1994; Wymann et al., 1996; Walker et al., 2000). Despite the potent inhibitory effect of wortmannin against PI3K, its short half-life, biological instability, and toxicity limits its clinical application (Yuan and Cantley, 2008). PX-866 is a biologically stable semisynthetic viridian derivative of wortmannin that shows good pharmacokinetics and has a prolonged inhibitory effect on PI3K (Ihle et al., 2004). A recent multicenter phase I trial of PX-866 reported tolerable toxicity and prolonged stable disease in patients with untreatable solid tumors including GC and CRC (Hong et al., 2012). BKM120 is an oral pyrimidine-derived inhibitor that targets class I PI3Ks but not class III PI3K or mTOR (Pecchi et al., 2010). In a phase I clinical trial, BKM120 was tolerated and demonstrated preliminary activity against advanced cancers (Bendell et al., 2012).
Isoform-Specific PI3K Inhibitors
Isoform-specific inhibitors were produced with the hope of taking advantage of the superior efficacy of pan PI3K inhibitors without the unwanted side effects. These inhibitors include NVP-BYL719, CAL-101, GSK2636771, and MLN1117 (INK1117). NVP-BYL719 is an α-specific PI3K inhibitor derived from the 2-aminothiazole class (Furet et al., 2013). A phase I clinical trial of BYL719 in combination with the heat shock protein (HSP) 90 inhibitor AUY922 in patients with advanced gastric cancer has been completed recently (NCT01613950). A phase I clinical trial of NVP-BYL719 including patients with metastatic CRC bearing PIK3CA mutations was performed (Juric et al., 2012). In this study, NVP-BYL719 had tolerable side effects and acceptable efficacy. INK1117 is a potent, α-selective PI3K inhibitor with good oral bioavailability that inhibits proliferation of tumor cell lines carrying PIK3CA mutations (Jessen et al., 2011). Results of a phase I clinical trial performed on patients with advanced solid malignancies including GC treated with MLN1117 were recently reported. The antitumor activity of this agent was demonstrated by showing an objective response in patients with breast cancer and GC (NCT01449370; Juric et al., 2015). The β-selective PI3K inhibitor GSK2636771 was developed based on the preclinical observation that selective depletion of a PI3K isoform expression reduces tumorigenesis in PTEN-deficient tumors (Rivero and Hardwicke, 2012). As loss of the PTEN is prevalent in CRCs, further assessment of GSK2636771 in patients with CRC is necessary. An in vitro study suggested that activation of PI3K plays a crucial role in resistance against a BRAF inhibitor in patients with CRC carrying the BRAF mutation (Mao et al., 2013).
Dual PI3K/mTOR Inhibitors
Pan PI3K/mTOR inhibitors block the activities of both PI3Ks and mTOR kinases by competitively binding to the ATP-binding sites. Because mTOR is structurally related to PI3Ks, ATP-competitive compounds inhibit these two kinases with equivalent potency. In comparison with mTORC1 inhibitor, dual PI3K/mTOR inhibitors could overcome loss of mTORC1-dependent negative feedback on PI3K signaling (Roper et al., 2011). mTORC1 blockade might be attenuated by resultant mTORC2-mediated activation of Akt by phosphorylation at Ser473 (Roper et al., 2011). NVP-BEZ235, NVP-BGT226, VS-5884, PI-103, XL765, GDC-0980, and PF-05212384 are dual Pan PI3K/mTOR inhibitors. In a preclinical study using a genetically engineered mouse model carrying wild-type PIK3CA with CRC, NVP-BEZ235 induced tumor regression (Roper et al., 2011). Considering the relatively low prevalence of the activating PIK3CA mutation (up to 20%), further studies should be performed on the efficacy of NVP-BEZ235 in patients with wild-type PIK3CA.
Akt Inhibitors
Akt/PKB is an essential protein kinase comprised of three isoforms, such as Akt1 (PKBα), Akt2 (PKBβ), and Akt3 (PKBγ). The Akt structure consists of three conserved domains, including an N-terminal PH domain, a CAT domain, and a C-terminal EXT domain. Akt inhibitors are classified depending on the mechanism of action, such as compounds that compete for the ATP-binding site, allosteric inhibitors, agents targeting the PH domain, and pseudosubstrate inhibitors (Kumar and Madison, 2005). Among these groups, allosteric inhibitors and ATP mimetics are the two main groups that have been investigated for clinical application. Several Akt inhibitors are currently being studied for GC, including AZD5363, MK-2206, triciribine phosphate monohydrate (TCN-PM), perifosine, GDC-0068, and GSK690693. AZD5363 is a potent ATP-competitive inhibitor of Akt and a novel pyrrolopyrimidine-derived compound that acts against all Akt isoforms (Luke et al., 2011; Davies et al., 2012). MK-2206 is an allosteric inhibitor that binds to the PH and kinase domains, which blocks transport of Akt to the membrane and it activation (Hirai et al., 2010). Results of a phase II study of MK-2206 in patients with GC have been recently released. Patients with GC and gastroesophageal junction cancer who progressed to first-line treatment were administrated MK-2206. The primary endpoint of median overall survival (OS) was 5.1 months (m) [95% confidence interval (CI), 3.7–9.4 m] and did not meet the study efficacy endpoint of 6.5 m (Ramanathan et al., 2015). In a phase I study, MK-2206 was well tolerated with evidence of Akt blockade (Yap et al., 2011). Subsequently, two phase II studies were planned. TCN-PM exerts its effect by targeting the PH domain and blocking translocation of Akt to the plasma membrane (Berndt et al., 2010). A phase I trial of TCN-PM in patients with solid tumors including gastroesophageal cancer carrying increased phosphorylated Akt (p-Akt) reported modest decreases in tumor p-AKT after TCN-PM monotherapy treatment (Garrett et al., 2011).
mTOR Inhibitors
Two kinds of mTOR inhibitors called rapalogs and mTORC1/2 inhibitors have been reported and categorized by their specificity for mTOR complexes. Rapamycin is the first mTOR inhibitor discovered. Formation of an inhibitory complex upon binding of rapamycin to the intracellular receptor FK506 binding protein 12 (FKBP12) leads to binding of the complex to the FKB12-rapamycin binding domain at the C-terminus of TOR proteins, which subsequently prevents mTOR from signaling downstream targets (Kunz and Hall, 1993; Chen et al., 1995; Choi et al., 1996; Zhou et al., 2010). Temsirolimus, everolimus, and ridaforolimus are rapalogs that have been studied for treating GC. Temsirolimus is a dihydroxymethyl propionic acid ester of rapamycin that inhibits phosphorylation of S6K1 and 4E-BP1 mediated by mTOR (Rini, 2008). A phase I trial to determine the pharmacokinetics of temsirolimus in patients with advanced cancers including GC showed an anti-tumor effect of this agent (Hidalgo et al., 2006). Everolimus is an oral formula of a rapamycin analog. A phase II trial was conducted to investigate the efficacy of everolimus combined with capecitabine in patients with refractory GC. The primary endpoint of overall response rate was 10.6% (Lee et al., 2013). In the phase III GRNITE-1 study, patients with refractory GC who had undergone at least one prior systemic chemotherapeutic trial were randomized to receive everolimus with best supportive care (BSC) or placebo plus BSC. However, the primary endpoint of significantly improved OS was not observed in the everolimus group (median OS: 5.4 vs. 4.3 m; hazard ratio, 0.9; 95% CI, 0.75–1.08; p = 0.124; Ohtsu et al., 2013). Despite the failure of meeting the primary endpoint, significant improvement in progression free survival was observed, and one of causes of the failure might have been the effect of a salvage therapy after progression to the treatment in clinical trial. mTOR1/2 inhibitors exert their effects against mTORC1 and mTORC2 at nanomolar concentrations without inhibiting other kinases. These new-generation ATP-competitive mTOR inhibitors include PP242, PP30, AZD2014, AZD8055, and OSI-027 (Feldman et al., 2009; Zhou et al., 2010).
Dual Targeted Strategy For the RAS/MEK/ERK and PI3K/Akt/mTOR Pathways
Treating patients with metastatic CRC using biologic agents combined with chemotherapeutic agents has become standard therapy. Although the epidermal growth factor receptor monoclonal antibody has brought improved outcomes in those with wild-type RAS (Fakih, 2015), the acquired resistance to the monoclonal antibody, even in patients with CRC carrying wild-type RAS, has been a treatment issue possibly due to crosstalk between signaling pathways (Chong and Jänne, 2013). Several studies have suggested the possibility of a combined therapy targeting the RAS/MEK/ERK and PI3K/Akt/mTOR pathways (Yu et al., 2008). A dual PI3K/mTOR inhibitor combined with the MEK inhibitor selumetinib produces growth-suppressive effects in patient-derived xenografts from CRC with mutated RAS (Migliardi et al., 2012). Shimizu et al. retrospectively analyzed clinical outcomes of 236 patients who received the dual targeted strategy involving the RAS/MEK/ERK and PI3K/AKT/mTOR pathways (Shimizu et al., 2012). Although the study focused on drug safety rather than efficacy, the clinical outcomes suggested that the dual targeted strategy is more effective compared with monotherapy in properly selected patients (Shimizu et al., 2012).
Potential Pharmacodynamic Markers of Inhibitors
Since PI3K pathway inhibitors have a role in physiological glucose metabolism, estimating insulin resistance could be a good pharmacodynamic marker (Engelman et al., 2006; Luo et al., 2006).
Assessment of phosphorylation of Akt at Thr308 and Ser473, 4E-BP1 at Ser65 and Thr70, ribosomal protein S6 (RPS6) at Ser240 and Ser244, or PRAS40 could offer potential as pharmacodynamic markers (Rodon et al., 2013). Quantification of these molecular pharmacodynamic biomarkers have been performed in not only tumor tissues but also surrogate tissues, like peripheral blood mononuclear cells, platelet-enriched plasma, skin, and hair, and should be feasible in the clinic (Jimeno et al., 2010; Biondo et al., 2011; Rodon et al., 2013). However, these methods have that limitation that increased RAS.RAF/ERK/mTORC1 activity could adjust phosphorylation of RPS6, 4E-BP1, or PRAS40 (Manning and Cantley, 2007).
Conclusion
Phosphoinositides are versatile and indispensable for regulating various cellular functions. The phosphoinositide signaling system can become deregulated occasionally because of mutations in genes encoding kinases or phosphatases, and consequently, becomes integral to carcinogenesis and progression of GC and CRC. Therefore, the phosphoinositide pathway is an important target for the development of anticancer drugs.
Several key steps are required to develop optimal targeted agents. A novel approach can be formulated by using tumor genotypic and molecular biologic analyses. Patients should be properly selected based on preclinical data and well standardized predictive markers for combined targeted therapy to be effective. Avoiding bias in well-planned clinical trials is also important for the success of targeted therapy.
Author Contributions
SL designed, wrote and edited the manuscript. HK wrote and edited the manuscript. SO supervised and edited the manuscript.
Conflict of Interest Statement
The authors declare that the research was conducted in the absence of any commercial or financial relationships that could be construed as a potential conflict of interest.
References
Al-Batran, S. E., Ducreux, M., and Ohtsu, A. (2012). mTOR as a therapeutic target in patients with gastric cancer. Int. J. Cancer 130, 491–496. doi: 10.1002/ijc.26396
Alessi, D. R., James, S. R., Downes, C. P., Holmes, A. B., Gaffney, P. R., Reese, C. B., et al. (1997). Characterization of a 3-phosphoinositide-dependent protein kinase which phosphorylates and activates protein kinase Bα. Curr. Biol. 7, 261–269. doi: 10.1016/S0960-9822(06)00122-9
Barker, N., Huch, M., Kujala, P., van de Wetering, M., Snippert, H. J., van Es, J. H., et al. (2010). Lgr5+ ve stem cells drive self-renewal in the stomach and build long-lived gastric units in vitro. Cell Stem Cell 6, 25–36. doi: 10.1016/j.stem.2009.11.013
Bendell, J. C., Rodon, J., Burris, H. A., de Jonge, M., Verweij, J., Birle, D., et al. (2012). Phase I, dose-escalation study of BKM120, an oral pan-Class I PI3K inhibitor, in patients with advanced solid tumors. J. Clin. Oncol. 30, 282–290. doi: 10.1200/JCO.2011.36.1360
Berndt, N., Yang, H., Trinczek, B., Betzi, S., Zhang, Z., Wu, B., et al. (2010). The Akt activation inhibitor TCN-P inhibits Akt phosphorylation by binding to the PH domain of Akt and blocking its recruitment to the plasma membrane. Cell Death Differ. 17, 1795–1804. doi: 10.1038/cdd.2010.63
Biondo, A., Yap, T., Yan, L., Patnaik, A., Fearen, I., Baird, R., et al. (2011). Phase I clinical trial of an allosteric AKT inhibitor, MK-2206, using a once weekly (QW) dose regimen in patients with advanced solid tumors. J. Clin. Oncol. 29(suppl; abstr 3037).
Bunney, T. D., and Katan, M. (2010). Phosphoinositide signalling in cancer: beyond PI3K and PTEN. Nat. Rev. Cancer 10, 342–352. doi: 10.1038/nrc2842
Cantley, L. C. (2002). The phosphoinositide 3-kinase pathway. Science 296, 1655–1657. doi: 10.1152/ajpheart.00562.2008
Chen, J., Zheng, X.-F., Brown, E. J., and Schreiber, S. L. (1995). Identification of an 11-kDa FKBP12-rapamycin-binding domain within the 289-kDa FKBP12-rapamycin-associated protein and characterization of a critical serine residue. Proc. Natl. Acad. Sci. U.S.A. 92, 4947–4951. doi: 10.1073/pnas.92.11.4947
Choi, J., Chen, J., Schreiber, S. L., and Clardy, J. (1996). Structure of the FKBP12-rapamycin complex interacting with binding domain of human FRAP. Science 273, 239–242. doi: 10.1126/science.273.5272.239
Chong, C. R., and Jänne, P. A. (2013). The quest to overcome resistance to EGFR-targeted therapies in cancer. Nat. Med. 19, 1389–1400. doi: 10.1038/nm.3388
Davies, B. R., Greenwood, H., Dudley, P., Crafter, C., Yu, D.-H., Zhang, J., et al. (2012). Preclinical pharmacology of AZD5363, an inhibitor of AKT: pharmacodynamics, antitumor activity, and correlation of monotherapy activity with genetic background. Mol. Cancer Ther. 11, 873–887. doi: 10.1158/1535-7163.MCT-11-0824-T
DeVita, V. T. Jr., Lawrence, T. S., and Rosenberg, S. A. (2008). DeVita, Hellman, and Rosenberg's Cancer: Principles & Practice of Oncology, 8th Edn. Philadelphia, PA; London: Wolters Kluwer/Lippincott Williams & Wilkins.
Di Paolo, G., and De Camilli, P. (2006). Phosphoinositides in cell regulation and membrane dynamics. Nature 443, 651–657. doi: 10.1038/nature05185
Eklöf, V., Wikberg, M. L., Edin, S., Dahlin, A. M., Jonsson, B.-A., Öberg, Å., et al. (2013). The prognostic role of KRAS, BRAF, PIK3CA and PTEN in colorectal cancer. Br. J. Cancer 108, 2153–2163. doi: 10.1038/bjc.2013.212
Engelman, J. A., Luo, J., and Cantley, L. C. (2006). The evolution of phosphatidylinositol 3-kinases as regulators of growth and metabolism. Nature Rev. Genet. 7, 606–619. doi: 10.1038/nrg1879
Fakih, M. G. (2015). Metastatic colorectal cancer: current state and future directions. J. Clin. Oncol. 33, 1809–1824. doi: 10.1200/JCO.2014.59.7633
Feldman, M. E., Apsel, B., Uotila, A., Loewith, R., Knight, Z. A., Ruggero, D., et al. (2009). Active-site inhibitors of mTOR target rapamycin-resistant outputs of mTORC1 and mTORC2. PLoS Biol. 7:e1000038. doi: 10.1371/journal.pbio.1000038
Fingar, D. C., Richardson, C. J., Tee, A. R., Cheatham, L., Tsou, C., and Blenis, J. (2004). mTOR controls cell cycle progression through its cell growth effectors S6K1 and 4E-BP1/eukaryotic translation initiation factor 4E. Mol. Cell. Biol. 24, 200–216. doi: 10.1128/MCB.24.1.200-216.2004
Fruman, D. A., Meyers, R. E., and Cantley, L. C. (1998). Phosphoinositide kinases. Annu. Rev. Biochem. 67, 481–507. doi: 10.1146/annurev.biochem.67.1.481
Furet, P., Guagnano, V., Fairhurst, R. A., Imbach-Weese, P., Bruce, I., Knapp, M., et al. (2013). Discovery of NVP-BYL719 a potent and selective phosphatidylinositol-3 kinase alpha inhibitor selected for clinical evaluation. Bioorg. Med. Chem. Lett. 23, 3741–3748. doi: 10.1016/j.bmcl.2013.05.007
Garrett, C. R., Coppola, D., Wenham, R. M., Cubitt, C. L., Neuger, A. M., Frost, T. J., et al. (2011). Phase I pharmacokinetic and pharmacodynamic study of triciribine phosphate monohydrate, a small-molecule inhibitor of AKT phosphorylation, in adult subjects with solid tumors containing activated AKT. Invest. New Drugs 29, 1381–1389. doi: 10.1007/s10637-010-9479-2
Gonzalez, E., and McGraw, T. E. (2009). The Akt kinases: isoform specificity in metabolism and cancer. Cell Cycle 8, 2502–2508. doi: 10.4161/cc.8.16.9335
Han, Z., Wu, K., Shen, H., Li, C., Han, S., Hong, L., et al. (2008). Akt1/protein kinase Bα is involved in gastric cancer progression and cell proliferation. Dig. Dis. Sci. 53, 1801–1810. doi: 10.1007/s10620-007-9824-2
Haramis, A.-P. G., Begthel, H., van den Born, M., van Es, J., Jonkheer, S., Offerhaus, G. J. A., et al. (2004). De novo crypt formation and juvenile polyposis on BMP inhibition in mouse intestine. Science 303, 1684–1686. doi: 10.1126/science.1093587
He, X. C., Yin, T., Grindley, J. C., Tian, Q., Sato, T., Tao, W. A., et al. (2007). PTEN-deficient intestinal stem cells initiate intestinal polyposis. Nat. Genet. 39, 189–198. doi: 10.1038/ng1928
Hidalgo, M., Buckner, J. C., Erlichman, C., Pollack, M. S., Boni, J. P., Dukart, G., et al. (2006). A phase I and pharmacokinetic study of temsirolimus (CCI-779) administered intravenously daily for 5 days every 2 weeks to patients with advanced cancer. Clin. Cancer Res. 12, 5755–5763. doi: 10.1158/1078-0432.CCR-06-0118
Hirai, H., Sootome, H., Nakatsuru, Y., Miyama, K., Taguchi, S., Tsujioka, K., et al. (2010). MK-2206, an allosteric Akt inhibitor, enhances antitumor efficacy by standard chemotherapeutic agents or molecular targeted drugs in vitro and in vivo. Mol. Cancer Ther. 9, 1956–1967. doi: 10.1158/1535-7163.MCT-09-1012
Hong, D. S., Bowles, D. W., Falchook, G. S., Messersmith, W. A., George, G. C., O'Bryant, C. L., et al. (2012). A multicenter phase I trial of PX-866, an oral irreversible phosphatidylinositol 3-kinase inhibitor, in patients with advanced solid tumors. Clin. Cancer Res. 18, 4173–4182. doi: 10.1158/1078-0432.CCR-12-0714
Howe, J. R., Bair, J. L., Sayed, M. G., Anderson, M. E., Mitros, F. A., Petersen, G. M., et al. (2001). Germline mutations of the gene encoding bone morphogenetic protein receptor 1A in juvenile polyposis. Nat. Genet. 28, 184–187. doi: 10.1038/88919
Ihle, N. T., Williams, R., Chow, S., Chew, W., Berggren, M. I., Paine-Murrieta, G., et al. (2004). Molecular pharmacology and antitumor activity of PX-866, a novel inhibitor of phosphoinositide-3-kinase signaling. Mol. Cancer Ther. 3, 763–772.
Iida, S., Kato, S., Ishiguro, M., Matsuyama, T., Ishikawa, T., Kobayashi, H., et al. (2012). PIK3CA mutation and methylation influences the outcome of colorectal cancer. Oncol. Lett. 3, 565–570. doi: 10.3892/ol.2011.544
Inoki, K., Li, Y., Zhu, T., Wu, J., and Guan, K.-L. (2002). TSC2 is phosphorylated and inhibited by Akt and suppresses mTOR signalling. Nat. Cell Biol. 4, 648–657. doi: 10.1038/ncb839
Jessen, K., Kessler, L., Kucharski, J., Guo, X., Staunton, J., Janes, M., et al. (eds.). (2011). A potent and selective PI3K inhibitor, INK1117, targets human cancers harboring oncogenic PIK3CA mutations, in AACRNCI-EORTC International Conference: Molecular Targets and Cancer Therapeutics (San Francisco, CA; Philadelphia, PA: American Association for Cancer Research).
Jimeno, A., Herbst, R., Falchook, G., Messersmith, W., Hecker, S., Peterson, S., et al. (eds.). (2010). Final results from a phase I, dose-escalation study of PX-866, an irreversible, pan-isoform inhibitor of PI3 kinase, in ASCO Annual Meeting Proceedings. (Chicago, IL).
Juric, D., Argiles, G., Burris, H., Gonzalez-Angulo, A., Saura, C., Quadt, C., et al. (2012). Abstract P6-10-07: Phase I study of BYL719, an alpha-specific PI3K inhibitor, in patients with PIK3CA mutant advanced solid tumors: preliminary efficacy and safety in patients with PIK3CA mutant ER-positive (ER+) metastatic breast cancer (MBC). Cancer Res. 72(24 Suppl.): P6-10-07. doi: 10.1158/0008-5472.SABCS12-P6-10-07
Juric, D., De Bono, J. S., LoRusso, P., Nemunaitis, J. J., Heath, E. I., Kwak, E. L., et al. (eds.). (2015). First-in-human, phase I, dose-escalation study of selective PI3K {alpha} isoform inhibitor MLN1117 in patients (pts) with advanced solid malignancies, in ASCO Annual Meeting Proceedings. (Chicago, IL).
Katso, R., Okkenhaug, K., Ahmadi, K., White, S., Timms, J., and Waterfield, M. D. (2001). Cellular function of phosphoinositide 3-kinases: implications for development, immunity, homeostasis, and cancer. Annu. Rev. Cell Dev. Biol. 17, 615–675. doi: 10.1146/annurev.cellbio.17.1.615
Kumar, C. C., and Madison, V. (2005). AKT crystal structure and AKT-specific inhibitors. Oncogene 24, 7493–7501. doi: 10.1038/sj.onc.1209087
Kunz, J., and Hall, M. N. (1993). Cyclosporin, A., FK506 and rapamycin: more than just immunosuppression. Trends Biochem. Sci. 18, 334–338. doi: 10.1016/0968-0004(93)90069-Y
Lee, S. J., Lee, J., Lee, J., Park, S. H., Park, J. O., Park, Y. S., et al. (2013). Phase II trial of capecitabine and everolimus (RAD001) combination in refractory gastric cancer patients. Invest. New Drugs 31, 1580–1586. doi: 10.1007/s10637-013-0022-0
Leslie, N. R., Batty, I. H., Maccario, H., Davidson, L., and Downes, C. (2008). Understanding PTEN regulation: PIP2, polarity and protein stability. Oncogene 27, 5464–5476. doi: 10.1038/onc.2008.243
Li, V. S., Wong, C. W., Chan, T. L., Chan, A. S., Zhao, W., Chu, K.-M., et al. (2005). Mutations of PIK3CA in gastric adenocarcinoma. BMC Cancer 5:29. doi: 10.1186/1471-2407-5-29
Liu, J.-F., Zhou, X.-K., Chen, J.-H., Yi, G., Chen, H.-G., Ba, M.-C., et al. (2010). Up-regulation of PIK3CA promotes metastasis in gastric carcinoma. World J. Gastroenterol. 16, 4986–4991. doi: 10.3748/wjg.v16.i39.4986
Lombardo, Y., Scopelliti, A., Cammareri, P., Todaro, M., Iovino, F., Ricci–Vitiani, L., et al. (2011). Bone morphogenetic protein 4 induces differentiation of colorectal cancer stem cells and increases their response to chemotherapy in mice. Gastroenterology 140, 297–309. doi: 10.1053/j.gastro.2010.10.005
Lowry, W. E., Blanpain, C., Nowak, J. A., Guasch, G., Lewis, L., and Fuchs, E. (2005). Defining the impact of β-catenin/Tcf transactivation on epithelial stem cells. Genes Dev. 19, 1596–1611. doi: 10.1101/gad.1324905
Luke, R. W., Addie, M., Box, M. R., Buttar, D., Crafter, C., Currie, G. S., et al. (2011). Discovery of AZD5363, an orally bioavailable, potent ATP-competitive inhibitor of AKT kinases. Cancer Res. 71(8 Suppl.):4478. doi: 10.1021/jm301762v
Luo, J., Sobkiw, C. L., Hirshman, M. F., Logsdon, M. N., Li, T. Q., Goodyear, L. J., et al. (2006). Loss of class I A PI3K signaling in muscle leads to impaired muscle growth, insulin response, and hyperlipidemia. Cell Metab. 3, 355–366. doi: 10.1016/j.cmet.2006.04.003
Manning, B. D., and Cantley, L. C. (2007). AKT/PKB signaling: navigating downstream. Cell 129, 1261–1274. doi: 10.1016/j.cell.2007.06.009
Mao, M., Tian, F., Mariadason, J. M., Tsao, C. C., Lemos, R., Dayyani, F., et al. (2013). Resistance to BRAF inhibition in BRAF-mutant colon cancer can be overcome with PI3K inhibition or demethylating agents. Clin. Cancer Res. 19, 657–667. doi: 10.1016/j.canlet.2016.02.040
Martini, M., Ciraolo, E., Gulluni, F., and Hirsch, E. (2013). Targeting PI3K in cancer: any good news? Front. Oncol. 3:108. doi: 10.3389/fonc.2013.00108
Merlos-Suárez, A., Barriga, F. M., Jung, P., Iglesias, M., Céspedes, M. V., Rossell, D., et al. (2011). The intestinal stem cell signature identifies colorectal cancer stem cells and predicts disease relapse. Cell Stem Cell 8, 511–524. doi: 10.1016/j.stem.2011.02.020
Migliardi, G., Sassi, F., Torti, D., Galimi, F., Zanella, E. R., Buscarino, M., et al. (2012). Inhibition of MEK and PI3K/mTOR suppresses tumor growth but does not cause tumor regression in patient-derived xenografts of RAS-mutant colorectal carcinomas. Clin. Cancer Res. 18, 2515–2525. doi: 10.1158/1078-0432.CCR-11-2683
Mouradov, D., Domingo, E., Gibbs, P., Jorissen, R. N., Li, S., Soo, P. Y., et al. (2013). Survival in stage II/III colorectal cancer is independently predicted by chromosomal and microsatellite instability, but not by specific driver mutations. Am. J. Gastroenterol. 108, 1785–1793. doi: 10.1038/ajg.2013.292
Nobukuni, T., Joaquin, M., Roccio, M., Dann, S. G., Kim, S. Y., Gulati, P., et al. (2005). Amino acids mediate mTOR/raptor signaling through activation of class 3 phosphatidylinositol 3OH-kinase. Proc. Natl. Acad. Sci. U.S.A. 102, 14238–14243. doi: 10.1073/pnas.0506925102
Ohtsu, A., Ajani, J. A., Bai, Y.-X., Bang, Y.-J., Chung, H.-C., Pan, H.-M., et al. (2013). Everolimus for previously treated advanced gastric cancer: results of the randomized, double-blind, phase III GRANITE-1 study. J. Clin. Oncol. 31, 3935–3943. doi: 10.1200/JCO.2012.48.3552
Ooms, L. M., Horan, K. A., Rahman, P., Seaton, G., Gurung, R., Kethesparan, D. S., et al. (2009). The role of the inositol polyphosphate 5-phosphatases in cellular function and human disease. Biochem. J. 419, 29–49. doi: 10.1042/BJ20081673
Pecchi, S., Burger, M., Nagel, T., Schnell, C., Menezes, D., Knapp, M., et al. (2010). Biological characterization of NVP-BKM120, a novel inhibitor of phosphoinosotide 3-kinase in Phase I/II clinical trials. Cancer Res. 70(8 Suppl.):4498. doi: 10.1158/1538-7445.AM10-4498
Persad, S., Troussard, A. A., McPhee, T. R., Mulholland, D. J., and Dedhar, S. (2001). Tumor suppressor PTEN inhibits nuclear accumulation of β-catenin and T cell/lymphoid enhancer factor 1–mediated transcriptional activation. J. Cell Biol. 153, 1161–1174. doi: 10.1083/jcb.153.6.1161
Phipps, A. I., Makar, K. W., and Newcomb, P. A. (2013). Descriptive profile of PIK3CA-mutated colorectal cancer in postmenopausal women. Int. J. Colorectal Dis. 28, 1637–1642. doi: 10.1007/s00384-013-1715-8
Potter, C. J., Pedraza, L. G., and Xu, T. (2002). Akt regulates growth by directly phosphorylating Tsc2. Nat. Cell Biol. 4, 658–665. doi: 10.1038/ncb840
Powis, G., Bonjouklian, R., Berggren, M. M., Gallegos, A., Abraham, R., Ashendel, C., et al. (1994). Wortmannin, a potent and selective inhibitor of phosphatidylinositol-3-kinase. Cancer Res. 54, 2419–2423.
Raimondi, C., and Falasca, M. (2012). Phosphoinositides signalling in cancer: focus on PI3K and PLC. Adv. Biol. Regul. 52, 166–182. doi: 10.1016/j.advenzreg.2011.09.016
Ramanathan, R. K., McDonough, S. L., Kennecke, H. F., Iqbal, S., Baranda, J. C., Seery, T. E., et al. (2015). Phase 2 study of MK-2206, an allosteric inhibitor of AKT, as second-line therapy for advanced gastric and gastroesophageal junction cancer: a SWOG cooperative group trial (S1005). Cancer 121, 2193–2197. doi: 10.1002/cncr.29363
Rini, B. I. (2008). Temsirolimus, an inhibitor of mammalian target of rapamycin. Clin. Cancer Res. 14, 1286–1290. doi: 10.1158/1078-0432.CCR-07-4719
Rivero, R. A., and Hardwicke, M. A. (2012). Identification of GSK2636771, a potent and selective, orally bioavailable inhibitor of phosphatidylinositol 3-kinase-beta (PI3Kα) for the treatment of PTEN deficient tumors. Cancer Res. 72(8 Suppl.):2913. doi: 10.1158/1538-7445.AM2012-2913
Rodon, J., Dienstmann, R., Serra, V., and Tabernero, J. (2013). Development of PI3K inhibitors: lessons learned from early clinical trials. Nat. Rev. Clin. Oncol. 10, 143–153. doi: 10.1038/nrclinonc.2013.10
Roper, J., Richardson, M. P., Wang, W. V., Richard, L. G., Chen, W., Coffee, E. M., et al. (2011). The dual PI3K/mTOR inhibitor NVP-BEZ235 induces tumor regression in a genetically engineered mouse model of PIK3CA wild-type colorectal cancer. PLoS ONE 6:e25132. doi: 10.1371/journal.pone.0025132
Sabatini, D. M., Ali, S. M., Guertin, D. A., and Sarbassov, D. D. (2005). Phosphorylation and regulation of Akt/PKB by the rictor-mTOR complex. Science 307, 1098–1101. doi: 10.1126/science.1106148
Samuels, Y., Diaz, L. A. Jr., Schmidt-Kittler, O., Cummins, J. M., DeLong, L., Cheong, I., et al. (2005). Mutant PIK3CA promotes cell growth and invasion of human cancer cells. Cancer Cell 7, 561–573. doi: 10.1016/j.ccr.2005.05.014
Samuels, Y., Wang, Z., Bardelli, A., Silliman, N., Ptak, J., Szabo, S., et al. (2004). High frequency of mutations of the PIK3CA gene in human cancers. Science 304, 554. doi: 10.1126/science.1096502
Sancho, E., Batlle, E., and Clevers, H. (2004). Signaling pathways in intestinal development and cancer. Annu. Rev. Cell Dev. Biol. 20, 695–723. doi: 10.1146/annurev.cellbio.20.010403.092805
Sarbassov, D. D., Ali, S. M., and Sabatini, D. M. (2005). Growing roles for the mTOR pathway. Curr. Opin. Cell Biol. 17, 596–603. doi: 10.1016/j.ceb.2005.09.009
Sasaki, T., Takasuga, S., Sasaki, J., Kofuji, S., Eguchi, S., Yamazaki, M., et al. (2009). Mammalian phosphoinositide kinases and phosphatases. Prog. Lipid Res. 48, 307–343. doi: 10.1016/j.plipres.2009.06.001
Shi, J., Yao, D., Liu, W., Wang, N., Lv, H., Zhang, G., et al. (2012). Highly frequent PIK3CA amplification is associated with poor prognosis in gastric cancer. BMC Cancer 12:50. doi: 10.1186/1471-2407-12-50
Shimizu, T., Tolcher, A. W., Papadopoulos, K. P., Beeram, M., Rasco, D. W., Smith, L. S., et al. (2012). The clinical effect of the dual-targeting strategy involving PI3K/AKT/mTOR and RAS/MEK/ERK pathways in patients with advanced cancer. Clin. Cancer Res. 18, 2316–2325. doi: 10.1158/1078-0432.CCR-11-2381
Singh, S. S., Yap, W. N., Arfuso, F., Kar, S., Wang, C., Cai, W., et al. (2015). Targeting the PI3K/Akt signaling pathway in gastric carcinoma: a reality for personalized medicine? World J. Gastroenterol. 21:12261. doi: 10.3748/wjg.v21.i43.12261
Stephens, L. R., Eguinoa, A., Erdjument-Bromage, H., Lui, M., Cooke, F., Coadwell, J., et al. (1997). The Gβγ sensitivity of a PI3K is dependent upon a tightly associated adaptor, p101. Cell 89, 105–114. doi: 10.1016/S0092-8674(00)80187-7
Vadas, O., Burke, J. E., Zhang, X., Berndt, A., and Williams, R. L. (2011). Structural basis for activation and inhibition of Class I Phosphoinositide 3-kinases. 4:re2. doi: 10.1126/scisignal.2002165
Velho, S., Oliveira, C., Ferreira, A., Ferreira, A. C., Suriano, G., Schwartz, S., et al. (2005). The prevalence of PIK3CA mutations in gastric and colon cancer. Eur. J. Cancer 41, 1649–1654. doi: 10.1016/j.ejca.2005.04.022
Walker, E. H., Pacold, M. E., Perisic, O., Stephens, L., Hawkins, P. T., Wymann, M. P., et al. (2000). Structural determinants of phosphoinositide 3-kinase inhibition by wortmannin, LY294002, quercetin, myricetin, and staurosporine. Mol. Cell 6, 909–919. doi: 10.1016/S1097-2765(05)00089-4
Wymann, M. P., Björklöf, K., Calvez, R., Finan, P., Thomast, M., Trifilieff, A., et al. (2003). Phosphoinositide 3-kinase gamma: a key modulator in inflammation and allergy. Biochem. Soc. Trans. 31(Pt 1), 275–280. doi: 10.1042/bst0310275
Wymann, M. P., Bulgarelli-Leva, G., Zvelebil, M. J., Pirola, L., Vanhaesebroeck, B., Waterfield, M. D., et al. (1996). Wortmannin inactivates phosphoinositide 3-kinase by covalent modification of Lys-802, a residue involved in the phosphate transfer reaction. Mol. Cell. Biol. 16, 1722–1733. doi: 10.1128/MCB.16.4.1722
Xing, C.-G., Zhu, B.-S., Fan, X.-Q., Liu, H.-H., Hou, X., Zhao, K., et al. (2009). Effects of LY294002 on the invasiveness of human gastric cancer in vivo in nude mice. World J. Gastroenterol. 15, 5044–5052. doi: 10.3748/wjg.15.5044
Yang, Q., and Guan, K.-L. (2007). Expanding mTOR signaling. Cell Res. 17, 666–681. doi: 10.1038/cr.2007.64
Yap, T. A., Yan, L., Patnaik, A., Fearen, I., Olmos, D., Papadopoulos, K., et al. (2011). First-in-man clinical trial of the oral pan-AKT inhibitor MK-2206 in patients with advanced solid tumors. J. Clin. Oncol. 29, 4688–4695. doi: 10.1200/JCO.2011.35.5263
Yu, K., Toral-Barza, L., Shi, C., Zhang, W.-G., and Zask, A. (2008). Response and determinants of cancer cell susceptibility to PI3K inhibitors: combined targeting of PI3K and Mek1 as an effective anticancer strategy. Cancer Biol. Ther. 7, 310–318. doi: 10.4161/cbt.7.2.5334
Yuan, T. L., and Cantley, L. C. (2008). PI3K pathway alterations in cancer: variations on a theme. Oncogene 27, 5497–5510. doi: 10.1038/onc.2008.245
Zhou, H., Luo, Y., and Huang, S. (2010). Updates of mTOR inhibitors. Anticancer Agents Med. Chem. 10, 571. doi: 10.2174/187152010793498663
Zhu, Y.-F., Yu, B.-H., Li, D.-L., Ke, H.-L., Guo, X.-Z., and Xiao, X.-Y. (2012). PI3K expression and PIK3CA mutations are related to colorectal cancer metastases. World J. Gastroenterol. 18:3745. doi: 10.3748/wjg.v18.i28.3745
Keywords: phosphoinositide, PI3K, mTOR, Akt, colorectal cancer, gastric cancer, targeted therapy
Citation: Kim HJ, Lee SY and Oh SC (2016) The Inositide Signaling Pathway As a Target for Treating Gastric Cancer and Colorectal Cancer. Front. Physiol. 7:168. doi: 10.3389/fphys.2016.00168
Received: 23 December 2015; Accepted: 25 April 2016;
Published: 09 May 2016.
Edited by:
Alexis E. Traynor-Kaplan, Immune System Management Therapeutics, USAReviewed by:
Michele Milella, Regina Elena National Cancer Institute, ItalyMassimo Libra, University of Catania, Italy
Copyright © 2016 Kim, Lee and Oh. This is an open-access article distributed under the terms of the Creative Commons Attribution License (CC BY). The use, distribution or reproduction in other forums is permitted, provided the original author(s) or licensor are credited and that the original publication in this journal is cited, in accordance with accepted academic practice. No use, distribution or reproduction is permitted which does not comply with these terms.
*Correspondence: Sang Cheul Oh, c2FjaG9oQGtvcmVhLmFjLmty
†These authors have contributed equally to this work.