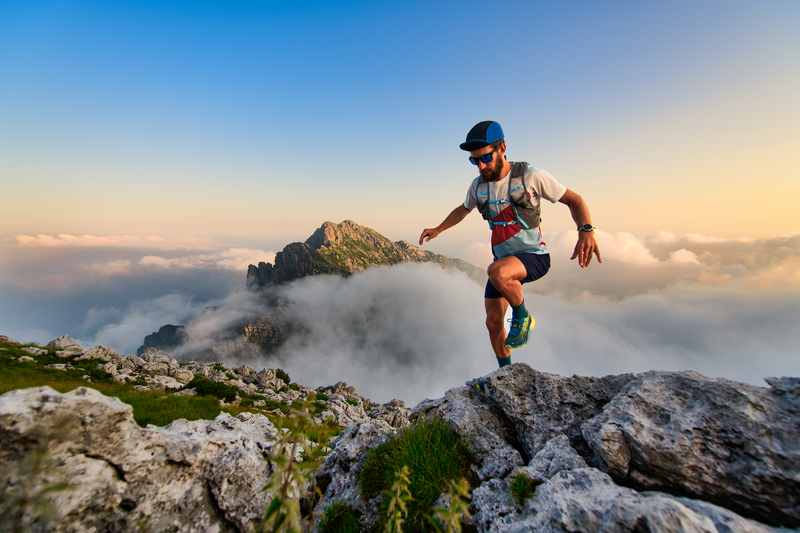
95% of researchers rate our articles as excellent or good
Learn more about the work of our research integrity team to safeguard the quality of each article we publish.
Find out more
MINI REVIEW article
Front. Physiol. , 23 December 2015
Sec. Integrative Physiology
Volume 6 - 2015 | https://doi.org/10.3389/fphys.2015.00384
This article is part of the Research Topic New translational insights on Metabolic Syndrome: Obesity, Hypertension, Diabetes and beyond View all 12 articles
The paraventricular nucleus of the hypothalamus (PVN) contains heterogeneous populations of neurons involved in autonomic and neuroendocrine regulation. The PVN plays an important role in the sympathoexcitatory response to increasing circulating levels of angiotensin II (Ang-II), which activates AT1 receptors in the circumventricular organs (OCVs), mainly in the subfornical organ (SFO). Circulating Ang-II induces a de novo synthesis of Ang-II in SFO neurons projecting to pre-autonomic PVN neurons. Activation of AT1 receptors induces intracellular increases in reactive oxygen species (ROS), leading to increases in sympathetic nerve activity (SNA). Chronic sympathetic nerve activation promotes a series of metabolic disorders that characterizes the metabolic syndrome (MetS): dyslipidemia, hyperinsulinemia, glucose intolerance, hyperleptinemia and elevated plasma hormone levels, such as noradrenaline, glucocorticoids, leptin, insulin, and Ang-II. This review will discuss the contribution of our laboratory and others regarding the sympathoexcitation caused by peripheral Ang-II-induced reactive oxygen species along the subfornical organ and paraventricular nucleus of the hypothalamus. We hypothesize that this mechanism could be involved in metabolic disorders underlying MetS.
The sympathoexcitation is linked to various diseases; circulating levels of Ang-II modulate SFO angiotensinergic projection to pre-autonomic PVN neurons, which lead to increases in sympathoexcitatory activity to the spinal cord via direct projections (Koshiya and Guyenet, 1996; Badoer, 2001; Stocker et al., 2004) or indirectly projecting to pre-sympathetic neurons in the RVLM (Koshiya and Guyenet, 1996; Badoer, 2001; Ito et al., 2002; Stocker et al., 2004). Increasing evidence supports the premise that Ang-II in the PVN is involved in pathological conditions originating from sympathoexcitation, such as hypertension, heart failure, diabetes, obesity, and metabolic syndrome (Gutkind et al., 1988; Ito et al., 2002; Oliveira-Sales et al., 2009; Braga et al., 2011). It has also been demonstrated that Ang-II increase reactive oxygen species (ROS) along the subfornical organ–paraventricular nucleus of the hypothalamus–rostral ventrolateral medulla axis [SFO-PVN-RVLM axis (Oliveira-Sales et al., 2008; Braga et al., 2011; Burmeister et al., 2011)].
The main ROS within the central nervous system is superoxide anions (O2−). Within the PVN, superoxide accumulation within the PVN ultimately results in sympathetic overactivity (Oliveira-Sales et al., 2009; Peterson et al., 2009; Burmeister et al., 2011; Cardinale et al., 2012; Campos et al., 2015). The aim of this mini-review is to discuss the role of sympathoexcitation induced by Ang-II-dependent ROS production in the pre-autonomic PVN neurons in modulating the development and/or metabolic disorders that results in the development and/or the maintenance of metabolic syndrome.
The paraventricular nucleus of the hypothalamus is anatomically connected to other hypothalamic areas and to the brainstem, playing a pivotal role in several homeostatic responses, being an important integrative nucleus for autonomic and neuroendocrine functions (Swanson and Sawchenko, 1980; Stern, 2001; Cruz et al., 2008; Cruz and Machado, 2009; Reis et al., 2010). Among the PVN functions are regulation of food intake, adipose afferent reflex (AAR), responses to stress, modulation of metabolic rate, thermoregulation, modulation of sympathetic nerve activity, and cardiovascular function (Swanson and Sawchenko, 1980; Stern, 2001; Benarroch, 2005; Cruz et al., 2008; Cruz and Machado, 2009; Reis et al., 2010; Cassaglia et al., 2011; Zsombok et al., 2011; Zhang et al., 2012; Ding et al., 2013; Xiong et al., 2014). The PVN is comprised of magnocellular and parvocellular subnuclei, which have different properties both neurochemically and electrophysiologically (Swanson and Sawchenko, 1980; Stern, 2001). The magnocellular subnucleus projects to the posterior hypophysis and parvocellular subnucleus, which include pre-autonomic neurons, send descending projections to cardiovascular autonomic brainstem nuclei as well as direct projections to the spinal cord (Koshiya and Guyenet, 1996; Badoer, 2001; Stocker et al., 2004; Cruz et al., 2008). Therefore, electrophysiological and functional studies support an essential role for the PVN in central blood pressure control (Cruz and Machado, 2009; Cruz et al., 2010; Busnardo et al., 2013) and sympathetic nerve activity (Koshiya and Guyenet, 1996; Badoer, 2001; Stocker et al., 2004). Our previous studies suggest that parvocellular pre-autonomic neurons modulate baseline blood pressure through activation of glutamatergic, GABAergic, purinergic, nitrergic, and angiotensinergic mechanisms (Chen et al., 2003; Cruz and Machado, 2009; Cruz et al., 2010; Busnardo et al., 2013). Accumulating evidence support the idea that imbalance between paraventricular inhibitory GABAergic and excitatory glutamatergic and/or angiotensinergic neurotransmission in the PVN, contribute to increase the pre-autonomic neuronal drive mediating neurogenic hypertension (Gören et al., 2000; Chen et al., 2003; Li and Pan, 2005; Li et al., 2006; Oliveira-Sales et al., 2009). Magnocellular and parvocellular neurons from PVN express receptors to a wide range of neurotransmitters and neurohormones including leptin, insulin, neuropeptide Y, Ang-II, GABA, glutamate, vasopressin, oxytocin, and noradrenaline (Stanley and Leibowitz, 1984; Saphier and Feldman, 1991; Lenkei et al., 1997; Håkansson and Meister, 1998; Zsombok et al., 2011). Therefore, it is suggested that an imbalance in synaptic function that modulates the pre-autonomic or neurosecretory neuron results in cardiovascular and neuroendocrine dysfunctions that, in turn, contribute to the development and potentiation of sympathoexcitatory response observed in hypertension, heart failure, atherosclerosis, diabetes, and obesity.
Some circulating lipophobic substances, incapable of crossing the blood brain barrier (BBB), such as glucose, insulin, leptin, noradrenaline, and angiotensin II have their receptors expressed in neurons of the circunventricular organs (CVOs), which have an incomplete BBB (Lenkei et al., 1997; Boundy and Cincotta, 2000; Braga et al., 2011; Cassaglia et al., 2011; Lob et al., 2013; Prior et al., 2014). One of the major CVOs receiving information from the peripheral circulation is the subfornical organ (SFO). Anatomical and functional evidence suggest that SFO is a pivotal nucleus in modulating pressor and dipsogenic actions of circulating Ang II (Bains et al., 1992; Li and Ferguson, 1993; Sakai et al., 2007; Braga et al., 2011). Genetic and physiological evidence shows that circulating Ang-II is involved in de novo synthesis of Ang II within the SFO, which is an integrative mechanism of fluid and cardiovascular homeostasis (Bains et al., 1992; Li and Ferguson, 1993; Sakai et al., 2007; Burmeister et al., 2011). Angiotensin II AT1 and AT2 receptors (AT1R; AT2R) are expressed in neurons and astrocytes of the PVN (Lenkei et al., 1997; Coleman et al., 2009; Oliveira-Sales et al., 2009) and angiotensinergic connections between the SFO and PVN is describe to control drinking and sympathetic nerve activity (Gutkind et al., 1988; Bains et al., 1992; Li and Ferguson, 1993; Anderson et al., 2001; Sakai et al., 2007; Burmeister et al., 2011). In that regard, several studies show that angiotensinergic connections between SFO and PVN are involved in the generation and maintenance of elevated baseline blood pressure in hypertensive rats (Gutkind et al., 1988; Burmeister et al., 2011). For example, studies by Miyakubo et al. (2002) demonstrated that excitatory response elicited by Ang-II in SFO neurons projecting to PVN was higher in spontaneous hypertensive rats (SHR) than in normotensive Wistar Kyoto rats (WKY). The brain Ang-II neurocircuitary also involves pre-autonomic PVN neurons projecting to rostral ventrolateral medulla (RVLM). The RVLM which tonically controls sympathetic vasomotor activity (Guyenet et al., 1989). Studies by Ito et al. (2002) indicate that RVLM vasomotor neurons in SHR, but not in the WKY rats, are tonically excited by PVN driven angiotensin II projections. Furthermore, several studies support the idea that Ang-II along the SFO-PVN-RVLM axis is a significant neuronal pathway involved in the maintenance of neurogenic hypertension (Ito et al., 2002; Oliveira-Sales et al., 2009; Braga et al., 2011).
Accumulating evidence support the idea, that Ang-II-induced oxidative stress within the PVN contributes to the pathogenesis of hypertension. In addition to increasing blood pressure and sympathetic nerve activity, central infusion of Ang-II leads to elevated levels of neurotransmitters (glutamate and norepinephrine), AT1R, pro-inflammatory cytokines, phosphorylated IKKbeta, NF-kappaB subunits, and superoxide in the central nervous system (Erdös et al., 2006; Oliveira-Sales et al., 2009; Peterson et al., 2009; Burmeister et al., 2011).
There is now growing evidence suggesting that inflammation and central Ang-II-induced ROS production are involved in the pathogenesis of neurogenic hypertension. For example, in Ang-II-treated rats, bilateral microinjection of NFkappaB blocker into the PVN induces a local decrease in NFkappaB p65 subunit activity, proinflammatory cytokines, ROS, AT1-R, as well as in blood pressure (Cardinale et al., 2012).
Ang-II acting on the AT1R induces activation of NADPH oxidase through protein kinase C (PKC). The NADPH oxidase is the major source of superoxide anion. This enzyme is composed of catalytic membrane (gp91phox and p22phox) and cytoplasmic (p40phox, p47phox, and p67phox) subunits, which transfer electrons to molecular oxygen, producing reactive oxygen species as superoxide (Chabrashvili et al., 2002; Lassègue and Clempus, 2003).
Oxidative stress is characterized by an imbalance between the production of ROS and antioxidant systems (Betteridge, 2000). Numerous studies support the concept that ROS production is increased in different nuclei in the brainstem and hypothalamus (Oliveira-Sales et al., 2009; Peterson et al., 2009; Braga et al., 2011; Burmeister et al., 2011; Campos et al., 2015). The role of oxidative stress in the development and/or maintenance of neurogenic hypertension has recently been reported in several animal models of hypertension, including the renovascular two-kidney–one-clip model [2K1C (Oliveira-Sales et al., 2008, 2009; Burmeister et al., 2011; de Queiroz et al., 2013)]. Studies by Lob et al. (2013) showed an increase in the superoxide production in the SFO after chronic angiotensin II infusion, which was blunted by SFO-targeted injections of an adenovirus encoding cre-recombinase for reducing of p22 (phox), Nox2, and Nox4 mRNA expression. In addition, studies by Yuan et al. (2013) showed that superoxide dismutase 1 (SOD1), an antioxidant enzyme, was overexpressed in the PVN, attenuating augmented sympathetic activity, and cardiac sympathetic afferent reflex, while improving the myocardial and vascular remodeling in spontaneous hypertensive rats (SHR). The expression of the isoforms Nox1, Nox2, and predominant Nox4 mRNA were found in the PVN under basal conditions. Furthermore, Nox4-generated superoxide within the PVN contributes to the sympathoexcitation and cardiac dysfunction observed in mice that experienced heart failure (Infanger et al., 2010).
It has been suggested that upregulation of ROS in the RVLM and PVN contributes to increased blood pressure and SNA in renovascular hypertensive rats, with ROS preceding the increase in blood pressure in Ang-II-dependent model of hypertension (Kitiyakara and Wilcox, 1998; Botelho-Ono et al., 2011; Burmeister et al., 2011; de Queiroz et al., 2013) mRNA expression studies revealed that AT-1 and NADPH oxidase subunits were greater in the RVLM and PVN in renovascular hypertensive rats (Oliveira-Sales et al., 2009; Campos et al., 2015). In addition, studies by Burmeister et al. (2011) documented that a significant increase in the superoxide production in the PVN of renovascular hypertensive mice leads to activator protein-1 (AP-1) activation, a nuclear transcription factor, resulting in hypertension, while inhibition of AP-1 activity in the prevented renovascular hypertension. Furthermore, microinjection of superoxide dismutase mimetic, 4 hydroxy-2, 2, 6, 6-tetramethyl piperidinoxyl (Tempol) into the RVLM and PVN decreased the mean arterial pressure and renal sympathetic nerve activity in renovascular hypertensive rats, supporting the idea that upregulation of ROS in central cardiovascular areas, such as RVLM and PVN, contributes to elevated arterial pressure and sympathetic activity (Oliveira-Sales et al., 2009). Interestingly, microinjection of an adenovirus (Ad) encoding superoxide dismutase (AdCuZnSOD) in the PVN not only decreased the local superoxide accumulation into the PVN, but also prevented hypertension. Together, these observations led to the proposal (Braga et al., 2011) that the formation of Ang-II-induced ROS along the SFO-PVN-RVLM axis is an important mechanism involved in the pathogenesis of neurogenic hypertension.
Diet and lifestyle associated to genetic factors are involved in the development of metabolic syndrome (MetS). Metabolic changes such as dyslipidemia, glucose intolerance, hyperinsulinemia, hyperleptinemia, systemic inflammation, and chronic increase in the SNA, which characterize MetS, also augment the risk of developing diseases such as obesity, diabetes, atherosclerosis, and arterial hypertension. The PVN, as described above, is a key central nucleus participating in the regulation of cardiovascular and sympathetic activity (Koshiya and Guyenet, 1996; Badoer, 2001; Stocker et al., 2004; Cruz et al., 2008). It is involved in the sympathetic overactivity in rats with hypertension (Gören et al., 2000; Chen et al., 2003; Li et al., 2006; Oliveira-Sales et al., 2009), obesity (Xiong et al., 2012; Ding et al., 2013), and insulin resistance [commonly seen in the form of diabetes (Cassaglia et al., 2011; Zhang et al., 2012)]. In addition, several reports suggest that ROS activation contributes to insulin resistance observed in diabetic rats accompanied by obesity and hypertension (Folli et al., 1997; Xiong et al., 2012; Zhang et al., 2012; Cruz et al., 2013; Ding et al., 2013; de Kloet et al., 2013)
It is known that activation of the renin-angiotensin system may lead to insulin resistance in the vasculature (Folli et al., 1997); Ang-II impairs insulin receptor intracellular signaling, inhibiting insulin receptor substrate-1 (IRS-1) phosphorylation and phosphatidylinositol (PI) 3–kinase activation (Folli et al., 1997; Cizmeci and Arkun, 2013). In addition, it has been documented that AT1R receptor expression is increased in the PVN of rats with diabetes and insulin resistance (Zhang et al., 2012; Sun et al., 2014). Furthermore, Ang-II activates NADPH oxidase via AT1 receptors, increasing superoxide anion accumulation in the PVN, thereby contributing to enhanced sympathetic activity in diabetic and insulin resistance rats (Patel et al., 2011; Zhang et al., 2012; Sun et al., 2014).
Sympathetically-mediated interactions between PVN and white adipose tissue via AAR are important for the maintenance of total body fat and energy balance (Xiong et al., 2012; Ding et al., 2013). AAR is increased in obese hypertensive rats (Xiong et al., 2012, 2014; Ding et al., 2013) and inhibition of PVN decreases SNA and mean arterial pressure, while abolishing AAR in hypertensive obese rats (Xiong et al., 2012). Furthermore, studies by Ding et al. (2013) showed that NADPH oxidase-derived superoxide anions in the PVN modulates AAR, while PVN microinjection of tempol decreases baseline renal SNA, blood pressure, and attenuated the AAR. Thus, Ang-II induces ROS in the PVN may be a significant central mechanism modulating AAR. Studies by de Kloet et al. (2013) observed that deletion of AT1 receptors in the PVN not only reduced the local expression of corticotrophin-releasing hormone (CRH), oxytocin, and tumor necrosis factor α (TNF-α, a pro-inflammatory cytokine), but also decreased systolic blood pressure in mice rendered obese by high fat diet. This suggests that AT1 receptors in the PVN regulates the central metabolic changes that promotes metabolic and cardiovascular disorders.
In the last years, our laboratory has been investigating the mechanisms underlying neurogenic hypertension and our results strongly suggest that this pathological condition is caused by Ang-II-dependent ROS accumulation along the SFO-PVN-RVLM axis (Peterson et al., 2009; Botelho-Ono et al., 2011; Braga et al., 2011; Burmeister et al., 2011; de Queiroz et al., 2013). Accumulating evidence suggest that hyperactivity of the angiotensin system within the PVN is involved not only in hypertension, but also in diabetes and obesity existing as comorbidities (Oliveira-Sales et al., 2008, 2009; de Kloet et al., 2010, 2013; Braga et al., 2011; Xiong et al., 2012, 2014; Cizmeci and Arkun, 2013; Ding et al., 2013). This mini-review supports the hypothesis illustrated in the Figure 1 that the increase in the circulating levels of Ang-II activates angiotensinergic neurons in the SFO, which projects to pre-autonomic neurons expressing AT1 receptors in the PVN. The stimulation of AT1 receptors in the PVN and RVLM induces intracellular signals activating NADPH oxidase through protein kinase C. NADPH oxidase activity increases ROS formation, contributing to overactivity of pre-autonomic PVN neurons, resulting in sympathoexcitation through an indirect pathway (angiotensinergic projections to RVLM) and/or directly projections to the spinal cord, thereby mediating increase in plasma renin-angiotensin system, insulin, glucose, leptin, lipolysis as well as vasoconstriction. All these metabolic changes are involved in the symptoms of MetS.
Figure 1. Angiotensin II (Ang-II) induces sympathetic nerve activation (SNA) by increasing reactive oxygen species (ROS) along SFO-PVN axis underlying Metabolic Syndrome (MetS) symptoms (see text). Modified by de Queiroz et al. (2013) and Benarroch (2005). SFO: subfonical organ; PVN, paraventricular nucleus of the hypothalamus; RVLM, rostral ventrolateral medulla; AT1R, AT1 Ang-II receptor; RAS, renin angiotensin system.
All authors participated in the design of the manuscript, drafted the manuscript, revised the manuscript critically and approved the final version.
The authors declare that the research was conducted in the absence of any commercial or financial relationships that could be construed as a potential conflict of interest.
The authors are grateful to CNPq and CAPES for financial support.
Anderson, J. W., Smith, P. M., and Ferguson, A. V. (2001). Subfornical organ neurons projecting to paraventricular nucleus: whole-cell properties. Brain Res. 921, 78–85. doi: 10.1016/S0006-8993(01)03093-1
Badoer, E. (2001). Hypothalamic paraventricular nucleus and cardiovascular regulation. Clin. Exp. Pharmacol. Physiol. 28, 95–99. doi: 10.1046/j.1440-1681.2001.03413.x
Bains, J. S., Potyok, A., and Ferguson, A. V. (1992). Angiotensin II actions in paraventricular nucleus: functional evidence for neurotransmitter role in efferents originating in subfornical organ. Brain Res. 599, 223–229.
Benarroch, E. E. (2005). Paraventricular nucleus, stress response, and cardiovascular disease. Clin. Aut. Res. 15, 254–263. doi: 10.1007/s10286-005-0290-7
Betteridge, D. J. (2000). What is oxidative stress? Metabolism 49, 3–8. doi: 10.1016/S0026-0495(00)80077-3
Botelho-Ono, M. S., Pina, H. V., Sousa, K. H. F., Nunes, F. C., Medeiros, I. A., and Braga, V. A. (2011). Acute superoxide scavenging restores depressed baroreflex sensitivity in renovascular hypertensive rats. Auton. Neurosci. Basic Clin. 159, 38–44. doi: 10.1016/j.autneu.2010.07.025
Boundy, V. A., and Cincotta, A. H. (2000). Hypothalamic adrenergic receptor changes in the metabolic syndrome of genetically obese (ob/ob) mice. Am. J. Physiol. Regul. Integr. Comp. Physiol. 279, R505–R514.
Braga, V. A., Medeiros, I. A., Ribeiro, T. P., França-Silva, M. S., Botelho-Ono, M. S., and Guimarães, D. D. (2011). Angiotensin-II-induced reactive oxygen species along the SFO-PVN-RVLM pathway: implications in neurogenic hypertension. Braz. J. Med. Biol. Res. 44, 871–876. doi: 10.1590/S0100-879X2011007500088
Burmeister, M. A., Young, C. N., Braga, V. A., Butler, S. D., Sharma, R. V., and Davisson, R. L. (2011). In vivo bioluminescence imaging reveals redox-regulated activator protein-1 activation in paraventricular nucleus of mice with renovascular hypertension. Hypertension 57, 289–297. doi: 10.1161/HYPERTENSIONAHA.110.160564
Busnardo, C., Ferreira-Junior, N. C., Cruz, J. C., Machado, B. H., Correa, F. M., and Resstel, L. B. M. (2013). Cardiovascular responses to ATP microinjected into the paraventricular nucleus are mediated by nitric oxide and NMDA glutamate receptors in awake rats. Exp. Physiol. 98, 1411–1421. doi: 10.1113/expphysiol.2013.073619
Campos, R. R., Oliveira-Sales, E. B., Nishi, E. E., Paton, J. F., and Bergamaschi, C. T. (2015). Mechanisms of renal sympathetic activation in renovascular hypertension. Exp. Physiol. 100, 496–501. doi: 10.1113/expphysiol.2014.079855
Cardinale, J. P., Sriramula, S., Mariappan, N., Agarwal, D., and Francis, J. (2012). Angiotensin II-induced hypertension is modulated by nuclear factor-κBin the paraventricular nucleus. Hypertension 59, 113–121. doi: 10.1161/HYPERTENSIONAHA.111.182154
Cassaglia, P. A., Hermes, S. M., Aicher, S. A., and Brooks, V. L. (2011). Insulin acts in the arcuate nucleus to increase lumbar sympathetic nerve activity and baroreflex function in rats. J. Physiol. 589, 1643–1662. doi: 10.1113/jphysiol.2011.205575
Chabrashvili, T., Tojo, A., Onozato, M. L., Kitiyakara, C., Quinn, M. T., Fujita, T., et al. (2002). Expression and cellular localization of classic NADPH oxidase subunits in the spontaneously hypertensive rat kidney. Hypertension 39, 269–274. doi: 10.1161/hy0202.103264
Chen, Q. H., Haywood, J. R., and Toney, G. M. (2003). Sympathoexcitation by PVN-injected bicuculline requires activation of excitatory amino acid receptors. Hypertension 42, 725–731. doi: 10.1161/01.HYP.0000085197.20043.44
Cizmeci, D., and Arkun, Y. (2013). Regulatory networks and complex interactions between the insulin and angiotensin II signalling systems: models and implications for hypertension and diabetes. PLoS ONE 8:e83640. doi: 10.1371/journal.pone.0083640
Coleman, C. G., Anrather, J., Iadecola, C., and Pickel, V. M. (2009). Angiotensin II type 2 receptors have a major somatodendritic distribution in vasopressin-containing neurons in the mouse hypothalamic paraventricular nucleus. Neuroscience 163, 129–142. doi: 10.1016/j.neuroscience.2009.06.032
Cruz, J. C., Bonagamba, L. G. H., and Machado, B. H. (2010). Modulation of arterial pressure by P2 purinoceptors in the paraventricular nucleus of the hypothalamus of awake rats. Auton. Neurosci. Basic Clin. 158, 79–85. doi: 10.1016/j.autneu.2010.06.012
Cruz, J. C., Bonagamba, L. G. H., Machado, B. H., Biancardi, V. C., and Stern, J. E. (2008). Intermittent activation of peripheral chemoreceptors in awake rats induces Fos expression in rostral ventrolateral medulla-projecting neurons in the paraventricular nucleus of the hypothalamus. Neuroscience 157, 463–472. doi: 10.1016/j.neuroscience.2008.08.070
Cruz, J. C., Cavalleri, M. T., Ceroni, A., and Michelini, L. C. (2013). Peripheral chemoreceptors mediate training-induced plasticity in paraventricular nucleus pre-autonomic oxytocinergic neurons. Exp. Physiol. 98, 386–396. doi: 10.1113/expphysiol.2012.065888
Cruz, J. C., and Machado, B. H. (2009). GABA and nitric oxide in the PVN are involved in arterial pressure control but not in the chemoreflex responses in rats. Auton. Neurosci. Basic Clin. 146, 47–55. doi: 10.1016/j.autneu.2008.11.011
de Kloet, A. D., Krause, E. G., and Woods, S. C. (2010). The renin angiotensin system and the metabolic syndrome. Physiol. Behav. 100, 525–534. doi: 10.1016/j.physbeh.2010.03.018
de Kloet, A. D., Pati, D., Wang, L., Hiller, H., Sumners, C., Frazier, C. J., et al. (2013). Angiotensin type 1a receptors in the paraventricular nucleus of the hypothalamus protect against diet-induced obesity. J. Neurosci. 33, 4825–4833. doi: 10.1523/JNEUROSCI.3806-12.2013
de Queiroz, T. M., Monteiro, M. M. O., and Braga, V. A. (2013). Angiotensin-II-derived reactive oxygen species on baroreflex sensitivity during hypertension: new perspectives. Front. Physiol. 4:105. doi: 10.3389/fphys.2013.00105
Ding, L., Zhang, L. L., Gao, R., Chen, D., Wang, J. J., Gao, X. Y., et al. (2013). Superoxide anions in paraventricular nucleus modulate adipose afferent reflex and sympathetic activity in rats. PLoS ONE 8:e83771. doi: 10.1371/journal.pone.0083771
Erdös, B., Broxson, C. S., King, M. A., Scarpace, P. J., and Tümer, N. (2006). Acute pressor effect of central angiotensin II is mediated by NAD(P)H-oxidase-dependent production of superoxide in the hypothalamic cardiovascular regulatory nuclei. J. Hypertens. 24, 109–116. doi: 10.1097/01.hjh.0000198026.99600.59
Folli, F., Kahn, C. R., Hansen, H., Bouchie, J. L., and Feener, E. P. (1997). Angiotensin II inhibits insulin signaling in aortic smooth muscle cells at multiple levels. A potential role for serine phosphorylation in insulin/angiotensin II crosstalk. J. Clin. Invest. 100, 2158–2169. doi: 10.1172/JCI119752
Gören, M. Z., Onat, F., and Berkman, K. (2000). Participation of NMDA and kainate receptors of paraventricular nucleus in cardiovascular responses to glutamate receptor agonist. Eur. J. Pharmacol. 388, 77–84. doi: 10.1016/S0014-2999(99)00838-9
Gutkind, J. S., Kurihara, M., Castren, E., and Saavedra, J. M. (1988). Increased concentration of angiotensin II binding sites in selected brain areas of spontaneously hypertensive rats. J. Hypertens. 6, 79–84. doi: 10.1097/00004872-198801000-00012
Guyenet, P. G., Haselton, J. R., and Sun, M. K. (1989). Sympathoexcitatory neurons of the rostroventrolateral medulla and the origin of the sympathetic vasomotor tone. Prog. Brain Res. 81, 105–116. doi: 10.1016/S0079-6123(08)62002-6
Håkansson, M. L., and Meister, B. (1998). Transcription factor STAT3 in leptin target neurons of the rat hypothalamus. Neuroendocrinology 68, 420–427. doi: 10.1159/000054392
Infanger, D. W., Cao, X., Butler, S. D., Burmeister, M. A., Zhou, Y., Stupinski, J., et al. (2010). Silencing nox4 in the paraventricular nucleus improves myocardial infarction-induced cardiac dysfunction by attenuating sympathoexcitation and periinfarct apoptosis. Circ. Res. 106, 1763–1774. doi: 10.1161/CIRCRESAHA.109.213025
Ito, S., Komatsu, K., Tsukamoto, K., Kanmatsuse, K., and Sved, A. F. (2002). Ventrolateral medulla AT1 receptors support blood pressure in hypertensive rats. Hypertension 40, 552–559. doi: 10.1161/01.HYP.0000033812.99089.92
Kitiyakara, C., and Wilcox, C. S. (1998). Antioxidants for hypertension. Curr. Opin. Nephrol. Hypertens. 7, 531–538. doi: 10.1097/00041552-199809000-00008
Koshiya, N., and Guyenet, P. G. (1996). NTS neurons with carotid chemoreceptor inputs arborize in the rostral ventrolateral medulla. Am. J. Physiol. 270, R1273–R1278.
Lassègue, B., and Clempus, R. E. (2003). Vascular NAD(P)H oxidases: specific features, expression, and regulation. Am. J. Physiol. Regul. Integr. Comp. Physiol. 285, R277–R297. doi: 10.1152/ajpregu.00758.2002
Lenkei, Z., Palkovits, M., Corvol, P., and Llorens-Cortès, C. (1997). Expression of angiotensin type-1 (AT1) and type-2 (AT2) receptor mRNAs in the adult rat brain: a functional neuroanatomical review. Front. Neuroendocrinol. 18:155. doi: 10.1006/frne.1997.0155
Li, D.-P., and Pan, H.-L. (2005). Angiotensin II attenuates synaptic GABA release and excites paraventricular-rostral ventrolateral medulla output neurons. J. Pharmacol. Exp. Ther. 313, 1035–1045. doi: 10.1124/jpet.104.082495
Li, Y.-F., Jackson, K. L., Stern, J. E., Rabeler, B., and Patel, K. P. (2006). Interaction between glutamate and GABA systems in the integration of sympathetic outflow by the paraventricular nucleus of the hypothalamus. Am. J. Physiol. Heart Circ. Physiol. 291, H2847–H2856. doi: 10.1152/ajpheart.00625.2005
Li, Z., and Ferguson, A. V. (1993). Subfornical organ efferents to paraventricular nucleus utilize angiotensin as a neurotransmitter. Am. J. Physiol. 265, R302–R309.
Lob, H. E., Schultz, D., Marvar, P. J., Davisson, R. L., and Harrison, D. G. (2013). Role of the NADPH oxidases in the subfornical organ in angiotensin II-induced hypertension. Hypertension 61, 382–387. doi: 10.1161/HYPERTENSIONAHA.111.00546
Miyakubo, H., Hayashi, Y., and Tanaka, J. (2002). Enhanced response of subfornical organ neurons projecting to the hypothalamic paraventricular nucleus toangiotensin II in spontaneously hypertensive rats. Auton. Neurosci. 95, 131–136. doi: 10.1016/S1566-0702(01)00388-5
Oliveira-Sales, E. B., Dugaich, A. P., Carillo, B. A., Abreu, N. P., Boim, M. A., Martins, P. J., et al. (2008). Oxidative stress contributes to renovascular hypertension. Am. J. Hypertens. 21, 98–104. doi: 10.1038/ajh.2007.12
Oliveira-Sales, E. B., Nishi, E. E., Carillo, B. A., Boim, M. A., Dolnikoff, M. S., Bergamaschi, C. T., et al. (2009). Oxidative stress in the sympathetic premotor neurons contributes to sympathetic activation in renovascular hypertension. Am. J. Hypertens. 22, 484–492. doi: 10.1038/ajh.2009.17
Patel, K. P., Mayhan, W. G., Bidasee, K. R., and Zheng, H. (2011). Enhanced angiotensin II-mediated central sympathoexcitation in streptozotocin-induced diabetes: role of superoxide anion. Am. J. Physiol. Regul. Integr. Comp. Physiol. 300, R311–R320. doi: 10.1152/ajpregu.00246.2010
Peterson, J. R., Burmeister, M. A., Tian, X., Zhou, Y., Guruju, M. R., Stupinski, J. A., et al. (2009). Genetic silencing of Nox2 and Nox4 reveals differential roles of these nadph oxidase homologues in the vasopressor and dipsogenic effects of brain angiotensin II. Hypertension 54, 1106–1114. doi: 10.1161/HYPERTENSIONAHA.109.140087
Prior, L. J., Davern, P. J., Burke, S. L., Lim, K., Armitage, J. A., and Head, G. A. (2014). Exposure to a high-fat diet during development alters leptin and ghrelin sensitivity and elevates renal sympathetic nerve activity and arterial pressure in rabbits. Hypertension 63, 338–345. doi: 10.1161/HYPERTENSIONAHA.113.02498
Reis, W. L., Saad, W. A., Camargo, L. A., Elias, L. L., and Antunes-Rodrigues, J. (2010). Central nitrergic system regulation of neuroendocrine secretion, fluid intake and blood pressure induced by angiotensin-II. Behav. Brain Funct. 6:64. doi: 10.1186/1744-9081-6-64
Sakai, K., Agassandian, K., Morimoto, S., Sinnayah, P., Cassell, M. D., Davisson, R. L., et al. (2007). Local production of angiotensin II in the subfornical organ causes elevated drinking. J. Clin. Invest. 117, 1088–1095. doi: 10.1172/JCI31242
Saphier, D., and Feldman, S. (1991). Catecholaminergic projections to tuberoinfundibular neurones of the paraventricular nucleus: III. Effects of adrenoceptor agonists and antagonists. Brain Res. Bull. 26, 863–870. doi: 10.1016/0361-9230(91)90250-N
Stanley, B. G., and Leibowitz, S. F. (1984). Neuropeptide Y: stimulation of feeding and drinking by injection into the paraventricular nucleus. Life Sci. 35, 2635–2642. doi: 10.1016/0024-3205(84)90032-8
Stern, J. E. (2001). Electrophysiological and morphological properties of pre-autonomic neurones in the rat hypothalamic paraventricular nucleus. J. Physiol. 537, 161–177. doi: 10.1111/j.1469-7793.2001.0161k.x
Stocker, S. D., Cunningham, J. T., and Toney, G. M. (2004). Water deprivation increases Fos immunoreactivity in PVN autonomic neurons with projections to the spinal cord and rostral ventrolateral medulla. Am. J. Physiol. Regul. Integr. Comp. Physiol. 287, R1172–R1183. doi: 10.1152/ajpregu.00394.2004
Sun, H. J., Zhou, H., Feng, X. M., Gao, Q., Ding, L., Tang, C. S., et al. (2014). Superoxide anions in the paraventricular nucleus mediate cardiac sympathetic afferent reflex in insulin resistance rats. Acta Physiol. (Oxf). 212, 267–282. doi: 10.1111/apha.12405
Swanson, L. W., and Sawchenko, P. E. (1980). Paraventricular nucleus: a site for the integration of neuroendocrine and autonomic mechanisms. Neuroendocrinology 31, 410–417. doi: 10.1159/000123111
Xiong, X.-Q., Chen, W.-W., Han, Y., Zhou, Y.-B., Zhang, F., Gao, X.-Y., et al. (2012). Enhanced adipose afferent reflex contributes to sympathetic activation in diet-induced obesity hypertension. Hypertension 60, 1280–1286. doi: 10.1161/HYPERTENSIONAHA.112.198002
Xiong, X. Q., Chen, W. W., and Zhu, G. Q. (2014). Adipose afferent reflex: sympathetic activation and obesity hypertension. Acta Physiol. 210, 468–478. doi: 10.1111/apha.12182
Yuan, N., Zhang, F., Zhang, L. L., Gao, J., Zhou, Y. B., Han, Y., et al. (2013). SOD1 gene transfer into paraventricular nucleus attenuates hypertension and sympathetic activity in spontaneously hypertensive rats. Pflugers Arch. Eur. J. Physiol. 465, 261–270. doi: 10.1007/s00424-012-1173-0
Zhang, L., Xiong, X.-Q., Fan, Z.-D., Gan, X.-B., Gao, X.-Y., and Zhu, G.-Q. (2012). Involvement of enhanced cardiac sympathetic afferent reflex in sympathetic activation in early stage of diabetes. J. Appl. Physiol. 113, 47–55. doi: 10.1152/japplphysiol.01228.2011
Zsombok, A., Gao, H., Miyata, K., Issa, A., and Derbenev, A. V. (2011). Immunohistochemical localization of transient receptor potential vanilloid type 1 and insulin receptor substrate 2 and their co-localization with liver-related neurons in the hypothalamus and brainstem. Brain Res. 1398, 30–39. doi: 10.1016/j.brainres.2011.04.048
Keywords: paraventricular nucleus of the hypothalamus, angiotensin II, oxidative stress, metabolic syndrome
Citation: Cruz JC, Flôr AFL, França-Silva MS, Balarini CM and Braga VA (2015) Reactive Oxygen Species in the Paraventricular Nucleus of the Hypothalamus Alter Sympathetic Activity During Metabolic Syndrome. Front. Physiol. 6:384. doi: 10.3389/fphys.2015.00384
Received: 01 August 2015; Accepted: 27 November 2015;
Published: 23 December 2015.
Edited by:
Elisabeth Lambert, BakerIDI Heart and Diabetes Institute, AustraliaReviewed by:
Tamara Paravicini, Royal Melbourne Institute of Technology University, AustraliaCopyright © 2015 Cruz, Flôr, França-Silva, Balarini and Braga. This is an open-access article distributed under the terms of the Creative Commons Attribution License (CC BY). The use, distribution or reproduction in other forums is permitted, provided the original author(s) or licensor are credited and that the original publication in this journal is cited, in accordance with accepted academic practice. No use, distribution or reproduction is permitted which does not comply with these terms.
*Correspondence: Josiane C. Cruz, am9zaWFuZWNydXpAY2Jpb3RlYy51ZnBiLmJy
Disclaimer: All claims expressed in this article are solely those of the authors and do not necessarily represent those of their affiliated organizations, or those of the publisher, the editors and the reviewers. Any product that may be evaluated in this article or claim that may be made by its manufacturer is not guaranteed or endorsed by the publisher.
Research integrity at Frontiers
Learn more about the work of our research integrity team to safeguard the quality of each article we publish.