- 1Spinal Cord Injury and Tissue Regeneration Centre Salzburg, Institute of Tendon and Bone Regeneration, Paracelsus Medical University, Salzburg, Austria
- 2Austrian Cluster for Tissue Regeneration, Vienna, Austria
Tendons represent a bradytrophic tissue which is poorly vascularized and, compared to bone or skin, heal poorly. Usually, a vascularized connective scar tissue with inferior functional properties forms at the injury site. Whether the increased vascularization is the root cause of tissue impairments such as loss of collagen fiber orientation, ectopic formation of bone, fat or cartilage, or is a consequence of these pathological changes remains unclear. This review provides an overview of the role of tendon vasculature in healthy and chronically diseased tendon tissue as well as its relevance for tendon repair. Further, the nature and the role of perivascular tendon stem/progenitor cells residing in the vascular niche will be discussed and compared to multipotent stromal cells in other tissues.
Introduction
Tendons are fibrous bands of connective tissue which connect muscle to bone and are able to withstand tension. Besides the mere transmission of force, their physiologic role is also the storage and recovery of energy, made possible by specific biomechanical properties. Upon damage, either due to traumatic injury and/or chronic degeneration, the macromolecular structure is disturbed, resulting in inferior tissue quality. Unlike in other, highly vascularized tissues such as skin or bone, neovascularization following tendon injury is not necessarily a hallmark of functional tissue repair. Instead, it is associated with degeneration as healthy tendons generally are poorly vascularized with relatively few cells embedded in an abundant collagen matrix. The aim of this review is to provide an overview of our current understanding of tendon vasculature and its role in healthy and developing tendon as well as the involvement of the vascular bed in tendon de- and regeneration.
Vasculature in Intact Tendons
In early medical literature tendons are described as “virtually dead during life” (Edwards, 1946), based on the fact that tendons are poorly vascularized and contain only few cells compared to other tissues. Even though the vascular and lymphatic network had been visualized by dye injections, tendons were considered to be “non-viable cables” (Peacock, 1959). Nevertheless, although tendons resemble a sparsely vascularized tissue type they generally harbor more vessels than commonly believed.
Mechanisms and Sources of Vascularization
Generally, blood vessels emanate into tendons from the musculo—tendineous junction, from the bone insertion site, and the so called “paratenon,” a loose areolar gliding tissue surrounding non-synovial tendons (Peacock, 1959; Schmidt-Rohlfing et al., 1992; Ahmed et al., 1998; Kannus, 2000). The complexity of the vascular network also depends on whether tendons are sheathed, that is embedded in synovial tissue, or is unsheathed. In 1953, Brockis J.G. has shown that in the sheathed digital flexor tendons of the palm vessels only enter the tendon at few distinct sites, whereas in the distal part of the pals and in the forearm, where the tendon is surrounded by paratenon tissue, vessels pass through the tissue more frequently (Brockis, 1953). These two “types” of tendon were later on referred to as “avascular tendons” and “vascular tendons,” with major implications for the understanding of adhesion formation following surgical repair (see below; Chaplin, 1973). Nevertheless, even though tendons clearly are not “non-viable cables,” they are poorly vascularized and particularly the avascular superficial zones of sheathed tendons are mainly nourished by diffusion from the synovial sheath.
Generally, the number of supplying vascular branches significantly differs between various tendons. For example, the patellar ligament is supplied by a total of three arteries and by the anastomotic arch from the Hoffa fat pad (Pang et al., 2009), whereas the Achilles tendon is supplied both by the peroneal and the posterior tibial arteries (Schmidt-Rohlfing et al., 1992). As tendons naturally are moving tissues which are extended by mechanical load, also the vasculature must be compliant to being stretched. In vascular tendons, vessels form “curves” within their embedding endotenon tissue, a loose areolar intratendineous tissue surrounding individual fascicles (Kannus, 2000). Upon loading of the tendon the vessels are stretched accordingly (Brockis, 1953). An illustrative and well described example for the anatomy of tendon vascularization is the rotator cuff, a group of four muscles and the connected tendons moving and stabilizing the glenohumeral joint. Despite the fact that all four tendons serve a relatively similar function, the supraspinatus tendon is unique in terms of its vascular bed. In this tendon initially an avascular zone, referred to as the “critical zone,” usually located about 1 cm proximal from the bony insertion has been described (Lindblom, 1939). However, subsequent studies demonstrated the presence of a vascular bed and it was postulated that the filling of the blood vessels was dependent on the positioning of the arm (Rathbun and MacNab, 1970). Such relative avascular zones have also been described for other tendons, i.e., the Achilles tendon (Stein et al., 2000) or the patella tendon (Clancy et al., 1981). Clinically these zones, also referred to as the “watershed area” in the Achilles tendon, are often prone to inflammatory episodes, potentially resulting in a painful and chronic tendinopathy (Józsa and Kannus, 1997) and/or rupture of the tendon (Alfredson et al., 2001).
The role of the vasculature during tendon development and maturation is also still poorly defined. Peacock (1959) describes embryonic tendons to be “supplied with a rich capillary network,” by analysing images of an 8 month old human embryo. A study in postnatal, immature sheep describes a massive decline in both cellularity and vessel density in the tendon of the extrinsic flexor muscles of the fingers (musculus flexor digitorum superficialis) (Meller et al., 2009). In line with these findings, several studies point out the (relative) decrease of cell density during tendon maturation (Ippolito et al., 1980; Józsa and Kannus, 1997; Oryan and Shoushtari, 2008). Given the fact that very little turnover of the extracellular matrix occurs in human tendons after termination of linear growth after (~ 17–18 years of age), low vascular supply seems appropriate (Heinemeier et al., 2013). For example, the half–life of collagen in mature equine tendons was calculated to be about 200 years (Thorpe et al., 2010).
The maintenance of hypo- or avascularity certainly requires either the production of antiangiogenic factors or the inhibition of proangiogenic factors. Indeed both mechanisms have been described in e.g., the hypovascular zones of sheathed tendons (Pufe et al., 2005). The proangiogenic protein vascular endothelial growth factor (VEGF) is found to be highly expressed in cells from fetal and injured human tendons, however only low expression is evident in intact adult tendons (Pufe et al., 2001) (Figure 1A). The antiangiogenic factor endostatin, a proteolytic fragment of Collagen XVIII, is also involved in tendon vascularization. The distribution of endostatin in gliding tendons correlates with the grade of vascularization. Endostatin expression is strong in the gliding area and reduced in areas without pressure and the expression levels are described to be influenced by mechanical load (Pufe et al., 2003) (Figure 1B). Taken together, our understanding of the molecular machinery controlling the complex vascularization process in tendons however remains fragmentary.
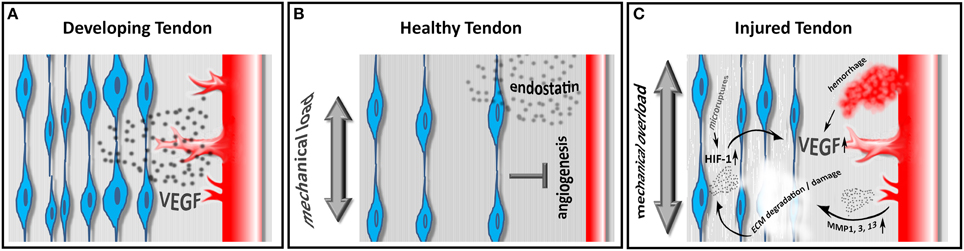
Figure 1. Embryonic tendons show a high density of tendon cells producing VEGF, ultimately resulting in a pronounced angiogenic response in developing tendons (A). In healthy adult tendons the relative cell density decreases and tenocytes produce the antiangiogenic factor endostatin in response to physiological mechanical load, thus limiting neo-angiogenesis (B). In diseased tendons, tenocytes produce HIF-1 in response to mechanical overload and/ or hypoxia. HIF-1 in turn induces the expression of VEGF, promoting neoangiogenesis. Hemorrhage due to vascular injury also leads to an increase in VEGF and to the production of matrix metalloproteinases (MMPs), resulting in a further weakening and degradation of the tendon matrix (C).
Lymphatic Drainage of Tendons
So far, the lymphatic drainage of intact tendons has gained very little attention. Early literature reports lymphatic vessels to be associated with blood vessels in calf tendons as demonstrated by injection of India ink (Edwards, 1946). However, by now it is commonly accepted that the identification of lymph vessels simply by morphologic parameters is insufficient and even by means of immunohistochemistry it is challenging to separate blood from lymph vessels. A minimum of three lymph associated markers is recommended to distinguish lymph from blood vessels (Schroedl et al., 2014). Lymphatics play a crucial role in tissue repair mechanisms, due to injury or inflammatory processes and it has long been known that lymph vessels proliferate during inflammation. However, the role of lymphatic vessels in tendon disease has been neglected so far. To our knowledge there is no literature available on the lymphatic drainage in common tendon disorders such as tendinopathy, calcific tendinitis, or chronic tendon inflammation due to mechanic overuse. Recently, we have shown that intact rat Achilles tendons are void of lymphatics, which start to grow into the tendon repair tissue upon injury (Tempfer et al., 2015). Whether lymphatic ingrowth is a cause for impaired tissue quality and scar formation or merely a side effect requires further investigation. This may pave the way for future attempts to target lymphatic vessels to improve tendon regeneration, as it is successfully performed in other, non-musculoskeletal diseases, such as corneal and ocular surface inflammation (Bock et al., 2013).
Tendon Vessels as a Niche for Stem/Progenitor Cells
As with many other tissues, vertebrate tendons harbor a population of stem/ progenitor cells (Salingcarnboriboon et al., 2003; Bi et al., 2007; Tempfer et al., 2009). These cells display mesenchymal stem cell (MSC)—like properties, displaying plastic adherence and a differentiation potential toward the osteoblast, adipocyte, and chondrocyte lineage. Further, they express classical MSC—associated surface markers such as Stro-1, CD44, CD90, and CD146 (Salingcarnboriboon et al., 2003; Bi et al., 2007; Rui et al., 2013) and reside within a fibromodulin- and biglycan-rich niche (Bi et al., 2007). We have shown that perivascular cells of the human supraspinatus tendon harbor a population of cells expressing both tendon- and stem cell associated markers (Tempfer et al., 2009). This is well in line with the finding that perivascular cells derived from a variety of tissues, such as skeletal muscle, pancreas, adipose tissue, and placenta show MSC–properties (Crisan et al., 2008). Interestingly, Mienaltowski et al. (2013) describe the presence of two distinct stem/progenitor cell populations within tendons, either located in the paratenon or the tendon proper. Both cell sources were negative for the perivascular surface marker CD133 and a differential expression pattern for the vascular marker endomucin as well as for tenomodulin and scleraxis. These data suggest that different stem/progenitor cell populations exist within distinct niches at the tendon proper and peritenon and the stem/progenitor cells in the peritenon might be more vascular in origin.
Generally, the role of perivascular MSCs in regeneration remains a matter of debate. Caplan A.I. proposes these cells to be activated upon local injury and to act as immunomodulatory agents guiding inflammation and regeneration (Caplan, 2008). Particularly in tendon, the function of resident stem/ progenitor cells remains unclear. As tendons naturally only have a poor regenerative capacity, leading to inferior tissue quality following injury or chronic degeneration, the pool of endogenous stem/progenitor cells apparently fails to functionally restore the damaged tissue within the milieu of an injured tendon (Voleti et al., 2012). Actually, tendon stem/progenitor cells are suspected to contribute to tendon degeneration by differentiation toward the osteoblast/chondrocyte lineage (Magne and Bougault, 2015). In diseases such as calcific tendinitis or in the formation of bony enthesophytes in spondyloarthritis, ectopic bone is formed within tendons. As tendon stem/progenitor cells have an even higher potential to undergo osteogenic differentiation than bone marrow derived MSC (Bi et al., 2007), it is speculated that they at least contribute to the pathogenesis of calcific tendinitis (Rui et al., 2011). Taken together, the in vivo role of tendon stem cells remains largely unknown and they potentially contribute to both tendon homeostasis and tendon pathologies by direct cell differentiation and/or production of trophic factors.
Nevertheless, transplantation of bone-marrow stromal cells and tendon-derived stem/progenitor cells has been proven to be beneficial for the functional repair of tendon tissue in various animal models (reviewed by Docheva et al., 2015). Recently, Lee CH et al. have shown that a rare tendon resident population of perivascular cells expressing CD146 can be expanded and stimulated in vivo by connective tissue growth factor (CTGF) in order to regenerate a tendon defect in a rat model (Lee et al., 2015). However, in order to fully harness the regenerative capacity of tendon stem cells we need to gain further insight into the in vivo identity of these cells and how they are modulated by the local niche. So far, this remains experimentally challenging due to the lack of tendon-specific markers.
Vasculature in Tendon Disease
Tendon Adhesion Formation
Peritendinous adhesions often lead to significant functional impairment after tendon surgery. Particularly sheathed tendons, such as the flexor tendon of the hand, frequently lose their gliding capacity after surgical repair, with a prevalence of ~ 4% being reported (Dy et al., 2012). In a rabbit study, three main factors have been identified, which in combination support the formation of adhesions: (i) suture of the partially damaged tendon, (ii) excision of the synovial sheath, and (iii) immobilization. If only one of these factors is avoided, adhesion formation can be significantly reduced (Matthews and Richards, 1976).
As nutrition of sheathed tendons is mainly provided by diffusion from the synovial membrane, the local loss of this tissue combined with a fibrin clot on the avascular outer layer of the tendon causes invasion of microvessels resulting in the formation of fibrous adhesions (Pennington, 1979). More recently, tendon adhesion formation using a mouse model for flexor tendon injury has been demonstrated to follow a typical wound healing response, with overlapping phases of inflammation, vessel ingrowth, and an increase in apoptotic cells over a follow-up time-period of 120 days (Wong et al., 2009).
Attempts to block adhesion formation by merely “wrapping” the tendon with organic or inorganic materials failed as the tendon proper became necrotic in many cases, indicating the importance of vascular supply (Weckesser and Shaw, 1949; Chaplin, 1973). Current research-based strategies include the use of multilayer membranes loaded with non-steroidal anti-inflammatory drugs (NSAIDs) to prevent fibrosis, mimicking the synovial membrane (Jiang et al., 2015) as well as the implantation of bioengineered synovia—like membranes (Baymurat et al., 2015).
Vasculature in Achilles Tendinopathy
Tendinopathy is a painful, chronic disease commonly affecting various tendons such as the Achilles tendon or the tendons of the lateral elbow (“Tennis elbow”). As Achilles tendinopathy (AT) is the most frequent and best studied form of this disease, we will focus on this particular tendon. AT often affects people with high levels of sports activities. For example, 52% of elite long-distance runners are at risk for sustaining an Achilles tendon injury during their career (Kujala et al., 2014). AT is characterized by pain in the tendon during initial loading, subsiding with continued activity; as the condition becomes chronic, pain can be persistent. Overuse is considered to be the underlying cause; however the etiology and pathogenesis have not yet been fully clarified. Similarly, the source of the pain and the underlying mechanisms of pain remain unclear. Histologically, matrix disruption is commonly observed in AT, but is not necessarily involved in the pathogenesis as it also occurs in asymptomatic tendons (Magnan et al., 2014). Neovascularization is commonly seen in AT, as shown by Doppler sonography (Ohberg et al., 2001; Zanetti et al., 2003) and along with the ingrowth of vessels, also innervation is enhanced in tendinopathic zones, which may be causative for the pain associated with AT (Alfredson et al., 2001).
As mentioned above, during tendon development, high levels of VEGF are expressed. Molecules that are developmentally regulated are often re-expressed during the disease state. Indeed, the expression of VEGF in degenerative and spontaneously ruptured Achilles tendons is detectable at high concentrations when compared with adult, healthy Achilles tendons (Pufe et al., 2001). In vitro, cyclic mechanical load induces the expression of VEGF and hypoxia inducible factor 1 (HIF-1) in a frequency dependent fashion, indicating this mechanism being involved in tendon cell response to overload (Petersen et al., 2006) (Figure 1C).
Some authors report AT to be a result of an inadequate repair process following microtrauma, i.e., due to overuse. Because of the lack of blood vessels within the mid portion of the tendon a neurogenic inflammatory process is activated to repair these microruptures. This neurogenic inflammation occurs in the tissue surrounding the Achilles tendon and matrix and induces the expression of metalloproteinases (MMPs) responsible for the degradation of extracellular matrix. Concomitantly, cytokines such as VEGF, epidermal growth factor (EGF), and platelet-derived growth factor (PDGF) are overexpressed. VEGF not only promotes angiogenesis, but also upregulates the expression of MMPs and downregulates tissue inhibitors of metalloproteinases (TIMP-3), further progressing the remodeling of the tendon tissue (van Sterkenburg and van Dijk, 2011).
Tendon stem/ progenitor cells are also suspected to contribute to the pathophysiology of tendinopathies. In a rabbit tendinopathy model it was shown that tendon stem/ progenitor cells display an altered cell fate in vitro. They proliferate less and have a greater potential do undergo osteogenic and chondrogenic differentiation (Rui et al., 2011). Indeed, mineralization processes are also found in human AT and patella tendinopathy. One study on human tendinopathic tissue demonstrated that some mineralized deposits in Achilles and patella tendons are formed by a process resembling endochondral ossification, with bone formation and remodeling mediated by populations of osteoblasts and osteoclasts (Fenwick et al., 2002).
Regarding treatment strategies for AT, eccentric loading (e.g., muscle movements leading to muscle and tendon elongation) has shown to be a safe, cheap, and effective method to reduce pain and to improve tendon structure (Beyer et al., 2015). Interestingly, this method also reduces the number of neovessels in the affected area, which is considered to be causative for the beneficial outcome (Ohberg and Alfredson, 2004). Similarly, a combination of cryotherapy and compression of the tendinopathic area was shown to be an effective treatment, leading to a significant reduction of tendon blood flow (Knobloch et al., 2006). Also the use of topical nitroglycerin and low level laser irradiation are discussed to exert their positive effects by affecting tendon microcirculation. Nitroglycerin is a vasodilator and the positive effects reported are likely due to improved clearance of metabolic products, whereas low level laser irradiation may cause microthrombosis and/ or partial destruction of neovessels. However, for both therapies the underlying mechanisms of action remain poorly understood (Knobloch, 2008).
Another area often affected is the adult enthesis organ that connects tendons with bone, allowing the transmission of force from muscle to bone. The Achilles enthesis is frequently affected by non-inflammatory enthesopathies due to overuse or microtraumas and inflammation may occur resulting in major pain and disability. However, the underlying intrinsic and extrinsic factors also remain poorly understood.
Generally, mineralized and non-mineralized entheses can be differentiated. The Achilles tendon enthesis organ is mineralized and four zones can be distinguished: Zone 1 is built of dense fibrous connective tissue (the tendon proper), and the extracellular matrix (ECM) is mainly composed of collagen type I and III. Zone 2 consists of non-mineralized fibrocartilage and fibrochondrocytes. Here the ECM is formed by aggrecan and the collagen types I, II, and III. Zone 3 is formed of mineralized fibrocartilage with fibrochondrocytes, the predominant collagen being Col II next to I and X as well as calcium phosphate crystals. Between Zone 2 and Zone 3 the so called tidemark forms the boundary between soft, non-mineralized, and hard, mineralized tissue. Zone 4 finally is made up of the bone itself (Benjamin and McGonagle, 2009; Apostolakos et al., 2014). This gradual transition from compliant soft tissue to rigid bone absorbs local stress concentrations minimizing the risk of injury. Further, it has been reported that there is no direct cellular communication between bone and tendon tissue and the fibrocartilage at the enthesis acts as a barrier between cells in the two tissues (Ralphs et al., 1998). Whereas osteocytes and tendon cells directly communicate via gap junctions, the fibrochondrocytes in the calcified fibrocartilage zone were shown to lack similar structures.
Other than tendon, in a healthy state the enthesis is avascular (Dörfl, 1969). The lack of blood vessels reflects the compressive forces to which fibrocartilage is subject: vessel lumina would be occluded by compression. However, intratendinous vessels can anastomose directly with those of the bone at fibrous entheses (Benjamin and McGonagle, 2001). Similar to tendon, in development the enthesis is vascularized. During the growing period, bone grows into the tendon by endochondral ossification, where fibrocartilage is replaced by bone. Generally, cartilage erosion must be preceded by vascular invasion, yet it remains unclear how the blood vessels in the fibrous zone regress as the fibrous tissue is replaced by fibrocartilage (Gao et al., 1996; Benjamin and McGonagle, 2001).
Conclusions
Neovascularization is critical to tissue repair and wound healing. Therefore, strategies to enhance vascularization to promote regeneration are considered promising treatment modalities, i.e., the use of platelet rich plasma (PRP) to restore functional bone (Zhang et al., 2013) or skin (Kakudo et al., 2011). However, in acute or chronic tendon injuries hypervascularity often does not pave the way to functional recovery of the tissue. Therefore, to overcome the limited intrinsic regeneration capacity of tendon and to achieve scarless healing will most likely require a balanced manipulation of the angiogenic response in tendon tissue. For a variety of treatment methods, such as the use of PRP, the availability of clinical data is limited, due to heterogeneity in application (Khan and Bedi, 2015). In order to develop rational strategies to achieve a well-balanced angiogenic response following tendon injury, we need a thorough understanding of the molecular and cellular networks driving tendon vascularization and regeneration—a challenge for years to come.
Author Contributions
HT and AT wrote the article.
Funding
This work was supported by the Lorenz Böhler Funds (Vienna, Austria) and by the Paracelsus Medical University Research Funds (Salzburg, Austria) and the Hermann and Marianne Straniak foundation (Sarnen, Switzerland).
Conflict of Interest Statement
The authors declare that the research was conducted in the absence of any commercial or financial relationships that could be construed as a potential conflict of interest.
References
Ahmed, I. M., Lagopoulos, M., McConnell, P., Soames, R. W., and Sefton, G. K. (1998). Blood supply of the Achilles tendon. J. Orthop. Res. 16, 591–596. doi: 10.1002/jor.1100160511
Alfredson, H., Forsgren, S., Thorsen, K., Fahlltröm, M., Johannson, M., and Lorentzon, R. (2001). Glutamate NMDAR1 receptors localised to nerves in human Achilles tendons. Implications for treatment. Knee Surg. Sports Traumatol. Arthrosc. 9, 334–338. doi: 10.1007/s001670000188
Apostolakos, J., Durant, T. J., Dwyer, C. R., Russell, R. P., Weinreb, J. H., Alaee, F., et al. (2014). The enthesis: a review of the tendon-to-bone insertion. Muscles Ligaments Tendons J. 4, 333–342. doi: 10.11138/mltj/2014.4.3.333
Baymurat, A. C., Ozturk, A. M., Yetkin, H., Ergun, M. A., Helvacıoglu, F., Ozkızılcık, A., et al. (2015). Bio-engineered synovial membrane to prevent tendon adhesions in rabbit flexor tendon model. J. Biomed. Mater Res. A 103, 84–90. doi: 10.1002/jbm.a.35151
Benjamin, M., and McGonagle, D. (2001). The anatomical basis for disease localisation in seronegative spondyloarthropathy at entheses and related sites. J. Anat. 199, 503–526. doi: 10.1046/j.1469-7580.2001.19950503.x
Benjamin, M., and McGonagle, D. (2009). Entheses: tendon and ligament attachment sites. Scand. J. Med. Sci. Sports 19, 520–527. doi: 10.1111/j.1600-0838.2009.00906.x
Beyer, R., Kongsgaard, M., Hougs Kjær, B., Øhlenschlæger, T., Kjær, M., and Magnusson, S. P. (2015). Heavy slow resistance versus eccentric training as treatment for achilles tendinopathy: a randomized controlled trial. Am. J. Sports Med. 43, 1704–1711. doi: 10.1177/0363546515584760
Bi, Y., Ehirchiou, D., Kilts, T. M., Inkson, C. A., Embree, M. C., Sonoyama, W., et al. (2007). Identification of tendon stem/progenitor cells and the role of the extracellular matrix in their niche. Nat. Med. 13, 1219–1227. doi: 10.1038/nm1630
Bock, F., Maruyama, K., Regenfuss, B., Hos, D., Steven, P., Heindl, L. M., et al. (2013). Novel anti(lymph)angiogenic treatment strategies for corneal and ocular surface diseases. Prog. Retin. Eye Res. 34, 89–124. doi: 10.1016/j.preteyeres.2013.01.001
Brockis, J. G. (1953). The blood supply of the flexor and extensor tendons of the fingers in man. Bone Joint Surg. Br. 35-B(1), 131–138.
Caplan, A. I. (2008). All MSCs are pericytes? Cell Stem Cell 3, 229–230. doi: 10.1016/j.stem.2008.08.008
Chaplin, D. M. (1973). The vascular anatomy within normal tendons, divided tendons, free tendon grafts and pedicle tendon grafts in rabbits. A microradioangiographic study. J. Bone Joint Surg. Br. 55, 369–389.
Clancy, W. G. Jr. Narechania, R. G., Rosenberg, T. D., Gmeiner, J. G., Wisnefske, D. D., and Lange, T. A. (1981). Anterior and posterior cruciate ligament reconstruction in rhesus monkeys. J. Bone Joint Surg. Am. 63, 1270–1284.
Crisan, M., Yap, S., Casteilla, L., Chen, C. W., Corselli, M., Park, T. S., et al. (2008). A perivascular origin for mesenchymal stem cells in multiple human organs. Cell Stem Cell 3, 301–313. doi: 10.1016/j.stem.2008.07.003
Docheva, D., Müller, S. A., Majewski, M., and Evans, C. H. (2015). Biologics for tendon repair. Adv. Drug Deliv. Rev. 84, 222–239. doi: 10.1016/j.addr.2014.11.015
Dörfl, J. (1969). Vessels in the region of tendinous insertions. I. Chondroapophyseal insertion. Folia Morphologica 17, 74–78.
Dy, C. J., Hernandez-Soria, A., Ma, Y., Roberts, T. R., and Daluiski, A. (2012). Complications after flexor tendon repair: a systematic review and meta-analysis. J. Hand Surg. Am. 37, 543–551. doi: 10.1016/j.jhsa.2011.11.006
Edwards, D. A. (1946). The blood supply and lymphatic drainage of tendons. J. Anat. 80(Pt 3), 147–152.2.
Fenwick, S., Harrall, R., Hackney, R., Bord, S., Horner, A., Hazleman, B., et al. (2002). Endochondral ossification in Achilles and patella tendinopathy. Rheumatology (Oxford) 41, 474–476. doi: 10.1093/rheumatology/41.4.474
Gao, J., Messner, K., Ralphs, J. R., and Benjamin, M. (1996). An immunohistochemical study of enthesis development in the medial collateral ligament of the rat knee joint. Anat. Embryol. 194, 399–406. doi: 10.1007/BF00198542
Heinemeier, K. M., Schjerling, P., Heinemeier, J., Magnusson, S. P., and Kjaer, M. (2013). Lack of tissue renewal in human adult Achilles tendon is revealed by nuclear bomb (14)C. FASEB J. 27, 2074–2079. doi: 10.1096/fj.12-225599
Ippolito, E., Natali, P. G., Postacchini, F., Accinni, L., and De Martino, C. (1980). Morphological, immunochemical, and biochemical study of rabbit achilles tendon at various ages J. Bone Joint Surg. Am. 62, 583–598.
Jiang, S., Yan, H., Fan, D., Song, J., and Fan, C. (2015). Multi-layer electrospun membrane mimicking tendon sheath for prevention of tendon adhesions. Int. J. Mol. Sci. 16, 6932–6944. doi: 10.3390/ijms16046932
Józsa, L. G., and Kannus, P. (1997). Embryonal and Postnatal Development of Tendons and their Disturbance Human Tendons in: Human Tendons- Anatomy, Physiology and Pathology. Champaign, IL: Human Kinetics.
Kakudo, N., Kushida, S., Minakata, T., Suzuki, K., and Kusumoto, K. (2011). Platelet-rich plasma promotes epithelialization and angiogenesis in a splitthickness skin graft donor site. Med. Mol. Morphol. 44, 233–236. doi: 10.1007/s00795-010-0532-1
Kannus, P. (2000). Structure of the tendon connective tissue. Scand. J. Med. Sci. Sports 10, 312–320. doi: 10.1034/j.1600-0838.2000.010006312.x
Khan, M., and Bedi, A. (2015). Chochrane in COOR®: Platelet-rich therapies for muskuloskeletal soft tissue injuries (Review). Clin. Orthop. Relat. Res. 473, 2207–2213. doi: 10.1007/s11999-015-4207-z
Knobloch, K. (2008). The role of tendon microcirculation in Achilles and patellar tendinopathy. J. Orthop. Surg. Res. 3:18. doi: 10.1186/1749-799X-3-18
Knobloch, K., Grasemann, R., Jagodzinski, M., Richter, M., Zeichen, J., and Krettek, C. (2006). Changes of Achilles midportion tendon microcirculation after repetitive simultaneous cryotherapy and compression using a Cryo/Cuff. Am. J. Sports Med. 34, 1953–1959. doi: 10.1177/0363546506293701
Kujala, U. M., Sarna, S., and Kaprio, J. (2014). Cumulative incidence of achilles tendon rupture and tendinopathy in male former elite athletes. Clin. J. Sport Med. 15, 133–135. doi: 10.1097/01.jsm.0000165347.55638.23
Lee, C. H., Lee, F. Y., Tarafder, S., Kao, K., Jun, Y., Yang, G., et al. (2015). Harnessing endogenous stem/progenitor cells for tendon regeneration. J. Clin. Invest. 125, 2690–2701. doi: 10.1172/JCI81589
Lindblom, K. (1939). On pathogenesis of ruptures of tendon aponeuroses of the shoulder joint. Acta Radiol. 20, 563–577. doi: 10.3109/00016923909174877
Magnan, B., Bondi, M., Pierantoni, S., and Samaila, E. (2014). The pathogenesis of Achilles tendinopathy: a systematic review. Foot Ankle Surg. 20, 154–159. doi: 10.1016/j.fas.2014.02.010
Magne, D., and Bougault, C. (2015). What understanding tendon cell differentiation can teach us about pathological tendon ossification. Histol. Histopathol. 30, 901–910. doi: 10.14670/HH-11-614
Matthews, P., and Richards, H. (1976). Factors in the adherence of flexor tendon after repair: an experimental study in the rabbit. J. Bone Joint Surg. Br. 58, 230–236.
Meller, R., Schiborra, F., Brandes, G., Knobloch, K., Tschernig, T., Hankemeier, S., et al. (2009). Postnatal maturation of tendon, cruciate ligament, meniscus and articular cartilage: a histological study in sheep. Ann. Anat. 191, 575–585. doi: 10.1016/j.aanat.2009.08.005
Mienaltowski, M. J., Adams, S. M., and Birk, D. E. (2013). Regional differences in stem cell/progenitor cell populations from the mouse Achilles tendon. Tissue Eng. Part A 19, 199–210 doi: 10.1089/ten.TEA.2012.0182
Ohberg, L., and Alfredson, H. (2004). Effects on neovascularisation behind the good results with eccentric training in chronic mid-portion Achilles tendinosis? Knee Surg. Sports Traumatol. Arthrosc. 12, 465–470. doi: 10.1007/s00167-004-0494-8
Ohberg, L., Lorentzon, R., and Alfredsn, H. (2001). Neovascularisation in Achilles tendons with painful tendinosis but not in normal tendons: an ultrasonographic investigation. Knee Surg. Sports Traumatol. Arthrosc. 9, 235–238. doi: 10.1007/s001670000189
Oryan, A., and Shoushtari, A. H. (2008). Histology and ultrastructure of the developing superficial digital flexor tendon in rabbits Anat. Histol. Embryol. 37, 134–140. doi: 10.1111/j.1439-0264.2007.00811.x
Pang, J., Shen, S., Pan, W. R., Jones, I. R., Rozen, W. M., and Taylor, G. I. (2009). The arterial supply of the patellar tendon: anatomical study with clinical implications for knee surgery. Clin. Anat. 22, 371–376. doi: 10.1002/ca.20770
Peacock, E. E. Jr. (1959). A study of the circulation in normal tendons and healing grafts. Ann. Surg. 149, 415–428. doi: 10.1097/00000658-195903000-00011
Pennington, D. G. (1979). The influence of tendon sheath integrity and vincular blood supply on adhesion formation following tendon repair in hens. Br. J. Plast. Surg. 32, 302–306. doi: 10.1016/0007-1226(79)90085-7
Petersen, W., Varoga, D., Zantop, T., Hassenpflug, J., Mentlein, R., and Pufe, T. (2006). Cyclic strain influences the expression of the vascular endothelial growth factor (VEGF) and the hypoxia inducible factor 1 alpha (HIF-1alpha) in tendon fibroblasts. J. Orthop. Res. 22, 847–853. doi: 10.1016/j.orthres.2003.11.009
Pufe, T., Petersen, W., Kurz, B., Tsokos, M., Tillmann, B., and Mentlein, R. (2003). Mechanical factors influence the expression of endostatin—an inhibitor of angiogenesis—in tendons. J. Orthop. Res. 21, 610–616. doi: 10.1016/S0736-0266(02)00262-0
Pufe, T., Petersen, W., Tillmann, B., and Mentlein, R. (2001). The angiogenic peptide vascular endothelial growth factor is expressed in foetal and ruptured tendons. Virchows Arch. 439, 579–585. doi: 10.1007/s004280100422
Pufe, T., Petersen, W. J., Mentlein, R., and Tillmann, B. N. (2005). The role of vasculature and angiogenesis for the pathogenesis of degenerative tendons disease. Scand. J. Med. Sci. Sports 15, 211–222. doi: 10.1111/j.1600-0838.2005.00465.x
Ralphs, J. R., Benjamin, M., Waggett, A. D., Russell, D. C., Messner, K., and Gao, J. (1998). Regional differences in cell shape and gap junction expression in rat Achilles tendon: relation to fibrocartilage differentiation. J. Anat. 193(Pt 2), 215–222. doi: 10.1046/j.1469-7580.1998.19320215.x
Rathbun, J. B., and MacNab, I. (1970). The microvascular pattern of the rotator cuff. J. Bone Joint Surg. Br. 52, 540–553.
Rui, Y. F., Lui, P. P., Chan, L. S., Chan, K. M., Fu, S. C., and Li, G. (2011). Does erroneous differentiation of tendon-derived stem cells contribute to the pathogenesis of calcifying tendinopathy? Chin. Med. J. 124, 606–610. doi: 10.3760/cma.j.issn.0366-6999.2011.04.022
Rui, Y. F., Lui, P. P., Wong, Y. M., Tan, Q., and Chan, K. M. (2013). Altered fate of tendon-derived stem cells isolated from a failed tendon-healing animal model of tendinopathy. Stem Cells Dev. 22, 1076–1085. doi: 10.1089/scd.2012.0555
Salingcarnboriboon, R., Yoshitake, H., Tsuji, K., Obinata, M., Amagasa, T., Nifuji, A., et al. (2003). Establishment of tendon-derived cell lines exhibiting pluripotent mesenchymal stem cell-like property. Exp. Cell Res. 287, 289–300. doi: 10.1016/S0014-4827(03)00107-1
Schmidt-Rohlfing, B., Graf, J., Schneider, U., and Niethard, F. U. (1992). The blood supply of the Achilles tendon. Int. Orthop. 16, 29–31. doi: 10.1007/BF00182980
Schroedl, F., Kaser-Eichberger, A., Schlereth, S. L., Bock, F., Regenfuss, B., Reitsamer, H. A., et al. (2014). Consensus statement on the immunohistochemical detection of ocular lymphatic vessels. Invest. Ophthalmol. Vis. Sci. 55, 6440–6442. doi: 10.1167/iovs.14-15638
Stein, V., Laprell, H., Tinnemeyer, S., and Petersen, W. (2000). Quantitative assessment of intravascular volume of the human Achilles tendon. Acta Orthop. Scand. 71, 60–63. doi: 10.1080/00016470052943919
Tempfer, H., Kaser-Eichberger, A., Korntner, S., Lehner, C., Kunkel, N., Traweger, A., et al. (2015). Presence of lymphatics in a rat tendon lesion model. Histochem. Cell Biol. 143, 411–419. doi: 10.1007/s00418-014-1287-x
Tempfer, H., Wagner, A., Gehwolf, R., Lehner, C., Tauber, M., Resch, H., et al. (2009). Perivascular cells of the supraspinatus tendon express both tendon- and stem cell-related markers. Histochem. Cell Biol. 131, 733–741. doi: 10.1007/s00418-009-0581-5
Thorpe, C. T., Streeter, I., Pinchbeck, G. L., Goodship, A. E., Clegg, P. D., and Birch, H. L. (2010). Aspartic acid racemization and collagen degradation markers reveal an accumulation of damage in tendon collagen that is enhanced with aging. J. Biol. Chem. 285, 15674–15681. doi: 10.1074/jbc.M109.077503
van Sterkenburg, M. N., and van Dijk, C. N. (2011). Mid-portion Achilles tendinopathy: why painful? An evidence-based philosophy. Knee Surg. Sports Traumatol. Arthrosc. 19, 1367–1375. doi: 10.1007/s00167-011-1535-8
Voleti, P. B., Buckley, M. R., and Soslowsky, L. J. (2012). Tendon healing: repair and regeneration. Annu. Rev. Biomed. Eng. 14, 47–71. doi: 10.1146/annurev-bioeng-071811-150122
Weckesser, E. C., and Shaw, B. W. (1949). A comparative study of various substances for the prevention of adhesions about tendons. Surgery 25, 361–369.
Wong, J. K., Lui, Y. H., Kapacee, Z., Kadler, K. E., Ferguson, M. W., and McGrouther, D. A. (2009). The cellular biology of flexor tendon adhesion formation: an old problem in a new paradigm. Am. J. Pathol. 175, 1938–1951. doi: 10.2353/ajpath.2009.090380
Zanetti, M., Metzdorf, A., Kundert, H. P., Zollinger, H., Vienne, P., Seifert, B., et al. (2003). Achilles tendons: clinical relevance of neovascularization diagnosed with power Doppler, U.S. Radiology 227, 556–560. doi: 10.1148/radiol.2272012069
Keywords: tendon vasculature, tendon stem/progenitor cells, tendinopathy, lymphatics, tendon regeneration
Citation: Tempfer H and Traweger A (2015) Tendon Vasculature in Health and Disease. Front. Physiol. 6:330. doi: 10.3389/fphys.2015.00330
Received: 11 September 2015; Accepted: 02 November 2015;
Published: 18 November 2015.
Edited by:
Michael A. Hill, University of Missouri, USAReviewed by:
Jessica E. Wagenseil, Washington University in St. Louis, USASofia Avnet, Istituto Ortopedico Rizzoli, Italy
P. Bryant Chase, The Florida State University, USA
Copyright © 2015 Tempfer and Traweger. This is an open-access article distributed under the terms of the Creative Commons Attribution License (CC BY). The use, distribution or reproduction in other forums is permitted, provided the original author(s) or licensor are credited and that the original publication in this journal is cited, in accordance with accepted academic practice. No use, distribution or reproduction is permitted which does not comply with these terms.
*Correspondence: Herbert Tempfer, aGVyYmVydC50ZW1wZmVyQHBtdS5hYy5hdA==