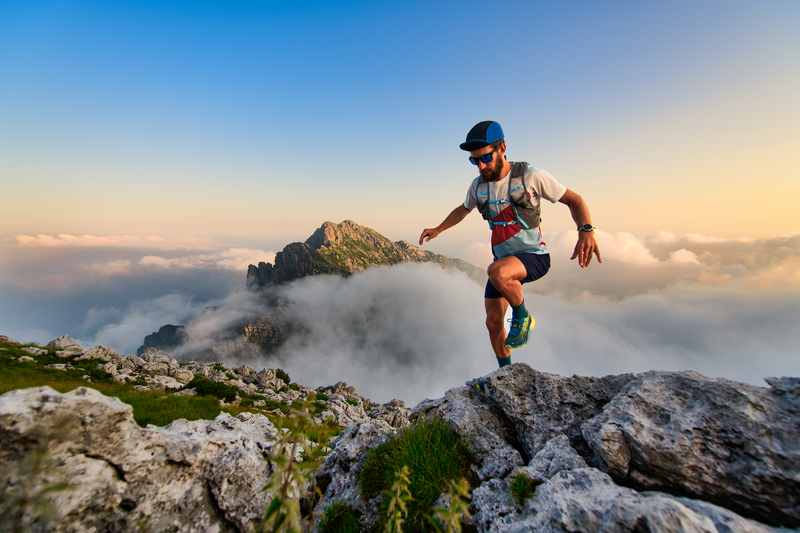
95% of researchers rate our articles as excellent or good
Learn more about the work of our research integrity team to safeguard the quality of each article we publish.
Find out more
MINI REVIEW article
Front. Physiol. , 29 July 2015
Sec. Striated Muscle Physiology
Volume 6 - 2015 | https://doi.org/10.3389/fphys.2015.00211
This article is part of the Research Topic Mitochondria in Skeletal Muscle Health, Aging and Diseases View all 14 articles
Inorganic nitrate is present at high levels in beetroot and celery, and in green leafy vegetables such as spinach and lettuce. Though long believed inert, nitrate can be reduced to nitrite in the human mouth and, further, under hypoxia and/or low pH, to nitric oxide. Dietary nitrate has thus been associated favorably with nitric-oxide-regulated processes including blood flow and energy metabolism. Indeed, the therapeutic potential of dietary nitrate in cardiovascular disease and metabolic syndrome—both aging-related medical disorders—has attracted considerable recent research interest. We and others have shown that dietary nitrate supplementation lowers the oxygen cost of human exercise, as less respiratory activity appears to be required for a set rate of skeletal muscle work. This striking observation predicts that nitrate benefits the energy metabolism of human muscle, increasing the efficiency of either mitochondrial ATP synthesis and/or of cellular ATP-consuming processes. In this mini-review, we evaluate experimental support for the dietary nitrate effects on muscle bioenergetics and we critically discuss the likelihood of nitric oxide as the molecular mediator of such effects.
Inorganic nitrate (NO) has long been considered an undesirable food component and pollutant of drinking water as nitrosation of secondary amines may produce carcinogenic N-nitrosamines (Magee and Barnes, 1956). However, the evidence that NO causes human cancers is weak and dietary NO, as e.g. found in beetroot and spinach, has instead been linked to many physiological benefits (Gilchrist et al., 2010). Humans do not only get NO from their diet as it is also generated endogenously (Tannenbaum et al., 1978) by oxidation of nitric oxide (NO) formed canonically via the L-arginine/NO synthase pathway (Moncada and Higgs, 1993). Importantly, inorganic NO can be reduced to nitrite (NO) and then NO, which offers an additional path of mammalian NO production that, unlike the canonical route, is independent of oxygen (O2) (Lundberg et al., 2008). NO is widely believed to mediate the benefits of NO (Lundberg et al., 2009) including protection against cardiovascular disease (Omar and Webb, 2014) and the metabolic syndrome (Carlström et al., 2010). It has recently been found that dietary NO lowers the O2 cost of human exercise as less respiratory activity is required for a set rate of skeletal muscle work (Larsen et al., 2007, 2010; Bailey et al., 2009, 2010). This finding is interesting as it challenges exercise physiology dogma that the steady-state O2 consumption for any individual is immutable at a given sub-maximal workload irrespective of age, fitness, diet, or pharmacological intervention (Poole and Richardson, 1997). This mini-review aims to evaluate the mechanistic understanding of NO effects on skeletal muscle function.
In a seminal publication, Larsen and colleagues reported that a 3-day supplementation with 0.1 mmol·kg−1 NaNO3· day−1 lowered pulmonary O2 uptake () by ~3–5% in humans completing sub-maximal cycling exercise (Larsen et al., 2007). Bailey and co-workers subsequently observed a 5% lower during low-intensity cycling exercise and a 16% improvement in the tolerable duration of high-intensity exercise over days 3–6 of a 6-day supplementation period with 5.6 mmol NO day−1, administered as 500 mL NO-rich beetroot juice· day−1 (Bailey et al., 2009). Importantly, NO-depleted beetroot juice does not improve exercise economy and performance (Lansley et al., 2011) eliminating antioxidants and polyphenols (Wootton-Beard and Ryan, 2011) as exclusive “active ingredients”. NO-induced improvements have been observed in humans completing walking, running, cycling, rowing and kayaking exercise, and positive responses arise both acutely, i.e., 1–3 h after NO ingestion, and after prolonged NO supplementation over 3–15 days (Table 1). Acute (2.5 h post-ingestion) lowering of during low-intensity exercise is progressively greater at 4.2, 8.4, and 16.8 mmol NO, whereas high-intensity exercise tolerance is unaffected by 4.2 mmol NO, but acutely improved to a similar extent by 8.4 and 16.8 mmol (Wylie et al., 2013). Therefore, short-term supplementation (≥3 days) with at least 5 mmol NO day−1, or acute ingestion of at least 8.4 mmol NO, might represent an effective dietary intervention to improve the economy and performance of human locomotion, at least in healthy, moderately fit adults (Porcelli et al., 2014). Since effects on resting are equivocal (Bailey et al., 2010; Kelly et al., 2013b; Larsen et al., 2014), NO benefits may be exclusive to contracting skeletal muscles.
Physiological NO effects appear muscle-fiber-type-specific as evidenced by improved perfusion (Ferguson et al., 2014) and calcium handling (Hernández et al., 2012) of murine fast-twitch type II but not slow-twitch type I muscle. Consistent with this, NO benefit on adjustment following the onset of exercise and on tolerance to high-intensity exercise is relatively large when the contribution of type II muscle fibers to force production is increased in human skeletal muscle (Breese et al., 2013; Bailey et al., 2015). These preferential physiological effects may relate to the comparably low microvascular PO2 in resting and contracting type II muscle (McDonough et al., 2005). Indeed, NO improves exercise economy and performance in hypoxia (Masschelein et al., 2012; Muggeridge et al., 2014) more markedly than in normoxia (Kelly et al., 2014). Importantly, NO attenuates the degree of exercise intolerance and the slowing of PCr recovery kinetics in hypoxia to the levels seen in normoxia (Vanhatalo et al., 2011). It thus appears that exercise economy and performance benefit most from NO when muscle O2 availability is low.
Although the majority of studies in healthy adults observe NO-improved exercise economy and/or performance, the effects are attenuated in well-trained endurance athletes (Bescós et al., 2012; Peacock et al., 2012; Christensen et al., 2013; Boorsma et al., 2014; Hoon et al., 2014; Lane et al., 2014), inconsistent in diseased populations (Berry et al., 2014; Kerley et al., 2015; Leong et al., 2015; Shepherd et al., 2015; Zamani et al., 2015), and possibly different in aging humans (Kelly et al., 2013b). More generally, there is evidence of distinct NO responders and non-responders in many studies. The relative efficacy of dietary NO effects on skeletal muscle thus appears variable, which underscores the need for detailed mechanistic understanding. To aid such understanding, it is important to ascertain how the human body processes dietary NO.
When humans eat NO-rich food, NO is converted to NO by nitrate reductases in commensal bacteria that reside in the posterior part of the tongue (Duncan et al., 1995). Salivary NO is rapidly protonated in the acidic environment of the stomach resulting in the formation of NO and other reactive nitrogen species (RNS) including nitrogen dioxide (NO2), nitrous acid (HNO2), and dinitrogen trioxide (N2O3) (Benjamin et al., 1994; Lundberg et al., 1994; Lundberg and Weitzberg, 2013). NO ingestion increases plasma NO levels in human subjects (e.g., Lundberg and Govoni, 2004; Webb et al., 2008; Bailey et al., 2009; Vanhatalo et al., 2010; Kelly et al., 2013a). Possibly catalyzed by xanthine oxidase (Zhang et al., 1998; Li et al., 2003) and/or deoxyhaemoglobin (Cosby et al., 2003; Gladwin et al., 2004; Gladwin and Kim-Shapiro, 2008), NO is reduced to NO under conditions of low oxygen tension (Figure 1). Other sites of NO reductase activity include cytochrome c (Basu et al., 2008) and mitochondrial respiratory complexes III (Kozlov et al., 1999) and IV (Castello et al., 2006). However, mammalian NO reductase activity has only been shown in vitro and in animal models (Feelisch et al., 2008; Jansson et al., 2008), and under low PO2 (Li et al., 2001; Feelisch et al., 2008) and low pH (Modin et al., 2001). Generally, the low pKa of NO (3.34, Oxtoby and Nachtrieb, 1996) limits its physiological reduction, which is an inefficient process per se (Li et al., 2008) and thus requires high NO concentrations. Indeed, at physiological NO levels (see below), even hypoxic red blood cells do not liberate significant NO (Bryan et al., 2004). O2 competitively inhibits NO reduction by xanthine oxidase (Li et al., 2004) and oxygenated haem effectively scavenges free NO (Feelisch et al., 2008). NO may be converted to N2O3 that in turn may react with free thiols to generate S-nitrosothiols (Hess et al., 2005) via an S-nitrosation reaction (Figure 1). NO is also able to modify proteins through nitrosylation, e.g., via reaction with the haem of myoglobin (Ignarro, 1991). NO furthermore binds, in a reversible and O2-competitive manner, to the haem of cytochrome c oxidase, and in an O2-independent way, to the enzyme's active site copper (Giulivi et al., 2006; Brown and Borutaite, 2007; Cooper and Giulivi, 2007). Peroxynitrite (ONOO−) arising from the reaction of NO with the superoxide anion radical may undergo a nitration reaction with tyrosine residues to form 3-nitrotyrosine (Figure 1—Radi, 2004). Importantly, tyrosine-containing proteins are also nitrated in a myeloperoxidase-catalyzed reaction using NO and hydrogen peroxide (Marquez and Dunford, 1995).
Figure 1. Putative mechanism by which dietary NO may lower the O2 cost of human exercise. Dietary NO increases plasma NO and NO levels thus improving efficiency of skeletal muscle ATP supply by oxidative phosphorylation and/or of ATP turnover. Effects on the bioenergetics of skeletal muscle cells may be direct or indirect through formation of NO. Shown reactions are examples of RNS-induced protein modifications (see text for details).
Physiological NO levels range from 50 to 500 nM in human plasma (Bryan et al., 2004; Dejam et al., 2005; Feelisch et al., 2008) and a mean concentration of 12 μM has been measured in human skin (Mowbray et al., 2009). In rodents, NO concentration varies substantially between tissues, from below quantifiable limit in the liver, heart and lung to as high as 2 μM in kidney and 3.7 μM in lymph nodes; NO varies from 1 μM in the kidney to 50 μM in the aorta (Garcia-Saura et al., 2010). In humans, the ingestion of 10 mg NaNO3·kg−1 has been shown to increase mean plasma NO within 90 min from 30 to 432 μM and mean plasma NO from 123 to 392 nM (Lundberg and Govoni, 2004). Similarly, 500 mL beetroot juice containing 45 mM NO on average raises mean plasma NO to 380 μM and NO to 600 nM within 30 min and 3 h of ingestion, respectively (Webb et al., 2008). Plasma NO and NO reach peak concentrations, respectively, 1–2 and 2–3 h post-ingestion, and NO gradually returns to its base level after about 24 h (Ender et al., 1964; McKnight et al., 1997; Webb et al., 2008; Wylie et al., 2013). Mammalian tissue NO and NO both have in vivo half-lives of tens of minutes (Bryan et al., 2005). The half-life of NO in whole human blood is only about 110 sec (Kelm, 1999) as it is rapidly oxidized to NO; the half-life of NO in blood is 5–8 h (Wagner et al., 1983; McKnight et al., 1997). About 60% of ingested NO is excreted by the kidneys (Green et al., 1981; Wagner et al., 1983).
The reliability of commonly reported NO and NO values very much depends on the assays used to measure these inorganic anions. The modified Griess reaction using sulfanilamide and N-1-napthylethylenediamine dihydrochloride is a frequently used assay for measuring NO (Tsikas, 2007). Plasma NO concentrations are readily determined using this spectrophotometric assay following NO reduction to NO by cadmium (Green et al., 1982) or vanadium salts (Miranda et al., 2001). However, the Griess test lacks the sensitivity to probe the nanomolar NO levels present in human plasma. Ozone-based chemiluminescence is a preferred method of detection, which often involves deproteinisation of plasma samples by zinc sulfate precipitation before analysis (Higuchi and Motomizu, 1999). NO measurement by chemiluminescence usually involves acetic acid/sodium iodide-mediated reduction to NO, which then reacts with ozone to produce a chemiluminescence signal (Bateman et al., 2002). NO can also be measured this way by reduction to NO via reflux of the sample in vanadium chloride at 95°C. Confounding the NO/NO literature, in some assays NO is reduced to NO by bacterial nitrate reductases (Sun et al., 2003) whose activity varies from batch to batch. Confusing matters further, mere “NOx” values are reported to denote the sum of NO and NO levels. A last analytical note concerns the use of NO-depleted beetroot juice as placebo control in in vivo studies (see Section O2 Cost of Human Exercise). It is important for experiments involving human participants to use a placebo juice that looks, tastes, and smells the same as the “real thing”. A placebo that meets these criteria can be prepared by passing beetroot juice through a Purolite a520e anion exchange column, which effectively and selectively removes NO (Gilchrist et al., 2013).
Dietary NO benefits on the O2 cost of exercise likely arise from increased efficiency of ATP synthesis and/or of skeletal muscle work (Figure 1). Indeed, NO increases the rate of human skeletal muscle PCr recovery after exercise in hypoxia suggesting an augmented maximum rate of oxidative ATP synthesis (Vanhatalo et al., 2011), and lowers the ATP cost of contractile force production (Bailey et al., 2010). These in vivo studies confirm that NO indeed affects skeletal muscle bioenergetics, but they do not disclose the underlying molecular mechanism. In vitro experiments with C2C12 myocytes show that beetroot juice provokes mitochondrial biogenesis and modestly increases basal cellular respiration without affecting respiratory capacity and proton leak (Vaughan et al., 2014). These observations indicate improved mitochondrial coupling efficiency as beetroot juice has increased the proportion of total O2 consumption coupled to ATP synthesis. Although the C2C12 experiments lack an appropriate NO-depleted beetroot juice control (see above), increased coupling efficiency of oxidative phosphorylation agrees with data reported by Larsen et al. (2011), who show that skeletal muscle mitochondria isolated from NO-supplemented subjects exhibit higher respiratory control and P/O ratios (defined in Brand and Nicholls, 2011) than mitochondria from non-supplemented controls, and that increases in P/O ratio correlate with NO-induced decreases in whole-body O2 uptake during exercise. This increased efficiency of ATP synthesis in isolated mitochondria, however, emerges from decreased respiration linked to mitochondrial proton leak, not from stimulated O2 uptake coupled to phosphorylation (Larsen et al., 2011). NO-lowered proton leak coincides with decreases in adenine nucleotide translocase protein and, to a lesser extent, uncoupling protein-3 (Larsen et al., 2011). It should be emphasized that these mitochondrial carriers do not necessarily contribute to proton leak (Nedergaard and Cannon, 2003; Vozza et al., 2014) and that leak is expected to account for little skeletal muscle respiration at low protonmotive force (Affourtit and Brand, 2006), i.e., the bioenergetic state attained during exercise. Dietary NO also lowers the apparent affinity of mitochondrial respiration for O2, an effect that is reproduced in vitro—acutely and pH-dependently—by NO (Larsen et al., 2011). Lowered affinity is attributed to an NO-induced rise in the apparent Km of cytochrome c oxidase for O2 (Larsen et al., 2011) but, inconsistently, NO does not affect mitochondrial respiration or efficiency (Larsen et al., 2011) like NO is expected to (Brown and Borutaite, 2007). Apparent mitochondrial respiratory affinity for O2 depends strongly on the extent to which respiration is controlled by the enzyme reacting with O2 (Affourtit et al., 2001)—control of cytochrome c oxidase over O2 consumption may well have been affected by NO and pH, and also by dietary NO-induced mitochondrial changes.
It remains to be demonstrated convincingly whether or not dietary NO effects in skeletal muscle are mediated by NO. Nitrite reductase activity requires high NO levels and exceptionally low PO2 and pH (see Section Molecular Fate of Dietary NO) that may indeed manifest in the ischaemic heart (Brown and Borutaite, 2007; Hendgen-Cotta et al., 2010), but are unlikely in healthy muscle. In contracting muscle, myoglobin O2 saturation remains as high as 50% (Takakura et al., 2015) and although globins indeed exhibit nitrite reductase activity at this saturation (Huang et al., 2005), cytoplasmic NO will likely be scavenged by oxymyoglobin (Hendgen-Cotta et al., 2010). Even if O2 were sufficiently low for NO reduction in exercising muscle, we stress that dietary NO intake remodels skeletal muscle bioenergetics in the hours to days before exercise (see Section O2 Cost of Human Exercise), i.e., when the muscles are at rest. Importantly, NO also modulates cell signaling independently of NO in hypoxia and normoxia (Bryan et al., 2005). NO activates AMPK in rat aortic smooth muscle cells thus stimulating mitochondrial biogenesis, and increasing coupling efficiency and cellular respiratory control (Mo et al., 2012). NO activates PKA in cultured cardiomyocytes, stimulating mitochondrial fusion and again increasing cellular respiratory control (Pride et al., 2013). In both systems, NO improves efficiency of oxidative ATP synthesis without apparent effect on proton leak, which agrees with the beetroot juice effects on C2C12 respiration (Vaughan et al., 2014). NO also activates PKA in cultured adipocytes, increasing mitochondrial fusion, and stimulating glucose uptake (Khoo et al., 2014). Moreover, NO increases proliferation of muscle (Totzeck et al., 2014) and epithelial cells (Wang et al., 2012).
RNS can modify proteins (see Section Molecular fate of dietary NO) and may thus improve mitochondrial coupling efficiency in various ways, e.g., by increasing proton translocation to electron transfer stoichiometries of respiratory complexes (cf. Clerc et al., 2007). By definition (Brand and Nicholls, 2011), coupling efficiency benefits from decreased proton leak and increased phosphorylation-linked respiration, as indeed reported by (Larsen et al., 2011) and (Vaughan et al., 2014), respectively. System-kinetic modeling furthermore suggests that substrate oxidation capacity, which is dependent on fuel and O2 availability, correlates positively with coupling efficiency (Affourtit and Brand, 2009). Dietary NO may thus improve efficiency of muscle ATP synthesis, at least in part, by increasing expression of glucose transporters (Jiang et al., 2014) and/or by raising insulin availability (Nyström et al., 2012).
Dietary NO increases the contractile force of fast-twitch mouse muscle by improving calcium handling (Hernández et al., 2012) suggesting the efficiency of ATP-demanding contraction may have increased. To our knowledge, no other data are available on the mechanism by which dietary NO affects ATP turnover. However, NO supplementation may also alter efficiency of other ATP-consumers and, importantly, the relative importance of dietary NO effects on skeletal muscle ATP supply and ATP turnover remains unclear. A systems-level functional analysis of cellular energy metabolism (cf. Brand, 1998) may shed light on these issues. Using myocytes isolated from human muscle biopsies (Nisr and Affourtit, 2014) the relative effects of RNS on ATP-generating and ATP-consuming fluxes—linked through the cell's phosphorylation potential (Figure 1)—may be identified and quantified in an unbiased manner. A challenge of such in vitro analysis will be the approximation of physiologically meaningful conditions, in particular the O2 tensions and energy demands that prevail during the development of dietary NO benefits.
The striking benefit of dietary NO on the O2 cost of exercise is of obvious interest to athletes (Jones, 2014), but may also well impact on the quality of life of aging people suffering from muscle weakness and exercise intolerance. To rationally evaluate translational potential, our mechanistic understanding of dietary NO benefits on human skeletal muscle needs to be improved. By integrating biochemistry and physiology, and studying subjects at different age, it will be important to demonstrate which reactive nitrogen species mediate dietary NO effects at the cellular level, disclose all effects of nitrogen species on myocellular bioenergetics, confirm if they are direct or indirect via action on other tissues, and quantify the relative importance of these effects.
CA wrote Sections Introduction, Skeletal Muscle Bioenergetics, and Conclusion, and produced the table and figure; SB and AJ wrote Section O2 Cost of Human Exercise; MS and PW wrote Section Molecular Fate of Dietary NO. All authors edited and approved the entire manuscript.
The development of a placebo beetroot juice preparation in PGW's laboratory was supported by James White Drinks Ltd. Otherwise, we confirm that this mini-review was written in the absence of any commercial or financial relationships that could be construed as a potential conflict of interest.
Research in the authors' laboratories is currently supported by the Medical Research Council (New Investigator Research Grant G1100165/1 to CA), Gatorade Sports Science Institute (Grants PEP-1330 and PEP-1420 to AJ), the Exeter Leukaemia Fund (Grant ST-06354 to AJ), the Dunhill Medical Trust (Grant R269/1112 to AJ) and the NIHR Exeter Clinical Research Facility (PW and MS). The views and opinions shown within this paper are those of the authors and do not necessarily represent those of the NIHR, NHS or the Department of Health.
AMPK, AMP-activated kinase; PCr, phosphocreatine; PKA, protein kinase A; PO2, partial oxygen tension; RNS, reactive nitrogen species; , pulmonary oxygen uptake.
Affourtit, C., and Brand, M. D. (2006). Stronger control of ATP/ADP by proton leak in pancreatic beta-cells than skeletal muscle mitochondria. Biochem. J. 393, 151–159. doi: 10.1042/BJ20051280
Affourtit, C., and Brand, M. D. (2009). Measuring mitochondrial bioenergetics in INS-1E insulinoma cells. Meth. Enzymol. 457, 405–424. doi: 10.1016/S0076-6879(09)05023-X
Affourtit, C., Krab, K., and Moore, A. L. (2001). Control of plant mitochondrial respiration. Biochim. Biophys. Acta 1504, 58–69. doi: 10.1016/S0005-2728(00)00239-5
Bailey, S. J., Fulford, J., Vanhatalo, A., Winyard, P. G., Blackwell, J. R., DiMenna, F. J., et al. (2010). Dietary nitrate supplementation enhances muscle contractile efficiency during knee-extensor exercise in humans. J. Appl. Physiol. 109, 135–148. doi: 10.1152/japplphysiol.00046.2010
Bailey, S. J., Varnham, R. L., Dimenna, F. J., Breese, B. C., Wylie, L. J., and Jones, A. M. (2015). Inorganic nitrate supplementation improves muscle oxygenation, O2 uptake kinetics, and exercise tolerance at high but not low pedal rates. J. Appl. Physiol. 118, 1396–1405. doi: 10.1152/japplphysiol.01141.2014
Bailey, S. J., Winyard, P., Vanhatalo, A., Blackwell, J. R., Dimenna, F. J., Wilkerson, D. P., et al. (2009). Dietary nitrate supplementation reduces the O2 cost of low-intensity exercise and enhances tolerance to high-intensity exercise in humans. J. Appl. Physiol. 107, 1144–1155. doi: 10.1152/japplphysiol.00722.2009
Basu, S., Azarova, N. A., Font, M. D., King, S. B., Hogg, N., Gladwin, M. T., et al. (2008). Nitrite reductase activity of cytochrome c. J. Biol. Chem. 283, 32590–32597. doi: 10.1074/jbc.M806934200
Bateman, R. M., Ellis, C. G., and Freeman, D. J. (2002). Optimization of nitric oxide chemiluminescence operating conditions for measurement of plasma nitrite and nitrate. Clin. Chem. 48, 570–573.
Benjamin, N., O'Driscoll, F., Dougall, H., Duncan, C., Smith, L., Golden, M., et al. (1994). Stomach NO synthesis. Nature 368, 502. doi: 10.1038/368502a0
Berry, M. J., Justus, N. W., Hauser, J. I., Case, A. H., Helms, C. C., Basu, S., et al. (2014). Dietary nitrate supplementation improves exercise performance and decreases blood pressure in COPD patients. Nitric Oxide. 48, 22–30. doi: 10.1016/j.niox.2014.10.007
Bescós, R., Ferrer-Roca, V., Galilea, P. A., Roig, A., Drobnic, F., Sureda, A., et al. (2012). Sodium nitrate supplementation does not enhance performance of endurance athletes. Med. Sci. Sports Exerc. 44, 2400–2409. doi: 10.1249/MSS.0b013e3182687e5c
Bond, H., Morton, L., and Braakhuis, A. J. (2012). Dietary nitrate supplementation improves rowing performance in well-trained rowers. Int. J. Sport Nutr. Exerc. Metab. 22, 251–256.
Boorsma, R. K., Whitfield, J., and Spriet, L. L. (2014). Beetroot juice supplementation does not improve performance of elite 1500-m runners. Med. Sci. Sports Exerc. 46, 2326–2334. doi: 10.1249/MSS.0000000000000364
Brand, M. D. (1998). Top-down elasticity analysis and its application to energy metabolism in isolated mitochondria and intact cells. Mol. Cell. Biochem. 184, 13–20. doi: 10.1023/A:1006893619101
Brand, M. D., and Nicholls, D. G. (2011). Assessing mitochondrial dysfunction in cells. Biochem. J. 435, 297–312. doi: 10.1042/BJ20110162
Breese, B. C., McNarry, M. A., Marwood, S., Blackwell, J. R., Bailey, S. J., and Jones, A. M. (2013). Beetroot juice supplementation speeds O2 uptake kinetics and improves exercise tolerance during severe-intensity exercise initiated from an elevated metabolic rate. Am. J. Physiol. Regul. Integr. Comp. Physiol. 305, R1441–R1450. doi: 10.1152/ajpregu.00295.2013
Brown, G., and Borutaite, V. (2007). Nitric oxide and mitochondrial respiration in the heart. Cardiovasc. Res. 75, 283–290. doi: 10.1016/j.cardiores.2007.03.022
Bryan, N. S., Fernandez, B. O., Bauer, S. M., Garcia-Saura, M. F., Milsom, A. B., Rassaf, T., et al. (2005). Nitrite is a signaling molecule and regulator of gene expression in mammalian tissues. Nat. Chem. Biol. 1, 290–297. doi: 10.1038/nchembio734
Bryan, N. S., Rassaf, T., Maloney, R. E., Rodriguez, C. M., Saijo, F., Rodriguez, J. R., et al. (2004). Cellular targets and mechanisms of nitros(yl)ation: an insight into their nature and kinetics in vivo. Proc. Natl. Acad. Sci. U.S.A. 101, 4308–4313. doi: 10.1073/pnas.0306706101
Carlström, M., Larsen, F. J., Nyström, T., Hezel, M., Borniquel, S., Weitzberg, E., et al. (2010). Dietary inorganic nitrate reverses features of metabolic syndrome in endothelial nitric oxide synthase-deficient mice. Proc. Natl. Acad. Sci. U.S.A. 107, 17716–17720. doi: 10.1073/pnas.1008872107
Castello, P. R., David, P. S., McClure, T., Crook, Z., and Poyton, R. O. (2006). Mitochondrial cytochrome oxidase produces nitric oxide under hypoxic conditions: implications for oxygen sensing and hypoxic signaling in eukaryotes. Cell Metab. 3, 277–287. doi: 10.1016/j.cmet.2006.02.011
Cermak, N. M., Gibala, M. J., and van Loon, L. J. C. (2012). Nitrate supplementation's improvement of 10-km time-trial performance in trained cyclists. Int. J. Sport Nutr. Exerc. Metab. 22, 64–71.
Christensen, P. M., Nyberg, M., and Bangsbo, J. (2013). Influence of nitrate supplementation on VO2 kinetics and endurance of elite cyclists. Scand. J. Med. Sci. Sports 23, e21–e31. doi: 10.1111/sms.12005
Clerc, P., Rigoulet, M., Leverve, X., and Fontaine, E. (2007). Nitric oxide increases oxidative phosphorylation efficiency. J. Bioenerg. Biomembr. 39, 158–166. doi: 10.1007/s10863-007-9074-1
Cooper, C. E., and Giulivi, C. (2007). Nitric oxide regulation of mitochondrial oxygen consumption II: molecular mechanism and tissue physiology. Am. J. Physiol. Cell Physiol. 292, C1993–C2003. doi: 10.1152/ajpcell.00310.2006
Cosby, K., Partovi, K. S., Crawford, J. H., Patel, R. P., Reiter, C. D., Martyr, S., et al. (2003). Nitrite reduction to nitric oxide by deoxyhemoglobin vasodilates the human circulation. Nat. Med. 9, 1498–1505. doi: 10.1038/nm954
Dejam, A., Hunter, C. J., Pelletier, M. M., Hsu, L. L., Machado, R. F., Shiva, S., et al. (2005). Erythrocytes are the major intravascular storage sites of nitrite in human blood. Blood 106, 734–739. doi: 10.1182/blood-2005-02-0567
Duncan, C., Dougall, H., Johnston, P., Green, S., Brogan, R., Leifert, C., et al. (1995). Chemical generation of nitric oxide in the mouth from the enterosalivary circulation of dietary nitrate. Nat. Med. 1, 546–551. doi: 10.1038/nm0695-546
Ender, F., Havre, G., Helgebostad, A., Koppang, N., Madsen, R., and Ceh, L. (1964). Isolation and identification of a hepatotoxic factor in herring meal produced from sodium nitrite preserved herring. Naturwissenschaften 51, 637–638. doi: 10.1007/BF00623677
Feelisch, M., Fernandez, B. O., Bryan, N. S., Garcia-Saura, M. F., Bauer, S., Whitlock, D. R., et al. (2008). Tissue processing of nitrite in hypoxia: an intricate interplay of nitric oxide-generating and -scavenging systems. J. Biol. Chem. 283, 33927–33934. doi: 10.1074/jbc.M806654200
Ferguson, S. K., Holdsworth, C. T., Wright, J. L., Fees, A. J., Allen, J. D., Jones, A. M., et al. (2014). Microvascular oxygen pressures in muscles comprised of different fiber types: impact of dietary nitrate supplementation. Nitric Oxide. 48, 38–43. doi: 10.1016/j.niox.2014.09.157
Garcia-Saura, M. F., Fernandez, B. O., McAllister, B. P., Whitlock, D. R., Cruikshank, W. W., and Feelisch, M. (2010). Dermal nitrite application enhances global nitric oxide availability: new therapeutic potential for immunomodulation? J. Invest. Dermatol. 130, 608–611. doi: 10.1038/jid.2009.303
Gilchrist, M., Winyard, P. G., Aizawa, K., Anning, C., Shore, A., and Benjamin, N. (2013). Effect of dietary nitrate on blood pressure, endothelial function, and insulin sensitivity in type 2 diabetes. Free Radic. Biol. Med. 60, 89–97. doi: 10.1016/j.freeradbiomed.2013.01.024
Gilchrist, M., Winyard, P. G., and Benjamin, N. (2010). Dietary nitrate – good or bad? Nitric Oxide 22, 104–109. doi: 10.1016/j.niox.2009.10.005
Giulivi, C., Kato, K., and Cooper, C. E. (2006). Nitric oxide regulation of mitochondrial oxygen consumption I: cellular physiology. Am. J. Physiol. Cell Physiol. 291, C1225–C1231. doi: 10.1152/ajpcell.00307.2006
Gladwin, M. T., Crawford, J. H., and Patel, R. P. (2004). The biochemistry of nitric oxide, nitrite, and hemoglobin: role in blood flow regulation. Free Radic. Biol. Med. 36, 707–717. doi: 10.1016/j.freeradbiomed.2003.11.032
Gladwin, M. T., and Kim-Shapiro, D. B. (2008). The functional nitrite reductase activity of the heme-globins. Blood 112, 2636–2647. doi: 10.1182/blood-2008-01-115261
Green, L. C., Ruiz de Luzuriaga, K., Wagner, D. A., Rand, W., Istfan, N., Young, V. R., et al. (1981). Nitrate biosynthesis in man. Proc. Natl. Acad. Sci. U.S.A. 78, 7764–7768. doi: 10.1073/pnas.78.12.7764
Green, L. C., Wagner, D. A., Glogowski, J., Skipper, P. L., Wishnok, J. S., and Tannenbaum, S. R. (1982). Analysis of nitrate, nitrite, and [15N]nitrate in biological fluids. Anal. Biochem. 126, 131–138. doi: 10.1016/0003-2697(82)90118-X
Hendgen-Cotta, U. B., Flogel, U., Kelm, M., and Rassaf, T. (2010). Unmasking the Janus face of myoglobin in health and disease. J. Exp. Biol. 213, 2734–2740. doi: 10.1242/jeb.041178
Hernández, A., Schiffer, T. A., Ivarsson, N., Cheng, A. J., Bruton, J. D., Lundberg, J. O., et al. (2012). Dietary nitrate increases tetanic [Ca 2+] iand contractile force in mouse fast-twitch muscle. J. Physiol. 590, 3575–3583. doi: 10.1113/jphysiol.2012.232777
Hess, D. T., Matsumoto, A., Kim, S.-O., Marshall, H. E., and Stamler, J. S. (2005). Protein S-nitrosylation: purview and parameters. Nat. Rev. Mol. Cell Biol. 6, 150–166. doi: 10.1038/nrm1569
Higuchi, K., and Motomizu, S. (1999). Flow-injection spectrophotometric determination of nitrite and nitrate in biological samples. Anal. Sci. 15, 129–134. doi: 10.2116/analsci.15.129
Hoon, M. W., Hopkins, W. G., Jones, A. M., Martin, D. T., Halson, S. L., West, N. P., et al. (2014). Nitrate supplementation and high-intensity performance in competitive cyclists. Appl. Physiol. Nutr. Metab. 39, 1043–1049. doi: 10.1139/apnm-2013-0574
Huang, Z., Shiva, S., Kim-Shapiro, D. B., Patel, R. P., Ringwood, L. A., Irby, C. E., et al. (2005). Enzymatic function of hemoglobin as a nitrite reductase that produces NO under allosteric control. J. Clin. Invest. 115, 2099–2107. doi: 10.1172/JCI24650
Ignarro, L. J. (1991). Signal transduction mechanisms involving nitric oxide. Biochem. Pharmacol. 41, 485–490. doi: 10.1016/0006-2952(91)90618-F
Jansson, E. A., Huang, L., Malkey, R., Govoni, M., Nihlén, C., Olsson, A., et al. (2008). A mammalian functional nitrate reductase that regulates nitrite and nitric oxide homeostasis. Nat. Chem. Biol. 4, 411–417. doi: 10.1038/nchembio.92
Jiang, H., Torregrossa, A. C., Potts, A., Pierini, D., Aranke, M., Garg, H. K., et al. (2014). Dietary nitrite improves insulin signaling through GLUT4 translocation. Free Radic. Biol. Med. 67, 51–57. doi: 10.1016/j.freeradbiomed.2013.10.809
Jones, A. M. (2014). Dietary nitrate supplementation and exercise performance. Sports Med. 44, S35–S45. doi: 10.1007/s40279-014-0149-y
Kelly, J., Fulford, J., Vanhatalo, A., Blackwell, J. R., French, O., Bailey, S. J., et al. (2013a). Effects of short-term dietary nitrate supplementation on blood pressure, O2 uptake kinetics, and muscle and cognitive function in older adults. Am. J. Physiol. Reg. Int. Comp. Physiol. 304, R73–R83. doi: 10.1152/ajpregu.00406.2012
Kelly, J., Vanhatalo, A., Bailey, S. J., Wylie, L. J., Tucker, C., List, S., et al. (2014). Dietary nitrate supplementation: effects on plasma nitrite and pulmonary O2 uptake dynamics during exercise in hypoxia and normoxia. Am. J. Physiol. Reg. Int. Comp. Physiol. 307, R920–R930. doi: 10.1152/ajpregu.00068.2014
Kelly, J., Vanhatalo, A., Wilkerson, D. P., Wylie, L. J., and Jones, A. M. (2013b). Effects of nitrate on the power-duration relationship for severe-intensity exercise. Med. Sci. Sports Exerc. 45, 1798–1806. doi: 10.1249/MSS.0b013e31828e885c
Kelm, M. (1999). Nitric oxide metabolism and breakdown. Biochim. Biophys. Acta 1411, 273–289. doi: 10.1016/S0005-2728(99)00020-1
Kerley, C. P., Cahill, K., Bolger, K., McGowan, A., Burke, C., Faul, J., et al. (2015). Dietary nitrate supplementation in COPD: an acute, double-blind, randomized, placebo-controlled, crossover trial. Nitric Oxide 44, 105–111. doi: 10.1016/j.niox.2014.12.010
Khoo, N. K. H., Mo, L., Zharikov, S., Kamga-Pride, C., Quesnelle, K., Golin-Bisello, F., et al. (2014). Nitrite augments glucose uptake in adipocytes through the protein kinase A-dependent stimulation of mitochondrial fusion. Free Radic. Biol. Med. 70, 45–53. doi: 10.1016/j.freeradbiomed.2014.02.009
Kozlov, A. V., Staniek, K., and Nohl, H. (1999). Nitrite reductase activity is a novel function of mammalian mitochondria. FEBS Lett. 454, 127–130. doi: 10.1016/S0014-5793(99)00788-7
Lane, S. C., Hawley, J. A., Desbrow, B., Jones, A. M., Blackwell, J. R., Ross, M. L., et al. (2014). Single and combined effects of beetroot juice and caffeine supplementation on cycling time trial performance. Appl. Physiol. Nutr. Metab. 39, 1050–1057. doi: 10.1139/apnm-2013-0336
Lansley, K. E., Winyard, P. G., Bailey, S. J., Vanhatalo, A., Wilkerson, D. P., Blackwell, J. R., et al. (2011). Acute dietary nitrate supplementation improves cycling time trial performance. Med. Sci. Sports Exerc. 43, 1125–1131. doi: 10.1249/MSS.0b013e31821597b4
Larsen, F. J., Schiffer, T. A., Borniquel, S., Sahlin, K., Ekblom, B., Lundberg, J. O., et al. (2011). Dietary inorganic nitrate improves mitochondrial efficiency in humans. Cell Metab. 13, 149–159. doi: 10.1016/j.cmet.2011.01.004
Larsen, F. J., Schiffer, T. A., Ekblom, B., Mattsson, M. P., Checa, A., Wheelock, C. E., et al. (2014). Dietary nitrate reduces resting metabolic rate: a randomized, crossover study in humans. Am. J. Clin. Nutr. 99, 843–850. doi: 10.3945/ajcn.113.079491
Larsen, F. J., Weitzberg, E., Lundberg, J. O., and Ekblom, B. (2007). Effects of dietary nitrate on oxygen cost during exercise. Acta Physiol. 191, 59–66. doi: 10.1111/j.1748-1716.2007.01713.x
Larsen, F. J., Weitzberg, E., Lundberg, J. O., and Ekblom, B. (2010). Dietary nitrate reduces maximal oxygen consumption while maintaining work performance in maximal exercise. Free Radic. Biol. Med. 48, 342–347. doi: 10.1016/j.freeradbiomed.2009.11.006
Leong, P., Basham, J. E., Yong, T., Chazan, A., Finlay, P., Barnes, S., et al. (2015). A double blind randomized placebo control crossover trial on the effect of dietary nitrate supplementation on exercise tolerance in stable moderate chronic obstructive pulmonary disease. BMC Pulm. Med. 15:52. doi: 10.1186/s12890-015-0057-4
Li, H., Cui, H., Kundu, T. K., Alzawahra, W., and Zweier, J. L. (2008). Nitric oxide production from nitrite occurs primarily in tissues not in the blood: critical role of xanthine oxidase and aldehyde oxidase. J. Biol. Chem. 283, 17855–17863. doi: 10.1074/jbc.M801785200
Li, H., Samouilov, A., Liu, X., and Zweier, J. L. (2001). Characterization of the magnitude and kinetics of xanthine oxidase-catalyzed nitrite reduction. Evaluation of its role in nitric oxide generation in anoxic tissues. J. Biol. Chem. 276, 24482–24489. doi: 10.1074/jbc.M011648200
Li, H., Samouilov, A., Liu, X., and Zweier, J. L. (2003). Characterization of the magnitude and kinetics of xanthine oxidase-catalyzed nitrate reduction: evaluation of its role in nitrite and nitric oxide generation in anoxic tissues. Biochemistry 42, 1150–1159. doi: 10.1021/bi026385a
Li, H., Samouilov, A., Liu, X., and Zweier, J. L. (2004). Characterization of the effects of oxygen on xanthine oxidase-mediated nitric oxide formation. J. Biol. Chem. 279, 16939–16946. doi: 10.1074/jbc.M314336200
Lundberg, J. O., Gladwin, M. T., Ahluwalia, A., Benjamin, N., Bryan, N. S., Butler, A., et al. (2009). Nitrate and nitrite in biology, nutrition and therapeutics. Nat. Chem. Biol. 5, 865–869. doi: 10.1038/nchembio.260
Lundberg, J. O., and Govoni, M. (2004). Inorganic nitrate is a possible source for systemic generation of nitric oxide. Free Radic. Biol. Med. 37, 395–400. doi: 10.1016/j.freeradbiomed.2004.04.027
Lundberg, J. O., and Weitzberg, E. (2013). Biology of nitrogen oxides in the gastrointestinal tract. Gut 62, 616–629. doi: 10.1136/gutjnl-2011-301649
Lundberg, J. O., Weitzberg, E., and Gladwin, M. T. (2008). The nitrate-nitrite-nitric oxide pathway in physiology and therapeutics. Nat. Rev. Drug Disc. 7, 156–167. doi: 10.1038/nrd2466
Lundberg, J. O., Weitzberg, E., Lundberg, J. M., and Alving, K. (1994). Intragastric nitric oxide production in humans: measurements in expelled air. Gut 35, 1543–1546. doi: 10.1136/gut.35.11.1543
Magee, P. N., and Barnes, J. M. (1956). The production of malignant primary hepatic tumours in the rat by feeding dimethylnitrosamine. Br. J. Cancer 10, 114–122. doi: 10.1038/bjc.1956.15
Marquez, L. A., and Dunford, H. B. (1995). Kinetics of oxidation of tyrosine and dityrosine by myeloperoxidase compounds I and II. Implications for lipoprotein peroxidation studies. J. Biol. Chem. 270, 30434–30440. doi: 10.1074/jbc.270.51.30434
Masschelein, E., Van Thienen, R., Wang, X., Van Schepdael, A., Thomis, M., and Hespel, P. (2012). Dietary nitrate improves muscle but not cerebral oxygenation status during exercise in hypoxia. J. Appl. Physiol. 113, 736–745. doi: 10.1152/japplphysiol.01253.2011
McDonough, P., Behnke, B. J., Padilla, D. J., Musch, T. I., and Poole, D. C. (2005). Control of microvascular oxygen pressures in rat muscles comprised of different fibre types. J. Physiol. 563, 903–913. doi: 10.1113/jphysiol.2004.079533
McKnight, G. M., Smith, L. M., Drummond, R. S., Duncan, C. W., Golden, M., and Benjamin, N. (1997). Chemical synthesis of nitric oxide in the stomach from dietary nitrate in humans. Gut 40, 211–214. doi: 10.1136/gut.40.2.211
Miranda, K. M., Espey, M. G., and Wink, D. A. (2001). A rapid, simple spectrophotometric method for simultaneous detection of nitrate and nitrite. Nitric Oxide 5, 62–71. doi: 10.1006/niox.2000.0319
Mo, L., Wang, Y., Geary, L., Corey, C., Alef, M. J., Beer-Stolz, D., et al. (2012). Free Radical Biology and Medicine. Free Radic. Biol. Med. 53, 1440–1450. doi: 10.1016/j.freeradbiomed.2012.07.080
Modin, A., Björne, H., Herulf, M., Alving, K., Weitzberg, E., and Lundberg, J. O. (2001). Nitrite-derived nitric oxide: a possible mediator of “acidic-metabolic” vasodilation. Acta Physiol. Scand. 171, 9–16. doi: 10.1046/j.1365-201x.2001.171001009.x
Moncada, S., and Higgs, A. (1993). The L-arginine-nitric oxide pathway. N. Engl. J. Med. 329, 2002–2012. doi: 10.1056/NEJM199312303292706
Mowbray, M., McLintock, S., Weerakoon, R., Lomatschinsky, N., Jones, S., Rossi, A. G., et al. (2009). Enzyme-independent NO stores in human skin: quantification and influence of UV radiation. J. Invest. Dermatol. 129, 834–842. doi: 10.1038/jid.2008.296
Muggeridge, D. J., Howe, C, C. F., Spendiff, O., Pedlar, C., James, P. E., and Easton, C. (2013). The effects of a single dose of concentrated beetroot juice on performance in trained flatwater kayakers. Int. J. Sport Nutr. Exerc. Metab. 23, 498–506.
Muggeridge, D. J., Howe, C. C. F., Spendiff, O., Pedlar, C., James, P. E., and Easton, C. (2014). A single dose of beetroot juice enhances cycling performance in simulated altitude. Med. Sci. Sports Exerc. 46, 143–150. doi: 10.1249/MSS.0b013e3182a1dc51
Nedergaard, J., and Cannon, B. (2003). The ‘novel’ ‘uncoupling’proteins UCP2 and UCP3: what do they really do? Pros and cons for suggested functions. Exp. Physiol. 88, 65–84. doi: 10.1113/eph8802502
Nisr, R. B., and Affourtit, C. (2014). Insulin acutely improves mitochondrial function of rat and human skeletal muscle by increasing coupling efficiency of oxidative phosphorylation. Biochim. Biophys. Acta 1837, 270–276. doi: 10.1016/j.bbabio.2013.10.012
Nyström, T., Ortsäter, H., Huang, Z., Zhang, F., Larsen, F. J., Weitzberg, E., et al. (2012). Inorganic nitrite stimulates pancreatic islet blood flow and insulin secretion. Free Radic. Biol. Med. 53, 1017–1023. doi: 10.1016/j.freeradbiomed.2012.06.031
Omar, S. A., and Webb, A. J. (2014). Nitrite reduction and cardiovascular protection. J. Mol. Cell. Cardiol. 73, 57–69. doi: 10.1016/j.yjmcc.2014.01.012
Oxtoby, D. W., and Nachtrieb, N. H., eds. (1996). Principles of Modern Chemistry, 3rd Edn. Fort Worth, TX: Hartcourt; Fort Worth Brace.
Peacock, O., Tjønna, A. E., James, P., Wisløff, U., Welde, B., Böhlke, N., et al. (2012). Dietary nitrate does not enhance running performance in elite cross-country skiers. Med. Sci. Sports Exerc. 44, 2213–2219. doi: 10.1249/MSS.0b013e3182640f48
Peeling, P., Cox, G. R., Bullock, N., and Burke, L. M. (2014). Beetroot juice improves on-water 500 m time-trial performance, and laboratory-based paddling economy in national and international-level Kayak athletes. Int. J. Sport Nutr. Exerc. Metab. 25, 278–284. doi: 10.1123/ijsnem.2014-0110
Poole, D. C., and Richardson, R. S. (1997). Determinants of oxygen uptake. Implications for exercise testing. Sports Med. 24, 308–320. doi: 10.2165/00007256-199724050-00003
Porcelli, S., Ramaglia, M., Bellistri, G., Pavei, G., Pugliese, L., Montorsi, M., et al. (2014). Aerobic fitness affects the exercise performance responses to nitrate supplementation. Med. Sci. Sports Exerc. 47, 1643–1651. doi: 10.1249/mss.0000000000000577
Pride, C. K., Mo, L., Quesnelle, K., Dagda, R. K., Murillo, D., Geary, L., et al. (2013). Nitrite activates protein kinase A in normoxia to mediate mitochondrial fusion and tolerance to ischaemia/reperfusion. Cardiovasc. Res. 101, 57–68. doi: 10.1093/cvr/cvt224
Radi, R. (2004). Nitric oxide, oxidants, and protein tyrosine nitration. Proc. Natl. Acad. Sci. U.S.A. 101, 4003–4008. doi: 10.1073/pnas.0307446101
Shepherd, A. I., Gilchrist, M., Winyard, P. G., Jones, A. M., Hallmann, E., Kazimierczak, R., et al. (2015). Effects of dietary nitrate supplementation on the oxygen cost of exercise and walking performance in individuals with type 2 diabetes: a randomised, double blind, placebo-controlled cross-over trial. Free Radic. Biol. Med. 86, 200–208. doi: 10.1016/j.freeradbiomed.2015.05.014
Sun, J., Zhang, X., Broderick, M., and Fein, H. (2003). Measurement of nitric oxide production in biological systems by using griess reaction assay. Sensors 3, 276–284. doi: 10.3390/s30800276
Takakura, H., Furuichi, Y., Yamada, T., Jue, T., Ojino, M., Hashimoto, T., et al. (2015). Endurance training facilitates myoglobin desaturation during muscle contraction in rat skeletal muscle. Sci. Rep. 5:9403. doi: 10.1038/srep09403
Tannenbaum, S. R., Fett, D., Young, V. R., Land, P. D., and Bruce, W. R. (1978). Nitrite and nitrate are formed by endogenous synthesis in the human intestine. Science 200, 1487–1489. doi: 10.1126/science.663630
Totzeck, M., Schicho, A., Stock, P., Kelm, M., Rassaf, T., and Hendgen-Cotta, U. B. (2014). Nitrite circumvents canonical cGMP signaling to enhance proliferation of myocyte precursor cells. Mol. Cell. Biochem. 401, 175–183. doi: 10.1007/s11010-014-2305-y
Tsikas, D. (2007). Analysis of nitrite and nitrate in biological fluids by assays based on the Griess reaction: appraisal of the Griess reaction in the L-arginine/nitric oxide area of research. J. Chrom. B 851, 51–70. doi: 10.1016/j.jchromb.2006.07.054
Vanhatalo, A., Bailey, S. J., Blackwell, J. R., DiMenna, F. J., Pavey, T. G., Wilkerson, D. P., et al. (2010). Acute and chronic effects of dietary nitrate supplementation on blood pressure and the physiological responses to moderate-intensity and incremental exercise. Am. J. Physiol. Reg. Int. Comp. Physiol. 299, R1121–R1131. doi: 10.1152/ajpregu.00206.2010
Vanhatalo, A., Fulford, J., Bailey, S. J., Blackwell, J. R., Winyard, P. G., and Jones, A. M. (2011). Dietary nitrate reduces muscle metabolic perturbation and improves exercise tolerance in hypoxia. J. Physiol. 589, 5517–5528. doi: 10.1113/jphysiol.2011.216341
Vaughan, R. A., Gannon, N. P., and Carriker, C. R. (2014). Nitrate-containing beetroot enhances myocyte metabolism and mitochondrial content. J. Tradit. Complement Med. doi: 10.1016/j.jtcme.2014.11.033. [Epub ahead of print].
Vozza, A., Parisi, G., De Leonardis, F., Lasorsa, F. M., Castegna, A., Amorese, D., et al. (2014). UCP2 transports C4 metabolites out of mitochondria, regulating glucose and glutamine oxidation. Proc. Natl. Acad. Sci. U.S.A. 111, 960–965. doi: 10.1073/pnas.1317400111
Wagner, D. A., Schultz, D. S., Deen, W. M., Young, V. R., and Tannenbaum, S. R. (1983). Metabolic fate of an oral dose of 15N-labeled nitrate in humans: effect of diet supplementation with ascorbic acid. Cancer Res. 43, 1921–1925.
Wang, L., Frizzell, S. A., Zhao, X., and Gladwin, M. T. (2012). Normoxic cyclic GMP-independent oxidative signaling by nitrite enhances airway epithelial cell proliferation and wound healing. Nitric Oxide 26, 203–210. doi: 10.1016/j.niox.2012.03.002
Webb, A. J., Patel, N., Loukogeorgakis, S., Okorie, M., Aboud, Z., Misra, S., et al. (2008). Acute blood pressure lowering, vasoprotective, and antiplatelet properties of dietary nitrate via bioconversion to nitrite. Hypertension 51, 784–790. doi: 10.1161/HYPERTENSIONAHA.107.103523
Wootton-Beard, P. C., and Ryan, L. (2011). A beetroot juice shot is a significant and convenient source of bioaccessible antioxidants. J. Funct. Foods 3, 329–334. doi: 10.1016/j.jff.2011.05.007
Wylie, L. J., Kelly, J., Bailey, S. J., Blackwell, J. R., Skiba, P. F., Winyard, P. G., et al. (2013). Beetroot juice and exercise: pharmacodynamic and dose-response relationships. J. Appl. Physiol. 115, 325–336. doi: 10.1152/japplphysiol.00372.2013
Zamani, P., Rawat, D., Shiva-Kumar, P., Geraci, S., Bhuva, R., Konda, P., et al. (2015). Effect of inorganic nitrate on exercise capacity in heart failure with preserved ejection fraction. Circulation 131, 371–380. doi: 10.1161/CIRCULATIONAHA.114.012957
Zhang, Z., Naughton, D., Winyard, P. G., and Benjamin, N. (1998). Generation of nitric oxide by a nitrite reductase activity of xanthine oxidase: a potential pathway for nitric oxide formation in the absence of nitric oxide synthase activity. Biochem. Biophys. Res. Comm. 249, 767–772. doi: 10.1006/bbrc.1998.9226
Keywords: dietary nitrate, nitrite, nitric oxide, oxygen cost of human exercise, cellular bioenergetics, skeletal muscle mitochondria, coupling efficiency of oxidative phosphorylation, ATP turnover
Citation: Affourtit C, Bailey SJ, Jones AM, Smallwood MJ and Winyard PG (2015) On the mechanism by which dietary nitrate improves human skeletal muscle function. Front. Physiol. 6:211. doi: 10.3389/fphys.2015.00211
Received: 23 June 2015; Accepted: 14 July 2015;
Published: 29 July 2015.
Edited by:
Gilles Gouspillou, Université du Québec à Montréal, CanadaReviewed by:
Sruti Shiva, University of Pittsburgh, USACopyright © 2015 Affourtit, Bailey, Jones, Smallwood and Winyard. This is an open-access article distributed under the terms of the Creative Commons Attribution License (CC BY). The use, distribution or reproduction in other forums is permitted, provided the original author(s) or licensor are credited and that the original publication in this journal is cited, in accordance with accepted academic practice. No use, distribution or reproduction is permitted which does not comply with these terms.
*Correspondence: Charles Affourtit, School of Biomedical and Healthcare Sciences, Plymouth University, Portland Square Building, Drake Circus, PL4 8AA, Plymouth, UK,Y2hhcmxlcy5hZmZvdXJ0aXRAcGx5bW91dGguYWMudWs=
Disclaimer: All claims expressed in this article are solely those of the authors and do not necessarily represent those of their affiliated organizations, or those of the publisher, the editors and the reviewers. Any product that may be evaluated in this article or claim that may be made by its manufacturer is not guaranteed or endorsed by the publisher.
Research integrity at Frontiers
Learn more about the work of our research integrity team to safeguard the quality of each article we publish.