- 1Laboratorio de Fisiología, Departamento de Ciencias Biológicas, Facultad de Ciencias Biológicas y Facultad de Medicina, Universidad Andrés Bello, Santiago, Chile
- 2Centro de Fisiología Celular e Integrativa, Facultad de Medicina, Clínica Alemana – Universidad del Desarrollo, Santiago, Chile
- 3Dirección de Investigación, Universidad Autónoma de Chile, Santiago, Chile
- 4Departamento de Biología, Facultad de Química y Biología, Universidad de Santiago de Chile, Santiago, Chile
- 5BioAdvising, Santiago, Chile
Sepsis progresses to multiple organ dysfunction due to the uncontrolled release of inflammatory mediators, and a growing body of evidence shows that neural signals play a significant role in modulating the immune response. Thus, similar toall other physiological systems, the immune system is both connected to and regulated by the central nervous system. The efferent arc consists of the activation of the hypothalamic–pituitary–adrenal axis, sympathetic activation, the cholinergic anti-inflammatory reflex, and the local release of physiological neuromodulators. Immunosensory activity is centered on the production of pro-inflammatory cytokines, signals that are conveyed to the brain through different pathways. The activation of peripheral sensory nerves, i.e., vagal paraganglia by the vagus nerve, and carotid body (CB) chemoreceptors by the carotid/sinus nerve are broadly discussed here. Despite cytokine receptor expression in vagal afferent fibers, pro-inflammatory cytokines have no significant effect on vagus nerve activity. Thus, the CB may be the source of immunosensory inputs and incoming neural signals and, in fact, sense inflammatory mediators, playing a protective role during sepsis. Considering that CB stimulation increases sympathetic activity and adrenal glucocorticoids release, the electrical stimulation of arterial chemoreceptors may be suitable therapeutic approach for regulating systemic inflammation.
Inflammatory Response and its Regulation
Inflammation can be defined as a “host defense in response to injury of vascularized tissues” (Majno and Joris, 1996). The inflammatory response –the first alert to the signals that address perturbation– involves an innate system of cellular and humoral responses that, following injury, will support the organism in attempts to restore tissue homeostasis (Chaplin, 2010). The inflammatory response involves a complex network of events involving several organizational levels: at the systemic level, the leakage of substances from the vascular compartment (e.g., water, salt, and proteins); at the cellular level, the activation of endothelial cells and macrophages, leukocyte-endothelium adhesive interactions, and the recruitment of leukocytes; at the subcellular level, the activation and aggregation of platelets, the release of proteases and oxidants from phagocytic cells, and the activation of the complement, clotting and fibrinolytic systems. All the above-mentioned events may assist in addressing a state of injury (Alvarez Perez Gil et al., 2012). During the inflammatory response, the number of inflammatory mediators found in the plasma and secreted by cells is broad and includes extracellular Danger-Associated Molecule Patterns (DAMPs), such as cellular debris, potassium, DNA, cytokines, histones and high-mobility group protein B1 (HMGB1), and Pathogen-Associated Molecular Patterns (PAMPs), such as bacterial lipopolysaccharide (LPS, endotoxin) or peptidoglycan, fungal zymosan or viral single stranded RNA (Deutschman and Tracey, 2014). DAMPs are sensed, leading to the assembly of the inflammasome, and DAMPs and PAMPs catalyze the formation of cell surface signalosomes. Both signalosomes and inflammasomes induce apoptosis, intracellular stress and other metabolic responses, such as the expression of pro-inflammatory cytokines (e.g., interleukin (IL)-1β, IL-6, tumor necrosis factor (TNF)-α, and HMGB1), cell proliferation and the amplification of the innate immune response (Lamkanfi et al., 2010).
Reversal or resolution of the inflammatory response implies that leukocytes are removed, either via lymphatics or apoptosis, and that the ongoing acute inflammatory response is terminated. As a consequence, during resolution, increased vascular permeability is reversed, and immune cell emigration from the blood compartment ceases (Rock et al., 2010).
Therefore, to maintain immunological homeostasis, inflammation resolution is essential for inhibiting the release and toxicity of potentially damaging inflammatory mediators (Nathan and Ding, 2010). Moreover, regulatory T cells, alternatively activated macrophages and other cellular mechanisms also suppress excessive immune responses to prevent tissue damage. Numerous anti-inflammatory factors, such as cytokines (e.g., IL-4, IL-10, and transforming growth factor (TGF)-β), protease inhibitors, and antioxidant enzymes, which are present in plasma at very low concentrations, are induced by the immune challenge and are powerful anti-inflammatory factors comprising contain the acute inflammatory response. As a result, these regulatory factors decreased the production of pro-inflammatory mediators and reduce the number of immune cells accumulating in tissues (Buras et al., 2005). Nevertheless, there are several limitations of these humoral and cellular mechanisms: (i) they are slow compared to environmental changes; (ii) they are unable to integrate biological responses to numerous stimulating inputs across a network of immune tissues; and (iii) these mechanisms rely on the circulatory system and thus are anable to respond efficiently in a specific tissue or confined region (Andersson and Tracey, 2012).
An increasing amount of research shows that neural signals play a significant role in modulating the immune response (Glaser and Kiecolt-Glaser, 2005). In fact, both immune-suppression and immune-enhancement can be behaviorally modified in experimental animals (Cohen et al., 1994). Therefore, the central nervous system (CNS) communicates with the immune system and regulates it. Several pathways allow the CNS to regulate the transcription of immune response genes in peripheral tissues (Figure 1): (i) the hypothalamic–pituitary–adrenal (HPA) axis and the production of glucocorticoids, which suppress pro-inflammatory immune response genes (Herman et al., 2012); (ii) the sympathetic nervous system (SNS), which innervates primary and secondary lymphoid organs, the vasculature and peripheral organs and tissues, acting through norepinephrine to modify hematopoiesis and the interactions between antigen-presenting cells and lymphocytes (Nance and Sanders, 2007); (iii) SNS-induced epinephrine release by the adrenal gland, which upregulates the transcription of pro-inflammatory cytokines (Cole et al., 2007); (iv) the activation of the cholinergic anti-inflammatory system, whereby parasympathetic (vagal) outflow arrives at the celiac ganglion, and then releases acetylcholine (ACh) through cholinergic fibers from the splenic nerve, attenuating spleen cytokine production (Borovikova et al., 2000; Tracey, 2002; Rosas-Ballina and Tracey, 2009); (v) the local (peripheral tissues) release of physiological neuromodulators such as pain-related neuropeptides and enteric system-regulating neuropeptides, and circulating mediators, such as endogenous opioids, insulin-like growth factor, growth hormone, and other hormones (e.g., prolactin, Freeman et al., 2000) that can affect the innate and adaptive immune system (Irwin and Cole, 2011).
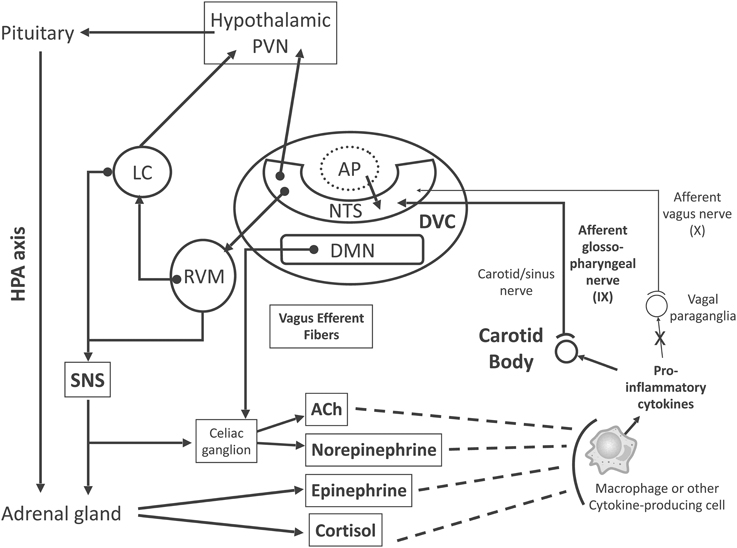
Figure 1. Proposed model for inflammation-induced central nervous system (CNS) activation through carotid body (CB) chemoreceptors and subsequent efferent neuroimmunomodulator activation. Briefly, chemosensory transduction begins in immune cells, which release inflammatory mediators (i.e., TNF-α, IL-1β, and IL-6) to activate the CB. The CB is innervated by glossopharyngeal (cranial nerve IX) afferent neurons, the cell bodies of which are located in the petrosal ganglion, and their central projections end primarily within the dorsal vagal complex (DVC) of the medulla oblongata. The DVC consists of the nucleus tractus solitarii (NTS), the dorsal motor nucleus of vagus nerve (DMN), and the area postrema (AP). The DMN is the main site of origin of preganglionic vagus efferent fibers, which evoke acetylcholine (ACh) release from celiac ganglion neurons, inhibiting the pro-inflammatory response. The AP lacks a brain-blood barrier (dotted line, AP), projects into the NTS and is an important site for humoral immune-to-brain communication. The main portion of the CB chemosensory inputs is received by neurons in the NTS, which coordinate autonomic function and interaction with the endocrine system. Ascending projections from the NTS reach the hypothalamic paraventricular nucleus (PVN), an important structure involved in hypothalamus-pituitary-adrenal (HPA) axis activation, releasing cortisol, which, in fact, inhibits the pro-inflammatory response. Synaptic contacts also exist between neurons in the NTS and rostral ventrolateral medulla (RVM), which plays an important role in the control of cardiovascular and respiratory homeostasis. Neurons from the RVM project to the locus coeruleus (LC), which innervates higher brain sites, such as the PVN. Neuronal projections emanate from the RVM and LC to sympathetic preganglionic neurons (SNS) in the spinal cord, which innervates both the adrenal medulla and celiac ganglion for epinephrine and norepinephrine release, respectively, inhibiting the pro-inflammatory response. These ascending and descending connections provide a neuronal substrate for neuroimmunomodulatory mechanisms. Excitatory pathways, continuous lines; inhibitory pathways, dashed lines.
It is clear that in additionto neural reflexes maintaining homeostasis in other body systems, neural circuits also regulate immunity. Therefore, what is(are) the afferent pathway(s) by which the immune system relay signals to the central nervous system?
Systemic Inflammation and Sepsis
Although inflammation is mostly a beneficial host response to foreign challenge or tissue injury, leading to the restoration of tissue structure and function, it can contribute to the pathogenesis of many diseases. Systemic inflammation results from the dysregulation of local inflammation when the host is unable to contain an insult, regardless of whether it is caused by bacteria, trauma, burn, or drug overdose. Sepsis is defined as “the systemic inflammatory response syndrome that occurs during infection” (Bone et al., 1992) and is mainly due to host cell stimulation (monocytes/macrophages, endothelial, and polymorphonuclear cells) to produce and release pro-inflammatory cytokines (Schletter et al., 1995). Sepsis involves the evidence of infection and may be associated with fever, tachycardia, tachypnea, altered white blood cell count, and decreased arterial oxygen partial pressure. Sepsis progression to severe sepsis and septic shock involves many pathological processes including hemodynamic abnormalities (e.g., hypoperfusion or hypotension) and also involves oliguria, lactic acidosis, acute alteration in mental state and multiple organ dysfunction (MOD) syndrome (Riedemann et al., 2003).
However, immunological mechanisms do not completely explain the basis of cellular dysfunction and MOD. Indeed, systemic inflammation affects several systems within the body, including metabolic, hormonal, and neural pathways (Singer et al., 2004; Carre and Singer, 2008). Thus, systemic inflammation initiates the disruption of communication between different organ systems, and subsequently, MOD reflects a progressive uncoupling that may become permanent. With an increasing projected incidence of 1.5% per annum in the United States, and an average cost per case of US$22,100 (Angus et al., 2001), sepsis syndromes and MOD are the main cause of death of critical care patients because despite many efforts and significant advances in maintaining therapies (Martin et al., 2003), there is no particularly effective therapy for these conditions (Riedemann et al., 2003). Thus, the knowledge of immunometabolic and neurophysiological mechanisms and the pathophysiology underlying sepsis progression to MOD and death could help to improve current therapies and identify new pharmacological therapeutic targets.
The pro-inflammatory cytokine TNF-α is an important mediator of the lethal effect of endotoxin (Tracey et al., 1986). In fact, reducing the activity or the expression of TNF-α significantly diminishes endotoxin-induced damage, and the degree of tissue damage can be correlated to the amount of TNF-α in serum (Yang et al., 2007). Damage may result in microvascular dysregulation and/or mitochondrial dysfunction (Crouser, 2004), which results in MOD and death. TNF-α is released during the first 30–90 min after exposure to LPS, triggering a second level of inflammatory cascades that involve other cytokines, reactive oxygen species, lipid mediators, and the up-regulation of cell adhesion molecules. Normally, the pro-inflammatory response is counter-balanced by a group of regulatory molecules, such as IL-10 (an anti-inflammatory cytokine), which attempt to restore immunological equilibrium (Scumpia and Moldawer, 2005). In fact, the main stimulus for IL-10 production is inflammation itself. Both TNF-α and IL-1β directly stimulate IL-10 production, suggesting the existence of a negative feedback loop, whereby the production of IL-10 is limited to the inflammatory process (Van Der Poll et al., 1994). Therefore, host damage can result directly by excessive inflammation, or indirectly through immune dysfunction, and host survival depends on the intensity of and the correct balance between pro- and anti-inflammatory responses.
Reflex Regulation of Systemic Inflammation: Immune-to-Brain Communication
Research into immunosensory activity has been focused on the origin of signaling, i.e., plasma pro-inflammatory cytokines such as TNF-α, IL-1β, and IL-6. In fact, direct injection of these cytokines into the brain causes fever, activation of the HPA axis and sickness-like symptoms, mimicking a real immune challenge (Quan, 2014). Immune system-derived signals are conveyed to the CNS through four different pathways. The circumventricular organs (CVOs) were among the first immune-to-brain pathways proposed (Blatteis et al., 1987; Stitt, 1990). These regions have a leaky brain-blood barrier (BBB), and several are situated near the CNS areas that are known to react against peripheral immune challenges, such as the area postrema (AP) and the organum vasculosum. The former contains neurons that project into the nucleus tractus solitarii (NTS) (Cai et al., 1996), a well-known target of neuroimmune activation, and the latter is involved in febrile responses. Another (second) afferent pathway occurs via the saturable transport of cytokines across the BBB (Banks and Erickson, 2010), contributing to an increase in neuroinflammation. A less direct pathway (third) is the binding of cytokines to brain endothelial cells, which evokes the release of paracrine mediators such as IL-1, IL6, and prostaglandins (Fabry et al., 1993; Cao et al., 1998; Quan, 2014). Finally, the fourth pathway occurs through the activation of peripheral sensory nerves, i.e., the vagus nerve (Goehler et al., 1997).
Wan et al. showed that subdiaphragmatic vagotomy blocks brain c-fos induction after the intraperitoneal (IP) administration of LPS (Wan et al., 1994), suggesting that neural, rather than humoral, pathways are capable of transmitting inflammatory signals to the brain. However, the involvement of peripheral sensory nerves in immunomodulation is controversial. Inflammatory mediators released by immune cells are able to activate both vagal paraganglia (Goehler et al., 1997, 1999) and primary afferent neurons located in sensory ganglia, which, in turn, evokes host defense reflexes. Vagal paraganglia consist of two cell types: type I (glomus) cells and type II (sustentacular) cells (Berthoud et al., 1995). The cell bodies of vagal afferent neurons innervating vagal glomus cells (GC) are located in the nodose ganglion, and the central projections of these neurons arrive primarily at the dorsal vagal complex (DVC) of the medulla oblongata.
The DVC, which involves the AP, the dorsal motor nucleus of the vagus (DMN) and the NTS (Berthoud and Neuhuber, 2000), is the main site of origin of preganglionic vagus efferent fibers, whereas cardiovascular vagal efferents originate within the medullar nucleus ambiguus (NA). As previously mentioned, the AP is an important CVO and a putative site for humoral immune-to-brain communication. Neurons in the NTS receive the main portion of vagal sensory inputs. In turn, the NTS coordinates autonomic function and interaction with the endocrine system, and its ascending projections reach the hypothalamic paraventricular nucleus (PVN), an important structure involved in the HPA axis activation. Similarly, the NTS projects to the rostral ventrolateral medulla (RVM), which has an important function in the control of cardiovascular and respiratory homeostasis. Neurons from the RVM project to the locus coeruleus (LC), which innervates higher brain sites, such as the hypothalamus and PVN. Neuronal projections from the RVM and LC reach sympathetic preganglionic neurons in the spinal cord (Fernandez and Acuna-Castillo, 2012). Conversely, the PVN projects back to the RVM and NTS (Pavlov et al., 2003), completing the necessary connections for interaction between the HPA axis and autonomic (sympathetic and parasympathetic) cardiorespiratory reflex activation. Furthermore, these interactions provide a neuronal substrate for an immunomodulatory mechanism (Figure 1).
Immunosensory Signaling through the Vagus Nerve
As previously mentioned, subdiaphragmatic vagotomy blocks brain c-fos induction after IP administration of LPS (Wan et al., 1994). Moreover, if the procedure is performed prior to the IP injection of IL-1β, the cytokine fails to evoke a hyperthermic response (Watkins et al., 1995). Thus, abdominal LPS or IL-1β activates sensory neurons in the vagus nerve, which, in turn, activates hyperthermia-related brainstem nuclei (Quan, 2014). In contrast, food-motivated behavior is suppressed by peripheral LPS or IL-1β; this depression is also blocked by vagotomy (Bret-Dibat et al., 1995).
Vagal primary afferent fibers are stimulated by IP administration of LPS (Goehler et al., 1999, 2000), and these fibers activate the CNS neurons responsible for the manifestations of systemic inflammatory response syndrome (Mascarucci et al., 1998; Borsody and Weiss, 2005). Bilateral subdiaphragmatic vagotomy prevents sickness manifestations and activation of the NTS, hypothalamus and LC in rats treated IP with LPS or IL-1β (Bluthe et al., 1994; Bret-Dibat et al., 1995; Gaykema et al., 1995; Hansen and Krueger, 1997; Borsody and Weiss, 2005).
TNF-α-induced vagal immunosensory activity increases (Emch et al., 2000) or decreases (Emch et al., 2002) vagal motor activity. The fever and hyperalgesia caused by IP LPS are suppressed by the abdominal transection of vagal trunks, though this has a little effect on the febrile response to intravenous (IV) or intramuscular LPS. As subdiaphragmatic vagotomy blocks the induction of c-Fos protein in the rat hypothalamus and brainstem following IP injection of LPS, visceral afferent innervations are involved in the response to LPS. Conversely, vagotomy has a minimal effect on c-Fos protein induced by the IV administration of LPS (Wan et al., 1994). Moreover, LPS induces c-Fos activation of NTS neurons, which persists after cervical bilateral vagotomy (Hermann et al., 2001). Nonetheless, these blockages of CNS c-Fos activation are controversial. Neurons from the abdominal region or vagus efferent fibers (perhaps those within celiac branches, which transport LPS from the peritoneal cavity to the blood) may mediate the response to LPS per se. Therefore, vagotomy may eliminate responsive neurons, or it may restrict the amount of LPS escaping into systemic circulation, diminishing the systemic responses to LPS (e.g., c-Fos protein induction in the CNS) (Lenczowski et al., 1997; Romanovsky et al., 2000).
Finally, IL-1β and TNF-α did not modify the frequency of discharge recorded from single fibers in a preparation of isolated superfused rat GC from vagal paraganglia (Mac Grory et al., 2010), despite the expression of cytokine receptor in vagal afferent fibers (Goehler et al., 1997). Furthermore, neither the basal nor hypoxia-induced discharge rate of vagal paraganglia are modulated by IL-1β, TNF-α or LPS, suggesting that these structures are not the afferent limb of an “immune reflex” (O'Connor et al., 2012) (Figure 1). Thus, the neural signals from immune chemosensory inputs should originate from other receptors, and the neural pathway of peripheral arterial chemoreceptors (i.e., the carotid body and its sensory ganglion) provides an interesting candidate.
The Stimulation of Efferent Vagus Nerve in Sepsis Therapy
The role of the vagus nerve and its stimulation has been studied in systemic inflammation. Vagus nerve stimulation has an anti-inflammatory effect in endotoxemia and downregulates proinflammatory cytokine production in sepsis, decreasing the plasma levels of HMGB1 and improving survival in cecal ligation and puncture (CLP), a model of septic peritonitis. Unilateral cervical (Van Westerloo et al., 2005) or subdiaphragmatic vagotomy (Kessler et al., 2006) increases the plasma levels of pro-inflammatory cytokines, tissue damage and mortality in sepsis. Additionally, in septic rats, vagus nerve electrical stimulation attenuates and prevents hypotension (Song et al., 2008) and modulates coagulation activation and fibrinolysis (Van Westerloo et al., 2006), decreasing MOD. The therapeutic potential of vagal (cholinergic) efferent fibers to treat disorders characterized by cytokine dysregulation is reviewed elsewhere (Rosas-Ballina and Tracey, 2009; Tracey, 2009).
Immunosensory Signaling through Carotid Body Chemoreceptors
Anatomically, the carotid body (CB) is the largest paraganglion in the body (Mascorro and Yates, 1980), and its sensory innervation occurs through the carotid/sinus nerve, the nerve endings of which establish abundant synapses with specialized GC (Verna, 1997). The cell bodies of sensory pseudo-monopolar neurons innervating the CB are mainly located in the petrosal ganglion (Kalia and Davies, 1978; Berger, 1980). Afferent carotid/sinus nerve fibers establish the first synapsis in the NTS, at the CNS, in the same way as vagal afferents (Figure 1) (Donoghue et al., 1984; Finley and Katz, 1992).
Due the rich vascularization and abundant chemosensory innervations, we recently proposed that the CB is a peripheral sensor for the presence of immunogenic agents in the blood. Although the canonical LPS receptor, Toll-like receptor (TLR)-4 (Fernandez et al., 2011), and TNF-α receptors are functional (Fernandez et al., 2008, 2011), TNF-α does not modify the chemosensory discharge recorded under normoxic conditions from the carotid nerves of in vitro perfused and superfused cat CB. Nevertheless, TNF-α reduces in a dose-dependent manner the hypoxia-induced enhanced frequency of chemosensory discharge (Fernandez et al., 2008) but enhances the [Ca2+]i response to acute hypoxia of dissociated GC. The increase is significantly larger in cells from the CB of rats exposed to chronic hypoxia or chronic intermittent hypoxia (Lam et al., 2008, 2012). Finally, TNF-α receptor expression in human and mouse carotid bodies was observed using microarray analysis, though the technique did not detect TNF-α (Mkrtchian et al., 2012).
Glomus (type I) cells from rat CB express both IL-1 receptor type I (Wang et al., 2002) and IL-6 receptor α (Wang et al., 2006). In vitro-cultured GC respond to IL-1β with depolarization and a transient rise in [Ca2+]i. Furthermore, IL-1β significantly increases carotid/sinus nerve chemosensory discharge in anesthetized rats (Shu et al., 2007), though the extracellular administration of IL-6 induces a rise in [Ca2+]i and catecholamine release from in vitro-cultured GC (Fan et al., 2009). In addition, the IP administration of IL-1β in rat GC up-regulates both IL-1 receptor type I and tyrosine hydroxylase (Zhang et al., 2007).
We have reported a significant and maintained increase in basal chemosensory discharge after IV infusion of LPS in cats (Fernandez et al., 2008). Additionally, LPS increases CB TNF-α expression in rats (Fernandez et al., 2011), though we have not assessed in situ TNF-α administration. Neither IL-1β nor IL-6 expression in the CB during sepsis has been reported, but systemic pro-inflammatory cytokines could reach the CB because of their extensive vascularization (Verna, 1979). Thus, increased basal CB chemosensory activity could be due to either IL-1β or IL-6 stimulation. IL-1β appears to mimic the responses of the CB to hypoxia (i.e., evokes GC [Ca2+]i oscillations and induces the expression of hypoxia-inducible factor (HIF), a transcription factor essential for the maintenance of normal CB activity during hypoxia) and may, therefore, act in an autocrine manner to enhance the peripheral chemoreceptor drive during systemic inflammation.
In septic cats, CB sensitivity to both stimulant (hypoxia and nicotine) and depressant (hyperoxia) stimuli is decreased (Fernandez et al., 2008). In vitro experiments have shown that TNF-α reduces the hypoxia-induced enhanced frequency of chemosensory discharge in a dose-dependent manner. Thus, TNF-α modulates CB chemosensory activity, perhaps by inducing the GC to release an inhibitory transmitter, such as dopamine. This fact has not yet been tested.
Microarray analyses of human and mouse CBs have shown increased expression of many other genes involved in immune and inflammatory responses. In addition to the above-mentioned pro-inflammatory cytokines, the transcripts of nuclear factor (NF)-κB, IL-10R (but not IL-10), and HMGB-1 have also been found in human and mouse CBs (Mkrtchian et al., 2012). In addition, the IP administration of LPS in rats decreased the cytosolic fraction of IκBα in the CB, evoking subsequent NF-κB p65 translocation into the GC nucleus, which resulted in gene expression, i.e., TNF-α up-regulation (Fernandez et al., 2011).
The expression of pro-inflammatory cytokines and their receptors in the CB suggests that those cytokines may activate chemosensory neurons, even in the absence of sepsis syndrome, exerting a tonic control of cardiorespiratory, endocrine, autonomic, and/or immune functions. Consequently, pro-inflammatory cytokines, through GC membrane receptors, may modify chemosensory activity reaching the NTS, modulating specific components of the systemic inflammatory response (Figure 1).
Pentobarbitone-anesthetized cats treated IV with LPS showed tachypnea, tachycardia, and hypotension, symptoms comparable to patients with severe sepsis and septic shock. Of note, bilateral section of the carotid and aortic nerves prevented increased respiratory rates (Fernandez et al., 2008). Additionally, LPS enhances tonic CB chemosensory activity (i.e., the frequency of chemosensory discharges), whereas LPS reduces CB responsiveness to both transient excitatory (hypoxia and nicotine) and depressant (FiO2 = 100%) stimuli (Fernandez et al., 2008). The reduced ventilatory responses to moderate and severe hypoxia observed in cats are similar to those observed in rats and in unanesthetized newborn piglets subjected to E. coli endotoxin infusion (McDeigan et al., 2003). This reduction in ventilatory responses is mediated –at least in part– by the inhibitory effect of endothelial nitric oxide (NO) on respiratory control mechanisms (Ladino et al., 2007).
Hyperoxia, which, in fact, reduces CB chemosensory activity (Fernandez et al., 2003), is associated with higher plasma levels of IL-6, IL-10 and TNF-α, a greater number of infected biological samples, and mortality in CLP-induced septic rats (Rodriguez-Gonzalez et al., 2014). Consequently, the withdrawal of carotid chemo/baro-sensory function modifies the inflammatory response during sepsis syndromes through a network of neural, humoral and cytokine elements.
The activation of DVC neurons did not require intact vagal pathways, suggesting that peripherally generated TNF-α could act either directly on these neurons –because DVC displays the attributes of CVOs– (Hermann et al., 2001) or, more likely, through another neural afferent pathway. Bilateral vagotomy does affect c-Fos expression in the NTS (Hermann et al., 2001). However, we found that bilateral carotid/sinus neurotomy after IP administration of LPS suppresses both the LPS-induced increase in the number of c-Fos-positive neurons of the NTS –with no significant changes in AP c-Fos immunoreactivity– and the increased levels of plasma cortisol (Reyes et al., 2012). Accordingly, we suggest that the neural signals provided by peripheral receptors that are distinct from vagal paraganglia –such as arterial carotid chemoreceptors, the function of which is intact after bilateral cervical vagotomy– produce prominent CNS manifestations of endotoxemia. These findings are particularly interesting because the CB induces –at least in part– an endocrine response to LPS by acting as an intermediate in the activation of the NTS by pro-inflammatory cytokines.
Carotid Body Stimulation as a Target for Sepsis Therapy: Sympathetic Activation and Glucocorticoids Release
The analysis of heart rate variability (HRV) gives a clear idea about the autonomic (sympathetic/parasympathetic) regulation of cardiorespiratory function. Decreased HRV is consistent with the pathogenesis of MOD; in fact, endotoxemic patients show decreased HRV (Godin et al., 1996; Rassias et al., 2005). Moreover, septic patients have an impaired sympatho-vagal balance that is characterized by a sustained sympatho-excitation accompanying hypotension (Barnaby et al., 2002), and chemo- and baro-denervation accelerates the drop in blood pressure (Vayssettes-Courchay et al., 2005). Finally, decreased parasympathetic activity is an excellent predictor of risk of death in patients with sepsis (Chen et al., 2008). Altogether, these data suggest that reflex arcs involved in maintaining the autonomic balance are altered during sepsis.
Carotid body stimulation provokes a wide array of cardiopulmonary and autonomic reflexes as well as endocrine responses (e.g., plasma release of catecholamines and cortisol) (Fitzgerald, 2014). In particular, chemoreflexes are important modulators of sympathetic activation (Abboud and Thames, 1983), and peripheral chemoreceptor activation elicits respiratory and cardiovascular effects and a sympatho-excitatory response (Alanis et al., 1968; Montarolo et al., 1976). Thus, tonic activation of carotid chemoreceptors during sepsis may also contribute to high levels of sympathetic activity (Kara et al., 2003). However, the administration of 100% O2 decreases the heart rate, blood pressure and central sympathetic outflow (Kara et al., 2003), and hyperoxia-induced CB chemosensory activity withdrawal is associated with higher plasma levels of pro-inflammatory cytokines and mortality in septic rats (Rodriguez-Gonzalez et al., 2014).
In addition, in anesthetized, paralyzed, ventilated and maintained normocapnic mongrel dogs, hypoxic hypoxia (the natural stimulus of CB chemoreceptors) increases the adrenal cortisol secretion rate, and surgical CB and/or aortic body deafferentation attenuates cortisol response (Raff et al., 1982). Thus, the CB exerts the main chemoreceptor influence on cortisol secretion during hypoxia. Interestingly, as mentioned above, bilateral carotid/sinus neurotomy attenuated the LPS-induced cortisol response in septic rats (Reyes et al., 2012).
Consequently, as a therapeutic target, the electrical stimulation of CB chemoreceptors could modify the inflammatory response during sepsis syndromes through a network consisting of neural (sympathetic activation), humoral (glucocorticoid secretion) and, as a consequence, cytokine elements.
Conclusions
The knowledge of immunometabolic and neurophysiological mechanisms and the pathophysiology of sepsis progression to produce organ dysfunction and death have helped in the improvement of current therapies and in identifying new pharmacological therapeutic targets.
Traditionally, the autonomic nervous system coordinates the fine-tuning of the cardiorespiratory relationship, maintaining appropriate metabolite and oxygen delivery to tissues. Several reflex arcs, such as arterial baroreflexes, central chemoreflexes, peripheral arterial chemoreflexes, and pulmonary stretch reflexes, maintain the autonomic (sympathetic-parasympathetic) equilibrium. Consequently, the interactions among those reflexes are clinically interesting because the pathophysiological over-reaction of a single reflex, which occurs in several disorders, may cause the suppression of the opposite reflex responses.
An increasing body of evidence obtained by us and other researchers shows that CB reflexes not only serves as a chemoreceptor for respiratory reflex responses but also as a sensor for immune status (Zapata et al., 2011) and as a modulator of autonomic balance, tending to coordinate the cardiorespiratory interplay devoted to maintaining oxygen homeostasis in different pathologies.
In summary, CB stimulation increases sympathetic activity and glucocorticoid release. Thus, increased basal CB chemosensory activity during sepsis could be responsible, at least in part, for the observed increase in plasma epinephrine and cortisol levels in septic patients. The resulting increased plasma anti-inflammatory mediators could modulate pro-inflammatory cytokine expression in cytokine-producing cells, thereby modifying systemic inflammation and sepsis resolution. The electrical stimulation of the carotid/sinus nerve is a potential therapeutic approach, though not yet assessed, for sepsis therapy.
Conflict of Interest Statement
The authors declare that the research was conducted in the absence of any commercial or financial relationships that could be construed as a potential conflict of interest.
Acknowledgments
This work was supported by research grants from Fondo Nacional de Desarrollo Científico y Tecnológico—FONDECYT 1120976 (Ricardo Fernandez), 1110734 (Claudio Acuña-Castillo), and 3140414 (Gino Nardocci); and UNAB DI-354-13/R (Ricardo Fernandez). The funders had no role in the study design, data collection and analysis, decision to publish, or preparation of the manuscript.
References
Abboud, F. M., and Thames, M. D. (1983). “Interaction of cardiovascular reflexes in circulatory control,” in Handbook of Physiology, Section 2, The Cardiovascular System, Vol. III. Peripheral Circulation and Organ Blood Flow, eds J. T. Sheperd and F. M. Abboud (Bethesda, MD: American Physiological Society), 675–753.
Alanis, J., Defillo, B., and Gordon, S. (1968). Changes in the efferent discharges of sympathetic and parasympathetic cardiac nerves provoked by activation of carotid chemoreceptors. Arch. Int. Physiol. Biochim. 76, 214–235. doi: 10.3109/13813456809058701
Pubmed Abstract | Pubmed Full Text | CrossRef Full Text | Google Scholar
Alvarez Perez Gil, A. L., Barbosa Navarro, L., Patipo Vera, M., and Petricevich, V. L. (2012). Anti-inflammatory and antinociceptive activities of the ethanolic extract of Bougainvillea xbuttiana. J. Ethnopharmacol. 144, 712–719. doi: 10.1016/j.jep.2012.10.018
Pubmed Abstract | Pubmed Full Text | CrossRef Full Text | Google Scholar
Andersson, U., and Tracey, K. J. (2012). Reflex principles of immunological homeostasis. Annu. Rev. Immunol. 30, 313–335. doi: 10.1146/annurev-immunol-020711-075015
Pubmed Abstract | Pubmed Full Text | CrossRef Full Text | Google Scholar
Angus, D. C., Linde-Zwirble, W. T., Lidicker, J., Clermont, G., Carcillo, J., and Pinsky, M. R. (2001). Epidemiology of severe sepsis in the United States: analysis of incidence, outcome, and associated costs of care. Crit. Care Med. 29, 1303–1310. doi: 10.1097/00003246-200107000-00002
Pubmed Abstract | Pubmed Full Text | CrossRef Full Text | Google Scholar
Banks, W. A., and Erickson, M. A. (2010). The blood-brain barrier and immune function and dysfunction. Neurobiol. Dis. 37, 26–32. doi: 10.1016/j.nbd.2009.07.031
Pubmed Abstract | Pubmed Full Text | CrossRef Full Text | Google Scholar
Barnaby, D., Ferrick, K., Kaplan, D. T., Shah, S., Bijur, P., and Gallagher, E. J. (2002). Heart rate variability in emergency department patients with sepsis. Acad. Emerg. Med. 9, 661–670. doi: 10.1111/j.1553-2712.2002.tb02143.x
Pubmed Abstract | Pubmed Full Text | CrossRef Full Text | Google Scholar
Berger, A. J. (1980). The distribution of the cat's carotid sinus nerve afferent and efferent cell bodies using the horseradish peroxidase technique. Brain Res. 190, 309–320. doi: 10.1016/0006-8993(80)90276-0
Pubmed Abstract | Pubmed Full Text | CrossRef Full Text | Google Scholar
Berthoud, H. R., Kressel, M., and Neuhuber, W. L. (1995). Vagal afferent innervation of rat abdominal paraganglia as revealed by anterograde DiI-tracing and confocal microscopy. Acta Anat. (Basel) 152, 127–132. doi: 10.1159/000147691
Pubmed Abstract | Pubmed Full Text | CrossRef Full Text | Google Scholar
Berthoud, H. R., and Neuhuber, W. L. (2000). Functional and chemical anatomy of the afferent vagal system. Auton. Neurosci. 85, 1–17. doi: 10.1016/S1566-0702(00)00215-0
Pubmed Abstract | Pubmed Full Text | CrossRef Full Text | Google Scholar
Blatteis, C. M., Hales, J. R., McKinley, M. J., and Fawcett, A. A. (1987). Role of the anteroventral third ventricle region in fever in sheep. Can. J. Physiol. Pharmacol. 65, 1255–1260. doi: 10.1139/y87-200
Pubmed Abstract | Pubmed Full Text | CrossRef Full Text | Google Scholar
Bluthe, R. M., Walter, V., Parnet, P., Laye, S., Lestage, J., Verrier, D., et al. (1994). Lipopolysaccharide induces sickness behaviour in rats by a vagal mediated mechanism. C. R. Acad. Sci. III 317, 499–503.
Bone, R. C., Balk, R. A., Cerra, F. B., Dellinger, R. P., Fein, A. M., Knaus, W. A., et al. (1992). Definitions for sepsis and organ failure and guidelines for the use of innovative therapies in sepsis. The ACCP/SCCM consensus conference committee. American college of chest physicians/society of critical care medicine. Chest 101, 1644–1655. doi: 10.1378/chest.101.6.1644
Pubmed Abstract | Pubmed Full Text | CrossRef Full Text | Google Scholar
Borovikova, L. V., Ivanova, S., Zhang, M., Yang, H., Botchkina, G. I., Watkins, L. R., et al. (2000). Vagus nerve stimulation attenuates the systemic inflammatory response to endotoxin. Nature 405, 458–462. doi: 10.1038/35013070
Pubmed Abstract | Pubmed Full Text | CrossRef Full Text | Google Scholar
Borsody, M. K., and Weiss, J. M. (2005). The subdiaphragmatic vagus nerves mediate activation of locus coeruleus neurons by peripherally administered microbial substances. Neuroscience 131, 235–245. doi: 10.1016/j.neuroscience.2004.09.061
Pubmed Abstract | Pubmed Full Text | CrossRef Full Text | Google Scholar
Bret-Dibat, J. L., Bluthe, R. M., Kent, S., Kelley, K. W., and Dantzer, R. (1995). Lipopolysaccharide and interleukin-1 depress food-motivated behavior in mice by a vagal-mediated mechanism. Brain Behav. Immun. 9, 242–246. doi: 10.1006/brbi.1995.1023
Pubmed Abstract | Pubmed Full Text | CrossRef Full Text | Google Scholar
Buras, J. A., Holzmann, B., and Sitkovsky, M. (2005). Animal models of sepsis: setting the stage. Nat. Rev. Drug Discov. 4, 854–865. doi: 10.1038/nrd1854
Pubmed Abstract | Pubmed Full Text | CrossRef Full Text | Google Scholar
Cai, Y., Hay, M., and Bishop, V. S. (1996). Synaptic connections and interactions between area postrema and nucleus tractus solitarius. Brain Res. 724, 121–124. doi: 10.1016/0006-8993(96)00282-X
Pubmed Abstract | Pubmed Full Text | CrossRef Full Text | Google Scholar
Cao, C., Matsumura, K., Yamagata, K., and Watanabe, Y. (1998). Cyclooxygenase-2 is induced in brain blood vessels during fever evoked by peripheral or central administration of tumor necrosis factor. Brain Res. Mol. Brain Res. 56, 45–56. doi: 10.1016/S0169-328X(98)00025-4
Pubmed Abstract | Pubmed Full Text | CrossRef Full Text | Google Scholar
Carre, J. E., and Singer, M. (2008). Cellular energetic metabolism in sepsis: the need for a systems approach. Biochim. Biophys. Acta 1777, 763–771. doi: 10.1016/j.bbabio.2008.04.024
Pubmed Abstract | Pubmed Full Text | CrossRef Full Text | Google Scholar
Chaplin, D. D. (2010). Overview of the immune response. J. Allergy Clin. Immunol. 125, S3–S23. doi: 10.1016/j.jaci.2009.12.980
Pubmed Abstract | Pubmed Full Text | CrossRef Full Text | Google Scholar
Chen, W. L., Chen, J. H., Huang, C. C., Kuo, C. D., Huang, C. I., and Lee, L. S. (2008). Heart rate variability measures as predictors of in-hospital mortality in ED patients with sepsis. Am. J. Emerg. Med. 26, 395–401. doi: 10.1016/j.ajem.2007.06.016
Pubmed Abstract | Pubmed Full Text | CrossRef Full Text | Google Scholar
Cohen, N., Moynihan, J. A., and Ader, R. (1994). Pavlovian conditioning of the immune system. Int. Arch. Allergy Immunol. 105, 101–106. doi: 10.1159/000236811
Pubmed Abstract | Pubmed Full Text | CrossRef Full Text | Google Scholar
Cole, S. W., Hawkley, L. C., Arevalo, J. M., Sung, C. Y., Rose, R. M., and Cacioppo, J. T. (2007). Social regulation of gene expression in human leukocytes. Genome Biol. 8:R189. doi: 10.1186/gb-2007-8-9-r189
Pubmed Abstract | Pubmed Full Text | CrossRef Full Text | Google Scholar
Crouser, E. D. (2004). Mitochondrial dysfunction in septic shock and multiple organ dysfunction syndrome. Mitochondrion 4, 729–741. doi: 10.1016/j.mito.2004.07.023
Pubmed Abstract | Pubmed Full Text | CrossRef Full Text | Google Scholar
Deutschman, C. S., and Tracey, K. J. (2014). Sepsis: current dogma and new perspectives. Immunity 40, 463–475. doi: 10.1016/j.immuni.2014.04.001
Pubmed Abstract | Pubmed Full Text | CrossRef Full Text | Google Scholar
Donoghue, S., Felder, R. B., Jordan, D., and Spyer, K. M. (1984). The central projections of carotid baroreceptors and chemoreceptors in the cat: a neurophysiological study. J. Physiol. 347, 397–409.
Emch, G. S., Hermann, G. E., and Rogers, R. C. (2000). TNF-alpha activates solitary nucleus neurons responsive to gastric distension. Am. J. Physiol. Gastrointest. Liver Physiol. 279, G582–G586.
Emch, G. S., Hermann, G. E., and Rogers, R. C. (2002). Tumor necrosis factor-alpha inhibits physiologically identified dorsal motor nucleus neurons in vivo. Brain Res. 951, 311–315. doi: 10.1016/S0006-8993(02)03178-5
Pubmed Abstract | Pubmed Full Text | CrossRef Full Text | Google Scholar
Fabry, Z., Fitzsimmons, K. M., Herlein, J. A., Moninger, T. O., Dobbs, M. B., and Hart, M. N. (1993). Production of the cytokines interleukin 1 and 6 by murine brain microvessel endothelium and smooth muscle pericytes. J. Neuroimmunol. 47, 23–34. doi: 10.1016/0165-5728(93)90281-3
Pubmed Abstract | Pubmed Full Text | CrossRef Full Text | Google Scholar
Fan, J., Zhang, B., Shu, H. F., Zhang, X. Y., Wang, X., Kuang, F., et al. (2009). Interleukin-6 increases intracellular Ca2+ concentration and induces catecholamine secretion in rat carotid body glomus cells. J. Neurosci. Res. 87, 2757–2762. doi: 10.1002/jnr.22107
Pubmed Abstract | Pubmed Full Text | CrossRef Full Text | Google Scholar
Fernandez, R., and Acuna-Castillo, C. (2012). “Neural reflex control of inflammation during sepsis syndromes,” in Sepsis—An Ongoing and Significant Challenge, ed L. Azevedo (Rijeka: InTech.), 133–156.
Fernandez, R., Arriagada, I., Garrido, A. M., Larrain, C., and Zapata, P. (2003). Ventilatory chemosensory drive in cats, rats and guinea-pigs. Adv. Exp. Med. Biol. 536, 489–495. doi: 10.1007/978-1-4419-9280-2_62
Pubmed Abstract | Pubmed Full Text | CrossRef Full Text | Google Scholar
Fernandez, R., Gonzalez, S., Rey, S., Cortes, P. P., Maisey, K. R., Reyes, E. P., et al. (2008). Lipopolysaccharide-induced carotid body inflammation in cats: functional manifestations, histopathology and involvement of tumour necrosis factor-alpha. Exp. Physiol. 93, 892–907. doi: 10.1113/expphysiol.2008.041152
Pubmed Abstract | Pubmed Full Text | CrossRef Full Text | Google Scholar
Fernandez, R., Nardocci, G., Simon, F., Martin, A., Becerra, A., Rodriguez-Tirado, C., et al. (2011). Lipopolysaccharide signaling in the carotid chemoreceptor pathway of rats with sepsis syndrome. Respir. Physiol. Neurobiol. 175, 336–348. doi: 10.1016/j.resp.2010.12.014
Pubmed Abstract | Pubmed Full Text | CrossRef Full Text | Google Scholar
Finley, J. C., and Katz, D. M. (1992). The central organization of carotid body afferent projections to the brainstem of the rat. Brain Res. 572, 108–116. doi: 10.1016/0006-8993(92)90458-L
Pubmed Abstract | Pubmed Full Text | CrossRef Full Text | Google Scholar
Fitzgerald, R. S. (2014). Carotid body: a new target for rescuing neural control of cardiorespiratory balance in disease. Front. Physiol. 5:304. doi: 10.3389/fphys.2014.00304
Pubmed Abstract | Pubmed Full Text | CrossRef Full Text | Google Scholar
Freeman, M. E., Kanyicska, B., Lerant, A., and Nagy, G. (2000). Prolactin: structure, function, and regulation of secretion. Physiol. Rev. 80, 1523–1631.
Gaykema, R. P., Dijkstra, I., and Tilders, F. J. (1995). Subdiaphragmatic vagotomy suppresses endotoxin-induced activation of hypothalamic corticotropin-releasing hormone neurons and ACTH secretion. Endocrinology 136, 4717–4720.
Glaser, R., and Kiecolt-Glaser, J. K. (2005). Stress-induced immune dysfunction: implications for health. Nat. Rev. Immunol. 5, 243–251. doi: 10.1038/nri1571
Pubmed Abstract | Pubmed Full Text | CrossRef Full Text | Google Scholar
Godin, P. J., Fleisher, L. A., Eidsath, A., Vandivier, R. W., Preas, H. L., Banks, S. M., et al. (1996). Experimental human endotoxemia increases cardiac regularity: results from a prospective, randomized, crossover trial. Crit. Care Med. 24, 1117–1124. doi: 10.1097/00003246-199607000-00009
Pubmed Abstract | Pubmed Full Text | CrossRef Full Text | Google Scholar
Goehler, L. E., Gaykema, R. P., Hansen, M. K., Anderson, K., Maier, S. F., and Watkins, L. R. (2000). Vagal immune-to-brain communication: a visceral chemosensory pathway. Auton. Neurosci. 85, 49–59. doi: 10.1016/S1566-0702(00)00219-8
Pubmed Abstract | Pubmed Full Text | CrossRef Full Text | Google Scholar
Goehler, L. E., Gaykema, R. P., Nguyen, K. T., Lee, J. E., Tilders, F. J., Maier, S. F., et al. (1999). Interleukin-1beta in immune cells of the abdominal vagus nerve: a link between the immune and nervous systems? J. Neurosci. 19, 2799–2806.
Goehler, L. E., Relton, J. K., Dripps, D., Kiechle, R., Tartaglia, N., Maier, S. F., et al. (1997). Vagal paraganglia bind biotinylated interleukin-1 receptor antagonist: a possible mechanism for immune-to-brain communication. Brain Res. Bull. 43, 357–364. doi: 10.1016/S0361-9230(97)00020-8
Pubmed Abstract | Pubmed Full Text | CrossRef Full Text | Google Scholar
Hansen, M. K., and Krueger, J. M. (1997). Subdiaphragmatic vagotomy blocks the sleep- and fever-promoting effects of interleukin-1beta. Am. J. Physiol. 273, R1246–R1253.
Herman, J. P., McKlveen, J. M., Solomon, M. B., Carvalho-Netto, E., and Myers, B. (2012). Neural regulation of the stress response: glucocorticoid feedback mechanisms. Braz. J. Med. Biol. Res. 45, 292–298. doi: 10.1590/S0100-879X2012007500041
Pubmed Abstract | Pubmed Full Text | CrossRef Full Text | Google Scholar
Hermann, G. E., Emch, G. S., Tovar, C. A., and Rogers, R. C. (2001). c-Fos generation in the dorsal vagal complex after systemic endotoxin is not dependent on the vagus nerve. Am. J. Physiol. Regul. Integr. Comp. Physiol. 280, R289–R299.
Irwin, M. R., and Cole, S. W. (2011). Reciprocal regulation of the neural and innate immune systems. Nat. Rev. Immunol. 11, 625–632. doi: 10.1038/nri3042
Pubmed Abstract | Pubmed Full Text | CrossRef Full Text | Google Scholar
Kalia, M., and Davies, R. O. (1978). A neuroanatomical search for glossopharyngeal efferents to the carotid body using the retrograde transport of horseradish peroxidase. Brain Res. 149, 477–481. doi: 10.1016/0006-8993(78)90489-4
Kara, T., Narkiewicz, K., and Somers, V. K. (2003). Chemoreflexes—physiology and clinical implications. Acta Physiol. Scand. 177, 377–384. doi: 10.1046/j.1365-201X.2003.01083.x
Pubmed Abstract | Pubmed Full Text | CrossRef Full Text | Google Scholar
Kessler, W., Traeger, T., Westerholt, A., Neher, F., Mikulcak, M., Muller, A., et al. (2006). The vagal nerve as a link between the nervous and immune system in the instance of polymicrobial sepsis. Langenbecks Arch. Surg. 391, 83–87. doi: 10.1007/s00423-006-0031-y
Pubmed Abstract | Pubmed Full Text | CrossRef Full Text | Google Scholar
Ladino, J., Bancalari, E., and Suguihara, C. (2007). Ventilatory response to hypoxia during endotoxemia in young rats: role of nitric oxide. Pediatr. Res. 62, 134–138. doi: 10.1203/PDR.0b013e318098721a
Pubmed Abstract | Pubmed Full Text | CrossRef Full Text | Google Scholar
Lam, S. Y., Liu, Y., Ng, K. M., Lau, C. F., Liong, E. C., Tipoe, G. L., et al. (2012). Chronic intermittent hypoxia induces local inflammation of the rat carotid body via functional upregulation of proinflammatory cytokine pathways. Histochem. Cell Biol. 137, 303–317. doi: 10.1007/s00418-011-0900-5
Pubmed Abstract | Pubmed Full Text | CrossRef Full Text | Google Scholar
Lam, S. Y., Tipoe, G. L., Liong, E. C., and Fung, M. L. (2008). Chronic hypoxia upregulates the expression and function of proinflammatory cytokines in the rat carotid body. Histochem. Cell Biol. 130, 549–559. doi: 10.1007/s00418-008-0437-4
Pubmed Abstract | Pubmed Full Text | CrossRef Full Text | Google Scholar
Lamkanfi, M., Sarkar, A., Van de Walle, L., Vitari, A. C., Amer, A. O., Wewers, M. D., et al. (2010). Inflammasome-dependent release of the alarmin HMGB1 in endotoxemia. J. Immunol. 185, 4385–4392. doi: 10.4049/jimmunol.1000803
Pubmed Abstract | Pubmed Full Text | CrossRef Full Text | Google Scholar
Lenczowski, M. J., Van Dam, A. M., Poole, S., Larrick, J. W., and Tilders, F. J. (1997). Role of circulating endotoxin and interleukin-6 in the ACTH and corticosterone response to intraperitoneal LPS. Am. J. Physiol. 273, R1870–R1877.
Mac Grory, B., O'Connor, E. T., O'Halloran, K. D., and Jones, J. F. (2010). The effect of pro-inflammatory cytokines on the discharge rate of vagal nerve paraganglia in the rat. Respir. Physiol. Neurobiol. 171, 122–127. doi: 10.1016/j.resp.2010.03.001
Pubmed Abstract | Pubmed Full Text | CrossRef Full Text | Google Scholar
Majno, G., and Joris, I. (1996). “Introduction to inflammation,” in Cells, Tissues, and Diseases: Principles of General Pathology, eds G. Majno and I. Joris (Cambridge: Blackwell), 291–317.
Martin, G. S., Mannino, D. M., Eaton, S., and Moss, M. (2003). The epidemiology of sepsis in the United States from 1979 through 2000. N. Engl. J. Med. 348, 1546–1554. doi: 10.1056/NEJMoa022139
Pubmed Abstract | Pubmed Full Text | CrossRef Full Text | Google Scholar
Mascarucci, P., Perego, C., Terrazzino, S., and De Simoni, M. G. (1998). Glutamate release in the nucleus tractus solitarius induced by peripheral lipopolysaccharide and interleukin-1 beta. Neuroscience 86, 1285–1290. doi: 10.1016/S0306-4522(98)00105-5
Pubmed Abstract | Pubmed Full Text | CrossRef Full Text | Google Scholar
Mascorro, J. A., and Yates, R. D. (1980). Paraneurons and paraganglia: histological and ultrastructural comparisons between intraganglionic paraneurons and extra-adrenal paraganglion cells. Adv. Biochem. Psychopharmacol. 25, 201–213.
McDeigan, G. E., Ladino, J., Hehre, D., Devia, C., Bancalari, E., and Suguihara, C. (2003). The effect of Escherichia coli endotoxin infusion on the ventilatory response to hypoxia in unanesthetized newborn piglets. Pediatr. Res. 53, 950–955. doi: 10.1203/01.PDR.0000064581.94126.1C
Pubmed Abstract | Pubmed Full Text | CrossRef Full Text | Google Scholar
Mkrtchian, S., Kahlin, J., Ebberyd, A., Gonzalez, C., Sanchez, D., Balbir, A., et al. (2012). The human carotid body transcriptome with focus on oxygen sensing and inflammation—a comparative analysis. J. Physiol. 590, 3807–3819. doi: 10.1113/jphysiol.2012.231084
Pubmed Abstract | Pubmed Full Text | CrossRef Full Text | Google Scholar
Montarolo, P. G., Passatore, M., and Raschi, F. (1976). Carotid chemoreceptor influence on the cardiac sympathetic nerve discharge. Experientia 32, 480–481. doi: 10.1007/BF01920808
Pubmed Abstract | Pubmed Full Text | CrossRef Full Text | Google Scholar
Nance, D. M., and Sanders, V. M. (2007). Autonomic innervation and regulation of the immune system (1987-2007). Brain Behav. Immun. 21, 736–745. doi: 10.1016/j.bbi.2007.03.008
Pubmed Abstract | Pubmed Full Text | CrossRef Full Text | Google Scholar
Nathan, C., and Ding, A. (2010). Nonresolving inflammation. Cell 140, 871–882. doi: 10.1016/j.cell.2010.02.029
Pubmed Abstract | Pubmed Full Text | CrossRef Full Text | Google Scholar
O'Connor, E. T., O'Halloran, K. D., and Jones, J. F. (2012). Pro-inflammatory cytokines do not affect basal or hypoxia-stimulated discharge of rat vagal paraganglia. Exp. Physiol. 97, 1203–1210. doi: 10.1113/expphysiol.2012.064907
Pubmed Abstract | Pubmed Full Text | CrossRef Full Text | Google Scholar
Pavlov, V. A., Wang, H., Czura, C. J., Friedman, S. G., and Tracey, K. J. (2003). The cholinergic anti-inflammatory pathway: a missing link in neuroimmunomodulation. Mol. Med. 9, 125–134.
Quan, N. (2014). In-depth conversation: spectrum and kinetics of neuroimmune afferent pathways. Brain Behav. Immun. 40, 1–8. doi: 10.1016/j.bbi.2014.02.006
Pubmed Abstract | Pubmed Full Text | CrossRef Full Text | Google Scholar
Raff, H., Tzankoff, S. P., and Fitzgerald, R. S. (1982). Chemoreceptor involvement in cortisol responses to hypoxia in ventilated dogs. J. Appl. Physiol. Respir. Environ. Exerc. Physiol. 52, 1092–1096.
Rassias, A. J., Holzberger, P. T., Givan, A. L., Fahrner, S. L., and Yeager, M. P. (2005). Decreased physiologic variability as a generalized response to human endotoxemia. Crit. Care Med. 33, 512–519. doi: 10.1097/01.CCM.0000155908.46346.ED
Pubmed Abstract | Pubmed Full Text | CrossRef Full Text | Google Scholar
Reyes, E. P., Abarzua, S., Martin, A., Rodriguez, J., Cortes, P. P., and Fernandez, R. (2012). LPS-induced c-Fos activation in NTS neurons and plasmatic cortisol increases in septic rats are suppressed by bilateral carotid chemodenervation. Adv. Exp. Med. Biol. 758, 185–190. doi: 10.1007/978-94-007-4584-1_26
Pubmed Abstract | Pubmed Full Text | CrossRef Full Text | Google Scholar
Riedemann, N. C., Guo, R. F., and Ward, P. A. (2003). The enigma of sepsis. J. Clin. Invest 112, 460–467. doi: 10.1172/JCI200319523
Pubmed Abstract | Pubmed Full Text | CrossRef Full Text | Google Scholar
Rock, K. L., Latz, E., Ontiveros, F., and Kono, H. (2010). The sterile inflammatory response. Annu. Rev. Immunol. 28, 321–342. doi: 10.1146/annurev-immunol-030409-101311
Pubmed Abstract | Pubmed Full Text | CrossRef Full Text | Google Scholar
Rodríguez-González, R., Martín-Barrasa, J, L., Ramos-Nuez, Á., Cañas-Pedrosa, A. M., Martínez-Saavedra, M. T., García-Bello, M. Á., et al. (2014). Multiple system organ response induced by hyperoxia in a clinically relevant animal model of sepsis. Shock. 42, 148–153. doi: 10.1097/SHK.0000000000000189
Pubmed Abstract | Pubmed Full Text | CrossRef Full Text | Google Scholar
Romanovsky, A. A., Ivanov, A. I., Lenczowski, M. J., Kulchitsky, V. A., Van Dam, A. M., Poole, S., et al. (2000). Lipopolysaccharide transport from the peritoneal cavity to the blood: is it controlled by the vagus nerve? Auton. Neurosci. 85, 133–140. doi: 10.1016/S1566-0702(00)00232-0
Pubmed Abstract | Pubmed Full Text | CrossRef Full Text | Google Scholar
Rosas-Ballina, M., and Tracey, K. J. (2009). Cholinergic control of inflammation. J. Intern. Med. 265, 663–679. doi: 10.1111/j.1365-2796.2009.02098.x
Pubmed Abstract | Pubmed Full Text | CrossRef Full Text | Google Scholar
Schletter, J., Heine, H., Ulmer, A. J., and Rietschel, E. T. (1995). Molecular mechanisms of endotoxin activity. Arch. Microbiol. 164, 383–389. doi: 10.1007/BF02529735
Pubmed Abstract | Pubmed Full Text | CrossRef Full Text | Google Scholar
Scumpia, P. O., and Moldawer, L. L. (2005). Biology of interleukin-10 and its regulatory roles in sepsis syndromes. Crit. Care Med. 33, S468–S471. doi: 10.1097/01.CCM.0000186268.53799.67
Pubmed Abstract | Pubmed Full Text | CrossRef Full Text | Google Scholar
Shu, H. F., Wang, B. R., Wang, S. R., Yao, W., Huang, H. P., Zhou, Z., et al. (2007). IL-1beta inhibits IK and increases [Ca2+]i in the carotid body glomus cells and increases carotid sinus nerve firings in the rat. Eur. J. Neurosci. 25, 3638–3647. doi: 10.1111/j.1460-9568.2007.05586.x
Pubmed Abstract | Pubmed Full Text | CrossRef Full Text | Google Scholar
Singer, M., De, S., V., Vitale, D., and Jeffcoate, W. (2004). Multiorgan failure is an adaptive, endocrine-mediated, metabolic response to overwhelming systemic inflammation. Lancet 364, 545–548. doi: 10.1016/S0140-6736(04)16815-3
Pubmed Abstract | Pubmed Full Text | CrossRef Full Text | Google Scholar
Song, X. M., Li, J. G., Wang, Y. L., Hu, Z. F., Zhou, Q., Du, Z. H., et al. (2008). The protective effect of the cholinergic anti-inflammatory pathway against septic shock in rats. Shock 30, 468–472. doi: 10.1097/SHK.0b013e31816d5e49
Pubmed Abstract | Pubmed Full Text | CrossRef Full Text | Google Scholar
Stitt, J. T. (1990). Passage of immunomodulators across the blood-brain barrier. Yale J. Biol. Med. 63, 121–131.
Tracey, K. J. (2002). The inflammatory reflex. Nature 420, 853–859. doi: 10.1038/nature01321
Pubmed Abstract | Pubmed Full Text | CrossRef Full Text | Google Scholar
Tracey, K. J. (2009). Reflex control of immunity. Nat. Rev. Immunol. 9, 418–428. doi: 10.1038/nri2566
Pubmed Abstract | Pubmed Full Text | CrossRef Full Text | Google Scholar
Tracey, K. J., Beutler, B., Lowry, S. F., Merryweather, J., Wolpe, S., Milsark, I. W., et al. (1986). Shock and tissue injury induced by recombinant human cachectin. Science 234, 470–474. doi: 10.1126/science.3764421
Pubmed Abstract | Pubmed Full Text | CrossRef Full Text | Google Scholar
Van Der Poll, T., Jansen, J., Levi, M., Ten Cate, H., Ten Cate, J. W., and Van Deventer, S. J. (1994). Regulation of interleukin 10 release by tumor necrosis factor in humans and chimpanzees. J. Exp. Med. 180, 1985–1988. doi: 10.1084/jem.180.5.1985
Pubmed Abstract | Pubmed Full Text | CrossRef Full Text | Google Scholar
Van Westerloo, D. J., Giebelen, I. A., Florquin, S., Daalhuisen, J., Bruno, M. J., De Vos, A. F., et al. (2005). The cholinergic anti-inflammatory pathway regulates the host response during septic peritonitis. J. Infect. Dis. 191, 2138–2148. doi: 10.1086/430323
Pubmed Abstract | Pubmed Full Text | CrossRef Full Text | Google Scholar
Van Westerloo, D. J., Giebelen, I. A., Meijers, J. C., Daalhuisen, J., De Vos, A. F., Levi, M., et al. (2006). Vagus nerve stimulation inhibits activation of coagulation and fibrinolysis during endotoxemia in rats. J. Thromb. Haemost. 4, 1997–2002. doi: 10.1111/j.1538-7836.2006.02112.x
Pubmed Abstract | Pubmed Full Text | CrossRef Full Text | Google Scholar
Vayssettes-Courchay, C., Bouysset, F., and Verbeuren, T. J. (2005). Sympathetic activation and tachycardia in lipopolysaccharide treated rats are temporally correlated and unrelated to the baroreflex. Auton. Neurosci. 120, 35–45. doi: 10.1016/j.autneu.2005.03.002
Pubmed Abstract | Pubmed Full Text | CrossRef Full Text | Google Scholar
Verna, A. (1979). Ulstrastructure of the carotid body in the mammals. Int. Rev. Cytol. 60, 271–330. doi: 10.1016/S0074-7696(08)61265-6
Pubmed Abstract | Pubmed Full Text | CrossRef Full Text | Google Scholar
Verna, A. (1997). “The mammalian carotid body: morphological data,” in The Carotid Body Chemoreceptors, ed C. González (New York, NY: Springer), 1–29.
Wan, W., Wetmore, L., Sorensen, C. M., Greenberg, A. H., and Nance, D. M. (1994). Neural and biochemical mediators of endotoxin and stress-induced c-fos expression in the rat brain. Brain Res. Bull. 34, 7–14. doi: 10.1016/0361-9230(94)90179-1
Pubmed Abstract | Pubmed Full Text | CrossRef Full Text | Google Scholar
Wang, X., Wang, B. R., Duan, X. L., Zhang, P., Ding, Y. Q., Jia, Y., et al. (2002). Strong expression of interleukin-1 receptor type I in the rat carotid body. J. Histochem. Cytochem. 50, 1677–1684. doi: 10.1177/002215540205001213
Pubmed Abstract | Pubmed Full Text | CrossRef Full Text | Google Scholar
Wang, X., Zhang, X. J., Xu, Z., Li, X., Li, G. L., Ju, G., et al. (2006). Morphological evidence for existence of IL-6 receptor alpha in the glomus cells of rat carotid body. Anat. Rec. A Discov. Mol. Cell Evol. Biol. 288, 292–296. doi: 10.1002/ar.a.20310
Pubmed Abstract | Pubmed Full Text | CrossRef Full Text | Google Scholar
Watkins, L. R., Goehler, L. E., Relton, J. K., Tartaglia, N., Silbert, L., Martin, D., et al. (1995). Blockade of interleukin-1 induced hyperthermia by subdiaphragmatic vagotomy: evidence for vagal mediation of immune-brain communication. Neurosci. Lett. 183, 27–31. doi: 10.1016/0304-3940(94)11105-R
Pubmed Abstract | Pubmed Full Text | CrossRef Full Text | Google Scholar
Yang, F. L., Li, C. H., Hsu, B. G., Tsai, N. M., Lin, S. Z., Harn, H. J., et al. (2007). The reduction of tumor necrosis factor-alpha release and tissue damage by pentobarbital in the experimental endotoxemia model. Shock 28, 309–316. doi: 10.1097/shk.0b013e31803dd04d
Pubmed Abstract | Pubmed Full Text | CrossRef Full Text | Google Scholar
Zapata, P., Larrain, C., Reyes, P., and Fernandez, R. (2011). Immunosensory signalling by carotid body chemoreceptors. Respir. Physiol. Neurobiol. 178, 370–374. doi: 10.1016/j.resp.2011.03.025
Pubmed Abstract | Pubmed Full Text | CrossRef Full Text | Google Scholar
Zhang, X. J., Wang, X., Xiong, L. Z., Fan, J., Duan, X. L., and Wang, B. R. (2007). Up-regulation of IL-1 receptor type I and tyrosine hydroxylase in the rat carotid body following intraperitoneal injection of IL-1beta. Histochem. Cell Biol. 128, 533–540. doi: 10.1007/s00418-007-0346-y
Pubmed Abstract | Pubmed Full Text | CrossRef Full Text | Google Scholar
Keywords: systemic inflammation, sepsis, reflex control of inflammation, carotid body, vagus nerve
Citation: Fernandez R, Nardocci G, Navarro C, Reyes EP, Acuña-Castillo C and Cortes PP (2014) Neural reflex regulation of systemic inflammation: potential new targets for sepsis therapy. Front. Physiol. 5:489. doi: 10.3389/fphys.2014.00489
Received: 30 September 2014; Accepted: 27 November 2014;
Published online: 15 December 2014.
Edited by:
Rodrigo Del Rio, Universidad Autonoma de Chile, ChileReviewed by:
Silvia V. Conde, Faculdade Ciencias Médicas, PortugalMan Lung Fung, The University of Hong Kong, China
Noah J. Marcus, University of Nebraska Medical Center, USA
Copyright © 2014 Fernandez, Nardocci, Navarro, Reyes, Acuña-Castillo and Cortes. This is an open-access article distributed under the terms of the Creative Commons Attribution License (CC BY). The use, distribution or reproduction in other forums is permitted, provided the original author(s) or licensor are credited and that the original publication in this journal is cited, in accordance with accepted academic practice. No use, distribution or reproduction is permitted which does not comply with these terms.
*Correspondence: Ricardo Fernandez, Laboratorio de Fisiología, Departamento de Ciencias Biológicas, Facultad de Ciencias Biológicas y Facultad de Medicina, Universidad Andrés Bello. Av. República 252, 8370134 Santiago, Chile e-mail:cmZlcm5hbmRlekB1bmFiLmNs