- Department of Physiology, University of Hong Kong, Pokfulam, Hong Kong
The renin-angiotensin system (RAS) plays pivotal roles in the regulation of cardiovascular and renal functions to maintain the fluid and electrolyte homeostasis. Experimental studies have demonstrated a locally expressed RAS in the carotid body, which is functional significant in the effect of angiotensin peptides on the regulation of the activity of peripheral chemoreceptors and the chemoreflex. The physiological and pathophysiological implications of the RAS in the carotid body have been proposed upon recent studies showing a significant upregulation of the RAS expression under hypoxic conditions relevant to altitude acclimation and sleep apnea and also in animal model of heart failure. Specifically, the increased expression of angiotensinogen, angiotensin-converting enzyme and angiotensin AT1 receptors plays significant roles in the augmented carotid chemoreceptor activity and inflammation of the carotid body. This review aims to summarize these results with highlights on the pathophysiological function of the RAS under hypoxic conditions. It is concluded that the maladaptive changes of the RAS in the carotid body plays a pathogenic role in sleep apnea and heart failure, which could potentially be a therapeutic target for the treatment of the pathophysiological consequence of sleep apnea.
Introduction
The renin-angiotensin system (RAS) plays important physiological roles in the humoral regulation of blood pressure, electrolyte and fluid homeostasis (Peach, 1977). The physiological effects are mediated by bioactive angiotensin (Ang) peptides including Ang II, Ang III, Ang IV, and Ang (1-7), produced by renin, angiotensin-converting enzyme (ACE), ACE-2 and angiotensin-processing peptidases, via the angiotensin AT1, AT2, AT4, and Mas receptors (Figure 1). In addition to the endocrine function of RAS, growing amount of evidence demonstrates that the intrinsic RAS functions in an autocrine-paracrine manner, mediated by the RAS components expressed in numerous tissues and organs. The local RAS has diverse effects on, for examples, the regulation of vascular tone, generation of reactive oxygen species (ROS), inflammation or fibrogenesis, which are regulated by various physiological stimuli or pathophysiological conditions (Campbell, 2003). As such, it has been proposed that the local RAS might be a potential therapeutic target for the treatment of disease.
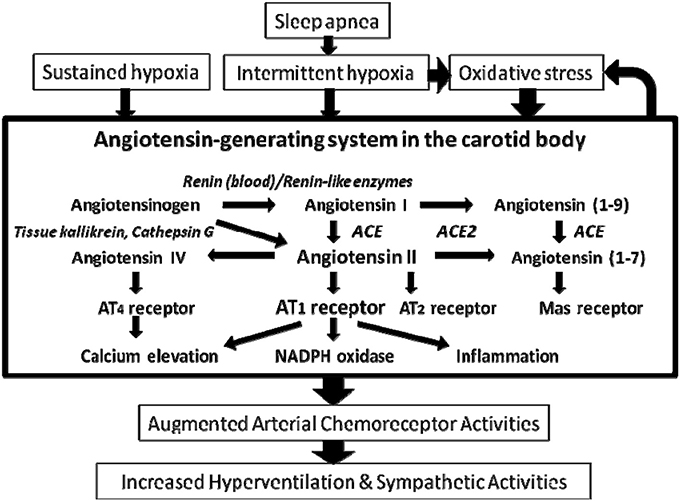
Figure 1. Main components of the renin angiotensin system and the enzymes mediating the proteolytic process in the carotid body. Arrows denote the main direction of the physiological or pathophysiological cascade. Note the renin-independent biosynthetic pathway and the enzymes involved are shown in italic. The major physiological effect of Ang II is mediated by the AT1 and AT2 receptors. In addition, the Ang II metabolites Ang IV and Ang (1-7) exert biological effects via AT4 and Mas receptors, respectively. ACE, angiotensin-converting enzyme.
Arterial chemoreceptors in the carotid body are important for the rapid adjustment of respiratory and cardiovascular activities via the chemoreflex elicited by the sensory afferent activity of the chemoreceptor responding to changes in chemical stimuli in the arterial blood. The carotid body is a highly vascularized organ with blood perfusion far exceeding the needs of its local tissue metabolism. Thus, changes in arterial oxygen tension or pH, circulating humoral and locally produced signaling substances acting as paracrines or autocrines can readily diffuse to the chemosensory components of the carotid body. In addition to the response to hypoxia, hypercapnia and acidosis, the carotid chemoreceptor responds to Ang II because AT receptors are expressed in the chemosensitive glomus cell of the carotid body (Allen, 1998; Fung et al., 2001). Moreover, the level of RAS gene expression in the carotid body is regulated by hypoxia (Leung et al., 2000; Fung et al., 2002). In this context, the augmented activity of the carotid body has been proposed to play a role in the pathophysiology of sleep apnea. Hence, the molecular and cellular mechanisms that mediate the effect of hypoxia on the RAS in the carotid body and the pathophysiological role of the RAS in disease conditions associated with hypoxemia are of great interest. Research studies have been focused on: (a) mechanisms underlying the carotid chemoreceptor response to Ang II; (b) the expression of RAS components in the carotid body; (c) the regulation of the RAS expression in the carotid body, and (d) pathophysiological roles of the alteration of the RAS in the carotid body in disease conditions associated with hypoxemia. This review aims to summarize the literature on the functional expression of RAS in the carotid body and its regulation by hypoxia, highlighting the pathophysiological roles of the RAS in the carotid body and its clinical implications.
Expression of RAS Components in the Carotid Body
The expression and localization of several key RAS components, notably angiotensinogen, which is an indispensable component for the existence of an intrinsic RAS, have been detected in the rat carotid body (Lam and Leung, 2002). Hence, the mRNA and protein of angiotensinogen are expressed in the chemosensitive glomus cells. In addition, mRNA expression of ACE is present despite an absence of the expression of renin in the rat carotid body. These strongly support the localization of an intrinsic angiotensin-generating system functioning via a locally renin-independent biosynthetic pathway in the carotid body (Figure 1). In this regard, the local angiotensin generation of Ang I and Ang II could be mediated by renin in the circulating blood or functional homologous enzymes of renin including tonin, cathepsin G, and kallikrein (Figure 1). Also, angiotensin peptides could be generated by renin-like enzymes expressed in the vasculature (Campbell, 2003). However, the expression of these alternative enzymes in the carotid body has not been investigated. Nevertheness, Ang II locally produced in the carotid body could elevate the local Ang II levels functioning as a paracrine-autocrine signal via the activation of AT1 receptors expressed in the glomus cells.
Expression and Function of Angiotensin Receptors in the Carotid Body
Peripheral infusion of Ang II stimulates respiration in anesthetized animals (Potter and McCloskey, 1979; Ohtake and Jennings, 1993; Ohtake et al., 1993). The respiratory response to Ang II is in part mediated by the carotid chemoreflex because Ang II increases afferent activities of the carotid body (McQueen, 1981; Allen, 1998). The effect of Ang II may be mediated by the sympathetic and parasympathetic efferent fibers innervating the carotid body, which could increase the release of norepinephrine and the afferent activity of the carotid body (McQueen, 1981; Gonzalez et al., 1994). Nevertheless, evidence suggests that Ang II exerts its effect on the chemosensory component of the carotid body. Hence, Ang II at concentrations ranging from physiological to pharmacological levels induces a brief inhibition followed by an excitation of afferent carotid sinus nerve activity in the rat superfused carotid body preparation (Allen, 1998; Leung et al., 2000). The resting activity of the carotid sinus nerve is dose-dependently increased by about two folds upon a threshold concentration of 100 pM at the physiological level of Ang II under normoxic conditions (Allen, 1998; Leung et al., 2000). Also, both the inhibitory and excitatory effects of Ang II are blocked by losartan, supporting the ligand binding is mediated by the AT1 receptors. In addition, in an autoradiographic study, AT receptor-ligand bindings in the carotid body is not reduced by sympathetic nor afferent denervation of the carotid body (Allen, 1998). Indeed, Ang II elevates the level of intracellular calcium in the chemosensitive glomus cells (Fung et al., 2001). The intracellular calcium response to Ang II is blocked by losartan but not by an antagonist for AT2 receptors PD-123319, suggesting an involvement of AT1 receptors. Furthermore, AT1-immunostaining is positively localized in the lobule of the rat carotid body, strongly supporting the expression of AT1 receptors in the glomus cells clustering in glomeruli (Fung et al., 2001). In fact, AT1 receptors are co-localized with cells containing tyrosine hydroxylase, which is a cellular marker of the biosynthesis of catecholamines for the chemotransduction in the carotid body (Fung et al., 2002). However, the AT1-immunoreactivity is not found in all lobules of the parenchyma, meaning that the expression of AT1 receptors is not ubiquitous in the carotid body under physiological conditions (Fung et al., 2001). This is in consistent with functional data showing that the proportional amount of glomus cells responsive to Ang II is about 40% (Fung et al., 2001). Nevertheless, these findings are conclusive that AT1 receptors expressed in the chemosensitive glomus cell mediate the carotid chemoreceptor response to Ang II. It is known that Ang II binding of the AT1 receptor stimulates the phospholipase C pathway in the plasma membrane and leads to the formation of 1,2-diacylglycerol and inositol-1,4,5-triphosphate (IP3), which mobilizes the endoplasmic calcium to store and elevate intracellular calcium (Balla et al., 1998). It is speculated that similar intracellular pathways could mediate the effect of Ang II on the glomus cells and the details of the intracellular signaling pathways need further study.
The mRNA transcript of two subtypes of AT1 receptors, AT1a and AT1b, is expressed in the rat carotid body (Leung et al., 2000; Fung et al., 2002). It has been shown that the AT1a receptor is the main one accounting for the Ang II effect on the chemoreceptor, whereas AT1b might have limited involvement, if any, in the early stage of the maturation. In the postnatal rat, there is an increase in the expression of AT1a subtype but a decrease in the AT1b subtype regulated by hypoxia (Fung et al., 2002). This suggests that the AT1a receptor is the major subtype accounting for the Ang II effect on the carotid body (Fung et al., 2002). In addition to AT1 receptors, mRNA transcripts of the AT2 receptor were found in the carotid body (Leung et al., 2000; Fung et al., 2002). Activation of the AT2 receptor has a wide spectrum of effects for instances on vasodilation, apoptosis and anti-proliferation depending on the cell type (Padia and Carey, 2013). Although the AT1 receptor is the major one mediating the excitatory response of the carotid chemoreceptor, it is possible that Ang II can also exert its effects via the AT2 receptors in the carotid body.
It has been reported that AT4 and Mas receptors are expressed in the carotid body (Fung et al., 2007; Schultz, 2011). Ang IV is an Ang II metabolite containing the 3–8 fragment of the octapeptide, which exerts its physiological effect via the AT4 receptor. It has been reported that activation of AT4 receptors by Ang IV augments the release of acetylcholine in the hippocampus (Chai et al., 2004). In the rat carotid body, positive immunoreactivity against AT4 receptors was found in the chemosensitive glomus cells containing the tyrosine hydroxylase (Fung et al., 2007). It is speculated that AT4 receptors binding with Ang IV could enhance the excitatory effect of Ang II on the carotid chemoreceptor mediated by the AT1 receptor. Supporting this idea, the expression of AT4 receptors in the carotid body is significantly increased under chronically hypoxic condition. Also, Ang IV elevates the intracellular calcium level of the chemosensitive glomus cells despite at a high concentration (10 μM) of Ang IV (Fung et al., 2007). Thus, the AT4 receptor could be a signaling pathway of the RAS in parallel and/or complementary to the AT1 receptor activation. The physiological or pathophysiological significance, if any, of the AT4 receptor expressed in the carotid body has yet to be fully elucidated in future studies. As for the Mas receptor, it is a G-protein coupling receptor with high affinity binding with Ang (1-7), a biologically active peptide converted from Ang I and Ang II, respectively, by ACE2 and ACE. Recent study reported the expression of Mas receptors in the rabbit carotid body and also its decreased expression under a disease condition associated with heart failure (Schultz, 2011). The signaling cascade of Mas receptor activation has been known to induce vasodilation, which is possibly a negative modulation of the functional effects of AT1 receptors. Thus, the Mas receptor may be functionally important in the modulation of the RAS activity in the carotid body and its alteration under disease condtions could be pathologically significant as it may contribute to the imbalance of excitatory and inhibitory modulation of the carotid chemoreceptor activity in disease (Schultz, 2011).
Functions of Local RAS Components in the Carotid Body
The circulating RAS is responsive to alterations in extracellular fluid volume, osmolarity, blood volume or sodium depletion, resulting in an elevated level of Ang II in the plasma (Reid et al., 1978; Matsusaka and Ichikawa, 1997). In addition to the vasoconstrictive effect of Ang II and its stimulating effect on the aldosterone secretion by the adrenal cortex, Ang II stimulates carotid chemoreceptors, which elicits the chemoreflex for the adjustment in cardiopulmonary and autonomic activities. Specifically, activation of the chemoreflex pathway is known to elevate renal sympathetic activities leading to the secretion of renin from the juxtaglomerular cells in the kidney, which could then increase sodium reabsorption and water intake (Honig, 1989; Marshall, 1994). Thus, the physiological significance of the effect of Ang II on the carotid chemoreceptor is that the baseline activity of the carotid chemoreceptor is regulated by Ang II in the circulating blood and also produced by the local RAS, which could elicit the chemoreflex in the absence of hypoxia, hypercapnia, or acidosis. As such, the carotid chemoreceptor could serve as an effector of Ang II for the regulation of blood pressure, electrolyte, and fluid homestasis via the chemoreflex pathway. Moreover, Ang II could potentiate the carotid chemoreceptor response to hypoxia. The plasma Ang II level significantly increases under hypoxic conditions (Zakheim et al., 1976). Also, the presence of local RAS in the carotid body could elevate the level of Ang II and also its metabolites in the local tissue, which could be a major source of Ang II for a more prominent effect on the modulation of the carotid chemoreceptor activity under hypoxic or disease conditions. Hence in parallel to the function of Ang II-sensitive neurons in the circumventricular organs of the brain (Ganong, 2000; McKinley et al., 2003), the carotid chemoreceptor can be an additional effector of Ang II, which confers and provides the peripheral signal integrating to the central output that alters sympathetic and parasympathetic activities for the regulation of cardiovascular and renal activities and also the electrolyte and fluid homeostasis under physiological, hypoxic, and disease conditions.
Sustained Hypoxia Regulates RAS Expression: Implications on Chronic Obstructive Pulmonary Disease
It has been shown that proportion of Ang II-responsive glomus cells (ca. 80%) is increased by 2 folds in the carotid body of rats exposed to 10% inspired oxygen for 4 weeks (sustained hypoxia) (Leung et al., 2000; Fung et al., 2001, 2002). Also, elevated intracellular calcium levels induced by Ang II is three times higher following sustained hypoxia than the normoxic group, which is blocked by losartan (Leung et al., 2000; Fung et al., 2002). Importantly, AT1 receptor-mediated excitation of carotid body chemoreceptor activity is two times higher in rats exposed to sustained hypoxia than in controls (Leung et al., 2000; Fung et al., 2002). Thus, sustained hypoxia induces a significant increase in the sensitivity of the chemoreceptor response to Ang II. In fact, the mRNA expression of the AT1 and AT2 receptor is increased in the carotid body of adult rats exposed to sustained hypoxia (Leung et al., 2000). Also, there is an increased immunoreactivity of AT1receptors in glomic clusters containing tyrosine hydroxylase in the carotid body of rats with sustained hypoxia (Fung et al., 2002). Interestingly, in postnatal exposure to sustained hypoxia, the mRNA expression of AT1a receptors but not the AT1b subtype in the rat carotid body is upregulated, suggesting that the expression of AT1 receptor subtypes is differentially regulated by postnatal hypoxia (Fung et al., 2002). Thus, chronic hypoxemia is a major factor that increases the expression of AT1a receptor at the transcriptional and protein level, resulting in a functional enchancement of the sensitivity of the carotid chemoreceptor to Ang II.
In addition to the upregulation of the AT1 receptor, sustained hypoxia induces increased expressions of several key components of the RAS in the rat carotid body. The effect of sustained hypoxia on the RAS component of the carotid body are: (i) it increases the mRNA and protein level of angiotensinogen expressed in the chemosensitive glomus cell, and (ii) elevated the mRNA expression and enzymatic activities of ACE (Lam and Leung, 2003; Lam et al., 2004). The significance of these RAS alterations regulated by hypoxia is that it could increase the local biosynthesis of Ang II and angiotensin peptides, which increases the carotid chemoreceptor activity; via the chemoreflex, changes the respiratory and cardiovascular activities to match metabolic needs and also adjustments of the autonomic and renal activities to regulate the electrolyte and fluid homeostasis in hypoxia (Honig, 1989). In addition, the increased expression of the AT1 receptor could raise the sensitivity of the carotid body to the electrolyte and fluid disturbance under hypoxic conditions. Indeed, the plasma concentration of Ang II rises in the first week of sustained hypoxia but it returns to a normoxic level by 2 weeks (Zakheim et al., 1976). The plasma renin activity has been reported to be unaltered or increased during hypoxia (Jain et al., 1990; Fletcher et al., 1999; Ip et al., 2002). Thus, the increased expression and activities of the RAS components could play a role in the augmented activity of the carotid chemoreceptor and the chemoreflex pathway by which increases and sustains the renal sympathetic activity under hypoxic conditions. This could lead to activation of the renin-angiotensin-aldosterone system, which is important to increase the sodium and water retention. This might be a part of the compensatory changes following the natriuretic and diuretic effects of carotid chemoreceptor stimulation in acute hypoxia (Honig, 1989). Consequently, the increased sensitivity of the carotid body to Ang II could play a role in the augmentation of cardiorespiratory and the renal sympathetic activities, which is an important part of the response to hypoxia. Moreover, Ang II levels are significantly elevated under pathological conditions for instances hypotension or hemorrhage. Indeed hypotension induces an increase in the discharge rate of carotid chemoreceptors which may be due to a decrease in the blood flow to the carotid body (Lahiri et al., 1980). The elevated carotid chemoreceptor activity could also be mediated by the AT1 receptors and the upregulated RAS components in the carotid body (Leung et al., 2000; Fung et al., 2002).
Sustained hypoxia is closely relevant to the physiological acclimation to high altitude, and also to clinical conditions including chronic obstructive pulmonary disease and congenital heart defects (Forth and Montgomery, 2003; Prabhakar and Peng, 2004). The upregulation of RAS components in the carotid body and the augmented sensitivity of the chemoreceptors to Ang II could play multiple roles in the response of the carotid body to sustained hypoxia. Specifically, the carotid body develops hypertrophy and hyperplasia and also increases vasculature with angiogenesis (Fung and Tipoe, 2003). Ang II is known as a mitogenic factor effecting on vascular cells, although the effect on the glomic tissue is not clear at the moment. In addition, sustained hypoxia modulates the ventilatory response to hypoxia (Bisgard, 2000; Lahiri et al., 2000, 2002). The chemosensitivity of the carotid body to hypoxia is modulated by the counterbalance of effects of excitatory and inhibitory components on the carotid chemoreceptor (Bisgard, 2000; Prabhakar, 2001). Thus, the excitatory effect of Ang II on the glomus cell could augment the chemosensitivity of the carotid chemoreceptor, which may counteract the blunting effect of sustained hypoxia on the ventilatory response to hypoxia. Yet, the extent of the effect of Ang II and the detail of the molecular and cellular mechanisms underlying the functional modulation of the chemoreceptor excitability for the acclimatized changes in the carotid body during hypoxia require further studies.
The RAS plays important roles in the pathogenesis of disease conditions including hypertension, cardiac hypertrophy and heart failure. It has been shown that AT1 receptor antagonist olmesartan significantly lowers the blood pressure and reduces proteinuria and glomerular damage mediated by oxidative stress in hypertensive diabetic animals (Izuhara et al., 2005). It has also been reported that ACE and Ang II play roles in the hypoxia-induced pulmonary hypertension and vascular remodeling (Morrell et al., 1995, 1999). The pathological development of hypoxic cor pulmonale is significantly decreased by olmesartan in rats exposed to sustained hypoxia (Nakamoto et al., 2005). However, in a double-blind study, losartan did not significantly attenuate pulmonary hypertension in a cohort of 40 patients with chronic obstructive pulmonary disease (Morrell et al., 2005). Also, irbesartan, an AT receptor blocker, did not significantly alter the strength of respiratory muscles or spirometric parameters in a randomized trial with about 60 patients with chronic obstructive pulmonary disease, although it significantly decreased the hematocrit. This raises the possibility that blockade of AT receptors may have beneficial effects in the patients with chronic obstructive pulmonary disease (Andreas et al., 2006). A more recent study reported that ACE inhibitor enalapril or AT receptor blocker losartan reduced the mortality of 2249 patients with severe COPD (Ekström et al., 2013). The effect of RAS blockade in disease associated with hypoxia needs more clinical trial studies in future.
Intermittent Hypoxia Regulates RAS Expression: Implications on Sleep-Disordered Breathing
Chronic exposure to episodic hypoxia (intermittent hypoxia) associated with recurrent apneas closely related to pathophysiological conditions including sleep-disordered breathing, obstructive sleep apnea and hypertension (Lesske et al., 1997; Fletcher, 2001). Evidence suggests that the carotid body plays a crucial role in the pathophysiology of sleep apnea and it pathophysiological consequences induced by intermittent hypoxia (Prabhakar et al., 2001, 2005; Peng et al., 2003; Iturriaga et al., 2005). Thus, there is an augmented carotid chemoreceptor activity and its chemosensitivity in animals exposed to intermittent hypoxia (Peng and Prabhakar, 2004; Peng et al., 2004; Rey et al., 2004). Also, intermittent hypoxia induces increases in the blood pressure (Fletcher et al., 1992a,b), activities of sympathetic nerve (Greenberg et al., 1999; Fletcher, 2003), plasm levels of catecholamines (Bao et al., 1997), long-term facilitation (LTF) of the respiratory motor activity and the ventilatory response to hypoxia (McGuire et al., 2004; Rey et al., 2004; Katayama et al., 2005). Moreover, denervation of the carotid afferent activity dramatically attenuates the elevated blood pressure in responding to intermittent hypoxia, indicating that the carotid chemoreceptor activity plays an important role in the pathogenic cascades induced by intermittent hypoxia (Fletcher et al., 1992a). Furthermore, intermittent hypoxia resembles ischemia-reperfusion of tissues and organs, leading to excessive production of ROS, which could underpin the long-term effects of intermittent hypoxia on the carotid body. This is supported by the observation that ROS scavengers attenuate the hypoxic sensitivity and the magnitude of LTF induced by intermittent hypoxia (Prabhakar and Kumar, 2004). Thus, ROS play a crucial role in the altered carotid body function in intermittent hypoxia.
As aforementioned, Ang II stimulates ventilation and the plasma Ang II level increases under hypoxic conditions (Zakheim et al., 1976; Ohtake et al., 1993). Activation of AT1 receptors in the carotid body increases the afferent activity and the hypoxic sensory response of the chemoreflex and sympathetic output (Leung et al., 2000). In experimental animals, losartan significantly reduces the elevated arterial pressure induced by intermittent hypoxia, suggesting that the RAS is involved in the pathogenic cascade (Fletcher et al., 1999). Indeed, there are significantly increased levels of serum Ang II and VEGF in patients with obstructive sleep apnea (Barcelo et al., 2001; Moller et al., 2003). Blocker of AT1 receptors olmesartan significantly decreases the VEGF expression induced by Ang II in the peripheral blood mononuclear cell (Takahashi et al., 2005). Thus, activation of the AT1 receptor plays a role in the pathogenic event of obstructive sleep apnea. Recent studies have examined the hypothesis that the RAS in the carotid body plays a role in the augmented carotid chemoreceptor activity induced by intermittent hypoxia, which may be mechanistically leading to breathing instability in the pathophysiology of sleep apnea.
Recent studies reported that intermittent hypoxia induces a functional upregulation of the RAS expression in the rat carotid body (Lam et al., 2014). The increased mRNA and protein expression of AT1 receptors causes an enhancement of the sensitivity to Ang II in the carotid chemosensitive cells, which could lead to an increase in the CB excitability and renal sympathetic activity (Marcus et al., 2010; Lam et al., 2014). In effect, the activation of RAS in the carotid body during intermittent hypoxia has pathophysiological and clinical significance because the chemoreflex plays an important role in the sustained increases in the sympathetic outflow and the systemic arterial pressure (Fletcher, 2001). Indeed, it has been shown that the systemic hypertension induced by intermittent hypoxia is blocked by the denervation of the carotid body, ablation of the sympathetic nerve, renal sympathectomy, adrenal medullectomy, and also by AT receptor antagonist (Fletcher et al., 1999; Fletcher, 2001). These data support the hypothesis that the RAS expression in the carotid body plays a role in the pathogenic cascade induced by intermittent hypoxia, which increases the cardiovascular morbidity in OSA patients.
Ang II stimulates the [Ca2+]i elevation in the chemosensitive glomus cells and the Ang II response is enhanced in the hypoxic group via the upregulation of AT1 receptor expression (Lam et al., 2014). The [Ca2+]i response to AT was inhibited by AT1 antagonist losartan but not by an AT2 antagonist, confirming that AT binding to the AT1 receptor stimulates the intracellular signaling pathway and elevates [Ca2+]i in the glomus cells. In effect, Ang II evokes sensory long-term potentiation of the carotid body, which was blocked by losartan (Peng et al., 2011). Also, losartan reduced the elevated sympathetic responses to hypoxia and cyanide (Marcus et al., 2010). These results suggest that the upregulation of the AT1 receptor expression plays a role in the augmented CB excitability during intermittent hypoxia, highlighting the mechanistic significance of the RAS in mediating the IH-induced pathophysiological development of sympathetic overactivity and hypertension.
In addition to AT1 receptors, the gene transcripts of AT2 receptor have shown to be increased in the carotid body in intermittent hypoxia. The increased expression of the AT2 receptors might be related to the lack of increase in the volume of the carotid body in the rat (Lam et al., 2008). Indeed, AT2 receptors have been implicated in the stimulation of apoptosis and the activation of AT2 receptor results in growth inhibition and promotion of apoptosis associated with the inhibition of MAP kinases, such as extracellular regulated kinases, probably by the activation of phospho-tyrosine phosphatase (Schmitz and Berk, 1997; de Gasparo and Siragy, 1999). The activated local RAS via AT2 receptors in the carotid body might inhibit the cell growth and promote apoptotic cell death, resulting in an insignificant change in the volume of the carotid body in intermittent hypoxia. However, further investigations are needed for addressing the role of AT2 receptors in the carotid body.
Moreover, the expression of angiotensinogen in the carotid body is significantly elevated by intermittent hypoxia (Lam et al., 2014). The mRNA and protein expression of angiotensinogen was specifically localized to the glomus cells of the carotid body, suggesting a transcriptional upregulation and/or mRNA stabilization of the angiotensinogen expression induced by intermittent hypoxia. Also, the mRNA level of ACE was significantly increased in the rat carotid body (Lam et al., 2014). Thus, evidence supports that intermittent hypoxia induces an upregulation of the local RAS components in the rat carotid body. Since angiotensinogen is the sole precursor of Ang II, elevated levels of the expression of angiotensinogen could lead to an increase in the local production of Ang II in the carotid body in intermittent hypoxia. Moreover, the increased expression of ACE in the carotid body could enhance the kinetics of enzymatic convertion of Ang I to Ang II in the carotid body. Also, increased ACE activities and plasma Ang II levels have been reported in OSA patients (Barcelo et al., 2001; Moller et al., 2003). In effect, the elevated level of circulating and locally produced Ang II, together with an increased expression of the AT receptors could enhance the effect of Ang II on the chemosensory component of the carotid body. Thus, evidence supports the upregulated AT receptors with increased expression of RAS components in the carotid body play a pathogenic role in the augmented excitability of the carotid chemoreceptor under intermittent hypoxia associated with sleep apnea.
Oxidative stress with an increased generation of ROS plays an essential role in IH-induced alterations in the carotid body function (Prabhakar et al., 2001). It has been shown that ROS modulate the mobilization of [Ca2+]i store mediated by IP3signaling pathway, leading to an increase in hypoxia-induced neurotransmitter release in IH (Prabhakar and Kumar, 2004). Studies have also shown that oxidative stress is involved in the augmented carotid chemoreceptor activity (Pawar et al., 2009; Peng et al., 2009; Del Rio et al., 2010, 2011b). Emerging data suggest that Ang II is a mediator of oxidative stress, in which ROS induced by Ang II are an important signaling intermediates in several signal transduction pathways involved in the pathophysiology (Paravicini and Touyz, 2006) and inflammation (Duprez, 2006). In this regard, vascular inflammation induced by Ang II is mainly mediated by AT1 receptors associated with an increased production of ROS via the NADPH oxidase in the vascular wall (Griendling et al., 1994; Rajagopalan et al., 1996; Dandona et al., 2003), which is closely related to the local RAS function (Shimizu et al., 1998). In the carotid body, AT receptors are also expressed in the vascular cells in addition to the glomus cells, although its role is unclear. Nevertheless, it has been shown that losartan treatment could normalize IH-induced superoxide production and expression of AT1 receptors (Marcus et al., 2010). Also, ROS production induced by Ang II mediates sensory long-term potentiation in the carotid body via activation of gp91phox (Peng et al., 2011), and the gp91phox is expressed in glomus cells of the CB (Youngson et al., 1997). Thus, the IH–induced RAS upregulation in the carotid body may contribute to the AT-induced ROS production. Furthermore, losartan treatment of the rat in intermittent hypoxia attenuates the levels of oxidative stress and macrophage infiltration, supporting a pathogenic role of AT1 receptors in the local inflammation of the carotid body (Lam et al., 2014). In this context, intermittent hypoxia induces increased expressions of proinflammatory cytokines and mediators as well as infiltration of immunogic cells to the carotid body under hypoxic condtions (Lam et al., 2008, 2012; Liu et al., 2009; Del Rio et al., 2011a, 2012). The inflammatory response of the carotid body to intermittent hypoxia has also been proposed to play a role in the augmented activity of the carotid chemoreceptor (Lam et al., 2008, 2012; Liu et al., 2009). These findings strongly suggest a paracrine-autocrine mechanism mediating altered functions of the carotid body, including the augmented chemosensitivity and the local inflammation of the carotid body. Thus, intermittent hypoxia could activate an intrinsic angiotensin-generating system, which increases local biosynthesis of Ang II via increased expressions of RAS components in the carotid body. The upregulation of angiotensinogen, AT receptors and ACE expression could play a pathogenic role in the augmented activity of carotid chemosensitive cells and the inflammation of the carotid body in intermittent hypoxia, which is relevant to the early pathogenesis in sleep-disordered breathing.
The RAS has been proposed to play a role in the cardiovascular consequences of sleep apnea, including systemic hypertension. In addition to the continuous positive airway pressure therapy, targeting the RAS has been proposed as a pharmacological management of the patients with sleep apnea. In fact, inhibitors of ACE have been shown to attenuate the arterial pressures and decrease the apnea and hypoapnea index in patients with obstructive sleep apnea (Peter et al., 1989; Mayer et al., 1990; Grote et al., 1995). Also, the AT receptor blockers have been shown to attenuate the elevated pressure and oxidative stress induced by intermittent hypoxia in subjects (Foster et al., 2010; Pialoux et al., 2011) or in the patient with obstructive sleep apnea (Heitmann et al., 2010; Dohi et al., 2011). The beneficial effect of the RAS blockade might be explained by diminished sympathetic and adrenergic activities (Fletcher, 2000, 2003). Nevertheless, these human studies were performed in a small number of patients and the long term treatment with RAS blockers has not been evaluated in clinical trials (Parati et al., 2013). Future clinical studies are required to support the antihypertensive benefit of blockade of RAS for the management of patients with obstructive sleep apnea and hypertension.
An Involvement of the RAS in Heart Failure
Experimental studies have shown that Ang II enhances the hypoxic chemosensitivity of the carotid body in a rabbit model of chronic heart failure (CHF). Hence, Ang II augments hypoxia-induced renal sympathetic nerve activity (RSNA) and there are significant increases in the expression of AT1 receptors in the carotid body of CHF rabbits (Li et al., 2006). Also, L-158809, an AT1 receptor antagonist, attenuates hypoxia-induced responses of the RSNA in CHF rabbits. In addition, L-158809 decreases the chemoreceptor responses to hypoxia in CHF rabbits, suggesting that increases in Ang II and the expression of AT1 receptor in the carotid body play a role in the augmented carotid chemoreceptor activity and chemoreflex-mediated sympathetic overactivity in CHF (Li et al., 2006). Furthermore, Ang II at a concentration of 0.1 nM increases the sensitivity of potassium (Kv) currents and resting membrane potential to hypoxia and L-158809 reduces the sensitivity of Kv currents and resting membrane potential to hypoxia in CHF glomus cells. These results suggest that Ang II-AT1 receptor signaling pathway increases the sensitivity of Kv channels to hypoxia in the glomus cells of the CHF rabbit (Li and Schultz, 2006). Moreover, the effect of Ang II on the augmented chemoreceptor activity is medated by a NADPH oxidase-superoxide signaling pathway (Li et al., 2007).
Sleep-disordered breathing with central or obstructive sleep apnea is frequently observed in patients with heart failure. Sleep-disordered breathing has been known to have a negative impact on the CHF patient and so clinical treatment of sleep-disordered breathing could improve cardiac performance and long-term outcomes in these patients. Also, cardiac dysfunction may play a role in the pathophysiology of sleep apnea, although the interrelationship between heart failure and sleep apnea remains to be established (Caples et al., 2005). In this context, an increase in sympathetic activities is a hallmark of the CHF state, which could be mediated by a decrease in the sensory feedback from cardiopulmonary activities and arterial baroreceptors. As mentioned above, the sustained increase in RSNA could involve Ang II and the RAS in the carotid body of CHF rabbits (Schultz, 2011; Patel and Schultz, 2013). Indeed, recent studies show that cryoablation of the carotid body normalizes the RSNA and breathing stability and improves survival in CHF animals (Del Rio et al., 2013; Marcus et al., 2014). Hence, the RAS components could be therapeutic targets for the treatment of CHF patients.
Conclusions
Findings of expression and functional studies suggest that the AT1 receptor regulates the excitability of the carotid chemoreceptor. Hence, Ang II elevates the level of intracellular calcium in the chemosensitive glomus cells and the activity of carotid chemoreceptors. As a result, activation of the chemoreflex could be a peripheral control important for the physiological response to hypoxia and the maintenance of electrolyte and fluid homeostasis. In addition, the expression of AT receptors in the carotid body is regulated by hypoxia. In effect, sustained hypoxia induces an upregulation of AT1 receptor expression, which increases the sensitivity of the chemoreceptor response to Ang II. This regulation may be important in the modulation of the carotid body functions responsible for the hypoxic ventilatory response, for enhancing the cardiorespiratory response and adjusting electrolyte and water homeostasis during sustained hypoxia. Furthermore, RAS components are locally expressed in the carotid body and the increased RAS expressions are closely relevant to the pathogenesis of disease including sleep-disordered breathing and heart failure. Specifically, the upregulation of the expression of angiotensinogen, ACE and AT1 receptors could play a significant role in the augmented carotid chemoreceptor activity, via the increased activity of chemoreflex, contributing to the pathophysiology of sleep apnea and the sympatho-excitation that is central to the endothelial dysfunction and heart failure during the course of pathogenesis. Future studies in this direction warrant a better understanding of the pathogenic role of RAS in the carotid body in the disease associated with hypoxemia.
Conflict of Interest Statement
The author declares that the research was conducted in the absence of any commercial or financial relationships that could be construed as a potential conflict of interest.
Acknowledgments
The authors wish to thank the support from the Research Grants Council of Hong Kong, Competitive Earmarked Research Grants: HKU 766110M, HKU 7510/06M and research grants from the University Research Committee of the University of Hong Kong.
References
Allen, A. M. (1998). Angiotensin AT1 receptor-mediated excitation of rat carotid body chemoreceptor afferent activity. J. Physiol. 510, 773–781. doi: 10.1111/j.1469-7793.1998.773bj.x
Andreas, S., Herrmann-Lingen, C., Raupach, T., Luthje, L., Fabricius, J. A., Hruska, N., et al. (2006). Angiotensin II blockers in obstructive pulmonary disease: a randomised controlled trial. Eur. Respir. J. 27, 972–979. doi: 10.1183/09031936.06.00098105
Balla, T., Varnai, P., Tian, Y., and Smith, R. D. (1998). Signaling events activated by angiotensin II receptors: what goes before and after the calcium signals. Endocr. Res. 24, 335–344. doi: 10.3109/07435809809032613
Bao, G., Metreveli, N., Li, R., Taylor, A., and Fletcher, E. C. (1997). Blood pressure response to chronic episodic hypoxia: role of the sympathetic nervous system. J. Appl. Physiol. 83, 95–101.
Barcelo, A., Elorza, M. A., Barbe, F., Santos, C., Mayoralas, L. R., and Agusti, A. G. (2001). Angiotensin converting enzyme in patients with sleep apnoea syndrome: plasma activity and gene polymorphisms. Eur. Respir. J. 17, 728–732. doi: 10.1183/09031936.01.17407280
Bisgard, G. E. (2000). Carotid body mechanisms in acclimatization to hypoxia. Respir. Physiol. 121, 237–246. doi: 10.1016/S0034-5687(00)00131-6
Campbell, D. J. (2003). The renin-angiotensin and the kallikrein-kinin systems. Int. J. Biochem. Cell Biol. 35, 784–791. doi: 10.1016/S1357-2725(02)00262-5
Caples, S. M., Wolk, R., and Somers, V. K. (2005). Influence of cardiac function and failure on sleep-disordered breathing: evidence for a causative role. J. Appl. Physiol. 99, 2433–2439. doi: 10.1152/japplphysiol.00676.2005
Chai, S. Y., Fernando, R., Peck, G., Ye, S. Y., Mendelsohn, F. A., Jenkins, T. A., et al. (2004). The angiotensin IV/AT4 receptor. Cell. Mol. Life Sci. 61, 2728–2737. doi: 10.1007/s00018-004-4246-1
Dandona, P., Kumar, V., Aljada, A., Ghanim, H., Syed, T., Hofmayer, D., et al. (2003). Angiotensin II receptor blocker valsartan suppresses reactive oxygen species generation in leukocytes, nuclear factor-kappa B, in mononuclear cells of normal subjects: evidence of an antiinflammatory action. J. Clin. Endocrinol. Metab. 88, 4496–4501. doi: 10.1210/jc.2002-021836
de Gasparo, M., and Siragy, H. M. (1999). The AT2 receptor: fact, fancy and fantasy. Regul. Pept. 81, 11–24. doi: 10.1016/S0167-0115(99)00023-3
Del Rio, R., Marcus, N. J., and Schultz, H. D. (2013). Carotid chemoreceptor ablation improves survival in heart failure: rescuing autonomic control of cardiorespiratory function. J. Am. Coll. Cardiol. 62, 2422–2430. doi: 10.1016/j.jacc.2013.07.079
Del Rio, R., Moya, E. A., and Iturriaga, R. (2010). Carotid body and cardiorespiratory alterations in intermittent hypoxia: the oxidative link. Eur. Respir. J. 36, 143–150. doi: 10.1183/09031936.00158109
Del Rio, R., Moya, E. A., and Iturriaga, R. (2011a). Differential expression of pro-inflammatory cytokines, endothelin-1 and nitric oxide synthases in the rat carotid body exposed to intermittent hypoxia. Brain Res. 1395, 74–85. doi: 10.1016/j.brainres.2011.04.028
Del Rio, R., Moya, E. A., Parga, M. J., Madrid, C., and Iturriaga, R. (2012). Carotid body inflammation and cardiorespiratory alterations in intermittent hypoxia. Eur. Respir. J. 39, 1492–1500. doi: 10.1183/09031936.00141511
Del Rio, R., Munoz, C., Arias, P., Court, F. A., Moya, E. A., and Iturriaga, R. (2011b). Chronic intermittent hypoxia-induced vascular enlargement and VEGF upregulation in the rat carotid body is not prevented by antioxidant treatment. Am. J. Physiol. Lung Cell. Mol. Physiol. 301, L702–L711. doi: 10.1152/ajplung.00128.2011
Dohi, T., Narui, K., Kasai, T., Takaya, H., Inoshita, A., Maeno, K., et al. (2011). Effects of olmesartan on blood pressure and insulin resistance in hypertensive patients with sleep-disordered breathing. Heart Vessels 26, 603–608. doi: 10.1007/s00380-010-0104-2
Duprez, D. A. (2006). Role of the renin-angiotensin-aldosterone system in vascular remodeling and inflammation: a clinical review. J. Hypertens. 24, 983–991. doi: 10.1097/01.hjh.0000226182.60321.69
Ekström, M. P., Hermansson, A. B., and Ström, K. E. (2013). Effects of cardiovascular drugs on mortality in severe chronic obstructive pulmonary disease. Am. J. Respir. Crit. Care Med. 187, 715–720. doi: 10.1164/rccm.201208-1565OC
Fletcher, E. C. (2000). Effect of episodic hypoxia on sympathetic activity and blood pressure. Respir. Physiol. 119, 189–197. doi: 10.1016/S0034-5687(99)00114-0
Fletcher, E. C. (2001). Invited review: physiological consequences of intermittent hypoxia: systemic blood pressure. J. Appl. Physiol. 90, 1600–1605.
Fletcher, E. C. (2003). Sympathetic over activity in the etiology of hypertension of obstructive sleep apnea. Sleep 26, 15–19.
Fletcher, E. C., Bao, G., and Li, R. (1999). Renin activity and blood pressure in response to chronic episodic hypoxia. Hypertension 34, 309–314. doi: 10.1161/01.HYP.34.2.309
Fletcher, E. C., Lesske, J., Behm, R., Miller, C. C. 3rd. Stauss, H., and Unger, T. (1992a). Carotid chemoreceptors, systemic blood pressure, and chronic episodic hypoxia mimicking sleep apnea. J. Appl. Physiol. 72, 1978–1984.
Fletcher, E. C., Lesske, J., Qian, W., Miller, C. C. 3rd., and Unger, T. (1992b). Repetitive, episodic hypoxia causes diurnal elevation of blood pressure in rats. Hypertension 19, 555–561.
Forth, R., and Montgomery, H. (2003). ACE in COPD: a therapeutic target? Thorax 58, 556–558. doi: 10.1136/thorax.58.7.556
Foster, G. E., Hanly, P. J., Ahmed, S. B., Beaudin, A. E., Pialoux, V., and Poulin, M. J. (2010). Intermittent hypoxia increases arterial blood pressure in humans through a Renin-Angiotensin system-dependent mechanism. Hypertension 56, 369–377. doi: 10.1161/HYPERTENSIONAHA.110.152108
Fung, M. L., Lam, S. Y., Chen, Y., Dong, X., and Leung, P. S. (2001). Functional expression of angiotensin II receptors in type-I cells of the rat carotid body. Pflugers Arch. 441, 474–480. doi: 10.1007/s004240000445
Fung, M. L., Lam, S. Y., Dong, X., Chen, Y., and Leung, P. S. (2002). Postnatal hypoxemia increases angiotensin II sensitivity and up-regulates AT1a angiotensin receptors in rat carotid body chemoreceptors. J. Endocrinol. 173, 305–313. doi: 10.1677/joe.0.1730305
Fung, M. L., Lam, S. Y., Wong, T. P., Tjong, Y. W., and Leung, P. S. (2007). Carotid Body AT(4) receptor expression and its upregulation in chronic hypoxia. Open Cardiovasc. Med. J. 1, 1–7. doi: 10.2174/1874192400701010001
Fung, M. L., and Tipoe, G. L. (2003). Role of HIF-1 in physiological adaptation of the carotid body during chronic hypoxia. Adv. Exp. Med. Biol. 536, 593–601. doi: 10.1007/978-1-4419-9280-2_75
Ganong, W. F. (2000). Circumventricular organs: definition and role in the regulation of endocrine and autonomic function. Clin. Exp. Pharmacol. Physiol. 27, 422–427. doi: 10.1046/j.1440-1681.2000.03259.x
Gonzalez, C., Almaraz, L., Obeso, A., and Rigual, R. (1994). Carotid body chemoreceptors: from natural stimuli to sensory discharges. Physiol. Rev. 74, 829–898.
Greenberg, H. E., Sica, A., Batson, D., and Scharf, S. M. (1999). Chronic intermittent hypoxia increases sympathetic responsiveness to hypoxia and hypercapnia. J. Appl. Physiol. 86, 298–305.
Griendling, K. K., Minieri, C. A., Ollerenshaw, J. D., and Alexander, R. W. (1994). Angiotensin II stimulates NADH and NADPH oxidase activity in cultured vascular smooth muscle cells. Circ. Res. 74, 1141–1148. doi: 10.1161/01.RES.74.6.1141
Grote, L., Heitmann, J., Penzel, T., Cassel, W., Ploch, T., Peter, J. H., et al. (1995). Arterial hypertension and sleep apnoea: effect of the angiotensin-converting enzyme (ACE) inhibitor cilazapril on continuously measured blood pressure during sleep and wakefulness. J. Sleep Res. 4, 112–116. doi: 10.1111/j.1365-2869.1995.tb00199.x
Heitmann, J., Greulich, T., Reinke, C., Koehler, U., Vogelmeier, C., Becker, H. F., et al. (2010). Comparison of the effects of nebivolol and valsartan on BP reduction and sleep apnoea activity in patients with essential hypertension and OSA. Curr. Med. Res. Opin. 26, 1925–1932. doi: 10.1185/03007995.2010.497326
Honig, A. (1989). Peripheral arterial chemoreceptors and reflex control of sodium and water homeostasis. Am. J. Physiol. 257, R1282–R1302.
Ip, S. P., Chan, Y. W., and Leung, P. S. (2002). Effects of chronic hypoxia on the circulating and pancreatic renin-angiotensin system. Pancreas 25, 296–300. doi: 10.1097/00006676-200210000-00013
Iturriaga, R., Rey, S., and Del Rio, R. (2005). Cardiovascular and ventilatory acclimatization induced by chronic intermittent hypoxia: a role for the carotid body in the pathophysiology of sleep apnea. Biol. Res. 38, 335–340. doi: 10.4067/S0716-97602005000400004
Izuhara, Y., Nangaku, M., Inagi, R., Tominaga, N., Aizawa, T., Kurokawa, K., et al. (2005). Renoprotective properties of angiotensin receptor blockers beyond blood pressure lowering. J. Am. Soc. Nephrol. 16, 3631–3641. doi: 10.1681/ASN.2005050522
Jain, S., Wilke, W. L., and Tucker, A. (1990). Age-dependent effects of chronic hypoxia on renin-angiotensin and urinary excretions. J. Appl. Physiol. 69, 141–146.
Katayama, K., Sato, K., Matsuo, H., Hotta, N., Sun, Z., Ishida, K., et al. (2005). Changes in ventilatory responses to hypercapnia and hypoxia after intermittent hypoxia in humans. Respir. Physiol. Neurobiol. 146, 55–65. doi: 10.1016/j.resp.2004.11.007
Lahiri, S., Di Giulio, C., and Roy, A. (2002). Lessons from chronic intermittent and sustained hypoxia at high altitudes. Respir. Physiol. Neurobiol. 130, 223–233. doi: 10.1016/S0034-5687(01)00343-7
Lahiri, S., Nishino, T., Mokashi, A., and Mulligan, E. (1980). Relative responses of aortic body and carotid body chemoreceptors to hypotension. J. Appl. Physiol. 48, 781–788.
Lahiri, S., Rozanov, C., and Cherniack, N. S. (2000). Altered structure and function of the carotid body at high altitude and associated chemoreflexes. High Alt. Med. Biol. 1, 63–74. doi: 10.1089/152702900320694
Lam, S. Y., Fung, M. L., and Leung, P. S. (2004). Regulation of the angiotensin-converting enzyme activity by a time-course hypoxia in the carotid body. J. Appl. Physiol. 96, 809–813. doi: 10.1152/japplphysiol.00684.2003
Lam, S. Y., and Leung, P. S. (2002). A locally generated angiotensin system in rat carotid body. Regul. Pept. 107, 97–103. doi: 10.1016/S0167-0115(02)00068-X
Lam, S. Y., and Leung, P. S. (2003). Chronic hypoxia activates a local angiotensin-generating system in rat carotid body. Mol. Cell. Endocrinol. 203, 147–153. doi: 10.1016/S0303-7207(03)00087-X
Lam, S. Y., Liu, Y., Ng, K. M., Lau, C. F., Liong, E. C., Tipoe, G. L., et al. (2012). Chronic intermittent hypoxia induces local inflammation of the rat carotid body via functional upregulation of proinflammatory cytokine pathways. Histochem. Cell Biol. 137, 303–317. doi: 10.1007/s00418-011-0900-5
Lam, S. Y., Liu, Y., Ng, K. M., Liong, E. C., Tipoe, G. L., Leung, P. S., et al. (2014). Up-regulation of a local renin-angiotensin system in the carotid body during chronic intermittent hypoxia. Exp. Physiol. 99, 220–231. doi: 10.1113/expphysiol.2013.074591
Lam, S. Y., Tipoe, G. L., Liong, E. C., and Fung, M. L. (2008). Chronic hypoxia upregulates the expression and function of proinflammatory cytokines in the rat carotid body. Histochem. Cell Biol. 130, 549–559. doi: 10.1007/s00418-008-0437-4
Lesske, J., Fletcher, E. C., Bao, G., and Unger, T. (1997). Hypertension caused by chronic intermittent hypoxia–influence of chemoreceptors and sympathetic nervous system. J. Hypertens. 15, 1593–1603. doi: 10.1097/00004872-199715120-00060
Leung, P. S., Lam, S. Y., and Fung, M. L. (2000). Chronic hypoxia upregulates the expression and function of AT(1) receptor in rat carotid body. J. Endocrinol. 167, 517–524. doi: 10.1677/joe.0.1670517
Li, Y. L., Gao, L., Zucker, I. H., and Schultz, H. D. (2007). NADPH oxidase-derived superoxide anion mediates angiotensin II-enhanced carotid body chemoreceptor sensitivity in heart failure rabbits. Cardiovasc. Res. 75, 546–554. doi: 10.1016/j.cardiores.2007.04.006
Li, Y. L., and Schultz, H. D. (2006). Enhanced sensitivity of Kv channels to hypoxia in the rabbit carotid body in heart failure: role of angiotensin II. J. Physiol. 575, 215–227. doi: 10.1113/jphysiol.2006.110700
Li, Y. L., Xia, X. H., Zheng, H., Gao, L., Li, Y. F., Liu, D., et al. (2006). Angiotensin II enhances carotid body chemoreflex control of sympathetic outflow in chronic heart failure rabbits. Cardiovasc. Res. 71, 129–138. doi: 10.1016/j.cardiores.2006.03.017
Liu, X., He, L., Stensaas, L., Dinger, B., and Fidone, S. (2009). Adaptation to chronic hypoxia involves immune cell invasion and increased expression of inflammatory cytokines in rat carotid body. Am. J. Physiol. 296, L158–L166. doi: 10.1152/ajplung.90383.2008
Marcus, N. J., Del Rio, R., Schultz, E. P., Xia, X. H., and Schultz, H. D. (2014). Carotid body denervation improves autonomic and cardiac function and attenuates disordered breathing in congestive heart failure. J. Physiol. 592, 391–408. doi: 10.1113/jphysiol.2013.266221
Marcus, N. J., Li, Y. L., Bird, C. E., Schultz, H. D., and Morgan, B. J. (2010). Chronic intermittent hypoxia augments chemoreflex control of sympathetic activity: role of the angiotensin II type 1 receptor. Respir. Physiol. Neurobiol. 171, 36–45. doi: 10.1016/j.resp.2010.02.003
Marshall, J. M. (1994). Peripheral chemoreceptors and cardiovascular regulation. Physiol. Rev. 74, 543–594.
Matsusaka, T., and Ichikawa, I. (1997). Biological functions of angiotensin and its receptors. Annu. Rev. Physiol. 59, 395–412. doi: 10.1146/annurev.physiol.59.1.395
Mayer, J., Weichler, U., Herres-Mayer, B., Schneider, H., Marx, U., and Peter, J. H. (1990). Influence of metoprolol and cilazapril on blood pressure and on sleep apnea activity. J. Cardiovasc. Pharmacol. 16, 952–961. doi: 10.1097/00005344-199012000-00014
McGuire, M., Zhang, Y., White, D. P., and Ling, L. (2004). Serotonin receptor subtypes required for ventilatory long-term facilitation and its enhancement after chronic intermittent hypoxia in awake rats. Am. J. Physiol. Regul. Integr. Comp. Physiol. 286, R334–R341. doi: 10.1152/ajpregu.00463.2003
McKinley, M. J., Albiston, A. L., Allen, A. M., Mathai, M. L., May, C. N., McAllen, R. M., et al. (2003). The brain renin-angiotensin system: location and physiological roles. Int. J. Biochem. Cell Biol. 35, 901–918. doi: 10.1016/S1357-2725(02)00306-0
McQueen, D. S. (1981). “Effects of some polypeptides on carotid chemoreceptor activity,” in Arterial Chemoreceptors, eds C. Belmonte, D. J. Pallot, H. Acker, and S. Fidone (Leicester: Leicester University Press), 299–308.
Moller, D. S., Lind, P., Strunge, B., and Pedersen, E. B. (2003). Abnormal vasoactive hormones and 24-hour blood pressure in obstructive sleep apnea. Am. J. Hypertens. 16, 274–280. doi: 10.1016/S0895-7061(02)03267-3
Morrell, N. W., Higham, M. A., Phillips, P. G., Shakur, B. H., Robinson, P. J., and Beddoes, R. J. (2005). Pilot study of losartan for pulmonary hypertension in chronic obstructive pulmonary disease. Respir. Res. 6:88. doi: 10.1186/1465-9921-6-88
Morrell, N. W., Morris, K. G., and Stenmark, K. R. (1995). Role of angiotensin-converting enzyme and angiotensin II in development of hypoxic pulmonary hypertension. Am. J. Physiol. 269, H1186–H1194.
Morrell, N. W., Upton, P. D., Kotecha, S., Huntley, A., Yacoub, M. H., Polak, J. M., et al. (1999). Angiotensin II activates MAPK and stimulates growth of human pulmonary artery smooth muscle via AT1 receptors. Am. J. Physiol. 277, L440–L448.
Nakamoto, T., Harasawa, H., Akimoto, K., Hirata, H., Kaneko, H., Kaneko, N., et al. (2005). Effects of olmesartan medoxomil as an angiotensin II-receptor blocker in chronic hypoxic rats. Eur. J. Pharmacol. 528, 43–51. doi: 10.1016/j.ejphar.2005.10.063
Ohtake, P. J., and Jennings, D. B. (1993). Angiotensin II stimulates respiration in awake dogs and antagonizes baroreceptor inhibition. Respir. Physiol. 91, 335–351. doi: 10.1016/0034-5687(93)90110-V
Ohtake, P. J., Walker, J. K., and Jennings, D. B. (1993). Renin-angiotensin system stimulates respiration during acute hypotension but not during hypercapnia. J. Appl. Physiol. 74, 1220–1228.
Padia, S. H., and Carey, R. M. (2013). AT2 receptors: beneficial counter-regulatory role in cardiovascular and renal function. Pflugers Arch. 465, 99–110. doi: 10.1007/s00424-012-1146-3
Parati, G., Lombardi, C., Hedner, J., Bonsignore, M. R., Grote, L., Tkacova, R., et al. (2013). Recommendations for the management of patients with obstructive sleep apnoea and hypertension. Eur. Respir. J. 41, 523–538. doi: 10.1183/09031936.00226711
Paravicini, T. M., and Touyz, R. M. (2006). Redox signaling in hypertension. Cardiovasc. Res. 71, 247–258. doi: 10.1016/j.cardiores.2006.05.001
Patel, K. P., and Schultz, H. D. (2013). Angiotensin peptides and nitric oxide in cardiovascular disease. Antioxid. Redox. Signal. 19, 1121–1132. doi: 10.1089/ars.2012.4614
Pawar, A., Nanduri, J., Yuan, G. X., Khan, S. A., Wang, N., Kumar, G. K., et al. (2009). Reactive oxygen species-dependent endothelin signaling is required for augmented hypoxic sensory response of the neonatal carotid body by intermittent hypoxia. Am. J. Physiol. 296, R735–R742. doi: 10.1152/ajpregu.90490.2008
Peach, M. J. (1977). Renin-angiotensin system: biochemistry and mechanisms of action. Physiol. Rev. 57, 313–370.
Peng, Y. J., Nanduri, J., Yuan, G., Wang, N., Deneris, E., Pendyala, S., et al. (2009). NADPH oxidase is required for the sensory plasticity of the carotid body by chronic intermittent hypoxia. J. Neurosci. 29, 4903–4910. doi: 10.1523/JNEUROSCI.4768-08.2009
Peng, Y. J., Overholt, J. L., Kline, D., Kumar, G. K., and Prabhakar, N. R. (2003). Induction of sensory long-term facilitation in the carotid body by intermittent hypoxia: implications for recurrent apneas. Proc. Natl. Acad. Sci. U.S.A. 100, 10073–10078. doi: 10.1073/pnas.1734109100
Peng, Y. J., and Prabhakar, N. R. (2004). Effect of two paradigms of chronic intermittent hypoxia on carotid body sensory activity. J. Appl. Physiol. 96, 1236–1242. doi: 10.1152/japplphysiol.00820.2003
Peng, Y. J., Raghuraman, G., Khan, S. A., Kumar, G. K., and Prabhakar, N. R. (2011). Angiotensin II evokes sensory long-term facilitation of the carotid body via NADPH oxidase. J. Appl. Physiol. 111, 964–970. doi: 10.1152/japplphysiol.00022.2011
Peng, Y. J., Rennison, J., and Prabhakar, N. R. (2004). Intermittent hypoxia augments carotid body and ventilatory response to hypoxia in neonatal rat pups. J. Appl. Physiol. 97, 2020–2025. doi: 10.1152/japplphysiol.00876.2003
Peter, J. H., Gassel, W., Mayer, J., Herrer-Mayer, B., Penzel, T., Schneider, H., et al. (1989). Effects of cilazapril on hypertension, sleep, and apnea. Am. J. Med. 87, 72S–78S.
Pialoux, V., Foster, G. E., Ahmed, S. B., Beaudin, A. E., Hanly, P. J., and Poulin, M. J. (2011). Losartan abolishes oxidative stress induced by intermittent hypoxia in humans. J. Physiol. 589, 5529–5537. doi: 10.1113/jphysiol.2011.218156
Potter, E. K., and McCloskey, D. I. (1979). Respiratory stimulation by angiotensin II. Respir. Physiol. 36, 367–373. doi: 10.1016/0034-5687(79)90048-3
Prabhakar, N. R. (2001). Oxygen sensing during intermittent hypoxia: cellular and molecular mechanisms. J. Appl. Physiol. 90, 1986–1994.
Prabhakar, N. R., Fields, R. D., Baker, T., and Fletcher, E. C. (2001). Intermittent hypoxia: cell to system. Am. J. Physiol. Lung Cell. Mol. Physiol. 281, L524–L528.
Prabhakar, N. R., and Kumar, G. K. (2004). Oxidative stress in the systemic and cellular responses to intermittent hypoxia. Biol. Chem. 385, 217–221. doi: 10.1515/BC.2004.015
Prabhakar, N. R., and Peng, Y. J. (2004). Peripheral chemoreceptors in health and disease. J. Appl. Physiol. 96, 359–366. doi: 10.1152/japplphysiol.00809.2003
Prabhakar, N. R., Peng, Y. J., Jacono, F. J., Kumar, G. K., and Dick, T. E. (2005). Cardiovascular alterations by chronic intermittent hypoxia: importance of carotid body chemoreflexes. Clin. Exp. Pharmacol. Physiol. 32, 447–449. doi: 10.1111/j.1440-1681.2005.04209.x
Rajagopalan, S., Kurz, S., Munzel, T., Tarpey, M., Freeman, B. A., Griendling, K. K., et al. (1996). Angiotensin II-mediated hypertension in the rat increases vascular superoxide production via membrane NADH/NADPH oxidase activation. Contribution to alterations of vasomotor tone. J. Clin. Invest. 97, 1916–1923. doi: 10.1172/JCI118623
Reid, I. A., Morris, B. J., and Ganong, W. F. (1978). The renin-angiotensin system. Annu. Rev. Physiol. 40, 377–410. doi: 10.1146/annurev.ph.40.030178.002113
Rey, S., Del Rio, R., Alcayaga, J., and Iturriaga, R. (2004). Chronic intermittent hypoxia enhances cat chemosensory and ventilatory responses to hypoxia. J. Physiol. 560, 577–586. doi: 10.1113/jphysiol.2004.072033
Schmitz, U., and Berk, B. C. (1997). Angiotensin II signal transduction: stimulation of multiple mitogen-activated protein kinase pathways. Trends Endocrinol. Metab. 8, 261–266. doi: 10.1016/S1043-2760(97)00101-X
Schultz, H. D. (2011). Angiotensin and carotid body chemoreception in heart failure. Curr. Opin. Pharmacol. 11, 144–149. doi: 10.1016/j.coph.2010.12.004
Shimizu, A., Yamagata, T., Tatsuno, H., Esato, M., Ueyama, T., Hayano, T., et al. (1998). [Radiofrequency catheter ablation therapy in elderly patients with supraventricular tachycardia]. Nippon Ronen Igakkai Zasshi 35, 451–457. doi: 10.3143/geriatrics.35.451
Takahashi, S., Nakamura, Y., Nishijima, T., Sakurai, S., and Inoue, H. (2005). Essential roles of angiotensin II in vascular endothelial growth factor expression in sleep apnea syndrome. Respir. Med. 99, 1125–1131. doi: 10.1016/j.rmed.2005.02.027
Youngson, C., Nurse, C., Yeger, H., Curnutte, J. T., Vollmer, C., Wong, V., et al. (1997). Immunocytochemical localization on O2-sensing protein (NADPH oxidase) in chemoreceptor cells. Microsc. Res. Tech. 37, 101–106.
Keywords: angiotensin II, AT1 receptor, carotid body, intermittent hypoxia, OSA
Citation: Fung ML (2014) The role of local renin-angiotensin system in arterial chemoreceptors in sleep-breathing disorders. Front. Physiol. 5:336. doi: 10.3389/fphys.2014.00336
Received: 27 June 2014; Accepted: 15 August 2014;
Published online: 05 September 2014.
Edited by:
Rodrigo Iturriaga, Pontificia Universidad Católica Chile, ChileReviewed by:
Olaf Grisk, University of Greifswald, GermanyRodrigo Del Rio, Universidad Autonoma de Chile, Chile
Copyright © 2014 Fung. This is an open-access article distributed under the terms of the Creative Commons Attribution License (CC BY). The use, distribution or reproduction in other forums is permitted, provided the original author(s) or licensor are credited and that the original publication in this journal is cited, in accordance with accepted academic practice. No use, distribution or reproduction is permitted which does not comply with these terms.
*Correspondence: Man Lung Fung, Department of Physiology, University of Hong Kong, 21 Sassoon Road, Pokfulam, Hong Kong e-mail:ZnVuZ21sQGhrdS5oaw==