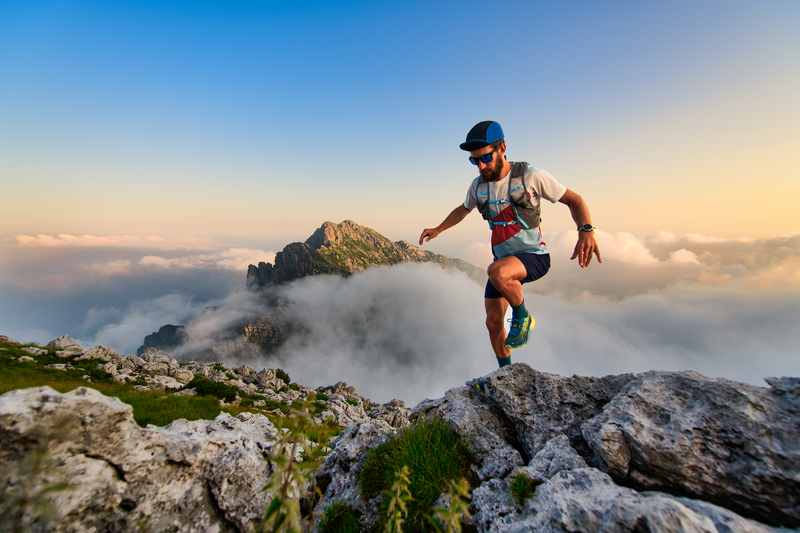
95% of researchers rate our articles as excellent or good
Learn more about the work of our research integrity team to safeguard the quality of each article we publish.
Find out more
REVIEW article
Front. Physiol. , 02 July 2014
Sec. Integrative Physiology
Volume 5 - 2014 | https://doi.org/10.3389/fphys.2014.00244
This article is part of the Research Topic Genome-wide view on the physiology of vitamin D View all 16 articles
Changes in vitamin D serum levels have been associated with inflammatory diseases, such as inflammatory bowel disease (IBD), rheumatoid arthritis, systemic lupus erythematosus, multiple sclerosis (MS), atherosclerosis, or asthma. Genome- and transcriptome-wide studies indicate that vitamin D signaling modulates many inflammatory responses on several levels. This includes (i) the regulation of the expression of genes which generate pro-inflammatory mediators, such as cyclooxygenases or 5-lipoxygenase, (ii) the interference with transcription factors, such as NF-κB, which regulate the expression of inflammatory genes and (iii) the activation of signaling cascades, such as MAP kinases which mediate inflammatory responses. Vitamin D targets various tissues and cell types, a number of which belong to the immune system, such as monocytes/macrophages, dendritic cells (DCs) as well as B- and T cells, leading to individual responses of each cell type. One hallmark of these specific vitamin D effects is the cell-type specific regulation of genes involved in the regulation of inflammatory processes and the interplay between vitamin D signaling and other signaling cascades involved in inflammation. An important task in the near future will be the elucidation of the regulatory mechanisms that are involved in the regulation of inflammatory responses by vitamin D on the molecular level by the use of techniques such as chromatin immunoprecipitation (ChIP), ChIP-seq, and FAIRE-seq.
It is now well established that the physiological importance of the vitamin D status extends far beyond the regulation of bone metabolism. According to its manifold functions in immune homeostasis, increasing evidence relates serum vitamin D levels as well as polymorphisms in enzymes involved in vitamin D metabolism to the incidence of chronic inflammatory diseases like asthma, atherosclerosis and autoimmune diseases (Stojanovic et al., 2011; Summerday et al., 2012; Szekely and Pataki, 2012). However, whether vitamin D exerts a salutatory or deteriorating role in such diseases is still under debate. This review will focus on the knowledge regarding the role of vitamin D in inflammatory diseases by the examples of asthma, atherosclerosis and autoimmune diseases.
According to the World Health Organization (WHO), asthma is the most common chronic disease among children (http://www.who.int/mediacentre/factsheets/fs307/en/index.html). In this context, several studies addressed the interrelationship of the maternal as well as infant vitamin D status and the prevalence and severity of asthma. Three studies by Brehm et al. analyzed the relationship between vitamin D levels and asthma severity in Costa Rican, North American and Puerto Rican children, respectively (Brehm et al., 2009, 2010, 2012). Collectively, they found high prevalences of vitamin D insufficiency in asthmatic children and vitamin D insufficiency was correlated with severe asthma exacerbations. However, the prevalence of vitamin D insufficiency was high in Puerto Rican children irrespective of the indisposition from asthma, with roughly comparable percentages between asthma patients and otherwise healthy children (Brehm et al., 2012). Although few studies showed no correlation between serum vitamin D levels and the presence of asthma (Menon et al., 2012; Gergen et al., 2013), many studies state a higher prevalence of vitamin D deficiency in asthmatic children (Freishtat et al., 2010; Chinellato et al., 2011a,b; Ehlayel et al., 2011; Hollams et al., 2011; Bener et al., 2012; Krobtrakulchai et al., 2013) and adults (Li et al., 2011b). Additionally, in many cases a relation between low vitamin D levels and reduced asthma control is found. Furthermore, metabolomic analysis of breath condensates revealed reduced levels of vitamin D metabolites in children with asthma (Carraro et al., 2013). Similarly, enhanced vitamin D binding protein levels were found in bronchoalveolar lavage fluid of asthmatic children (Gupta et al., 2012b). Interestingly, one study describes an age-dependent association between serum vitamin D level and asthma prevalence in children (Van Oeffelen et al., 2011).
A different relationship between the vitamin D status and asthma has been brought up by a northern Finland birth cohort study, which revealed an increased risk of asthma in adults who received high dose vitamin D supplementation in their childhood (Hypponen et al., 2004). In accordance with these findings, a prospective study by Tolppanen et al. revealed an increased risk of wheezing in association with higher vitamin D levels, but no correlation of lower vitamin D levels to respiratory sicknesses (Tolppanen et al., 2013). Another study reinforces the finding of increased susceptibility to asthma after vitamin D supplementation, yet only regarding supplementation of water soluble formulations and not in connection with vitamin D supplementation in peanut oil (Kull et al., 2006).
There is debate as to whether maternal vitamin D levels during the pregnancy influence the susceptibility to asthma of the progeny. Whereas some reports showed no correlation between maternal or cord blood vitamin D levels and an increased risk of childhood asthma (Camargo et al., 2011; Rothers et al., 2011; Morales et al., 2012; Pike et al., 2012; Magnus et al., 2013), another report indicates that high maternal vitamin D levels correlate with enhanced probability of asthma development in children (Gale et al., 2008). In contrast, some reports associate higher vitamin D intake during pregnancy with reduced risk of childhood wheezing and asthma (Camargo et al., 2007; Devereux et al., 2007; Erkkola et al., 2009).
Mechanistically, vitamin D induced protection against airway inflammation has been related to a modulated T cell response to allergens as well as induction of the immunoglobulin-like anti-inflammatory cell surface protein CD200 on T cells, that acts on target immune cells which express the CD200 receptor (CD200R) (Dimeloe et al., 2012; Gorman et al., 2012; Urry et al., 2012). Many authors suggest that the beneficial effect of sufficient vitamin D levels on asthma development results from the immune enhancing effect of vitamin D and the simultaneous prevention of respiratory infections (Ginde et al., 2009; Camargo et al., 2011; Majak et al., 2011; Morales et al., 2012).
Furthermore, there is evidence that the serum vitamin D level has also an influence on asthma therapy, as vitamin D has been demonstrated to enhance glucocorticoid (GC) action and lower serum vitamin D levels are associated with higher corticosteroid requirement, at least in children, or even therapy-resistance (Searing et al., 2010; Goleva et al., 2012; Gupta et al., 2012a; Wu et al., 2012). Additionally, the therapeutic effect of specific allergen immunotherapy has been correlated to serum vitamin D levels (Majak et al., 2012).
Besides serum vitamin D levels also polymorphisms of genes of the vitamin D pathway such as the vitamin D receptor (VDR) have been associated with asthma (Poon et al., 2004; Raby et al., 2004; Saadi et al., 2009; Li et al., 2011a; Pillai et al., 2011; Maalmi et al., 2013), yet, not all studies revealed a correlation between vitamin D pathway polymorphisms and asthma prevalence (Vollmert et al., 2004; Fang et al., 2009).
Another chronic inflammatory disease that is more prevalent in the elderly population is atherosclerosis. Early studies on atherosclerosis development in several animal models revealed an accelerating effect of high doses of vitamin D. Vascular calcification was observed in some of these studies, but not all (Zemplenyi and Mrhova, 1965; Kudejko, 1968; Taura et al., 1979; Kunitomo et al., 1981; Toda et al., 1983, 1985). Moreover, 1α,25-dihydroxyvitamin D3, the active form of vitamin D, stimulated vascular calcification by in vitro by reducing the expression of parathyroid hormone-related peptide as well as stimulating alkaline phosphatase activity in bovine vascular smooth muscle cells (Jono et al., 1998). On the other hand, there is a large body of research from clinical studies in humans indicating that low levels of serum 25-hydroxy vitamin D are associated with atherosclerosis (Reis et al., 2009; Carrelli et al., 2011; Shanker et al., 2011; Cheraghi et al., 2012). In line with this, the incidence of osteoporosis, a disease known to be related to vitamin D inadequacy, correlates with the incidence of atherosclerosis (Stojanovic et al., 2011). Therefore, different mechanisms may account for the promotion of atherogenesis by high and low vitamin D levels, respectively, and calcification may be crucial in the case of hypervitaminosis. Moreover, differences between the animal and human system may account for the conflicting results.
With respect to atherogenesis, 1α,25-dihydroxyvitamin D3 has been demonstrated to reduce macrophage adhesion and migration as well as foam cell formation in monocytes isolated from type 2 diabetic patients (Oh et al., 2012; Riek et al., 2013a,b). Mechanistic investigations in the context of these studies attributed the beneficial effects of vitamin D to a reduction of endoplasmatic reticulum stress in macrophages. This has been investigated in two mouse models, where vitamin D deficiency facilitated atherosclerosis, which could be reversed in the course of macrophage endoplasmatic reticulum stress suppression (Weng et al., 2013). Further evidence on beneficial effects of calcitriol treatment on atherosclerosis development has been obtained from an investigation with apolipoprotein E knock-out mice. In this study, oral calcitriol treatment decreased the production of proinflammatory chemokines, led to a reduced amount of inflammatory effector cells in atherosclerotic plaques and simultaneously increased amounts of regulatory T cells (Takeda et al., 2010). A similar link between vitamin D, T cell modulation, and atherosclerosis has also been established in humans with chronic kidney disease (CKD) (Yadav et al., 2012).
The renin-angiotensin-system is known for its detrimental effects on the cardiovascular system and has been shown to play an important role in the development of atherosclerosis. Interestingly, numerous studies in mice document that vitamin D signaling suppresses the renin-angiotensin-system and that vitamin D deficiency is associated with an increased activity of the renin-angiotensin-system (Li et al., 2002; Zhou et al., 2008; Szeto et al., 2012; Weng et al., 2013). Moreover, the inverse associations which are described for vitamin D and the occurrence of inflammatory cytokines, C-reactive protein, and adhesion molecules suggest a inhibitory role for vitamin D in the genesis of atherosclerosis (Brewer et al., 2011). Additionally, there is experimental evidence that vitamin D reduces the expression of matrix metalloproteinases that are involved in vascular calcification (Nakagawa et al., 2005; Qin et al., 2006).
However, there are also studies that found no evidence for an association between low vitamin D and atherosclerosis in patients suffering from different autoimmune diseases (Mok et al., 2012; Sachs et al., 2013). Similarly, there was no evidence for an association of BsmI polymorphism, an intronic single nucleotide variation of the VDR gene, with atherosclerosis (El-Shehaby et al., 2013). Yet, it has been shown that atherosclerosis in monkeys is associated with low levels of VDR expression in coronary arteries even in the presence of higher plasma vitamin D concentrations (Schnatz et al., 2012a,b). Moreover, the activation of vitamin D can occur locally in macrophages that infiltrate atherosclerotic lesions and local vitamin D response might thus not necessarily correlate with serum vitamin D levels (Richart et al., 2007).
It is well established that vitamin D plays an important role in the regulation of immune functions (Schwalfenberg, 2011; Zhang et al., 2013a). Accordingly, several inflammatory autoimmune diseases like rheumatic disorders and type 1 diabetes have been associated with vitamin D deficiency (Adorini and Penna, 2008; Shapira et al., 2010). Inflammatory processes in the central nervous system are a hallmark of the autoimmune disease multiple sclerosis (MS) (Deckx et al., 2013). Several studies indicate that MS patients have lower levels of vitamin D and that higher vitamin D levels as well as vitamin D supplementation have a protective effect against MS (Munger et al., 2004, 2006; Ozgocmen et al., 2005). Moreover, vitamin D levels have been shown to vary in concordance with MS exacerbations (Correale et al., 2009) and it is possible that low vitamin D levels are rather a consequence of the sun avoidance of MS patients and not a direct cause of the disease (Munger et al., 2006). Regarding the effectiveness of vitamin D supplementation in the course of MS treatment, there are studies in mice and humans that suggest a beneficial effect of treatment (Goldberg et al., 1986; Wingerchuk et al., 2005; Pedersen et al., 2007; Burton et al., 2010). Interestingly, a gender specific effect of vitamin D has been demonstrated in mice and humans, which points to greater effects of vitamin D in females (Spach and Hayes, 2005; Correale et al., 2010).
Overall, there have been only a few controlled trials documenting the outcome of vitamin D supplementation on disease activity in rheumatic conditions, and the role of vitamin D in rheumatoid arthritis is therefore controversially discussed (Gatenby et al., 2013). Yet, a metaanalysis of observational studies on the vitamin D intake and vitamin D serum levels suggests an inverse association with rheumatoid arthritis (Song et al., 2012). Additionally, in vitro experiments with macrophages from healthy donors and rheumatoid arthritis patients indicate an enhanced anti-inflammatory potential of vitamin D in macrophages from the latter group (Neve et al., 2013).
It has been shown that the onset of autoimmunity in type 1 diabetes is preceded by a proinflammatory metabolic serum profile (Knip and Simell, 2012). Concurrently, a study in Italian children revealed reduced vitamin D serum levels in children at the onset of type 1 diabetes compared to children hospitalized for other reasons (Franchi et al., 2013). In conformity with these findings, metaanalyses suggest an association between vitamin D intake in early life and susceptibility for type 1 diabetes (Zipitis and Akobeng, 2008; Dong et al., 2013).
For inflammatory bowel disease (IBD), another autoimmune disorder, similar associations to that described above regarding vitamin D status and sunlight exposure have been reported (Garg et al., 2012; Ananthakrishnan, 2013). Animal studies in vitamin D deficient and VDR knockout (KO) mice reveal a dysregulation of T cells that might be of importance in the pathogenesis of IBD (Ooi et al., 2012).
In summary, there is considerable evidence for an association between vitamin D deficiency and inflammatory diseases. However, regarding the causality of this association and the benefit of vitamin D supplementation, only limited information is available and the existing data are still inconsistent.
Cell type specific up-regulation of proinflammatory genes and down-regulation of anti-inflammatory genes is a hallmark of the onset of an inflammatory reaction. Depending on the cell type, up-regulation of certain cytokines or enzymes which generate mediators of inflammation can occur at the transcriptional or posttranscriptional level. In addition, there is considerable crosstalk between various pathways which allows adaptation of the host defense reactions to the environment. According to their functions, the regulators of inflammatory reactions can be receptors such as toll like receptors, signal transducers as well as transcription factors which translate the activation of certain signal cascades into gene transcription. Additionally, regulation of gene expression during inflammatory processes can also occur on posttranslational level which is not focus of this review.
At the level of intracellular signal transduction, MAP kinases such as JNK or p38 have been identified as central signal transducers of inflammatory signals. Interestingly, it has been observed that there is a cross talk between VDR/RXR and MAP kinase signaling on many levels and the outcome, e.g., stimulation or inhibition, depends on the stimulus, cell type and the response (Miodovnik et al., 2012). Regarding inflammation, it has become obvious that vitamin D inhibits production of proinflammatory cytokines like IL-6 or TNFα in monocytes via the inhibition of p38 MAP kinase (Zhang et al., 2012). Inhibition of p38 in monocytes was found to be due to induction of MAPK phosphatase-1 (MKP1) which dephosphorylates p38 and thus reduces p38 activation (Figure 1). A similar mechanism was found in prostate cells where induction of MKP5 by 1α,25(OH)2D3 was responsible for down-regulation of IL-6 mRNA expression (Nonn et al., 2006). 1α,25(OH)2D3 increases MKP5 transcription by induction of VDR/RXR binding to a VDRE in the MKP5 promoter. Beside this indirect modulation of signaling cascades, 1α,25(OH)2D3 and its receptor complex VDR/RXR can interact with other transcription factors such as NF-κB, nuclear factor of activated T-cells (NFAT), or the glucocorticoid receptor (GCR) which leads to anti-inflammatory effects (Figure 2). Activation of VDR inhibits NF-κB activation and signaling. NFκB is a ubiquitously expressed transcription factor which represents a heterodimer. In the inactive state it interacts with IκB which keeps it in the cytosol (Karin and Lin, 2002). Upon cell activation by proinflammatory stimuli, IκB is phosphorylated and subsequently ubiquitinylated, which leads to proteasomal degradation of the IκB protein. Free NFκB translocates to the nucleus where it activates transcription of proinflammatory cytokines, antiapoptotic factors as well as of enzymes involved in the generation of proinflammatory mediators such as COX-2 (Karin and Lin, 2002; Tsatsanis et al., 2006). It has been shown that 1α,25(OH)2D3 down-regulates NF-κB levels in lymphocytes (Yu et al., 1995) and that the vitamin D analog TX 527 prevents NF-κB activation in monocytes (Stio et al., 2007). Inhibition of NFκB activation by 1α,25(OH)2D3-mediated up-regulation of IκB expression was reported in human peritoneal macrophages (Cohen-Lahav et al., 2006) (Figure 2). Additionally, interference of vitamin D signaling with DNA binding of NFκB was found (Harant et al., 1998). It was shown that 1α,25(OH)2D3 inhibits NF-κB activity in human MRC-5 fibroblasts but not translocation of its subunits p50 and p65. The partial inhibition of NFκB DNA binding by 1α,25(OH)2D3 was dependent on de novo protein synthesis, suggesting that 1α,25(OH)2D3 may regulate expression of cellular factors which contribute to reduced DNA binding of NFκB (Harant et al., 1998). Thus, it seems that vitamin D is able to inhibit NFκB activation as well DNA binding (Figure 2).
Figure 1. Inhibition of the p38 MAP kinase pathway by 1α,25(OH)2D3 and a mechanism for the synergistic anti-inflammatory effects of 1α,25(OH)2D3 and glucocorticoids. Proinflammatory stimuli lead to p38 MAP kinase phosphorylation and activation which subsequently induces expression of many proinflammatory proteins such as IL-6 and TNFα. 1α,25(OH)2D3 induces MKP1 expression which dephosphorylates and inactivates p38 MAP kinase. 1α,25(OH)2D3 stimulates glucocorticoid-induced MKP1 expression via enhanced expression of Med14.
Figure 2. SMAD, NFAT and NFκB signaling and modulation of these signaling pathways by 1α,25(OH)2D3, respective VDR/RXR. IκB phosphorylation after various cell stress signals leads to its ubiquitinylation and subsequent proteosomal degradation. After IκB degradation, NFκB is released and translocates into the nucleus where it binds to DNA and modulates gene expression. Activation of NFAT is mediated by the protein phosphatase calcineurin which dephosphorylates NFAT. After dephosphorylation, NFAT translocates into the nucleus, interacts with a variety of other transcription factors and modulates gene expression. Activation of TGFβ receptors leads to phosphorylation of SMAD2 and SMAD3 as well as subsequent translocation into the nucleus. SMAD3 forms a complex with SMAD4 and modulates gene expression of its target genes. After activation by 1α,25(OH)2D3 the VDR/RXR heterodimer can inhibit NFκB signaling either by induction of IκB or by interference with NFκB DNA binding. Also, inhibition of NFAT signaling was reported by prevention of NFAT binding to its response elements.
Another interesting target for the anti-inflammatory signaling of vitamin D is NFAT (Figure 2). This transcription factor is activated by dephosphorylation by calcineurin which leads to translocation of this protein and transcriptional activation of proinflammatory genes such as interleukin 2 and cyclooxygenase-2 (Duque et al., 2005; Muller and Rao, 2010). In T-lymphocytes, it was shown for the interleukin 2 promoter that VDR-RXR heterodimers bind to an NFAT binding site and thus inhibit NFAT activity (Takeuchi et al., 1998). Similar data were obtained for interleukin 17 where 1α,25(OH)2D3 blocked NFAT activity which contributed to repression of interleukin17A expression in inflammatory CD4+ T cells by the hormone (Joshi et al., 2011).
Another interesting finding was that vitamin D enhances the anti-inflammatory activities of GCs (Figure 1). The strong anti-inflammatory activities of GCs are mediated by the GCR. It belongs to the nuclear receptor family. Upon ligand binding the receptor dimerizes and translocates into the nucleus where it binds to GC-responsive elements (GRE) and modulates gene expression (Barnes, 1998). In general, GCs down-regulate expression of pro-inflammatory genes and up-regulate anti-inflammatory genes. It was found in asthmatics that dexamethasone-induced MKP-1 expression as a marker for GC responsiveness is significantly increased when serum vitamin D levels increase suggesting that vitamin D may enhance GC responsiveness (Sutherland et al., 2010). It is interesting to note that MKP-1 is also a vitamin D target gene as mentioned above (Zhang et al., 2012). Vitamin D enhancement of GC-induction of MKP1 was abolished both in purified CD14+ and CD14− cells and it was found that the synergism depends on vitamin D-induced GM-CSF release from CD14− cells and GM-CSF-dependent MED14 induction in CD14+ cells (Zhang et al., 2013b). MED14 is part of the mediator complex involved in the regulation of transcriptional initiation and it was found to form a complex with VDR and mediate ligand-dependent enhancement of transcription by the VDR (Rachez et al., 1999) (Figure 1). Interestingly, MED14 also enhances gene activation by the GCR in a gene-specific manner (Chen et al., 2006). For MKP1 it was found in human monocytes that VDR and GCR bind to a corresponding VDRE and two GREs after ligand stimulation (Figure 1). After GM-CSF treatment, MED14 was recruited to the promoter after addition of 1α,25(OH)2D3 but not dexamethasone indicating that MED14 recruitment depends on the VDR (Zhang et al., 2013b). 1α,25(OH)2D3 enhanced the binding of the GCR to the GRE in close proximity to the VDRE in the presence of GM-CSF and ChIP analysis suggest a MED14-VDR-GCR complex at the MKP1 promoter with bridges the crosstalk between vitamin D and GCs (Zhang et al., 2013b). The data from single gene analyses such as MKP1 suggest that the VDR interacts with other signaling pathways.
At present there are genome-wide data available from immortalized lymphoblastoid cell lines (Ramagopalan et al., 2010), undifferentiated and LPS stimulated THP-1 cells (Heikkinen et al., 2011; Tuoresmäki et al., 2014), LS180 colorectal cancer cells (Meyer et al., 2012) and LX2 hepatic stellate cells (Ding et al., 2013). These six ChIP-seq data sets showed 21,776 non-overlapping VDR binding sites whereas only 54 sites were common in all six data sets. The data suggest that, apart from a few sites, VDR binding is strongly cell and stimulus specific. In the non-overlapping binding sites, only 17.5% contain a DR3-type VDRE whereas the percentage of DR3-type response elements is enriched in highly ligand-responsive loci. All these data suggest that the VDR interacts with other transcription factors and that these interactions might only be in part ligand dependent. Regarding inflammation, the genome-wide effects of LPS on VDR location in THP-1 cells are of special interest (Tuoresmäki et al., 2014). From the 805 VDR binding sites, only 462 overlap in untreated and LPS-treated THP cells which were stimulated with 1α,25(OH)2D3. Thus, LPS treatment leads to a considerable change in VDR location. In THP-1 cells, bioinformatic searches for shared binding sites revealed motifs for CEBP1, PU.1 in stimulated THP-1 cells whereas NFYA, LHX3-like and NANOG were found for unstimulated cells but no transcription factor has been identified in conjunction with LPS treatment. Of note, binding sites for JUN, a component of the AP1 transcription factor, were found to be enriched at VDR loci in LX2 hepatic stellate cells. This is of interest regarding inflammation as AP1 is known to be a transcription factor that regulates expression of many proinflammatory genes. At present, there are many data available on single gene levels but there is still a missing link between these data and the genome-wide observations. Since VDR signaling seems to be strongly cell type and stimulus-dependent, more genome-wide data with different cell types and stimuli are required to understand the mechanisms how 1α,25(OH)2D3 modulates gene expression under inflammatory conditions.
Arachidonic acid derived eicosanoids which comprise prostaglandins and leukotrienes play an important role in inflammatory processes (Harizi et al., 2008). Of the enzymes involved in prostaglandin synthesis, cyclooxygenase-2 (COX-2) and microsomal prostaglandin E synthase 1 (mPGES-1) have been shown to be induced in many inflammatory conditions (Tomasoni et al., 1998; Murakami et al., 2000; Cipollone and Fazia, 2006; Petrovic et al., 2006) and inhibition of both enzymes is a common approach in the treatment of inflammatory diseases (Fahmi, 2004; Ramalho et al., 2009; Dallaporta et al., 2010).
In prostate cancer cells it has been demonstrated that 1α,25(OH)2D3 inhibits the expression of COX-2 on mRNA and protein level as well as the expression of prostaglandin receptors on mRNA level and simultaneously upregulates prostaglandin catabolism via 15-hydroxyprostaglandin dehydrogenase (Moreno et al., 2005). In addition, the combination of calcitriol with COX-inhibitors led to synergistic growth inhibition (Moreno et al., 2005). Similar results were obtained with the combination of 1α,25(OH)2D3 and COX-inhibitors in different leukemia cells (Jamshidi et al., 2008). In accordance with the previous findings, treatment with the vitamin D analog elocalcitol resulted in decreased COX-2 expression and diminished PGE2 synthesis in prostate cells (Penna et al., 2009). The COX-2/PGE2-pathway was also identified as the mediator of the growth inhibitory effect of calcitriol in breast cancer cells (Yuan et al., 2012). Furthermore, COX-2 upregulation in placental trophoblasts in response to oxidative stress and in myometrial cells in response to interleukin-1β was inhibited by 1α,25(OH)2D3 (Sun et al., 2013; Thota et al., 2013).
Thill et al. found correlations between VDR expression and expression of COX-2 as well as 15-hydroxy PG dehydrogenase in malignant breast cells and in cells from female reproductive tissues (Thill et al., 2009, 2010, 2012).
In human lung fibroblasts inhibition of PGE2-production by vitamin D was found which was not due to altered COX-expression. Yet, vitamin D inhibited IL-1β-induced mPGES-1 expression and simultaneously stimulated 15-hydroxy PG dehydrogenase (Liu et al., 2014).
5-lipoxygenase (5-LO) accounts for the first two steps in leukotriene biosynthesis. Leukotrienes exert potent proinflammatory actions and have been associated with several chronic inflammatory diseases (Haeggstrom and Funk, 2011).
In the myeloid cell line HL-60, treatment with 1α,25(OH)2D3 triggers differentiation into monocytic cells. Simultaneously, 1α,25(OH)2D3 has been shown to induce 5-LO expression on mRNA and protein level as well as to increase 5-LO enzyme activity (Bennett et al., 1993; Brungs et al., 1994). A similar effect was also observed in the monocytic cell line Mono Mac 6. Additionally, this effect was strongly enhanced by the combination of 1α,25(OH)2D3 with transforming growth factor β (TGF-β) (Brungs et al., 1995; Harle et al., 1998). Mechanistically, the effect of 1α,25(OH)2D3 on 5-LO expression was related to VDR binding sites in the 5-LO promoter and distal parts of the 5-LO gene (Sorg et al., 2006; Stoffers et al., 2010) and is due to stimulation of 5-LO transcript elongation (Stoffers et al., 2010).
Previous results suggest a modulatory role of vitamin D in the inflammatory response of cells of the monocyte/macrophage lineage, which is again modulated by TGF-β. In this context, it is interesting that macrophages contain 1α-hydroxylase and therefore are capable of autocrine or paracrine activation of vitamin D (Lagishetty et al., 2011). Moreover, in keratinocytes autocrine TGF-β production is induced by vitamin D (Kim et al., 1992). Crucial participation of monocytes/macrophages in diverse inflammatory processes has been demonstrated (Cutolo, 1999; Yoon and Jun, 1999; Moore et al., 2013). Besides induction of 5-lipoxygenase, the combination of TGF-β and 1α,25(OH)2D3 has been shown to induce the differentiation antigen CD69 in monocytic cells (Wobke et al., 2013). Overexpression of CD69 again, has been shown in the context of local dermal inflammation, systemic lupus erythematosus, hyperthyroid Graves' disease and autoimmune thyroiditis (Fernandez-Herrera et al., 1996; Portales-Perez et al., 1997; Crispin et al., 1998; Gessl and Waldhausl, 1998).
TGF-β is a pleiotropic cytokine with a broad range of biologic effects, which is involved in the regulation of inflammatory processes on several levels. A main mechanism in this respect is the maintenance of T cell tolerance to self or innocuous antigens (Li and Flavell, 2008). In cancer-associated inflammation, TGF-β suppresses the anti-tumor activity of diverse immune cells, including T-cells, natural killer (NK) cells, neutrophils, monocytes and macrophages (Bierie and Moses, 2010). A great number of studies focused on the role of TGF-β in fibrosis and associated inflammation. In these diseases, TGF-β regulates influx and activation of immune cells, as well as the actual fibrotic process, and thus the delicate balance between an appropriate inflammatory response and the development of pathologic fibrosis (Flanders, 2004; Sheppard, 2006; Lan, 2011). Several mechanistic links between inflammation and fibrosis are known, but the complete picture remains to be established (Lee and Kalluri, 2010). TGF-β signaling in these processes has been attributed both to canonical TGF-β signaling via the Smad proteins (signal-dependent transcription factors) as well as non-Smad signaling pathways (e.g., via MAPK pathways) (Figure 2).
Independent of inflammatory model systems, 1α,25(OH)2D3 and TGF-β/Smad signaling pathways have been found to be interrelated through three mechanisms: (i) the existence of a common regulator protein, the oncoprotein Ski, which can repress both pathways (Ueki and Hayman, 2003), (ii) the possibility of joint gene regulation via VDR and Smad recognition elements that are located in close proximity to a target promoter (Subramaniam et al., 2001) (Figure 2) or (ii) direct interaction of Smad3 and vitamin D signaling, whereby Smad acts as a coregulator specific for ligand-induced VDR transactivation (Yanagisawa et al., 1999).
The influence of vitamin D on inflammation-related signaling via TGF-β and Smad has mainly been investigated in models of fibrosis, and distinct mechanisms have been elucidated. Activation of 1α,25(OH)2D3 signaling by the natural ligand itself or its synthetic analogs reduces TGF-β expression (Kim et al., 2013) and interferes with the downstream signaling. The latter occurs via several mechanisms: downregulation of phosphorylated activatory Smads (Smad2/3 and 4) accompanied by upregulation of inhibitory Smad6 (Kim et al., 2013) (Figure 2); an inhibitory interaction between 1α,25(OH)2D3-bound VDR and Smad3 (Ito et al., 2013) or inhibition of Smad2 phosphorylation and nuclear translocation of Smad2/3, coincident with inhibited protein expression from TGF-β target genes (Halder et al., 2011). Similar findings have been made in studies with nephropathy models where suppression of TGF-β and p-Smad2/3 expression (Xiao et al., 2009) or a decrease in Smad2 and an increase in inhibitory Smad7 (Hullett et al., 2005) have been detected. In a large-scale study using hepatic stellate cells, TGF-β has been shown to cause chromatin remodeling events that led to a redistribution of genome-wide VDR binding sites (the VDR cistrome) with a shift toward VDR binding to Smad3-dependent, profibrotic target genes. In this study, VDR ligands led to a reduced Smad3 occupancy at these genes and thus antifibrotic effects (Ding et al., 2013). Although hepatic stellate cells do not belong to the immune system, and the interplay between VDR and TGF-β/Smad signaling may be dependent on the cell type, key aspects of this elaborate study deserve mention. More than 104 genomic sites were found to be co-occupied by both VDR and SMAD3 in these cells, and an analysis of the spatial relationships between the two transcription factors revealed that the respective response elements were located within a range of 200 base pairs (one nucleosomal window). Mechanistically, TGF-β signaling seems to deplete nucleosomes from the co-occupied sites and thus allow access of VDR to these sites. Vitamin D signaling on the other hand seems to limit TGF-β activation by inhibited coactivator recruitment. Spatiotemporal analysis revealed that 1α,25(OH)2D3/TGF-β-induced VDR and SMAD3 binding to the co-occupied sites were inversely correlated. The maximum of SMAD3 binding occurred 1 h after treatment and was reduced by 70% after 4 h, when VDR binding was maximal. Therefore, TGF-β signaling seems to change the chromatin architecture in a way in which liganded VDR can reverse Smad activation.
The finding that 1α,25(OH)2D3 interacts with the production of interleukins (Tsoukas et al., 1984) is of certain interest in the history of vitamin D research, as a crucial finding that expanded the view to roles beyond calcium homeostasis and crucially contributed to establish an immunoregulatory function of vitamin D (Tsoukas et al., 1984).
The interleukins are a large group of cytokines of central importance for the intercellular communication between the different cells generally involved in inflammatory responses. These cells mainly encompass the leukocytes in their various stages of differentiation (distinct T-cells subsets, monocytes, macrophages, dendritic cells (DCs), granulocytes and B-lymphocytes) and cells of the connective tissue and vasculature (fibroblasts, endothelial cells). Furthermore, in specific organ-related diseases with inflammatory components (psoriasis, CKD, placental infection/inflammation, obesity, and others), further cell types are involved, e.g., keratinocytes, endothelial cells, trophoblasts, and adipocytes. All of them are capable of synthesizing interleukins, and the influence of 1α,25(OH)2D3 on IL gene expression has been investigated. The influence of 1α,25(OH)2D3 on IL gene expression and signaling in the different cell types will be outlined in the following.
Several studies, especially the early ones, included ex vivo experiments with cellular samples from healthy individuals, mainly with PBMC (Rigby et al., 1984; Tsoukas et al., 1984; Saggese et al., 1989; Muller and Bendtzen, 1992), (partly) isolated T-cells (Bhalla et al., 1986), (partly) isolated monocytes (Bhalla et al., 1986; Muller et al., 1992; Zarrabeitia et al., 1992; Lemire et al., 1995; Lyakh et al., 2005), or cocultures of T-cells and monocytes (Tsoukas et al., 1989).
In stimulated PBMC, as a preparation that includes different cell types, 1α,25(OH)2D3 caused suppression of IL-2 production (Rigby et al., 1984; Tsoukas et al., 1984; Saggese et al., 1989) and reduced release of IL-1β, IL-6, and IL-10 (Joshi et al., 2011). Furthermore, the vitamin D analog paracalcitol led to reduced IL-8 production in stimulated PBMC (Eleftheriadis et al., 2010).
In more cell specific experiments with (partly) isolated T-cells, 1α,25(OH)2D3-mediated inhibition of IL-2 mRNA synthesis induced by lectin/phorbol ester (Matsui et al., 1986) or protein production induced by lectin (Bhalla et al., 1986), was confirmed. This was also observed for the two subsets of CD4+ and CD8+ T-cells (Jordan et al., 1989), which however displayed stimulus-dependency for IL-2 protein production. In a more detailed analysis, IL production by CD4+ and CD8+ cells was studied by flow cytometry on single cell level. In both populations, a decrease in IL-2 production was found. Conversely, regarding other IL class members analyzed in the same study, 1α,25(OH)2D3 increased the low percentage of IL-13-producing cells in both subsets and IL-6 producing CD4+ and CD8+ T-cells could only be detected after incubation with 1α,25(OH)2D3 (Willheim et al., 1999) (Figure 3).
Figure 3. The influence of 1α,25(OH)2D3 on the expression of interleukins, TNFα and IFNγ in monocytes, dendritic cells, and different T-cell subsets. Blue arrows indicate IL signaling between the different cell types and red arrows indicate differentiation processes. IL-12 and IL-23 expression is downregulated in monocytes and dendritic cells by 1α,25(OH)2D3. In contrast, IL-10 expression is enhanced. A shift from a Th1 profile toward the Th2 type and a decrease in Th17 responses is to be anticipated from these changes. Inhibition of T-cell autoregulation by 1α,25(OH)2D3-mediated suppression of IL-2 expression is not shown. Abbreviations and symbols: APC, antigen presenting cell; MΦ, macrophage; DC, dendritic cell; ↑, upregulation; ↓, downregulation; -, no changes.
The finding that IL-2 gene expression is reduced by 1α,25(OH)2D3 in T-cells has moreover been confirmed in two studies using the human T-cell line Jurkat, and the mechanisms have been studied. It has been found that the VDR seems to cause direct transcriptional repression of IL-2 gene expression via blockage of a positive regulatory element recognized by the transcription factor NFAT within the IL-2 promoter (Alroy et al., 1995). In a later study, the repression has been kinetically classified as a primary response to 1α,25(OH)2D3, and ligand-dependent VDR binding at the IL-2 gene locus was detected using ChIP assays (Matilainen et al., 2010b) (Figure 2). It has to be mentioned, however, that long term pretreatment of Jurkat cells with 1α,25(OH)2D3 before stimulation with mitogen and phorbol ester seems to enhance IL-2 mRNA expression (Prehn and Jordan, 1989). Studies using T-cells from other species confirmed the inhibitory effect of 1α,25(OH)2D3 on IL-2 production (Hodler et al., 1985).
Similar findings as for IL-2 have been made regarding the inhibition of IL-17 production by 1α,25(OH)2D3 from T-cells in a more recent report. It has been found that (i) the VDR competes for binding with NFAT and recruits histone deacetylase (HDAC) to the human IL-17 promoter, thus inhibiting its activation, (ii) binding of the activatory transcription factor Runx1 to the mouse IL-17A promoter was inhibited through sequestration of Runx1 by the VDR in the presence of 1α,25(OH)2D3 and (iii) 1α,25(OH)2D3 induced the IL-17 inhibiting transcription factor Foxp3 (Joshi et al., 2011). Other studies suggest a post-transcriptional mechanism of IL-17 inhibition by VDR via induction of the translation inhibitor C/EBP homologous protein (CHOP) (Chang et al., 2010).
Apart from studies with PBMC or T-cells from healthy individuals or experiments with cell lines, a few studies exist with cell samples from patients suffering from inflammatory diseases. In contrast to the findings with cells from healthy individuals after 1α,25(OH)2D3 treatment, PBMC isolated from hemodialysis patients responded to treatment with 1α(OH)D3 by enhanced IL-2 protein production, however, starting from a significantly lower level of IL-2 production compared to healthy controls (Tabata et al., 1988). The capacity of PBMC from Crohn's disease patients to produce IL-6 has been elevated by 1α,25(OH)2D3 treatment of the patients (Bendix-Struve et al., 2010). IL-6 and IL-8 production and mRNA expression have been found to be decreased by 1α,25(OH)2D3 in stimulated PBMC of psoriatic patients (Inoue et al., 1998). In PBMC from treatment-naive patients with early rheumatoid arthritis (RA), reduced IL-17A and increased IL-4 levels have been observed in the presence of 1α,25(OH)2D3. In the FACS-separated subpopulation of memory T-cells (CD45RO+), 1α,25(OH)2D3 suppressed IL-17A, IL-17F and IL-22 (Colin et al., 2010) (Figure 3).
In an early report, IL-1 production by human monocytes/macrophages enriched from PBMC has been found to be elevated by single 1α,25(OH)2D3 treatment (Bhalla et al., 1986). In subsequent studies with stimulated, monocyte-enriched cultures from PBMC, either no 1α,25(OH)2D3 effect has been detected (Zarrabeitia et al., 1992) or a reduction of IL-1 (and IL-6) production has been found, which seemed to be based on post-transcriptional events (Muller et al., 1992). The decrease in IL-1 production has been confirmed for co-cultures of T-cells and monocyte-enriched PBMC (Tsoukas et al., 1989). However, it has to be pointed out that different stimuli to elicit IL-1 production had been used in these studies. In human monocytic cell lines, (U937, HL-60 or THP-1), no induction (THP-1), or upregulation of IL-1β mRNA (U937, HL-60) by 1α,25(OH)2D3 has been detected, which varied with the presence or absence and the type of the co-stimulus that was used (phorbol ester, lipopolysaccharide) (Bhalla et al., 1991; Blifeld et al., 1991; Fagan et al., 1991). Further it is noteworthy that conflicting data exist for studies with U937 cells regarding the actual secretion of IL-1β protein (Blifeld et al., 1991; Fagan et al., 1991; Taimi et al., 1993). In THP-1 cells stimulated with agonists for Toll-like receptor 8, IL-1β mRNA was induced and could be suppressed by 1α,25(OH)2D3 (Li et al., 2013).
In a more recent study, expression of IL-1 and IL-6 mRNA in freshly isolated monocytes and macrophages cultured for 7 days has been investigated. Interestingly, IL-1 and IL-6 gene expression has been regulated differently in these two distinct stages of monocyte/macrophage maturation. In the monocytes, basal IL-1 and IL-6 mRNA expression has been found to be slightly upregulated by 1α,25(OH)2D3 treatment compared to untreated controls. For 1α,25(OH)2D3 treated monocytes that were additionally stimulated with LPS or TNFα, no or only marginal differences have been found compared to LPS or TNFα treatment without 1α,25(OH)2D3 preincubation. In contrast, 1α,25(OH)2D3 treatment reduced basal IL-1 and IL-6 levels in macrophages. In 1α,25(OH)2D3 treated macrophages that were additionally stimulated with LPS or TNFα, only TNFα-stimulated IL-6 mRNA expression was influenced, whereas no significant changes were observed for IL-1 and IL-6 after 1α,25(OH)2D3/LPS-treatment. These findings show that in monocytes/macrophages, the influence of 1α,25(OH)2D3 on IL expression depends on the type of IL under consideration, the degree of maturation, and the stimulus that is employed (Di Rosa et al., 2012). In a second recent investigation, significant inhibition of IL-6 mRNA expression and protein secretion was observed in PBMC, and subsequent FACS-based analysis revealed a concomitant decrease in CD14+ IL-6-producing monocytes (Zhang et al., 2012) (Figure 3).
Apart from the two prominent monokines IL-1 and IL-6, the synthesis of IL-3 has been found to be influenced by 1α,25(OH)2D3 in the murine monocytic cell line WEHI-3. However, whereas one report describes dose-dependent inhibition of IL-3 production in this cell line (Abe et al., 1986), the second finds concentration-dependent stimulation or inhibition of IL-3 production (Hodler et al., 1985). Furthermore, the interleukin family members IL-8, IL10, and IL-12 have been studied more intensely on mechanistic level.
IL-10 and IL-12-production by stimulated primary human monocytes has been found to be negatively regulated by 1α,25(OH)2D3 (Lemire et al., 1995; Lyakh et al., 2005). These two genes have been identified as primary 1α,25(OH)2D3 target genes as judged by rapid VDR recruitment detected via ChIP assays in the monocytic cell line THP-1 (Matilainen et al., 2010b). Further studies with this cell line include extensive mechanistic analyses regarding the influence of 1α,25(OH)2D3 on the expression of IL-8, IL-10, and IL-12B. The IL-8 gene has been shown to be an up-regulated, primary target gene, located within an insulated cluster of CXC motif ligand (CXCL) genes. IL-8 and its neighboring genes CXCL1 and CXCL6 seem to be under the control of a consensus VDR binding motif located 22 kb downstream of the IL-8 transcription start site, which mediates 1α,25(OH)2D3–dependent chromatin opening (Ryynanen and Carlberg, 2013). As discussed in this report, this finding is seemingly in contradiction with other studies (e.g., Di Rosa et al., 2012). These studies used different cells and foremost, cells were stimulated with agents like LPS that activate transcription factors, e.g., NF-κB, that are themselves regulated by 1α,25(OH)2D3. As described above, NF-κB activity is inhibited by 1α,25(OH)2D3 (Harant et al., 1998) (Figure 2). It has been put forward that 1α,25(OH)2D3 may have a dual effect: primary up-regulation of genes like IL-8, which supports the inflammatory response in the early phase of inflammation, e.g., by IL-8 production, and secondary effects which would help to shut down the inflammatory process, e.g., by inhibition of NF-κB-mediated pro-inflammatory responses (Ryynanen and Carlberg, 2013). This could explain that in another study in which THP-1 cells were used, no significant effect of 1α,25(OH)2D3 on IL-8 expression was found on protein level. In this study, the cells have been stimulated with LPS after only 2 h of 1α,25(OH)2D3 treatment before IL-8 protein was analyzed after 24 and 48 h (Kuo et al., 2010). Similarly, U937 cells exposed to high glucose (a condition which leads to different stress responses like NF-κB or MAPK activation) (Stan et al., 2011; Yang et al., 2013) showed lower IL-8 secretion after pretreatment with 1α,25(OH)2D3 (Jain and Micinski, 2013). Therefore, the interference of 1α,25(OH)2D3 with cell signaling pathways of inflammatory or cell stress responses, like NF-κB or MAPK activation, and differences in treatment schedules may explain the different findings. In contrast to IL-8 as an up-regulated gene, the primary effect of 1α,25(OH)2D3 on IL-10 expression is down-regulation, followed by up-regulation at a later stage (Figure 3). Cyclic binding of VDR to a distal promoter region with conserved VDREs, that loops 1α,25(OH)2D3-dependently to the transcription start site and induces epigenetic changes and chromatin remodeling, was detected (Matilainen et al., 2010a,b). IL-12B has been identified as a 1α,25(OH)2D3-dependently down-regulated gene in LPS-treated THP-1 cells. The gene harbors two VDR binding sites within ~6 kb upstream of the transcription start site to which the VDR and its partner retinoid receptor (RXR) recruit co-repressors and consequently induce epigenetic changes associated with gene repression (Matilainen et al., 2010b; Gynther et al., 2011). An earlier report attributed the down-regulation of IL-12 via interference of 1α,25(OH)2D3/VDR with NF-κB binding to proximal IL-12 promoter regions (D'Ambrosio et al., 1998). It has been suggested in the more recent report that this suppression of proximal sites is due to epigenetic changes at that location via the distal VDRE binding sites identified in the more recent study (Gynther et al., 2011) (Figure 3).
In addition to data from experiments with monocytes, macrophages, and DCs as differentiated members of the monocytic lineage have been investigated.
In macrophages from vitamin D-deficient mice, IL-1, and IL-6 production (evaluated as biological activity) was significantly reduced relative to control mice. Notably, this was paralleled by a decrease in macrophage cytotoxicity. Furthermore, the vitamin D deficient mice had reduced serum levels of IL-1 and IL-6 after challenge with LPS (Kankova et al., 1991). In human monocyte-derived macrophages and PMA-differentiated U937 cells, which were stimulated with LPS or PMA, IL-1β production was strongly stimulated by 1α,25(OH)2D3. This effect was ascribed to increased IL-1β transcription, but not by RNA stabilization, and seemed to be mediated by Erk1/2. Moreover, 1α,25(OH)2D3 induced the expression and phosphorylation of CCAAT enhancer-binding protein β as a known IL-1 β-regulating transcription factor (Lee et al., 2011). The upregulation of IL-1β by 1α,25(OH)2D3 is also relevant for infection-induced inflammation, as in THP-1 cells or primary human macrophages infected with Mycobacterium tuberculosis (as well as in non-infected controls), 1α,25(OH)2D3 increased the expression of IL-1β mRNA. IL-1β is a critical factor for host defense in this disease. Notably, mature intracellular IL-1β protein was only detected in infected, 1α,25(OH)2D3 treated THP-1 cells, which represents a further level of gene expression control exerted by 1α,25(OH)2D3. Secretion of IL-1β was only seen in infected cells, and significantly enhanced by 1α,25(OH)2D3. With respect to the mechanism, the study revealed 1α,25(OH)2D3–dependent binding of VDR to a promoter-proximal consensus VDRE, which was paralleled by upregulated VDR-expression, and recruitment of RNA polymerase II to the transcription start site (Verway et al., 2013).
In a further study with mouse macrophages, 1α,25(OH)2D3 led to reduced mRNA expression of the IL-12 subunit p40 in response to LPS/interferon gamma (IFNγ) stimulation (Korf et al., 2012), which is in line with the effects seen in monocytes, as described above (Figure 3). Stimulation of the macrophages with 1α,25(OH)2D3 was accompanied by upregulation of VDR and the 1α,25(OH)2D3–catabolic enzyme CYP24. Further changes concerned the potential to stimulate T-cells, as assessed by co-culture experiments including FACS analysis of surface markers. These effects could not be observed with IL-10 deficient macrophages. Notably, the effects on IL-12 p40 expression and T-cell stimulation also occurred in monocytes/macrophages from non-obese diabetic (NOD) mice, which have a background of inflammatory features seen in type 1 diabetes (Korf et al., 2012).
Analogous studies have been conducted for DCs from NOD mice or non- obese diabetes-resistant (NOR) control mice. In both cases, 1α,25(OH)2D3 altered the phenotype of DCs and inhibited the LPS/IFNγ–induced mRNA expression and protein secretion of IL-10 and IL-12 (Van Etten et al., 2004). In general, it has been shown that 1α,25(OH)2D3 prevents in vitro differentiation of human monocytes into immature DCs, associated with decreased capacity to activate T-cells. Furthermore, 1α,25(OH)2D3 inhibits maturation of DCs. In maturating DCs, 1α,25(OH)2D3 reduces IL-12p70 and enhances IL-10 secretion upon stimulation of the DCs by CD40-crosslinking (Penna and Adorini, 2000). This has been independently confirmed for IL-12p70 production upon LPS stimulation (Sochorova et al., 2009). Additionally, these findings are in line with a study on the generation of regulatory DCs for therapeutic use from human monocytes, which were differentiated in the presence of 1α,25(OH)2D3. Apart from reduced LPS-induced IL-12 and enhanced IL-10 secretion of the maturating cells, a major characteristic of these 1α,25(OH)2D3–treated DCs is their low level of IL-23 secretion, which was apparent with or without stimulation with LPS (Pedersen et al., 2009) (Figure 3). A further recent investigation used monocyte-derived DCs from Crohn's disease patients. When the cells were cultured in the presence of 25(OH)D3 or 1α,25(OH)2D3 and matured with LPS, they exhibited significantly increased IL-6 production, and non-significant reductions in and IL-10 and IL-12p70. IL-1β and Il-8 levels were not affected in this study (Bartels et al., 2013).
B-cells and neutrophils have been less intensively studied, but the available data show that IL gene expression in these cells is also targeted by 1α,25(OH)2D3. In isolated human peripheral B-cells, IL-10 secretion can be induced by stimulation (cross-linking of B-cell receptor/CD40 antibody/IL-4). This production can be enhanced by 1α,25(OH)2D3. Besides the influence on IL gene expression, 1α,25(OH)2D3 induces the expression of VDR and Cyp24 mRNA in the stimulated B-cells. These activated cells also express Cyp27b1 mRNA and are able to produce 1α,25(OH)2D3 from 25(OH)D3. Binding of VDR to a VDRE in the proximal IL-10 promoter has been shown by ChIP assay, and binding of RNA-polymerase II could only be detected in IL-10 secreting B-cells (Heine et al., 2008).
Neutrophils respond to 1α,25(OH)2D3 by a slight reduction of IL-1β mRNA expression. Notably, the abundance of VDR mRNA in neutrophils has been found to be comparable with monocytes (Takahashi et al., 2002).
In a first study where these cell types were used, IL-1-stimulated normal human dermal fibroblasts, normal human keratinocytes and normal human endothelial cells were investigated regarding changes of IL-8 mRNA and protein expression in dependence of 1α,25(OH)2D3 treatment. IL-8 expression was reduced by 1α,25(OH)2D3 on both levels of gene expression for fibroblasts and keratinocytes, but not for endothelial cells, where no significant changes have been found (Larsen et al., 1991).
For IL-8, and also for IL-6 protein production, this result has been confirmed in studies using phorbol ester stimulated human fibroblast cell lines (Srviastava et al., 1994), and in experiments employing TNF-α-stimulated human dermal fibroblasts (Fukuoka et al., 1998). Similar results have been obtained with fibroblast cultures obtained from surgery of patients suffering from nasal polyposis, which is defined as a chronic inflammatory process. However, rather high concentrations (10–100 μM) of 1α,25(OH)2D3 were necessary to significantly reduce IL-6 and IL-8 production in these cells (Rostkowska-Nadolska et al., 2010).
In cultured normal human keratinocytes, only minor effects were observed for IL-1α and IL-8 production, when the influence of 1α,25(OH)2D3 was investigated for otherwise untreated cells. However, TNF-α-stimulation led to slightly enhanced IL-1α and markedly increased IL-8 secretion, which could be reduced by 1α,25(OH)2D3 (Zhang et al., 1994). This was confirmed for IL-8 (Koizumi et al., 1997). On the other hand, stimulation with phorbol ester plus LPS caused a rise in IL-8 production, but a decrease in IL-1α. 1α,25(OH)2D3 inhibited IL-8 secretion and restored IL-1α production (Zhang et al., 1994). Stimulation of normal human keratinocytes with IL-17A resulted in a pronounced increase in IL-6 mRNA and IL-8 protein secretion, which could be effectively blocked by 1α,25(OH)2D3 treatment (Peric et al., 2008). In a mechanistically insightful study, the effect of 1α,25(OH)2D3 on the expression of IL-1α, the intracellular IL-1 receptor antagonist (icIL-1Ra) and IL-18 was studied in mouse primary keratinocytes. Treatment with 1α,25(OH)2D3 induced IL-1α and icIL-1Ra mRNA and protein, however, the ratio of icIL-1Ra to IL-1, which determines the effect on IL-1 activity, was markedly increased, and indeed reduced IL-1 activity could be detected. The use of keratinocytes from VDR−/− mice confirmed that the effect was mediated by VDR. Regarding the mechanism of gene regulation, increased IL-1α mRNA stability was observed and enhanced icIL-1Ra gene transcription via a secondary mechanism have been suggested to account for the effects on these gene. 1α,25(OH)2D3 markedly suppressed IL-18 mRNA expression, and the effect was dependent on VDR, as no effect of 1α,25(OH)2D3 was seen in VDR−/− mice. These mice exhibit markedly elevated basal levels of IL-18 mRNA and protein, and expression of human VDR in these mice could restore basal levels (Kong et al., 2006).
A further cell type involved in inflammatory responses, especially in infection-mediated inflammation, are epithelial cells. Treatment of human microvessel endothelial cells with 1α,25(OH)2D3 suppresses LPS-induced IL-6 and IL-8 release, whereas 1α,25(OH)2D3 alone does not affect IL production. As assessed by reporter gene assay, this seems to be based on inhibition of LPS-induced NF-κB activation. This activation usually occurs via the MyD88-dependent branch of TLR4-signaling. In contrast, 1α,25(OH)2D3 did not influence the activity of interferon-β-promoter constructs, which has been determined as a measure of MyD88-independent LPS/TLR4 signaling (Equils et al., 2006). Reduced IL-6 and IL-8 production was also seen in 1α,25(OH)2D3-treated cystic fibrosis respiratory epithelial cell lines challenged with LPS. With respect to NF-κB-signaling, reduced IκBα phosphorylation and increased total cellular IκBα upon 1α,25(OH)2D3 treatment have been found in this study (McNally et al., 2011) (Figure 2). Similar findings have been made for human umbilical vein cord endothelial cells (HUVEC) incubated cultured in a CKD-like environment (hypocalcemia, advanced glycation end products, parathyroid hormone) and 1α,25(OH)2D3. This environment provoked enhanced IL-6 expression and secretion, increased DNA-binding of NF-κB-p65 and decreased IκBα expression. These changes were counteracted by 1α,25(OH)2D3 (Talmor-Barkan et al., 2011). In TNFα-stimulated human coronary arterial cells, a slight, but significant reduction of IL-8 production has been observed for 1α,25(OH)2D3 treatment in certain concentrations, but IL-6 production could not be influenced (Kudo et al., 2012). An interesting novel mechanism for interference of 1α,25(OH)2D3 and LPS-stimulated IL-8 production from epithelial cells has been proposed in a recent study, where a vitamin D3 derivative have been found to increase the release of the soluble form of CD14 (sCD14) via ERK1/2 activation. Neutralization of LPS by sCD14 could account for the effect of the vitamin D analog (Hidaka et al., 2013).
Placental inflammation including release of interleukins is associated with preeclampsia, preterm labor, and abortion. Therefore, cell types involved in this inflammatory condition have been investigated regarding the influence of 1α,25(OH)2D3 on IL secretion. In cultured human trophoblasts, 1α,25(OH)2D3 reduced TNFα-induced IL-6 mRNA expression and protein secretion (Diaz et al., 2009). Mechanistic evidence regarding the influence of vitamin D signaling on IL gene expression in placental tissue was presented in a study with for Cyp27b1−/− (vitamin D-activating 1α-hydroxylase) mice and VDR−/− mice. In these mice, basal expression of IL-10 mRNA was decreased relative to wildtype placentas, and LPS stimulation resulted in higher levels of IL-6 mRNA in the −/− placentas compared to wildtype. PCR array analysis of LPS-stimulated placental tissue from Cyp27b1−/− mice revealed enhanced expression of IL-4, IL-15, and IL-18 mRNA relative to WT and the same experiments with VDR−/− mice yielded higher IL-1α and IL-6 mRNA levels. Further experiments with LPS-stimulated placentas from WT mice showed that treatment with 25(OH)D3 as the substrate of CYP27B1 reduces IL-6 mRNA expression. Moreover, LPS challenge of pregnant WT mice led to enhanced expression of Cyp27b1 and VDR. Apart from the mechanistic conclusion that VDR signaling is a factor that controls IL gene expression, these results show that pro-inflammatory stimuli are able to enhance the expression of crucial vitamin D signaling components which are able to mediate anti-inflammatory responses (Liu et al., 2011).
In line with these findings, experiments using human endometrial cells from women with unexplained recurrent spontaneous abortion (URSA) or in controls, significant down-regulation of IL-6 by 1,25(OH)2D3 was observed in two cell types (whole endometrial cells and endometrial stromal cells), but for IL-8, opposed effects were observed for the two cell types in URSA samples, which highlights the complexity of these responses given the fact that several cell types are involved in inflammatory processes (Tavakoli et al., 2011).
Obesity is a disease condition which is strongly associated with low-grade inflammation, therefore adipocytes have been used as a further model system regarding the interplay of vitamin D signaling and IL gene expression/production. In a recent report, human adipocytes from biopsies and from differentiated human mesenchymal stromal cells were studied with respect to IL-6 gene expression/release depending on the presence of 1α,25(OH)2D3. LPS-induced IL-6 mRNA and protein were reduced in both systems by cotreatment with 1α,25(OH)2D3. Regarding the underlying signal transduction events, it was shown that 1α,25(OH)2D3 inhibited IκB phosphorylation and thus NF-κB translocation into the nucleus (Figure 2). DNA binding of NF-κB complexes upon LPS stimulation was significantly reduced in 1α,25(OH)2D3-pretreated cells compared to controls (Mutt et al., 2012). A further recent investigation addressed the influence of in vitro and in vivo administered 1α,25(OH)2D3 on IL-6 and IL-8 gene expression from IL-1β-stimulated human adipose tissue. The adipose tissue samples have been either (i) treated in vitro with 1α,25(OH)2D3 or have been (ii) obtained from obese subjects with low plasma levels of 25(OH)D3 after in vivo (oral) treatment with high-dose 1α,25(OH)2D3 or placebo. In the in vitro study, reduced mRNA levels of IL-6 and IL-8 and reduced IL-6 and IL-8 protein (significance only shown for IL-8) have been found. However, although the in vivo treatment led to a small decrease of IL-6 and IL-8 mRNA expression in the adipose tissue, there were no significant differences between the 1α,25(OH)2D3–treated and the control group. Oral treatment with 1α,25(OH)2D3 did also not significantly change circulating levels of IL protein in the subjects pre- and post-treatment (Wamberg et al., 2013). These findings urge caution about the extrapolation of in vitro findings to the in vivo situation.
Apart from studies with primary cells, cultures of adipocyte-like murine 3T3-L1 cells have been used, but contradictory results have been reported e.g., regarding IL-6 gene expression (Sun and Zemel, 2008; Marcotorchino et al., 2012).
A further aspect that underscores the importance of vitamin D signal transduction on IL biosynthesis is the effect of the VDR receptor gene variants on IL gene expression. The single-nucleotide polymorphism FokI, which comprises a shorter VDR protein of 424 aa or the long isoform with 427 aa, influences IL-12 expression. In human monocytes and DCs, presence of the short VDR isoform leads to a higher expression of IL-12 compared to the long isoform, a result which was reflected by results from reporter gene assays with IL-12 promoter fragments (Van Etten et al., 2007). Moreover, VDR gene promoter variants have an impact on the expression of IL-10 in blood mononuclear cells (Selvaraj et al., 2008).
Changes in IL production can be observed in VDR KO mice. VDR KO considerably facilitates development of IL-17 secreting T-cells (Th17 cells) in response to respective in vitro stimuli. Further, enhanced IL-17 production was observed in these Th17 cells compared to wildtype. Conversely, a reduction in regulatory T-cells and tolerogenic DCs was observed. Moreover, IBD can be induced experimentally in these mice by transfer of naive T-cells that develop into specific, IBD-inducing subsets. The severity of IBD was strongly enhanced in VDR KO mice compared to control animals, which was ascribed to the increased propensity for development into Th17 cells (Bruce et al., 2011).
Apart from induction of IL gene expression/protein release, 1α,25(OH)2D3 may also modulate IL signaling via regulation of IL receptor expression. In early reports, moderate downregulation (Matsui et al., 1986) or no changes (Jordan et al., 1989) were found regarding IL-2 receptor expression in 1α,25(OH)2D3 treated, mitogen-stimulated PBMC, or mitogen/phorbol ester-stimulated T-cells, respectively. However, IL-2 mediated expression of IL-2 receptor units was superinduced by 1α,25(OH)2D3 in mitogen-stimulated PBMC (Rigby et al., 1990). The vitamin D3 upregulated protein 1 (VDUP1), which is expressed in a 1α,25(OH)2D3-dependent manner, has been found to inhibit the activity of the IL-3 receptor promoter (Han et al., 2003). On the other hand, IL-1 and IL-4 receptor densities seem to be upregulated by 1α,25(OH)2D3 on a murine T-cell line and a murine osteoblast cell line, respectively (Lacey et al., 1993a,b). Furthermore, downregulated IL-22 mRNA and protein levels have been detected in cultured epidermis tissue treated with calcipotriol, a vitamin D analog (Moniaga et al., 2013).
The impact of 1α,25(OH)2D3 on TNFα gene expression was primarily studied in PBMC, primary monocytes/macrophages or in monocytic cell lines. Expression was investigated both on mRNA level and/or on the level of protein production, and was sometimes reported in terms of protein release as a secondary readout.
In general, in vitro or in vivo treatment with 1α,25(OH)2D3 of PBMC caused a decrease in TNFα gene expression and/or TNFα production. This was the case for PBMC from healthy donors that were stimulated with different agents (LPS, Muller et al., 1992; Panichi et al., 1998; Rausch-Fan et al., 2002); live Mycobacterium tuberculosis (Prabhu Anand et al., 2009), as well as for PBMC from patients suffering from diseases with inflammatory features [renal disease (Riancho et al., 1993; Panichi et al., 1998); pulmonary tuberculosis (Prabhu Anand et al., 2009)]. Analogous findings were obtained with monocyte-enriched PBMC after stimulation with LPS (Muller et al., 1992), IFNγ or phorbol ester (Zarrabeitia et al., 1992) (however, not with LPS in this particular report). In one of the latter studies, nuclear run-off analysis did not indicate that TNFα gene transcription was affected by 1α,25(OH)2D3 (Muller et al., 1992).
In contrast to the findings with PBMC or monocyte-enriched PBMC, studies that used human primary monocytes or macrophages often found increased TNFα expression/secretion after 1α,25(OH)2D3 exposure, either regarding basal levels (human monocyte-derived macrophages; Bermudez et al., 1990) or with respect to stimulus-induced mRNA or protein levels (murine alveolar macrophages/LPS- or PMA-stimulation, Higashimoto et al., 1995; peritoneal macrophages from continuous peritoneal dialysis patients/LPS-stimulation, Cohen et al., 2001). In line with this, murine bone-marrow derived macrophages (BMMs) responded to 1α,25(OH)2D3 with an increase in TNFα mRNA abundance, which was synergistically enhanced by LPS stimulation. This study also addressed molecular mechanisms. Treatment with 1α,25(OH)2D3 and stimulation with LPS did not influence TNFα mRNA stability, but the data suggested that 1α,25(OH)2D3 regulates the TNFα gene on transcriptional level, as a VDR-binding sequence could be identified in the TNFα promoter region using electrophoretic mobility shift assays (Hakim and Bar-Shavit, 2003).
When human monocytic cell lines were studied, heterogeneous results were obtained, and the outcome seems to depend on the differentiation status of the cells (e.g., Bhalla et al., 1991). For the three cell lines that were mainly employed, the order of their stage of maturation is known. HL-60 cells are myelomonocytic stem-cells and thus are the least mature cell line; U937 are characterized as monoblasts, and represent an intermediate stage; and THP-1 cells are regarded as promonocytic cells and are therefore the most mature cell line (Frankenberger et al., 1994).
In HL-60 cells, 1α,25(OH)2D3 had no influence on PMA-induced TNFα mRNA expression, but enhanced it in U937 cells (Bhalla et al., 1991). In a second study, 1α,25(OH)2D3 preincubation of U937 cells accelerated LPS-induced TNFα mRNA expression and led to higher steady-state mRNA levels which were associated with enhanced TNFα protein production. Mechanistic analysis pointed to a secondary effect since 1α,25(OH)2D3 pretreatment was needed for more than 6 h in order to achieve enhanced TNFα protein synthesis. The requirement of 1α,25(OH)2D3-driven expression of the LPS co-receptor CD14, was suggested to be the mechanistic basis of his secondary effect (Prehn et al., 1992). In a further investigation, differentiation by 1α,25(OH)2D3 enhanced LPS-induced TNFα secretion in U937 and THP-1 cells. Concomitant increase in TNFα mRNA was confirmed for U937 cells (Taimi et al., 1993). In contrast, 1α,25(OH)2D3 was reported to significantly suppress TNFα release in LPS-stimulated THP-1 cells and human primary monocytes (Kuo et al., 2010), and a further study reported reduced TNFα production and secretion from 1α,25(OH)2D3-treated, IFNγ-activated THP-1 cells (Villaggio et al., 2012).
In one report, TNFα mRNA levels of 1α,25(OH)2D3–treated human PBMC, U937 and THP-1 cells, that were stimulated either with LPS or with phytohemagglutinin (PHA), were compared. Differences occurred between the two sample types and the two stimuli. In PBMC, LPS had no influence on TNFα expression in the presence of 1α,25(OH)2D3, whereas upon PHA-stimulation, reduced TNFα mRNA levels were observed. In contrast, U937 cells (but not THP-1 cells) responded by an increase in TNFα mRNA expression (Blifeld et al., 1991).
Taken together, several studies report an increase in TNFα mRNA and protein expression in 1α,25(OH)2D3-treated, subsequently stimulated U937 cells, but equivocal effects were found with the more mature THP-1 cells. In monocyte-derived DCs from patients that suffer from Crohn's disease, TNFα production was decreased when the cells were differentiated with LPS in the presence of 1α,25(OH)2D3 (Bartels et al., 2013).
T-cells have not been intensively studied, but regulation of TNFα-expression by 1α,25(OH)2D3 has been analyzed in T-cell subsets obtained from normal healthy subjects and pulmonary tuberculosis patients. Here, 1α,25(OH)2D3 reduced the percentage of TNFα-expressing T-cell subsets (CD3+, CD3+CD4+, CD3+CD8+) (Prabhu Anand et al., 2009) (Figure 3).
Other cell types that were analyzed are prostate cancer lines, where 1α,25(OH)2D3 reduced basal TNFα mRNA expression (Golovko et al., 2005), or 1α,25(OH)2D3/IL-1β-stimulated synoviocytes, where TNFα mRNA was decreased (Feng et al., 2013).
In summary, 1α,25(OH)2D3-mediated downregulation of TNFα gene expression has been found in cell preparations which contain a high percentage of T-cells (PBMC or monocyte-enriched PBMC). In monocytic cells, upregulation has been reported for cell lines that represent an intermediate monocytic differentiation state, whereas for more mature cells, heterogeneous results have been found. Regarding the mechanism, it has been suggested that primary effects may play a role for 1α,25(OH)2D3 regulation of TNFα gene expression, since a VDR binding element has been found in the TNFα promoter region (Hakim and Bar-Shavit, 2003). On the other hand, kinetic analysis pointed to a secondary effect, where the expression of CD14 could play a role, at least for LPS-induced TNFα expression (Prehn et al., 1992). It has to be noted, however, that cell-type specific mechanisms have been found for T-cell specific expression of the TNFα gene. Cell type-specific DNA-protein-interactions have been identified for the TNFα gene when T-cells and monocytic cells were compared. A highly conserved region in intron 3 seems to be responsible for cell specificity, as this sequence induces specific activity of a TNFα-reporter plasmid in Jurkat T-cells, but not THP-1 cells (Barthel and Goldfeld, 2003). Possibly, cell specific protein complexes within this region interact with 1α,25(OH)2D3 signaling components in T-cells.
IFNγ is a well-established effector in anti-infectious host reactions, autoimmune diseases and inflammation. IFNγ is mainly produced by NK and T-cells. Inhibition of IFNγ mRNA and protein secretion has been described for 1α,25(OH)2D3-treated human PBMC, peripheral blood lymphocytes or T-cells that were stimulated with phytohemagglutinin and phorbol ester (Matsui et al., 1986; Reichel et al., 1987; Rigby et al., 1987; Inoue et al., 1998) (Figure 3). Mechanistic insights exist from experiments using transient transfection of IFNγ promoter constructs in Jurkat T-cells. Here, it could be concluded that two VDR binding regions, one around −200 bp from the transcription start site and the second directly around the transcription start site, are involved in the regulation of IFNγ gene expression by 1α,25(OH)2D3 (Cippitelli and Santoni, 1998).
It is well established that 1α,25(OH)2D3 influences cytokine gene expression and signaling in several different cell types. Firstly, this is the case for the pleiotropic mediator TGF-β, for which it has been shown that either the expression of the cytokine itself or expression of associated signaling components is downregulated by 1α,25(OH)2D3. In hepatocytes, 1α,25(OH)2D3 has been found to influence TGF-β signaling in a genome wide scale by directing binding of Smad proteins to target genes. These actions of 1α,25(OH)2D3 on TGF-β expression or signaling were able to inhibit fibrosis and associated inflammation. Second, the interleukins are a vast group of inflammatory cytokines that are clearly regulated by 1α,25(OH)2D3 in a cell-specific manner. However, for several members of this family (e.g., IL-1, IL-6, and IL-8), both positive or negative regulation by 1α,25(OH)2D3 has been observed. A closer look at the parameters that determine the outcome of 1α,25(OH)2D3 action on the expression of these genes is warranted. This applies in particular to the time-scale of changes in gene expression, as different responses may occur during separate stages of 1α,25(OH)2D3 action. Regarding the mechanisms, recruitment of VDR to the respective genomic regions, as well as interaction of 1α,25(OH)2D3 signaling with other transcription factors involved in IL expression (NFAT, NF-κB, Runx1), seem to occur. Concerning the p38 MAP kinase phosphatase MKP1, it was found that GCR and VDR/RXR act in a synergistic manner to induce MKP1 expression in monocytes. This results in reduced p38 activation and reduced formation of proinflammatory cytokines. As a further cytokine, the proinflammatory mediator TNFα has been identified as a 1α,25(OH)2D3 target gene. Also in this case, the vitamin D effects are cell-specific: With cell samples that mainly contain T-cells, downregulation of TNFα has been observed, whereas for monocytic cells, either positive or negative regulation occurred depending on the differentiation state. Finally, gene expression of the proinflammatory mediator IFNγ has been described to be suppressed by 1α,25(OH)2D3 in T-cells. Altogether, the influence of 1α,25(OH)2D3 on the expression of interleukins, TNFα, and IFNγ by different cell types, and the consequences for the cellular interplay that are to be anticipated, amounts to a complex picture. In Figure 3, the influence of 1α,25(OH)2D3 on the expression of these cytokines is summarized for the major immune cells (monocytes, DCs, and different T-cell subsets). The resulting pattern supports a shift of T-cell responses from a Th1 type toward Th2 reactions and a suppression of Th17 responses. The effect of 1α,25(OH)2D3 on cytokine expression in antigen presenting cells (monocytes, DCs) remains unclear and seems to depend on the time of stimulation, the differentiation state and other factors.
Modulation of GCR, NFκB, NFAT as well as SMAD signaling plays a central role in the immunomodulatory activities of 1α,25(OH)2D3. Mechanistic studies on individual genes gave some mechanistic insights into the mechanisms involved in the interaction between VDR/RXR and the above mentioned transcription factors. These mechanisms include competitive binding as well as a crosstalk between the signaling pathways on multiple levels including the promoter level. However, by using ChIP seq and other techniques which allow a genome-wide view, we are just starting to understand the signaling network which is responsible for cell-type-specific and locus-dependent gene activation by ligand-regulated transcription factors such as VDR/RXR. For example, intersecting VDR/SMAD regulatory circuits have just been unraveled and it was shown that TGFβ signaling facilitates VDR binding to certain gene loci. More such data are required to increase our understanding of the complex gene regulatory network that is affected by 1α,25(OH)2D3. Especially, genome-wide data on VDR loci in conjunction with analyses of other, inflammation-related key transcription factors in different cell types and various stimuli are necessary to understand the complex regulation of gene transcription during inflammation.
The authors declare that the research was conducted in the absence of any commercial or financial relationships that could be construed as a potential conflict of interest.
Studies in our laboratory were supported by the Dr. Hans Kröner Graduiertenkolleg, the LOEWE Initiative (Fraunhofer-TMP) the cluster of excellence Macromolecular Complexes (EXC 115) and DFG (SFB 1039).
Abe, J., Moriya, Y., Saito, M., Sugawara, Y., Suda, T., and Nishii, Y. (1986). Modulation of cell growth, differentiation, and production of interleukin-3 by 1 alpha,25-dihydroxyvitamin D3 in the murine myelomonocytic leukemia cell line WEHI-3. Cancer Res. 46, 6316–6321.
Adorini, L., and Penna, G. (2008). Control of autoimmune diseases by the vitamin D endocrine system. Nat. Clin. Pract. Rheumatol. 4, 404–412. doi: 10.1038/ncprheum0855
Alroy, I., Towers, T. L., and Freedman, L. P. (1995). Transcriptional repression of the interleukin-2 gene by vitamin D3: direct inhibition of NFATp/AP-1 complex formation by a nuclear hormone receptor. Mol. Cell. Biol. 15, 5789–5799.
Ananthakrishnan, A. N. (2013). Environmental triggers for inflammatory bowel disease. Curr. Gastroenterol. Rep. 15, 302. doi: 10.1007/s11894-012-0302-4
Barnes, P. J. (1998). Anti-inflammatory actions of glucocorticoids: molecular mechanisms. Clin. Sci. (Lond.) 94, 557–572.
Bartels, L. E., Jorgensen, S. P., Bendix, M., Hvas, C. L., Agnholt, J., Agger, R., et al. (2013). 25-Hydroxy vitamin D3 modulates dendritic cell phenotype and function in Crohn's disease. Inflammopharmacology 21, 177–186. doi: 10.1007/s10787-012-0168-y
Barthel, R., and Goldfeld, A. E. (2003). T cell-specific expression of the human TNF-alpha gene involves a functional and highly conserved chromatin signature in intron 3. J. Immunol. 171, 3612–3619. doi: 10.4049/jimmunol.171.7.3612
Bendix-Struve, M., Bartels, L. E., Agnholt, J., Dige, A., Jorgensen, S. P., and Dahlerup, J. F. (2010). Vitamin D3 treatment of Crohn's disease patients increases stimulated T cell IL-6 production and proliferation. Aliment. Pharmacol. Ther. 32, 1364–1372. doi: 10.1111/j.1365-2036.2010.04463.x
Bener, A., Ehlayel, M. S., Tulic, M. K., and Hamid, Q. (2012). Vitamin D deficiency as a strong predictor of asthma in children. Int. Arch. Allergy Immunol. 157, 168–175. doi: 10.1159/000323941
Bennett, C. F., Chiang, M. Y., Monia, B. P., and Crooke, S. T. (1993). Regulation of 5-lipoxygenase and 5-lipoxygenase-activating protein expression in HL-60 cells. Biochem. J. 289 (Pt. 1), 33–39.
Bermudez, L. E., Young, L. S., and Gupta, S. (1990). 1,25 Dihydroxyvitamin D3-dependent inhibition of growth or killing of Mycobacterium avium complex in human macrophages is mediated by TNF and GM-CSF. Cell. Immunol. 127, 432–441. doi: 10.1016/0008-8749(90)90144-G
Bhalla, A. K., Amento, E. P., and Krane, S. M. (1986). Differential effects of 1,25-dihydroxyvitamin D3 on human lymphocytes and monocyte/macrophages: inhibition of interleukin-2 and augmentation of interleukin-1 production. Cell. Immunol. 98, 311–322. doi: 10.1016/0008-8749(86)90291-1
Bhalla, A. K., Paavonen, T., Williams, M. M., Delves, P. J., and Lydyard, P. M. (1991). Regulation of interleukin-1 and tumour necrosis factor gene expression in myelomonocytic cell lines by 1,25-dihydroxyvitamin D3. Immunology 72, 61–64.
Bierie, B., and Moses, H. L. (2010). Transforming growth factor beta (TGF-beta) and inflammation in cancer. Cytokine Growth Factor Rev. 21, 49–59. doi: 10.1016/j.cytogfr.2009.11.008
Blifeld, C., Prehn, J. L., and Jordan, S. C. (1991). Stimulus-specific 1,25(OH)2D3 modulation of TNF and IL-1-beta gene expression in human peripheral blood mononuclear cells and monocytoid cell lines. Transplantation 51, 498–503. doi: 10.1097/00007890-199102000-00043
Brehm, J. M., Acosta-Perez, E., Klei, L., Roeder, K., Barmada, M., Boutaoui, N., et al. (2012). Vitamin D insufficiency and severe asthma exacerbations in Puerto Rican children. Am. J. Respir. Crit. Care Med. 186, 140–146. doi: 10.1164/rccm.201203-0431OC
Brehm, J. M., Celedon, J. C., Soto-Quiros, M. E., Avila, L., Hunninghake, G. M., Forno, E., et al. (2009). Serum vitamin D levels and markers of severity of childhood asthma in Costa Rica. Am. J. Respir. Crit. Care Med. 179, 765–771. doi: 10.1164/rccm.200808-1361OC
Brehm, J. M., Schuemann, B., Fuhlbrigge, A. L., Hollis, B. W., Strunk, R. C., Zeiger, R. S., et al. (2010). Serum vitamin D levels and severe asthma exacerbations in the Childhood Asthma Management Program study. J. Allergy Clin. Immunol. 126, 52–58. doi: 10.1016/j.jaci.2010.03.043
Brewer, L. C., Michos, E. D., and Reis, J. P. (2011). Vitamin D in atherosclerosis, vascular disease, and endothelial function. Curr. Drug Targets 12, 54–60. doi: 10.2174/138945011793591617
Bruce, D., Yu, S., Ooi, J. H., and Cantorna, M. T. (2011). Converging pathways lead to overproduction of IL-17 in the absence of vitamin D signaling. Int. Immunol. 23, 519–528. doi: 10.1093/intimm/dxr045
Brungs, M., Radmark, O., Samuelsson, B., and Steinhilber, D. (1994). On the induction of 5-lipoxygenase expression and activity in HL-60 cells: effects of vitamin D3, retinoic acid, DMSO and TGF beta. Biochem. Biophys. Res. Commun. 205, 1572–1580. doi: 10.1006/bbrc.1994.2846
Brungs, M., Radmark, O., Samuelsson, B., and Steinhilber, D. (1995). Sequential induction of 5-lipoxygenase gene expression and activity in Mono Mac 6 cells by transforming growth factor beta and 1,25-dihydroxyvitamin D3. Proc. Natl. Acad. Sci. U.S.A. 92, 107–111. doi: 10.1073/pnas.92.1.107
Burton, J. M., Kimball, S., Vieth, R., Bar-Or, A., Dosch, H. M., Cheung, R., et al. (2010). A phase I/II dose-escalation trial of vitamin D3 and calcium in multiple sclerosis. Neurology 74, 1852–1859. doi: 10.1212/WNL.0b013e3181e1cec2
Camargo, C. A. Jr., Ingham, T., Wickens, K., Thadhani, R., Silvers, K. M., Epton, M. J., et al. (2011). Cord-blood 25-hydroxyvitamin D levels and risk of respiratory infection, wheezing, and asthma. Pediatrics 127, e180–e187. doi: 10.1542/peds.2010-0442
Camargo, C. A. Jr., Rifas-Shiman, S. L., Litonjua, A. A., Rich-Edwards, J. W., Weiss, S. T., Gold, D. R., et al. (2007). Maternal intake of vitamin D during pregnancy and risk of recurrent wheeze in children at 3 y of age. Am. J. Clin. Nutr. 85, 788–795.
Carraro, S., Giordano, G., Reniero, F., Carpi, D., Stocchero, M., Sterk, P. J., et al. (2013). Asthma severity in childhood and metabolomic profiling of breath condensate. Allergy 68, 110–117. doi: 10.1111/all.12063
Carrelli, A. L., Walker, M. D., Lowe, H., McMahon, D. J., Rundek, T., Sacco, R. L., et al. (2011). Vitamin D deficiency is associated with subclinical carotid atherosclerosis: the Northern Manhattan study. Stroke 42, 2240–2245. doi: 10.1161/STROKEAHA.110.608539
Chang, S. H., Chung, Y., and Dong, C. (2010). Vitamin D suppresses Th17 cytokine production by inducing C/EBP homologous protein (CHOP) expression. J. Biol. Chem. 285, 38751–38755. doi: 10.1074/jbc.C110.185777
Chen, W., Rogatsky, I., and Garabedian, M. J. (2006). MED14 and MED1 differentially regulate target-specific gene activation by the glucocorticoid receptor. Mol. Endocrinol. 20, 560–572. doi: 10.1210/me.2005-0318
Cheraghi, N., Dai, H., and Raghuveer, G. (2012). Vitamin D deficiency is associated with atherosclerosis-promoting risk factor clustering but not vascular damage in children. Med. Sci. Monit. 18, CR687–CR692. doi: 10.12659/MSM.883593
Chinellato, I., Piazza, M., Sandri, M., Peroni, D. G., Cardinale, F., Piacentini, G. L., et al. (2011b). Serum vitamin D levels and exercise-induced bronchoconstriction in children with asthma. Eur. Respir. J. 37, 1366–1370. doi: 10.1183/09031936.00044710
Chinellato, I., Piazza, M., Sandri, M., Peroni, D., Piacentini, G., and Boner, A. L. (2011a). Vitamin D serum levels and markers of asthma control in Italian children. J. Pediatr. 158, 437–441. doi: 10.1016/j.jpeds.2010.08.043
Cipollone, F., and Fazia, M. L. (2006). COX-2 and atherosclerosis. J. Cardiovasc. Pharmacol. 47(Suppl. 1), S26–S36. doi: 10.1097/00005344-200605001-00006
Cippitelli, M., and Santoni, A. (1998). Vitamin D3: a transcriptional modulator of the interferon-gamma gene. Eur. J. Immunol. 28, 3017-3030.
Cohen, M. L., Douvdevani, A., Chaimovitz, C., and Shany, S. (2001). Regulation of TNF-alpha by 1alpha,25-dihydroxyvitamin D3 in human macrophages from CAPD patients. Kidney Int. 59, 69–75. doi: 10.1046/j.1523-1755.2001.00467.x
Cohen-Lahav, M., Shany, S., Tobvin, D., Chaimovitz, C., and Douvdevani, A. (2006). Vitamin D decreases NFkappaB activity by increasing IkappaBalpha levels. Nephrol. Dial. Transplant. 21, 889–897. doi: 10.1093/ndt/gfi254
Colin, E. M., Asmawidjaja, P. S., Van Hamburg, J. P., Mus, A. M., Van Driel, M., Hazes, J. M., et al. (2010). 1,25-dihydroxyvitamin D3 modulates Th17 polarization and interleukin-22 expression by memory T cells from patients with early rheumatoid arthritis. Arthritis Rheum. 62, 132–142. doi: 10.1002/art.25043
Correale, J., Ysrraelit, M. C., and Gaitan, M. I. (2009). Immunomodulatory effects of Vitamin D in multiple sclerosis. Brain 132, 1146–1160. doi: 10.1093/brain/awp033
Correale, J., Ysrraelit, M. C., and Gaitan, M. I. (2010). Gender differences in 1,25 dihydroxyvitamin D3 immunomodulatory effects in multiple sclerosis patients and healthy subjects. J. Immunol. 185, 4948–4958. doi: 10.4049/jimmunol.1000588
Crispin, J. C., Martinez, A., De Pablo, P., Velasquillo, C., and Alcocer-Varela, J. (1998). Participation of the CD69 antigen in the T-cell activation process of patients with systemic lupus erythematosus. Scand. J. Immunol. 48, 196–200. doi: 10.1046/j.1365-3083.1998.00366.x
Cutolo, M. (1999). Macrophages as effectors of the immunoendocrinologic interactions in autoimmune rheumatic diseases. Ann. N.Y. Acad. Sci. 876, 32–41. discussion: 41–32.
Dallaporta, M., Pecchi, E., Thirion, S., Jean, A., and Troadec, J. D. (2010). Toward the management of inflammation: recent developments of mPGES-1 inhibitors. Recent Pat. CNS Drug Discov. 5, 70–80. doi: 10.2174/157488910789753549
D'Ambrosio, D., Cippitelli, M., Cocciolo, M. G., Mazzeo, D., Di Lucia, P., Lang, R., et al. (1998). Inhibition of IL-12 production by 1,25-dihydroxyvitamin D3. Involvement of NF-kappaB downregulation in transcriptional repression of the p40 gene. J. Clin. Invest. 101, 252–262. doi: 10.1172/JCI1050
Deckx, N., Lee, W. P., Berneman, Z. N., and Cools, N. (2013). Neuroendocrine Immunoregulation in Multiple Sclerosis. Clin. Dev. Immunol. 2013:705232. doi: 10.1155/2013/705232
Devereux, G., Litonjua, A. A., Turner, S. W., Craig, L. C., McNeill, G., Martindale, S., et al. (2007). Maternal vitamin D intake during pregnancy and early childhood wheezing. Am. J. Clin. Nutr. 85, 853–859.
Diaz, L., Noyola-Martinez, N., Barrera, D., Hernandez, G., Avila, E., Halhali, A., et al. (2009). Calcitriol inhibits TNF-alpha-induced inflammatory cytokines in human trophoblasts. J. Reprod. Immunol. 81, 17–24. doi: 10.1016/j.jri.2009.02.005
Dimeloe, S., Richards, D. F., Urry, Z. L., Gupta, A., Stratigou, V., Farooque, S., et al. (2012). 1alpha,25-dihydroxyvitamin D3 promotes CD200 expression by human peripheral and airway-resident T cells. Thorax 67, 574–581. doi: 10.1136/thoraxjnl-2011-200651
Ding, N., Yu, R. T., Subramaniam, N., Sherman, M. H., Wilson, C., Rao, R., et al. (2013). A vitamin D receptor/SMAD genomic circuit gates hepatic fibrotic response. Cell 153, 601–613. doi: 10.1016/j.cell.2013.03.028
Di Rosa, M., Malaguarnera, G., De Gregorio, C., Palumbo, M., Nunnari, G., and Malaguarnera, L. (2012). Immuno-modulatory effects of vitamin D3 in human monocyte and macrophages. Cell. Immunol. 280, 36–43. doi: 10.1016/j.cellimm.2012.10.009
Dong, J. Y., Zhang, W. G., Chen, J. J., Zhang, Z. L., Han, S. F., and Qin, L. Q. (2013). Vitamin D intake and risk of type 1 diabetes: a meta-analysis of observational studies. Nutrients 5, 3551–3562. doi: 10.3390/nu5093551
Duque, J., Fresno, M., and Iniguez, M. A. (2005). Expression and function of the nuclear factor of activated T cells in colon carcinoma cells: involvement in the regulation of cyclooxygenase-2. J. Biol. Chem. 280, 8686–8693. doi: 10.1074/jbc.M413076200
Ehlayel, M. S., Bener, A., and Sabbah, A. (2011). Is high prevalence of vitamin D deficiency evidence for asthma and allergy risks? Eur. Ann. Allergy Clin. Immunol. 43, 81–88.
Eleftheriadis, T., Antoniadi, G., Liakopoulos, V., Kartsios, C., Stefanidis, I., and Galaktidou, G. (2010). Paricalcitol reduces basal and lipopolysaccharide-induced (LPS) TNF-alpha and IL-8 production by human peripheral blood mononuclear cells. Int. Urol. Nephrol. 42, 181–185. doi: 10.1007/s11255-009-9541-1
El-Shehaby, A. M., El-Khatib, M. M., Marzouk, S., and Battah, A. A. (2013). Relationship of BsmI polymorphism of vitamin D receptor gene with left ventricular hypertrophy and atherosclerosis in hemodialysis patients. Scand. J. Clin. Lab. Invest. 73, 75–81. doi: 10.3109/00365513.2012.743163
Equils, O., Naiki, Y., Shapiro, A. M., Michelsen, K., Lu, D., Adams, J., et al. (2006). 1,25-Dihydroxyvitamin D inhibits lipopolysaccharide-induced immune activation in human endothelial cells. Clin. Exp. Immunol. 143, 58–64. doi: 10.1111/j.1365-2249.2005.02961.x
Erkkola, M., Kaila, M., Nwaru, B. I., Kronberg-Kippila, C., Ahonen, S., Nevalainen, J., et al. (2009). Maternal vitamin D intake during pregnancy is inversely associated with asthma and allergic rhinitis in 5-year-old children. Clin. Exp. Allergy 39, 875–882. doi: 10.1111/j.1365-2222.2009.03234.x
Fagan, D. L., Prehn, J. L., Adams, J. S., and Jordan, S. C. (1991). The human myelomonocytic cell line U-937 as a model for studying alterations in steroid-induced monokine gene expression: marked enhancement of lipopolysaccharide-stimulated interleukin-1 beta messenger RNA levels by 1,25-dihydroxyvitamin D3. Mol. Endocrinol. 5, 179–186. doi: 10.1210/mend-5-2-179
Fahmi, H. (2004). mPGES-1 as a novel target for arthritis. Curr. Opin. Rheumatol. 16, 623–627. doi: 10.1097/01.bor.0000129664.81052.8e
Fang, W. L., Gao, L. B., Liang, W. B., Xue, H., Bai, P., Lv, M. L., et al. (2009). Association analysis of vitamin D receptor gene polymorphisms in chinese population with asthma. Iran. J. Allergy Asthma Immunol. 8, 141–147.
Feng, X., Lv, C., Wang, F., Gan, K., Zhang, M., and Tan, W. (2013). Modulatory effect of 1,25-dihydroxyvitamin D 3 on IL1 beta -induced RANKL, OPG, TNF alpha, and IL-6 expression in human rheumatoid synoviocyte MH7A. Clin. Dev. Immunol. 2013:160123. doi: 10.1155/2013/160123
Fernandez-Herrera, J., Fernandez-Ruiz, E., Lopez-Cabrera, M., Garcia-Diez, A., Sanchez-Madrid, F., and Gonzalez-Amaro, R. (1996). CD69 expression and tumour necrosis factor-alpha immunoreactivity in the inflammatory cell infiltrate of halo naevi. Br. J. Dermatol. 134, 388–393. doi: 10.1111/j.1365-2133.1996.tb16219.x
Flanders, K. C. (2004). Smad3 as a mediator of the fibrotic response. Int. J. Exp. Pathol. 85, 47–64. doi: 10.1111/j.0959-9673.2004.00377.x
Franchi, B., Piazza, M., Sandri, M., Mazzei, F., Maffeis, C., and Boner, A. L. (2013). Vitamin D at the onset of type 1 diabetes in Italian children. Eur. J. Pediatr. 173, 477–482. doi: 10.1007/s00431-013-2204-3
Frankenberger, M., Pforte, A., Sternsdorf, T., Passlick, B., Baeuerle, P. A., and Ziegler-Heitbrock, H. W. (1994). Constitutive nuclear NF-kappa B in cells of the monocyte lineage. Biochem. J. 304(Pt 1), 87–94.
Freishtat, R. J., Iqbal, S. F., Pillai, D. K., Klein, C. J., Ryan, L. M., Benton, A. S., et al. (2010). High prevalence of vitamin D deficiency among inner-city African American youth with asthma in Washington, DC. J. Pediatr. 156, 948–952. doi: 10.1016/j.jpeds.2009.12.033
Fukuoka, M., Ogino, Y., Sato, H., Ohta, T., and Komoriya, K. (1998). Regulation of RANTES and IL-8 production in normal human dermal fibroblasts by active vitamin D3 (tacalcitol). Br. J. Pharmacol. 124, 1433–1438. doi: 10.1038/sj.bjp.0701988
Gale, C. R., Robinson, S. M., Harvey, N. C., Javaid, M. K., Jiang, B., Martyn, C. N., et al. (2008). Maternal vitamin D status during pregnancy and child outcomes. Eur. J. Clin. Nutr. 62, 68–77. doi: 10.1038/sj.ejcn.1602680
Garg, M., Lubel, J. S., Sparrow, M. P., Holt, S. G., and Gibson, P. R. (2012). Review article: vitamin D and inflammatory bowel disease–established concepts and future directions. Aliment. Pharmacol. Ther. 36, 324–344. doi: 10.1111/j.1365-2036.2012.05181.x
Gatenby, P., Lucas, R., and Swaminathan, A. (2013). Vitamin D deficiency and risk for rheumatic diseases: an update. Curr. Opin. Rheumatol. 25, 184–191. doi: 10.1097/BOR.0b013e32835cfc16
Gergen, P. J., Teach, S. J., Mitchell, H. E., Freishtat, R. F., Calatroni, A., Matsui, E., et al. (2013). Lack of a relation between serum 25-hydroxyvitamin D concentrations and asthma in adolescents. Am. J. Clin. Nutr. 97, 1228–1234. doi: 10.3945/ajcn.112.046961
Gessl, A., and Waldhausl, W. (1998). Elevated CD69 expression on naive peripheral blood T-cells in hyperthyroid Graves' disease and autoimmune thyroiditis: discordant effect of methimazole on HLA-DR and CD69. Clin. Immunol. Immunopathol. 87, 168–175. doi: 10.1006/clin.1998.4524
Ginde, A. A., Mansbach, J. M., and Camargo, C. A. Jr. (2009). Vitamin D, respiratory infections, and asthma. Curr. Allergy Asthma Rep. 9, 81–87. doi: 10.1007/s11882-009-0012-7
Goldberg, P., Fleming, M. C., and Picard, E. H. (1986). Multiple sclerosis: decreased relapse rate through dietary supplementation with calcium, magnesium and vitamin D. Med. Hypotheses 21, 193–200. doi: 10.1016/0306-9877(86)90010-1
Goleva, E., Searing, D. A., Jackson, L. P., Richers, B. N., and Leung, D. Y. (2012). Steroid requirements and immune associations with vitamin D are stronger in children than adults with asthma. J. Allergy Clin. Immunol. 129, 1243–1251. doi: 10.1016/j.jaci.2012.01.044
Golovko, O., Nazarova, N., and Tuohimaa, P. (2005). Vitamin D-induced up-regulation of tumour necrosis factor alpha (TNF-alpha) in prostate cancer cells. Life Sci. 77, 562–577. doi: 10.1016/j.lfs.2004.10.072
Gorman, S., Tan, D. H., Lambert, M. J., Scott, N. M., Judge, M. A., and Hart, P. H. (2012). Vitamin D(3) deficiency enhances allergen-induced lymphocyte responses in a mouse model of allergic airway disease. Pediatr. Allergy Immunol. 23, 83–87. doi: 10.1111/j.1399-3038.2011.01146.x
Gupta, A., Bush, A., Hawrylowicz, C., and Saglani, S. (2012a). Vitamin D and asthma in children. Paediatr. Respir. Rev. 13, 236–243. quiz 243. doi: 10.1016/j.prrv.2011.07.003
Gupta, A., Dimeloe, S., Richards, D. F., Bush, A., Saglani, S., and Hawrylowicz, C. M. (2012b). Vitamin D binding protein and asthma severity in children. J. Allergy Clin. Immunol. 129, 1669–1671. doi: 10.1016/j.jaci.2012.02.017
Gynther, P., Toropainen, S., Matilainen, J. M., Seuter, S., Carlberg, C., and Vaisanen, S. (2011). Mechanism of 1alpha,25-dihydroxyvitamin D(3)-dependent repression of interleukin-12B. Biochim. Biophys. Acta 1813, 810–818. doi: 10.1016/j.bbamcr.2011.01.037
Haeggstrom, J. Z., and Funk, C. D. (2011). Lipoxygenase and leukotriene pathways: biochemistry, biology, and roles in disease. Chem. Rev. 111, 5866–5898. doi: 10.1021/cr200246d
Hakim, I., and Bar-Shavit, Z. (2003). Modulation of TNF-alpha expression in bone marrow macrophages: involvement of vitamin D response element. J. Cell. Biochem. 88, 986–998. doi: 10.1002/jcb.10453
Halder, S. K., Goodwin, J. S., and Al-Hendy, A. (2011). 1,25-Dihydroxyvitamin D3 reduces TGF-beta3-induced fibrosis-related gene expression in human uterine leiomyoma cells. J. Clin. Endocrinol. Metab. 96, E754–E762. doi: 10.1210/jc.2010-2131
Han, S. H., Jeon, J. H., Ju, H. R., Jung, U., Kim, K. Y., Yoo, H. S., et al. (2003). VDUP1 upregulated by TGF-beta1 and 1,25-dihydorxyvitamin D3 inhibits tumor cell growth by blocking cell-cycle progression. Oncogene 22, 4035–4046. doi: 10.1038/sj.onc.1206610
Harant, H., Wolff, B., and Lindley, I. J. (1998). 1Alpha,25-dihydroxyvitamin D3 decreases DNA binding of nuclear factor-kappaB in human fibroblasts. FEBS Lett. 436, 329–334. doi: 10.1016/S0014-5793(98)01153-3
Harizi, H., Corcuff, J. B., and Gualde, N. (2008). Arachidonic-acid-derived eicosanoids: roles in biology and immunopathology. Trends Mol. Med. 14, 461–469. doi: 10.1016/j.molmed.2008.08.005
Harle, D., Radmark, O., Samuelsson, B., and Steinhilber, D. (1998). Calcitriol and transforming growth factor-beta upregulate 5-lipoxygenase mRNA expression by increasing gene transcription and mRNA maturation. Eur. J. Biochem. 254, 275–281. doi: 10.1046/j.1432-1327.1998.2540275.x
Heikkinen, S., Vaisanen, S., Pehkonen, P., Seuter, S., Benes, V., and Carlberg, C. (2011). Nuclear hormone 1alpha,25-dihydroxyvitamin D3 elicits a genome-wide shift in the locations of VDR chromatin occupancy. Nucleic Acids Res. 39, 9181–9193. doi: 10.1093/nar/gkr654
Heine, G., Niesner, U., Chang, H. D., Steinmeyer, A., Zugel, U., Zuberbier, T., et al. (2008). 1,25-dihydroxyvitamin D(3) promotes IL-10 production in human B cells. Eur. J. Immunol. 38, 2210–2218. doi: 10.1002/eji.200838216
Hidaka, M., Wakabayashi, I., Takeda, Y., and Fukuzawa, K. (2013). Vitamin D(3) derivatives increase soluble CD14 release through ERK1/2 activation and decrease IL-8 production in intestinal epithelial cells. Eur. J. Pharmacol. 721, 305–312. doi: 10.1016/j.ejphar.2013.09.014
Higashimoto, Y., Ohata, M., Iwamoto, Y., Fujimoto, H., Uetani, K., Suruda, T., et al. (1995). Stimulatory effect of 1 alpha,25-dihydroxyvitamin D3 on mouse alveolar macrophage tumor necrosis factor-alpha production in vitro: involvement of protein kinase C and Ca2+/calmodulin-dependent kinase. Respiration 62, 89–94. doi: 10.1159/000196398
Hodler, B., Evequoz, V., Trechsel, U., Fleisch, H., and Stadler, B. (1985). Influence of vitamin D3 metabolites on the production of interleukins 1,2 and 3. Immunobiology 170, 256–269. doi: 10.1016/S0171-2985(85)80075-9
Hollams, E. M., Hart, P. H., Holt, B. J., Serralha, M., Parsons, F., De Klerk, N. H., et al. (2011). Vitamin D and atopy and asthma phenotypes in children: a longitudinal cohort study. Eur. Respir. J. 38, 1320–1327. doi: 10.1183/09031936.00029011
Hullett, D. A., Laeseke, P. F., Malin, G., Nessel, R., Sollinger, H. W., and Becker, B. N. (2005). Prevention of chronic allograft nephropathy with vitamin D. Transpl. Int. 18, 1175–1186. doi: 10.1111/j.1432-2277.2005.00187.x
Hypponen, E., Sovio, U., Wjst, M., Patel, S., Pekkanen, J., Hartikainen, A. L., et al. (2004). Infant vitamin d supplementation and allergic conditions in adulthood: northern Finland birth cohort 1966. Ann. N.Y. Acad. Sci. 1037, 84–95. doi: 10.1196/annals.1337.013
Inoue, M., Matsui, T., Nishibu, A., Nihei, Y., Iwatsuki, K., and Kaneko, F. (1998). Regulatory effects of 1alpha,25-dihydroxyvitamin D3 on inflammatory responses in psoriasis. Eur. J. Dermatol. 8, 16–20.
Ito, I., Waku, T., Aoki, M., Abe, R., Nagai, Y., Watanabe, T., et al. (2013). A nonclassical vitamin D receptor pathway suppresses renal fibrosis. J. Clin. Invest. 123, 4579–4594. doi: 10.1172/JCI67804
Jain, S. K., and Micinski, D. (2013). Vitamin D upregulates glutamate cysteine ligase and glutathione reductase, and GSH formation, and decreases ROS and MCP-1 and IL-8 secretion in high-glucose exposed U937 monocytes. Biochem. Biophys. Res. Commun. 437, 7–11. doi: 10.1016/j.bbrc.2013.06.004
Jamshidi, F., Zhang, J., Harrison, J. S., Wang, X., and Studzinski, G. P. (2008). Induction of differentiation of human leukemia cells by combinations of COX inhibitors and 1,25-dihydroxyvitamin D3 involves Raf1 but not Erk 1/2 signaling. Cell Cycle 7, 917–924. doi: 10.4161/cc.7.7.5620
Jono, S., Nishizawa, Y., Shioi, A., and Morii, H. (1998). 1,25-Dihydroxyvitamin D3 increases in vitro vascular calcification by modulating secretion of endogenous parathyroid hormone-related peptide. Circulation 98, 1302–1306. doi: 10.1161/01.CIR.98.13.1302
Jordan, S. C., Toyoda, M., Prehn, J., Lemire, J. M., Sakai, R., and Adams, J. S. (1989). 1,25-Dihydroxyvitamin-D3 regulation of interleukin-2 and interleukin-2 receptor levels and gene expression in human T cells. Mol. Immunol. 26, 979–984. doi: 10.1016/0161-5890(89)90116-8
Joshi, S., Pantalena, L. C., Liu, X. K., Gaffen, S. L., Liu, H., Rohowsky-Kochan, C., et al. (2011). 1,25-dihydroxyvitamin D(3) ameliorates Th17 autoimmunity via transcriptional modulation of interleukin-17A. Mol. Cell. Biol. 31, 3653–3669. doi: 10.1128/MCB.05020-11
Kankova, M., Luini, W., Pedrazzoni, M., Riganti, F., Sironi, M., Bottazzi, B., et al. (1991). Impairment of cytokine production in mice fed a vitamin D3-deficient diet. Immunology 73, 466–471.
Karin, M., and Lin, A. (2002). NF-kappaB at the crossroads of life and death. Nat. Immunol. 3, 221–227. doi: 10.1038/ni0302-221
Kim, C. S., Joo, S. Y., Lee, K. E., Choi, J. S., Bae, E. H., Ma, S. K., et al. (2013). Paricalcitol attenuates 4-hydroxy-2-hexenal-induced inflammation and epithelial-mesenchymal transition in human renal proximal tubular epithelial cells. PLoS ONE 8:e63186. doi: 10.1371/journal.pone.0063186
Kim, H. J., Abdelkader, N., Katz, M., and McLane, J. A. (1992). 1,25-Dihydroxy-vitamin-D3 enhances antiproliferative effect and transcription of TGF-beta1 on human keratinocytes in culture. J. Cell. Physiol. 151, 579–587. doi: 10.1002/jcp.1041510318
Knip, M., and Simell, O. (2012). Environmental triggers of type 1 diabetes. Cold Spring Harb. Perspect. Med. 2:a007690. doi: 10.1101/cshperspect.a007690
Koizumi, H., Kaplan, A., Shimizu, T., and Ohkawara, A. (1997). 1,25-Dihydroxyvitamin D3 and a new analogue, 22-oxacalcitriol, modulate proliferation and interleukin-8 secretion of normal human keratinocytes. J. Dermatol. Sci. 15, 207–213. doi: 10.1016/S0923-1811(97)00609-9
Kong, J., Grando, S. A., and Li, Y. C. (2006). Regulation of IL-1 family cytokines IL-1alpha, IL-1 receptor antagonist, and IL-18 by 1,25-dihydroxyvitamin D3 in primary keratinocytes. J. Immunol. 176, 3780–3787. doi: 10.4049/jimmunol.176.6.3780
Korf, H., Wenes, M., Stijlemans, B., Takiishi, T., Robert, S., Miani, M., et al. (2012). 1,25-Dihydroxyvitamin D3 curtails the inflammatory and T cell stimulatory capacity of macrophages through an IL-10-dependent mechanism. Immunobiology 217, 1292–1300. doi: 10.1016/j.imbio.2012.07.018
Krobtrakulchai, W., Praikanahok, J., Visitsunthorn, N., Vichyanond, P., Manonukul, K., Pratumvinit, B., et al. (2013). The effect of vitamin d status on pediatric asthma at a university hospital, Thailand. Allergy Asthma Immunol. Res. 5, 289–294. doi: 10.4168/aair.2013.5.5.289
Kudejko, J. (1968). Effect of vitamin D 3 and induced calciphylaxis on atherosclerosis of rabbits treated with cholesterol and calcium gluconate. Gerontologia 14, 35–44. doi: 10.1159/000211640
Kudo, K., Hasegawa, S., Suzuki, Y., Hirano, R., Wakiguchi, H., Kittaka, S., et al. (2012). 1alpha,25-Dihydroxyvitamin D(3) inhibits vascular cellular adhesion molecule-1 expression and interleukin-8 production in human coronary arterial endothelial cells. J. Steroid Biochem. Mol. Biol. 132, 290–294. doi: 10.1016/j.jsbmb.2012.07.003
Kull, I., Bergstrom, A., Melen, E., Lilja, G., Van Hage, M., Pershagen, G., et al. (2006). Early-life supplementation of vitamins A and D, in water-soluble form or in peanut oil, and allergic diseases during childhood. J. Allergy Clin. Immunol. 118, 1299–1304. doi: 10.1016/j.jaci.2006.08.022
Kunitomo, M., Kinoshita, K., and Bando, Y. (1981). Experimental atherosclerosis in rats fed a vitamin D, cholesterol-rich diet. J. Pharmacobiodyn. 4, 718–723. doi: 10.1248/bpb1978.4.718
Kuo, Y. T., Kuo, C. H., Lam, K. P., Chu, Y. T., Wang, W. L., Huang, C. H., et al. (2010). Effects of vitamin D3 on expression of tumor necrosis factor-alpha and chemokines by monocytes. J. Food Sci. 75, H200–H204. doi: 10.1111/j.1750-3841.2010.01704.x
Lacey, D. L., Erdmann, J. M., and Tan, H. L. (1993a). 1,25-Dihydroxyvitamin D3 increases type 1 interleukin-1 receptor expression in a murine T cell line. J. Cell. Biochem. 52, 159–170. doi: 10.1002/jcb.240520208
Lacey, D. L., Erdmann, J. M., Tan, H. L., and Ohara, J. (1993b). Murine osteoblast interleukin 4 receptor expression: upregulation by 1,25 dihydroxyvitamin D3. J. Cell. Biochem. 53, 122–134. doi: 10.1002/jcb.240530205
Lagishetty, V., Liu, N. Q., and Hewison, M. (2011). Vitamin D metabolism and innate immunity. Mol. Cell. Endocrinol. 347, 97–105. doi: 10.1016/j.mce.2011.04.015
Lan, H. Y. (2011). Diverse roles of TGF-beta/Smads in renal fibrosis and inflammation. Int. J. Biol. Sci. 7, 1056–1067. doi: 10.7150/ijbs.7.1056
Larsen, C. G., Kristensen, M., Paludan, K., Deleuran, B., Thomsen, M. K., Zachariae, C., et al. (1991). 1,25(OH)2-D3 is a potent regulator of interleukin-1 induced interleukin-8 expression and production. Biochem. Biophys. Res. Commun. 176, 1020–1026. doi: 10.1016/0006-291X(91)90384-J
Lee, B. N., Kim, T. H., Jun, J. B., Yoo, D. H., Woo, J. H., Choi, S. J., et al. (2011). Upregulation of interleukin-1beta production by 1,25-dihydroxyvitamin D(3) in activated human macrophages. Mol. Biol. Rep. 38, 2193–2201. doi: 10.1007/s11033-010-0348-z
Lee, S. B., and Kalluri, R. (2010). Mechanistic connection between inflammation and fibrosis. Kidney Int. Suppl. 78, S22–S26. doi: 10.1038/ki.2010.418
Lemire, J. M., Archer, D. C., Beck, L., and Spiegelberg, H. L. (1995). Immunosuppressive actions of 1,25-dihydroxyvitamin D3: preferential inhibition of Th1 functions. J. Nutr. 125, 1704s–1708s.
Li, B., Baylink, D. J., Deb, C., Zannetti, C., Rajaallah, F., Xing, W., et al. (2013). 1,25-Dihydroxyvitamin D3 suppresses TLR8 expression and TLR8-mediated inflammatory responses in monocytes in vitro and experimental autoimmune encephalomyelitis in vivo. PLoS ONE 8:e58808. doi: 10.1371/journal.pone.0058808
Li, F., Jiang, L., Willis-Owen, S. A., Zhang, Y., and Gao, J. (2011a). Vitamin D binding protein variants associate with asthma susceptibility in the Chinese Han population. BMC Med. Genet. 12:103. doi: 10.1186/1471-2350-12-103
Li, F., Peng, M., Jiang, L., Sun, Q., Zhang, K., Lian, F., et al. (2011b). Vitamin D deficiency is associated with decreased lung function in Chinese adults with asthma. Respiration 81, 469–475. doi: 10.1159/000322008
Li, M. O., and Flavell, R. A. (2008). Contextual regulation of inflammation: a duet by transforming growth factor-beta and interleukin-10. Immunity 28, 468–476. doi: 10.1016/j.immuni.2008.03.003
Li, Y. C., Kong, J., Wei, M., Chen, Z. F., Liu, S. Q., and Cao, L. P. (2002). 1,25-Dihydroxyvitamin D(3) is a negative endocrine regulator of the renin-angiotensin system. J. Clin. Invest. 110, 229–238. doi: 10.1172/JCI0215219
Liu, N. Q., Kaplan, A. T., Lagishetty, V., Ouyang, Y. B., Ouyang, Y., Simmons, C. F., et al. (2011). Vitamin D and the regulation of placental inflammation. J. Immunol. 186, 5968–5974. doi: 10.4049/jimmunol.1003332
Liu, X., Nelson, A., Wang, X., Farid, M., Gunji, Y., Ikari, J., et al. (2014). Vitamin d modulates prostaglandin e2 synthesis and degradation in human lung fibroblasts. Am. J. Respir. Cell Mol. Biol. 50, 40–50. doi: 10.1165/rcmb.2013-0211OC
Lyakh, L. A., Sanford, M., Chekol, S., Young, H. A., and Roberts, A. B. (2005). TGF-beta and vitamin D3 utilize distinct pathways to suppress IL-12 production and modulate rapid differentiation of human monocytes into CD83+ dendritic cells. J. Immunol. 174, 2061–2070. doi: 10.4049/jimmunol.174.4.2061
Maalmi, H., Sassi, F. H., Berraies, A., Ammar, J., Hamzaoui, K., and Hamzaoui, A. (2013). Association of vitamin D receptor gene polymorphisms with susceptibility to asthma in Tunisian children: a case control study. Hum. Immunol. 74, 234–240. doi: 10.1016/j.humimm.2012.11.005
Magnus, M. C., Stene, L. C., Haberg, S. E., Nafstad, P., Stigum, H., London, S. J., et al. (2013). Prospective study of maternal mid-pregnancy 25-hydroxyvitamin D Level and early childhood respiratory disorders. Paediatr. Perinat. Epidemiol. 27, 532–541. doi: 10.1111/ppe.12080
Majak, P., Jerzynska, J., Smejda, K., Stelmach, I., Timler, D., and Stelmach, W. (2012). Correlation of vitamin D with Foxp3 induction and steroid-sparing effect of immunotherapy in asthmatic children. Ann. Allergy Asthma Immunol. 109, 329–335. doi: 10.1016/j.anai.2012.08.002
Majak, P., Olszowiec-Chlebna, M., Smejda, K., and Stelmach, I. (2011). Vitamin D supplementation in children may prevent asthma exacerbation triggered by acute respiratory infection. J. Allergy Clin. Immunol. 127, 1294–1296. doi: 10.1016/j.jaci.2010.12.016
Marcotorchino, J., Gouranton, E., Romier, B., Tourniaire, F., Astier, J., Malezet, C., et al. (2012). Vitamin D reduces the inflammatory response and restores glucose uptake in adipocytes. Mol. Nutr. Food Res. 56, 1771–1782. doi: 10.1002/mnfr.201200383
Matilainen, J. M., Husso, T., Toropainen, S., Seuter, S., Turunen, M. P., Gynther, P., et al. (2010a). Primary effect of 1alpha,25(OH)(2)D(3) on IL-10 expression in monocytes is short-term down-regulation. Biochim. Biophys. Acta 1803, 1276–1286. doi: 10.1016/j.bbamcr.2010.07.009
Matilainen, J. M., Rasanen, A., Gynther, P., and Vaisanen, S. (2010b). The genes encoding cytokines IL-2, IL-10 and IL-12B are primary 1alpha,25(OH)2D3 target genes. J. Steroid Biochem. Mol. Biol. 121, 142–145. doi: 10.1016/j.jsbmb.2010.03.020
Matsui, T., Takahashi, R., Nakao, Y., Koizumi, T., Katakami, Y., Mihara, K., et al. (1986). 1,25-Dihydroxyvitamin D3-regulated expression of genes involved in human T-lymphocyte proliferation and differentiation. Cancer Res. 46, 5827–5831.
McNally, P., Coughlan, C., Bergsson, G., Doyle, M., Taggart, C., Adorini, L., et al. (2011). Vitamin D receptor agonists inhibit pro-inflammatory cytokine production from the respiratory epithelium in cystic fibrosis. J. Cyst. Fibros. 10, 428–434. doi: 10.1016/j.jcf.2011.06.013
Menon, J., Maranda, L., and Nwosu, B. U. (2012). Serum 25-hydroxyvitamin D levels do not correlate with asthma severity in a case-controlled study of children and adolescents. J. Pediatr. Endocrinol. Metab. 25, 673–679. doi: 10.1515/jpem-2012-0143
Meyer, M. B., Goetsch, P. D., and Pike, J. W. (2012). VDR/RXR and TCF4/beta-catenin cistromes in colonic cells of colorectal tumor origin: impact on c-FOS and c-MYC gene expression. Mol. Endocrinol. 26, 37–51. doi: 10.1210/me.2011-1109
Miodovnik, M., Koren, R., Ziv, E., and Ravid, A. (2012). The inflammatory response of keratinocytes and its modulation by vitamin D: the role of MAPK signaling pathways. J. Cell. Physiol. 227, 2175–2183. doi: 10.1002/jcp.22951
Mok, C. C., Birmingham, D. J., Leung, H. W., Hebert, L. A., Song, H., and Rovin, B. H. (2012). Vitamin D levels in Chinese patients with systemic lupus erythematosus: relationship with disease activity, vascular risk factors and atherosclerosis. Rheumatology (Oxf.) 51, 644–652. doi: 10.1093/rheumatology/ker212
Moniaga, C. S., Egawa, G., Miyachi, Y., and Kabashima, K. (2013). Calcipotriol modulates IL-22 receptor expression and keratinocyte proliferation in IL-22-induced epidermal hyperplasia. J. Dermatol. Sci. 71, 76–77. doi: 10.1016/j.jdermsci.2013.03.011
Moore, K. J., Sheedy, F. J., and Fisher, E. A. (2013). Macrophages in atherosclerosis: a dynamic balance. Nat. Rev. Immunol. 13, 709–721. doi: 10.1038/nri3520
Morales, E., Romieu, I., Guerra, S., Ballester, F., Rebagliato, M., Vioque, J., et al. (2012). Maternal vitamin D status in pregnancy and risk of lower respiratory tract infections, wheezing, and asthma in offspring. Epidemiology 23, 64–71. doi: 10.1097/EDE.0b013e31823a44d3
Moreno, J., Krishnan, A. V., Swami, S., Nonn, L., Peehl, D. M., and Feldman, D. (2005). Regulation of prostaglandin metabolism by calcitriol attenuates growth stimulation in prostate cancer cells. Cancer Res. 65, 7917–7925. doi: 10.1158/0008-5472.can-05-1435
Muller, K., and Bendtzen, K. (1992). Inhibition of human T lymphocyte proliferation and cytokine production by 1,25-dihydroxyvitamin D3. Differential effects on CD45RA+ and CD45R0+ cells. Autoimmunity 14, 37–43. doi: 10.3109/08916939309077355
Muller, K., Haahr, P. M., Diamant, M., Rieneck, K., Kharazmi, A., and Bendtzen, K. (1992). 1,25-Dihydroxyvitamin D3 inhibits cytokine production by human blood monocytes at the post-transcriptional level. Cytokine 4, 506–512. doi: 10.1016/1043-4666(92)90012-G
Muller, M. R., and Rao, A. (2010). NFAT, immunity and cancer: a transcription factor comes of age. Nat. Rev. Immunol. 10, 645–656. doi: 10.1038/nri2818
Munger, K. L., Levin, L. I., Hollis, B. W., Howard, N. S., and Ascherio, A. (2006). Serum 25-hydroxyvitamin D levels and risk of multiple sclerosis. JAMA 296, 2832–2838. doi: 10.1001/jama.296.23.2832
Munger, K. L., Zhang, S. M., O'Reilly, E., Hernan, M. A., Olek, M. J., Willett, W. C., et al. (2004). Vitamin D intake and incidence of multiple sclerosis. Neurology 62, 60–65. doi: 10.1212/01.WNL.0000101723.79681.38
Murakami, M., Naraba, H., Tanioka, T., Semmyo, N., Nakatani, Y., Kojima, F., et al. (2000). Regulation of prostaglandin E2 biosynthesis by inducible membrane-associated prostaglandin E2 synthase that acts in concert with cyclooxygenase-2. J. Biol. Chem. 275, 32783–32792. doi: 10.1074/jbc.M003505200
Mutt, S. J., Karhu, T., Lehtonen, S., Lehenkari, P., Carlberg, C., Saarnio, J., et al. (2012). Inhibition of cytokine secretion from adipocytes by 1,25-dihydroxyvitamin D(3) via the NF-kappaB pathway. FASEB J. 26, 4400–4407. doi: 10.1096/fj.12-210880
Nakagawa, K., Sasaki, Y., Kato, S., Kubodera, N., and Okano, T. (2005). 22-Oxa-1alpha,25-dihydroxyvitamin D3 inhibits metastasis and angiogenesis in lung cancer. Carcinogenesis 26, 1044–1054. doi: 10.1093/carcin/bgi049
Neve, A., Corrado, A., and Cantatore, F. P. (2013). Immunomodulatory effects of vitamin D in peripheral blood monocyte-derived macrophages from patients with rheumatoid arthritis. Clin. Exp. Med. doi: 10.1007/s10238-013-0249-2. [Epub ahead of print].
Nonn, L., Peng, L., Feldman, D., and Peehl, D. M. (2006). Inhibition of p38 by vitamin D reduces interleukin-6 production in normal prostate cells via mitogen-activated protein kinase phosphatase 5: implications for prostate cancer prevention by vitamin D. Cancer Res. 66, 4516–4524. doi: 10.1158/0008-5472.CAN-05-3796
Oh, J., Riek, A. E., Weng, S., Petty, M., Kim, D., Colonna, M., et al. (2012). Endoplasmic reticulum stress controls M2 macrophage differentiation and foam cell formation. J. Biol. Chem. 287, 11629–11641. doi: 10.1074/jbc.M111.338673
Ooi, J. H., Chen, J., and Cantorna, M. T. (2012). Vitamin D regulation of immune function in the gut: why do T cells have vitamin D receptors? Mol. Aspects Med. 33, 77–82. doi: 10.1016/j.mam.2011.10.014
Ozgocmen, S., Bulut, S., Ilhan, N., Gulkesen, A., Ardicoglu, O., and Ozkan, Y. (2005). Vitamin D deficiency and reduced bone mineral density in multiple sclerosis: effect of ambulatory status and functional capacity. J. Bone Miner. Metab. 23, 309–313. doi: 10.1007/s00774-005-0604-9
Panichi, V., De Pietro, S., Andreini, B., Bianchi, A. M., Migliori, M., Taccola, D., et al. (1998). Calcitriol modulates in vivo and in vitro cytokine production: a role for intracellular calcium. Kidney Int. 54, 1463–1469. doi: 10.1046/j.1523-1755.1998.00152.x
Pedersen, A. W., Holmstrom, K., Jensen, S. S., Fuchs, D., Rasmussen, S., Kvistborg, P., et al. (2009). Phenotypic and functional markers for 1alpha,25-dihydroxyvitamin D(3)-modified regulatory dendritic cells. Clin. Exp. Immunol. 157, 48–59. doi: 10.1111/j.1365-2249.2009.03961.x
Pedersen, L. B., Nashold, F. E., Spach, K. M., and Hayes, C. E. (2007). 1,25-dihydroxyvitamin D3 reverses experimental autoimmune encephalomyelitis by inhibiting chemokine synthesis and monocyte trafficking. J. Neurosci. Res. 85, 2480–2490. doi: 10.1002/jnr.21382
Penna, G., and Adorini, L. (2000). 1 Alpha,25-dihydroxyvitamin D3 inhibits differentiation, maturation, activation, and survival of dendritic cells leading to impaired alloreactive T cell activation. J. Immunol. 164, 2405–2411. doi: 10.4049/jimmunol.164.5.2405
Penna, G., Fibbi, B., Amuchastegui, S., Corsiero, E., Laverny, G., Silvestrini, E., et al. (2009). The vitamin D receptor agonist elocalcitol inhibits IL-8-dependent benign prostatic hyperplasia stromal cell proliferation and inflammatory response by targeting the RhoA/Rho kinase and NF-kappaB pathways. Prostate 69, 480–493. doi: 10.1002/pros.20896
Peric, M., Koglin, S., Kim, S. M., Morizane, S., Besch, R., Prinz, J. C., et al. (2008). IL-17A enhances vitamin D3-induced expression of cathelicidin antimicrobial peptide in human keratinocytes. J. Immunol. 181, 8504–8512. doi: 10.4049/jimmunol.181.12.8504
Petrovic, N., Knight, D. A., Bomalaski, J. S., Thompson, P. J., and Misso, N. L. (2006). Concomitant activation of extracellular signal-regulated kinase and induction of COX-2 stimulates maximum prostaglandin E2 synthesis in human airway epithelial cells. Prostaglandins Other Lipid Mediat. 81, 126–135. doi: 10.1016/j.prostaglandins.2006.08.006
Pike, K. C., Inskip, H. M., Robinson, S., Lucas, J. S., Cooper, C., Harvey, N. C., et al. (2012). Maternal late-pregnancy serum 25-hydroxyvitamin D in relation to childhood wheeze and atopic outcomes. Thorax 67, 950–956. doi: 10.1136/thoraxjnl-2012-201888
Pillai, D. K., Iqbal, S. F., Benton, A. S., Lerner, J., Wiles, A., Foerster, M., et al. (2011). Associations between genetic variants in vitamin D metabolism and asthma characteristics in young African Americans: a pilot study. J. Investig. Med. 59, 938–946. doi: 10.231/JIM.0b013e318220df41
Poon, A. H., Laprise, C., Lemire, M., Montpetit, A., Sinnett, D., Schurr, E., et al. (2004). Association of vitamin D receptor genetic variants with susceptibility to asthma and atopy. Am. J. Respir. Crit. Care Med. 170, 967–973. doi: 10.1164/rccm.200403-412OC
Portales-Perez, D., Gonzalez-Amaro, R., Abud-Mendoza, C., and Sanchez-Armass, S. (1997). Abnormalities in CD69 expression, cytosolic pH and Ca2+ during activation of lymphocytes from patients with systemic lupus erythematosus. Lupus 6, 48–56. doi: 10.1177/096120339700600107
Prabhu Anand, S., Selvaraj, P., and Narayanan, P. R. (2009). Effect of 1,25 dihydroxyvitamin D3 on intracellular IFN-gamma and TNF-alpha positive T cell subsets in pulmonary tuberculosis. Cytokine 45, 105–110. doi: 10.1016/j.cyto.2008.11.004
Prehn, J. L., Fagan, D. L., Jordan, S. C., and Adams, J. S. (1992). Potentiation of lipopolysaccharide-induced tumor necrosis factor-alpha expression by 1,25-dihydroxyvitamin D3. Blood 80, 2811–2816.
Prehn, J. L., and Jordan, S. C. (1989). Incubation of T cell or monocytic cell lines with 1,25-dihydroxyvitamin D3 before mitogen stimulation potentiates IL-2 and IL-1 beta mRNA levels. Transplant. Proc. 21, 90–91.
Qin, X., Corriere, M. A., Matrisian, L. M., and Guzman, R. J. (2006). Matrix metalloproteinase inhibition attenuates aortic calcification. Arterioscler. Thromb. Vasc. Biol. 26, 1510–1516. doi: 10.1161/01.ATV.0000225807.76419.a7
Raby, B. A., Lazarus, R., Silverman, E. K., Lake, S., Lange, C., Wjst, M., et al. (2004). Association of vitamin D receptor gene polymorphisms with childhood and adult asthma. Am. J. Respir. Crit. Care Med. 170, 1057–1065. doi: 10.1164/rccm.200404-447OC
Rachez, C., Lemon, B. D., Suldan, Z., Bromleigh, V., Gamble, M., Naar, A. M., et al. (1999). Ligand-dependent transcription activation by nuclear receptors requires the DRIP complex. Nature 398, 824–828. doi: 10.1038/19783
Ramagopalan, S. V., Heger, A., Berlanga, A. J., Maugeri, N. J., Lincoln, M. R., Burrell, A., et al. (2010). A ChIP-seq defined genome-wide map of vitamin D receptor binding: associations with disease and evolution. Genome Res. 20, 1352–1360. doi: 10.1101/gr.107920.110
Ramalho, T. C., Rocha, M., Da Cunha, E. F., and Freitas, M. P. (2009). The search for new COX-2 inhibitors: a review of 2002 - 2008 patents. Expert Opin. Ther. Pat. 19, 1193–1228. doi: 10.1517/13543770903059125
Rausch-Fan, X., Leutmezer, F., Willheim, M., Spittler, A., Bohle, B., Ebner, C., et al. (2002). Regulation of cytokine production in human peripheral blood mononuclear cells and allergen-specific th cell clones by 1alpha,25-dihydroxyvitamin D3. Int. Arch. Allergy Immunol. 128, 33–41. doi: 10.1159/000058001
Reichel, H., Koeffler, H. P., Tobler, A., and Norman, A. W. (1987). 1 alpha,25-Dihydroxyvitamin D3 inhibits gamma-interferon synthesis by normal human peripheral blood lymphocytes. Proc. Natl. Acad. Sci. U.S.A. 84, 3385–3389. doi: 10.1073/pnas.84.10.3385
Reis, J. P., Von Muhlen, D., Michos, E. D., Miller, E. R. 3rd, Appel, L. J., Araneta, M. R., et al. (2009). Serum vitamin D, parathyroid hormone levels, and carotid atherosclerosis. Atherosclerosis 207, 585–590. doi: 10.1016/j.atherosclerosis.2009.05.030
Riancho, J. A., Zarrabeitia, M. T., De Francisco, A. L., Amado, J. A., Napal, J., Arias, M., et al. (1993). Vitamin D therapy modulates cytokine secretion in patients with renal failure. Nephron 65, 364–368. doi: 10.1159/000187514
Richart, T., Li, Y., and Staessen, J. A. (2007). Renal versus extrarenal activation of vitamin D in relation to atherosclerosis, arterial stiffening, and hypertension. Am. J. Hypertens. 20, 1007–1015. doi: 10.1016/j.amjhyper.2007.03.017
Riek, A. E., Oh, J., and Bernal-Mizrachi, C. (2013a). 1,25(OH)2 vitamin D suppresses macrophage migration and reverses atherogenic cholesterol metabolism in type 2 diabetic patients. J. Steroid Biochem. Mol. Biol. 136, 309–312. doi: 10.1016/j.jsbmb.2012.12.019
Riek, A. E., Oh, J., Darwech, I., Moynihan, C. E., Bruchas, R. R., and Bernal-Mizrachi, C. (2013b). 25(OH) vitamin D suppresses macrophage adhesion and migration by downregulation of ER stress and scavenger receptor A1 in type 2 diabetes. J. Steroid Biochem. Mol. Biol. doi: 10.1016/j.jsbmb.2013.10.016. [Epub ahead of print].
Rigby, W. F., Denome, S., and Fanger, M. W. (1987). Regulation of lymphokine production and human T lymphocyte activation by 1,25-dihydroxyvitamin D3. Specific inhibition at the level of messenger RNA. J. Clin. Invest. 79, 1659–1664. doi: 10.1172/JCI113004
Rigby, W. F., Hamilton, B. J., and Waugh, M. G. (1990). 1,25-Dihydroxyvitamin D3 modulates the effects of interleukin 2 independent of IL-2 receptor binding. Cell. Immunol. 125, 396–414. doi: 10.1016/0008-8749(90)90094-8
Rigby, W. F., Stacy, T., and Fanger, M. W. (1984). Inhibition of T lymphocyte mitogenesis by 1,25-dihydroxyvitamin D3 (calcitriol). J. Clin. Invest. 74, 1451–1455. doi: 10.1172/JCI111557
Rostkowska-Nadolska, B., Sliupkas-Dyrda, E., Potyka, J., Kusmierz, D., Fraczek, M., Krecicki, T., et al. (2010). Vitamin D derivatives: calcitriol and tacalcitol inhibits interleukin-6 and interleukin-8 expression in human nasal polyp fibroblast cultures. Adv. Med. Sci. 55, 86–92. doi: 10.2478/v10039-010-0012-9
Rothers, J., Wright, A. L., Stern, D. A., Halonen, M., and Camargo, C. A. Jr. (2011). Cord blood 25-hydroxyvitamin D levels are associated with aeroallergen sensitization in children from Tucson, Arizona. J. Allergy Clin. Immunol. 128, 1093.e5–1099.e5. doi: 10.1016/j.jaci.2011.07.015
Ryynanen, J., and Carlberg, C. (2013). Primary 1,25-dihydroxyvitamin D3 response of the interleukin 8 gene cluster in human monocyte- and macrophage-like cells. PLoS ONE 8:e78170. doi: 10.1371/journal.pone.0078170
Saadi, A., Gao, G., Li, H., Wei, C., Gong, Y., and Liu, Q. (2009). Association study between vitamin D receptor gene polymorphisms and asthma in the Chinese Han population: a case-control study. BMC Med. Genet. 10:71. doi: 10.1186/1471-2350-10-71
Sachs, M. C., Brunzell, J. D., Cleary, P. A., Hoofnagle, A. N., Lachin, J. M., Molitch, M. E., et al. (2013). Circulating vitamin D metabolites and subclinical atherosclerosis in type 1 diabetes. Diabetes Care 36, 2423–2429. doi: 10.2337/dc12-2020
Saggese, G., Federico, G., Balestri, M., and Toniolo, A. (1989). Calcitriol inhibits the PHA-induced production of IL-2 and IFN-gamma and the proliferation of human peripheral blood leukocytes while enhancing the surface expression of HLA class II molecules. J. Endocrinol. Invest. 12, 329–335. doi: 10.1007/BF03349999
Schnatz, P. F., Nudy, M., O'Sullivan, D. M., Jiang, X., Cline, J. M., Kaplan, J. R., et al. (2012a). Coronary artery vitamin D receptor expression and plasma concentrations of 25-hydroxyvitamin D: their association with atherosclerosis. Menopause 19, 967–973. doi: 10.1097/gme.0b013e31824cfa8f
Schnatz, P. F., Nudy, M., O'Sullivan, D. M., Jiang, X., Cline, J. M., Kaplan, J. R., et al. (2012b). The quantification of vitamin D receptors in coronary arteries and their association with atherosclerosis. Maturitas 73, 143–147. doi: 10.1016/j.maturitas.2012.03.014
Schwalfenberg, G. K. (2011). A review of the critical role of vitamin D in the functioning of the immune system and the clinical implications of vitamin D deficiency. Mol. Nutr. Food Res. 55, 96–108. doi: 10.1002/mnfr.201000174
Searing, D. A., Zhang, Y., Murphy, J. R., Hauk, P. J., Goleva, E., and Leung, D. Y. (2010). Decreased serum vitamin D levels in children with asthma are associated with increased corticosteroid use. J. Allergy Clin. Immunol. 125, 995–1000. doi: 10.1016/j.jaci.2010.03.008
Selvaraj, P., Vidyarani, M., Alagarasu, K., Prabhu Anand, S., and Narayanan, P. R. (2008). Regulatory role of promoter and 3′ UTR variants of vitamin D receptor gene on cytokine response in pulmonary tuberculosis. J. Clin. Immunol. 28, 306–313. doi: 10.1007/s10875-007-9152-5
Shanker, J., Maitra, A., Arvind, P., Nair, J., Dash, D., Manchiganti, R., et al. (2011). Role of vitamin D levels and vitamin D receptor polymorphisms in relation to coronary artery disease: the Indian atherosclerosis research study. Coron. Artery Dis. 22, 324–332. doi: 10.1097/MCA.0b013e3283472a57
Shapira, Y., Agmon-Levin, N., and Shoenfeld, Y. (2010). Geoepidemiology of autoimmune rheumatic diseases. Nat. Rev. Rheumatol. 6, 468–476. doi: 10.1038/nrrheum.2010.86
Sheppard, D. (2006). Transforming growth factor beta: a central modulator of pulmonary and airway inflammation and fibrosis. Proc. Am. Thorac. Soc. 3, 413–417. doi: 10.1513/pats.200601-008AW
Sochorova, K., Budinsky, V., Rozkova, D., Tobiasova, Z., Dusilova-Sulkova, S., Spisek, R., et al. (2009). Paricalcitol (19-nor-1,25-dihydroxyvitamin D2) and calcitriol (1,25-dihydroxyvitamin D3) exert potent immunomodulatory effects on dendritic cells and inhibit induction of antigen-specific T cells. Clin. Immunol. 133, 69–77. doi: 10.1016/j.clim.2009.06.011
Song, G. G., Bae, S. C., and Lee, Y. H. (2012). Association between vitamin D intake and the risk of rheumatoid arthritis: a meta-analysis. Clin. Rheumatol. 31, 1733–1739. doi: 10.1007/s10067-012-2080-7
Sorg, B. L., Klan, N., Seuter, S., Dishart, D., Radmark, O., Habenicht, A., et al. (2006). Analysis of the 5-lipoxygenase promoter and characterization of a vitamin D receptor binding site. Biochim. Biophys. Acta 1761, 686–697. doi: 10.1016/j.bbalip.2006.04.005
Spach, K. M., and Hayes, C. E. (2005). Vitamin D3 confers protection from autoimmune encephalomyelitis only in female mice. J. Immunol. 175, 4119–4126. doi: 10.4049/jimmunol.175.6.4119
Srviastava, M. D., Deluca, H., and Ambrus, J. L. (1994). Inhibition of IL-6 and IL-8 production in human fibroblast cell lines by 1,25 (OH)2 vitamin D3 and two of its analogs with lower calcemic activity. Res. Commun. Chem. Pathol. Pharmacol. 83, 145–150.
Stan, D., Calin, M., Manduteanu, I., Pirvulescu, M., Gan, A. M., Butoi, E. D., et al. (2011). High glucose induces enhanced expression of resistin in human U937 monocyte-like cell line by MAPK- and NF-kB-dependent mechanisms; the modulating effect of insulin. Cell Tissue Res. 343, 379–387. doi: 10.1007/s00441-010-1092-3
Stio, M., Martinesi, M., Bruni, S., Treves, C., Mathieu, C., Verstuyf, A., et al. (2007). The Vitamin D analogue TX 527 blocks NF-kappaB activation in peripheral blood mononuclear cells of patients with Crohn's disease. J. Steroid Biochem. Mol. Biol. 103, 51–60. doi: 10.1016/j.jsbmb.2006.07.008
Stoffers, K. L., Sorg, B. L., Seuter, S., Rau, O., Radmark, O., and Steinhilber, D. (2010). Calcitriol upregulates open chromatin and elongation markers at functional vitamin D response elements in the distal part of the 5-lipoxygenase gene. J. Mol. Biol. 395, 884–896. doi: 10.1016/j.jmb.2009.10.022
Stojanovic, O. I., Lazovic, M., Lazovic, M., and Vuceljic, M. (2011). Association between atherosclerosis and osteoporosis, the role of vitamin D. Arch. Med. Sci. 7, 179–188. doi: 10.5114/aoms.2011.22066
Subramaniam, N., Leong, G. M., Cock, T. A., Flanagan, J. L., Fong, C., Eisman, J. A., et al. (2001). Cross-talk between 1,25-dihydroxyvitamin D3 and transforming growth factor-beta signaling requires binding of VDR and Smad3 proteins to their cognate DNA recognition elements. J. Biol. Chem. 276, 15741–15746. doi: 10.1074/jbc.M011033200
Summerday, N. M., Brown, S. J., Allington, D. R., and Rivey, M. P. (2012). Vitamin D and multiple sclerosis: review of a possible association. J. Pharm. Pract. 25, 75–84. doi: 10.1177/0897190011421839
Sun, J., Zhong, W., Gu, Y., Groome, L. J., and Wang, Y. (2013). 1,25(OH)D suppresses COX-2 up-regulation and thromboxane production in placental trophoblast cells in response to hypoxic stimulation. Placenta 35, 143–145. doi: 10.1016/j.placenta.2013.12.002
Sun, X., and Zemel, M. B. (2008). Calcitriol and calcium regulate cytokine production and adipocyte-macrophage cross-talk. J. Nutr. Biochem. 19, 392–399. doi: 10.1016/j.jnutbio.2007.05.013
Sutherland, E. R., Goleva, E., Jackson, L. P., Stevens, A. D., and Leung, D. Y. (2010). Vitamin D levels, lung function, and steroid response in adult asthma. Am. J. Respir. Crit. Care Med. 181, 699–704. doi: 10.1164/rccm.200911-1710OC
Szekely, J. I., and Pataki, A. (2012). Effects of vitamin D on immune disorders with special regard to asthma, COPD and autoimmune diseases: a short review. Expert Rev. Respir. Med. 6, 683–704. doi: 10.1586/ers.12.57
Szeto, F. L., Reardon, C. A., Yoon, D., Wang, Y., Wong, K. E., Chen, Y., et al. (2012). Vitamin D receptor signaling inhibits atherosclerosis in mice. Mol. Endocrinol. 26, 1091–1101. doi: 10.1210/me.2011-1329
Tabata, T., Shoji, T., Kikunami, K., Matsushita, Y., Inoue, T., Tanaka, S., et al. (1988). In vivo effect of 1 alpha-hydroxyvitamin D3 on interleukin-2 production in hemodialysis patients. Nephron 50, 295–298. doi: 10.1159/000185191
Taimi, M., Defacque, H., Commes, T., Favero, J., Caron, E., Marti, J., et al. (1993). Effect of retinoic acid and vitamin D on the expression of interleukin-1 beta, tumour necrosis factor-alpha and interleukin-6 in the human monocytic cell line U937. Immunology 79, 229–235.
Takahashi, K., Nakayama, Y., Horiuchi, H., Ohta, T., Komoriya, K., Ohmori, H., et al. (2002). Human neutrophils express messenger RNA of vitamin D receptor and respond to 1alpha,25-dihydroxyvitamin D3. Immunopharmacol. Immunotoxicol. 24, 335–347. doi: 10.1081/IPH-120014721
Takeda, M., Yamashita, T., Sasaki, N., Nakajima, K., Kita, T., Shinohara, M., et al. (2010). Oral administration of an active form of vitamin D3 (calcitriol) decreases atherosclerosis in mice by inducing regulatory T cells and immature dendritic cells with tolerogenic functions. Arterioscler. Thromb. Vasc. Biol. 30, 2495–2503. doi: 10.1161/ATVBAHA.110.215459
Takeuchi, A., Reddy, G. S., Kobayashi, T., Okano, T., Park, J., and Sharma, S. (1998). Nuclear factor of activated T cells (NFAT) as a molecular target for 1alpha,25-dihydroxyvitamin D3-mediated effects. J. Immunol. 160, 209–218.
Talmor-Barkan, Y., Bernheim, J., Green, J., Benchetrit, S., and Rashid, G. (2011). Calcitriol counteracts endothelial cell pro-inflammatory processes in a chronic kidney disease-like environment. J. Steroid Biochem. Mol. Biol. 124, 19–24. doi: 10.1016/j.jsbmb.2011.01.001
Taura, S., Taura, M., Kamio, A., and Kummerow, F. A. (1979). Vitamin D-induced coronary atherosclerosis in normolipemic swine: comparison with human disease. Tohoku J. Exp. Med. 129, 9–16. doi: 10.1620/tjem.129.9
Tavakoli, M., Jeddi-Tehrani, M., Salek-Moghaddam, A., Rajaei, S., Mohammadzadeh, A., Sheikhhasani, S., et al. (2011). Effects of 1,25(OH)2 vitamin D3 on cytokine production by endometrial cells of women with recurrent spontaneous abortion. Fertil. Steril. 96, 751–757. doi: 10.1016/j.fertnstert.2011.06.075
Thill, M., Becker, S., Fischer, D., Cordes, T., Hornemann, A., Diedrich, K., et al. (2009). Expression of prostaglandin metabolising enzymes COX-2 and 15-PGDH and VDR in human granulosa cells. Anticancer Res. 29, 3611–3618.
Thill, M., Fischer, D., Kelling, K., Hoellen, F., Dittmer, C., Hornemann, A., et al. (2010). Expression of vitamin D receptor (VDR), cyclooxygenase-2 (COX-2) and 15-hydroxyprostaglandin dehydrogenase (15-PGDH) in benign and malignant ovarian tissue and 25-hydroxycholecalciferol (25(OH2)D3) and prostaglandin E2 (PGE2) serum level in ovarian cancer patients. J. Steroid Biochem. Mol. Biol. 121, 387–390. doi: 10.1016/j.jsbmb.2010.03.049
Thill, M., Hoellen, F., Becker, S., Dittmer, C., Fischer, D., Kummel, S., et al. (2012). Expression of prostaglandin- and vitamin D-metabolising enzymes in benign and malignant breast cells. Anticancer Res. 32, 367–372.
Thota, C., Farmer, T., Garfield, R. E., Menon, R., and Al-Hendy, A. (2013). Vitamin D elicits anti-inflammatory response, inhibits contractile-associated proteins, and modulates Toll-like receptors in human myometrial cells. Reprod. Sci. 20, 463–475. doi: 10.1177/1933719112459225
Toda, T., Ito, M., Toda, Y., Smith, T., and Kummerow, F. (1985). Angiotoxicity in swine of a moderate excess of dietary vitamin D3. Food Chem. Toxicol. 23, 585–592. doi: 10.1016/0278-6915(85)90183-8
Toda, T., Leszczynski, D. E., and Kummerow, F. A. (1983). The role of 25-hydroxy-vitamin D3 in the induction of atherosclerosis in swine and rabbit by hypervitaminosis D. Acta Pathol. Jpn. 33, 37–44.
Tolppanen, A. M., Sayers, A., Granell, R., Fraser, W. D., Henderson, J., and Lawlor, D. A. (2013). Prospective association of 25-hydroxyvitamin d3 and d2 with childhood lung function, asthma, wheezing, and flexural dermatitis. Epidemiology 24, 310–319. doi: 10.1097/EDE.obo13e318280dd5e
Tomasoni, S., Noris, M., Zappella, S., Gotti, E., Casiraghi, F., Bonazzola, S., et al. (1998). Upregulation of renal and systemic cyclooxygenase-2 in patients with active lupus nephritis. J. Am. Soc. Nephrol. 9, 1202–1212.
Tsatsanis, C., Androulidaki, A., Venihaki, M., and Margioris, A. N. (2006). Signalling networks regulating cyclooxygenase-2. Int. J. Biochem. Cell Biol. 38, 1654–1661. doi: 10.1016/j.biocel.2006.03.021
Tsoukas, C. D., Provvedini, D. M., and Manolagas, S. C. (1984). 1,25-dihydroxyvitamin D3: a novel immunoregulatory hormone. Science 224, 1438–1440. doi: 10.1126/science.6427926
Tsoukas, C. D., Watry, D., Escobar, S. S., Provvedini, D. M., Dinarello, C. A., Hustmyer, F. G., et al. (1989). Inhibition of interleukin-1 production by 1,25-dihydroxyvitamin D3. J. Clin. Endocrinol. Metab. 69, 127–133. doi: 10.1210/jcem-69-1-127
Tuoresmäki, P., Vaisanen, S., Neme, A., Heikkinen, S., and Carlberg, C. (2014). Patterns of genome-wide VDR locations. PLoS ONE 9:e96105. doi: 10.1371/journal.pone.0096105
Ueki, N., and Hayman, M. J. (2003). Signal-dependent N-CoR requirement for repression by the Ski oncoprotein. J. Biol. Chem. 278, 24858–24864. doi: 10.1074/jbc.M303447200
Urry, Z., Chambers, E. S., Xystrakis, E., Dimeloe, S., Richards, D. F., Gabrysova, L., et al. (2012). The role of 1alpha,25-dihydroxyvitamin D3 and cytokines in the promotion of distinct Foxp3+ and IL-10+ CD4+ T cells. Eur. J. Immunol. 42, 2697–2708. doi: 10.1002/eji.201242370
Van Etten, E., Dardenne, O., Gysemans, C., Overbergh, L., and Mathieu, C. (2004). 1,25-Dihydroxyvitamin D3 alters the profile of bone marrow-derived dendritic cells of NOD mice. Ann. N.Y. Acad. Sci. 1037, 186–192. doi: 10.1196/annals.1337.030
Van Etten, E., Verlinden, L., Giulietti, A., Ramos-Lopez, E., Branisteanu, D. D., Ferreira, G. B., et al. (2007). The vitamin D receptor gene FokI polymorphism: functional impact on the immune system. Eur. J. Immunol. 37, 395–405. doi: 10.1002/eji.200636043
Van Oeffelen, A. A., Bekkers, M. B., Smit, H. A., Kerkhof, M., Koppelman, G. H., Haveman-Nies, A., et al. (2011). Serum micronutrient concentrations and childhood asthma: the PIAMA birth cohort study. Pediatr. Allergy Immunol. 22, 784–793. doi: 10.1111/j.1399-3038.2011.01190.x
Verway, M., Bouttier, M., Wang, T. T., Carrier, M., Calderon, M., An, B. S., et al. (2013). Vitamin D induces interleukin-1beta expression: paracrine macrophage epithelial signaling controls M. tuberculosis infection. PLoS Pathog 9:e1003407. doi: 10.1371/journal.ppat.1003407
Villaggio, B., Soldano, S., and Cutolo, M. (2012). 1,25-dihydroxyvitamin D3 downregulates aromatase expression and inflammatory cytokines in human macrophages. Clin. Exp. Rheumatol. 30, 934–938.
Vollmert, C., Illig, T., Altmuller, J., Klugbauer, S., Loesgen, S., Dumitrescu, L., et al. (2004). Single nucleotide polymorphism screening and association analysis–exclusion of integrin beta 7 and vitamin D receptor (chromosome 12q) as candidate genes for asthma. Clin. Exp. Allergy 34, 1841–1850. doi: 10.1111/j.1365-2222.2004.02047.x
Wamberg, L., Cullberg, K. B., Rejnmark, L., Richelsen, B., and Pedersen, S. B. (2013). Investigations of the anti-inflammatory effects of vitamin D in adipose tissue: results from an in vitro study and a randomized controlled trial. Horm. Metab. Res. 45, 456–462. doi: 10.1055/s-0032-1331746
Weng, S., Sprague, J. E., Oh, J., Riek, A. E., Chin, K., Garcia, M., et al. (2013). Vitamin D deficiency induces high blood pressure and accelerates atherosclerosis in mice. PLoS ONE 8:e54625. doi: 10.1371/journal.pone.0054625
Willheim, M., Thien, R., Schrattbauer, K., Bajna, E., Holub, M., Gruber, R., et al. (1999). Regulatory effects of 1alpha,25-dihydroxyvitamin D3 on the cytokine production of human peripheral blood lymphocytes. J. Clin. Endocrinol. Metab. 84, 3739–3744.
Wingerchuk, D. M., Lesaux, J., Rice, G. P., Kremenchutzky, M., and Ebers, G. C. (2005). A pilot study of oral calcitriol (1,25-dihydroxyvitamin D3) for relapsing-remitting multiple sclerosis. J. Neurol. Neurosurg. Psychiatr. 76, 1294–1296. doi: 10.1136/jnnp.2004.056499
Wobke, T. K., Von Knethen, A., Steinhilber, D., and Sorg, B. L. (2013). CD69 is a TGF-beta/1alpha,25-dihydroxyvitamin D3 target gene in monocytes. PLoS ONE 8, e64635. doi: 10.1371/journal.pone.0064635
Wu, A. C., Tantisira, K., Li, L., Fuhlbrigge, A. L., Weiss, S. T., and Litonjua, A. (2012). Effect of vitamin D and inhaled corticosteroid treatment on lung function in children. Am. J. Respir. Crit. Care Med. 186, 508–513. doi: 10.1164/rccm.201202-0351OC
Xiao, H. Q., Shi, W., Liu, S. X., Zhang, B., Xu, L. X., Liang, X. L., et al. (2009). Podocyte injury is suppressed by 1,25-dihydroxyvitamin D via modulation of transforming growth factor-beta 1/bone morphogenetic protein-7 signalling in puromycin aminonucleoside nephropathy rats. Clin. Exp. Pharmacol. Physiol. 36, 682–689. doi: 10.1111/j.1440-1681.2008.05133.x
Yadav, A. K., Banerjee, D., Lal, A., and Jha, V. (2012). Vitamin D deficiency, CD4+CD28null cells and accelerated atherosclerosis in chronic kidney disease. Nephrology (Carlton) 17, 575–581. doi: 10.1111/j.1440-1797.2012.01611.x
Yanagisawa, J., Yanagi, Y., Masuhiro, Y., Suzawa, M., Watanabe, M., Kashiwagi, K., et al. (1999). Convergence of transforming growth factor-beta and vitamin D signaling pathways on SMAD transcriptional coactivators. Science 283, 1317–1321. doi: 10.1126/science.283.5406.1317
Yang, J., Han, Y., Chen, C., Sun, H., He, D., Guo, J., et al. (2013). EGCG attenuates high glucose-induced endothelial cell inflammation by suppression of PKC and NF-kappaB signaling in human umbilical vein endothelial cells. Life Sci. 92, 589–597. doi: 10.1016/j.lfs.2013.01.025
Yoon, J. W., and Jun, H. S. (1999). Cellular and molecular roles of beta cell autoantigens, macrophages and T cells in the pathogenesis of autoimmune diabetes. Arch. Pharm. Res. 22, 437–447. doi: 10.1007/BF02979150
Yu, X. P., Bellido, T., and Manolagas, S. C. (1995). Down-regulation of NF-kappa B protein levels in activated human lymphocytes by 1,25-dihydroxyvitamin D3. Proc. Natl. Acad. Sci. U.S.A. 92, 10990–10994. doi: 10.1073/pnas.92.24.10990
Yuan, L., Jiang, R., Yang, Y., Ding, S., and Deng, H. (2012). 1,25-Dihydroxyvitamin D3 inhibits growth of the breast cancer cell line MCF-7 and downregulates cytochrome P4501B1 through the COX-2/PGE2 pathway. Oncol. Rep. 28, 2131–2137. doi: 10.3892/or.2012.2031
Zarrabeitia, M. T., Riancho, J. A., Amado, J. A., Olmos, J. M., and Gonzalez-Macias, J. (1992). Effect of calcitriol on the secretion of prostaglandin E2, interleukin 1, and tumor necrosis factor alpha by human monocytes. Bone 13, 185–189. doi: 10.1016/8756-3282(92)90010-T
Zemplenyi, T., and Mrhova, O. (1965). Vascular enzyme activity changes accompanying the induction of experimental atherosclerosis. 2. Rats fed excess vitamin D. J. Atheroscler. Res. 5, 548–555. doi: 10.1016/S0368-1319(65)80031-X
Zhang, H., Shih, D. Q., and Zhang, X. (2013a). Mechanisms underlying effects of 1,25-Dihydroxyvitamin D3 on the Th17 cells. Eur. J. Microbiol. Immunol. (Bp). 3, 237–240. doi: 10.1556/EuJMI.3.2013.4.1
Zhang, J. Z., Maruyama, K., Ono, I., Iwatsuki, K., and Kaneko, F. (1994). Regulatory effects of 1,25-dihydroxyvitamin D3 and a novel vitamin D3 analogue MC903 on secretion of interleukin-1 alpha (IL-1 alpha) and IL-8 by normal human keratinocytes and a human squamous cell carcinoma cell line (HSC-1). J. Dermatol. Sci. 7, 24–31. doi: 10.1016/0923-1811(94)90018-3
Zhang, Y., Leung, D. Y., and Goleva, E. (2013b). Vitamin D enhances glucocorticoid action in human monocytes: involvement of granulocyte-macrophage colony-stimulating factor and mediator complex subunit 14. J. Biol. Chem. 288, 14544–14553. doi: 10.1074/jbc.M112.427054
Zhang, Y., Leung, D. Y., Richers, B. N., Liu, Y., Remigio, L. K., Riches, D. W., et al. (2012). Vitamin D inhibits monocyte/macrophage proinflammatory cytokine production by targeting MAPK phosphatase-1. J. Immunol. 188, 2127–2135. doi: 10.4049/jimmunol.1102412
Zhou, C., Lu, F., Cao, K., Xu, D., Goltzman, D., and Miao, D. (2008). Calcium-independent and 1,25(OH)2D3-dependent regulation of the renin-angiotensin system in 1alpha-hydroxylase knockout mice. Kidney Int. 74, 170–179. doi: 10.1038/ki.2008.101
Keywords: 1α,25(OH)2D3, VDR, cyclooxygenase, NFκB, NFAT, MKP1, interleukins, innate immune system
Citation: Wöbke TK, Sorg BL and Steinhilber D (2014) Vitamin D in inflammatory diseases. Front. Physiol. 5:244. doi: 10.3389/fphys.2014.00244
Received: 22 April 2014; Accepted: 12 June 2014;
Published online: 02 July 2014.
Edited by:
Carsten Carlberg, University of Eastern Finland, FinlandReviewed by:
Martin Hewison, University of California, Los Angeles, USACopyright © 2014 Wöbke, Sorg and Steinhilber. This is an open-access article distributed under the terms of the Creative Commons Attribution License (CC BY). The use, distribution or reproduction in other forums is permitted, provided the original author(s) or licensor are credited and that the original publication in this journal is cited, in accordance with accepted academic practice. No use, distribution or reproduction is permitted which does not comply with these terms.
*Correspondence: Dieter Steinhilber, Institute of Pharmaceutical Chemistry, Goethe University Frankfurt, Max-von-Laue-Str. 9, Frankfurt, D-60438, Germany e-mail:c3RlaW5oaWxiZXJAZW0udW5pLWZyYW5rZnVydC5kZQ==
Disclaimer: All claims expressed in this article are solely those of the authors and do not necessarily represent those of their affiliated organizations, or those of the publisher, the editors and the reviewers. Any product that may be evaluated in this article or claim that may be made by its manufacturer is not guaranteed or endorsed by the publisher.
Research integrity at Frontiers
Learn more about the work of our research integrity team to safeguard the quality of each article we publish.