- 1 Department of Neuroscience, “Federico II” University Medical School, Naples, Italy
- 2 Rheumatology Unit, Department of Internal Medicine, University of Pisa, Pisa, Italy
- 3 Institute of Clinical Physiology, Consiglio Nazionale delle Ricerche, Pisa, Italy
The aim of this study was to assess the in vivo effects of quercetin on pial microvascular responses during transient bilateral common carotid artery occlusion (BCCAO) and reperfusion. Rat pial microcirculation was visualized by fluorescence microscopy through a closed cranial window. Pial arterioles were classified in five orders of branchings. Capillaries were assigned order 0, the smallest arterioles order 1, and the largest ones order 5. In ischemic rats, 30 min BCCAO and 60 min reperfusion caused arteriolar diameter decrease (by 14.5 ± 3.3% of baseline in order 2), microvascular leakage [0.47 ± 0.04, normalized gray levels (NGL)], leukocyte adhesion in venules (9 ± 2/100 μm venular length, v.l./30 s), and reduction of capillary perfusion (by 40 ± 7% of baseline). Moreover, at the end of BCCAO and reperfusion there was a significant increase in reactive oxygen species (ROS) formation when compared with baseline. Quercetin highest dose determined dilation in all arteriolar orders (by 40 ± 4% of baseline in order 2) and prevented microvascular permeability (0.15 ± 0.02 NGL), leukocyte adhesion (3 ± 1/100 μm v.l./30 s) as well as ROS formation, while capillary perfusion was protected. Inhibition of endothelial nitric oxide synthase (NOS) prior to quercetin reduced arteriolar dilation (order 2 diameter increase by 10.3 ± 2.5% of baseline) and caused permeability increase (0.29 ± 0.03 NGL); inhibition of neuronal NOS or inducible NOS did not affect quercetin-induced effects. Inhibition of guanylyl cyclase prior to quercetin reversed the quercetin’s effects on pial arteriolar diameter and leakage. In conclusion, quercetin was able to protect pial microcirculation from ischemia–reperfusion damage inducing arteriolar dilation likely by nitric oxide release. Moreover, quercetin scavenger activity blunted ROS formation preserving the blood–brain barrier integrity.
Introduction
The cerebral transient hypoperfusion, induced in experimental models by bilateral common carotid artery occlusion (BCCAO) and followed by reperfusion, causes significant microvascular alterations. These are characterized by disruption of the blood–brain barrier, edema, and swelling of perivascular astrocyte foot processes, decrease in arteriolar endothelium-dependent relaxation, altered expression of proteases, and matrix metalloproteinases, increase in inflammatory mediators (Chan, 1996; Nakashima et al., 1999; Cho et al., 2006; Lapi et al., 2008a). Recent data indicate that 30 min of BCCAO and 45 min of reperfusion induce lipoperoxidation of brain tissue due to formation of reactive oxygen species (ROS; Xu et al., 2002). On the other hand, several studies, carried out in humans, have suggested a close relationship between cerebral ischemia and oxidative stress (Dugan and Kim-Han, 2006). The cerebral tissue is vulnerable to the effects of ROS, because under oxidative stress superoxide anion and hydrogen peroxide cannot be readily neutralized due to the low catalase, superoxide dismutase (SOD) and glutathione peroxidase activities present in the brain. Furthermore, brain membrane lipids are very rich in polyunsaturated fatty acids, which are sensitive to free radical-induced peroxidation, likely one of the most important events in cerebral cell damage (Del Zoppo, 1994; Pietta, 2000).
Drugs able to limit ischemia–reperfusion injury are free radical scavengers (antioxidant substances) and agents forming stable adducts with free radicals, as previously shown. Therefore, antioxidant substances, particularly those ones present in food sources such as flavonoids, have been extensively studied (Ikeda et al., 2003). Flavonoids have a wide range of activities in the prevention of coronary heart disease, cancer, gastrointestinal disorders, and inflammation (Moreira et al., 2004). They are polyphenolic compounds, benzo-γ-pyrone derivatives, widely distributed in dietary fruits and vegetables. One of the most available is quercetin which represents about 60–75% of the average amount of flavonoids in the usual Western country diet (Rice-Evans, 2001).
The in vivo protection exerted by quercetin on cerebral ischemia has been partially described in previous studies. Shutenko et al. (1999) demonstrated that the intraperitoneal or intravenous administration of quercetin in rats, subjected to ischemia and reperfusion and sacrified afterward, increases the nitric oxide (NO) levels only in cerebral cortex with consequent reduction in superoxide levels and lowering of peroxynitrite formation. The results of Kumura et al. (1996) support this hypothesis because they found that the NO levels measured in jugular vein blood during reperfusion after middle cerebral artery occlusion (2 h) are significantly increased by SOD treatment. Recent studies have shown that quercetin induces vessel dilation by activation of the NO pathway in smooth muscle cells and triggers systemic and coronary vasodilation (Ajay et al., 2003). Quercetin reduces blood pressure, cardiac hypertrophy, and vascular remodeling in spontaneously hypertensive and NO-deficient rats (Lopez et al., 2004).
Recently, Ahmad et al. (2011) have shown quercetin dehydrate, intraperitoneally administered in rats, protects against neuronal damage induced by transient middle cerebral artery occlusion through prevention of free radical formation. However, the mechanism of flavonoid action still remains elusive.
Up to now, there are no data on the effects exerted by quercetin on in vivo pial microvasculature and on its specific mechanism of action.
The purpose of the present study was to investigate the in vivo vascular effects of quercetin in preventing injury due to BCCAO and reperfusion. Our suggestion was that quercetin could induce vasodilation through the NO pathway; therefore, we studied the effects of inhibiting endothelial nitric oxide synthase (eNOS) by NG-nitro-L-arginine-methyl ester (L-NAME), or neuronal NOS (nNOS) by 7-nitroindazole, or inducible NOS (iNOS) by L-N6-(1-iminoethyl) lysine hydrochloride. Moreover, we used 1H-(1, 2, 4) oxadiazolo (4, 3-alpha) quinoxalin-1-one (ODQ) to inhibit guanylyl cyclase, known to be triggered by NO. The principal aim was to analyze pial microvascular responses, such as arteriolar diameter changes, permeability increase, leukocyte adhesion, and capillary perfusion, after administration of quercetin in rats subjected to BCCAO and reperfusion. In particular, we evaluated the pial arteriole changes by fluorescence microscopy, because these vessels constitute a complex surface network characterized by five orders of vessels, supplying blood to superficial layers of cerebral cortex (Lapi et al., 2008b). We focused our attention on the responses of order 2 arterioles, the most reactive to hypoperfusion due to BCCAO. The neuronal physiological activity, indeed, depends on adequate blood flow supply; therefore, the pial microvascular behavior during BCCAO and reperfusion might be important to evaluate changes in cerebral cortex blood flow. Finally, we evaluated ROS formation by 2′-7′-dichlorofluorescein-diacetate (DCFH-DA) at the end of BCCAO and reperfusion.
Materials and Methods
Experimental Groups
Male Wistar rats weighing 250–300 g (Harlan, Italy) were randomly assigned to eight groups.
(1) The first group was composed from animals not subjected to BCCAO and reperfusion [Sham-Operated (S) group, n = 18]. Three animals were treated with artificial cerebrospinal fluid (aCSF) containing 250 mM 2′-7′dichlorofluorescein-diacetate (DCFH-DA), five animals were used for microvascular observations, five animals were utilized to evaluate the eNOS expression by Western blotting and five animals were used to determine neuronal damage by 2,3,5-Triphenyltetrazolium Chloride (TTC) Staining. Moreover, sham-operated rats received intravenously (i.v.) quercetin 1.0, 2.5, 5.0 mg/kg body weight (b.w.; subgroup SQ1, subgroup SQ2, and subgroup SQ3; n = 3, respectively) or i.v. L-NAME (eNOS inhibitor) 10 mg/kg b.w. (group SL, n = 3) or i.v. 1H-(1, 2, 4) oxadiazolo (4, 3-alpha) quinoxalin-1-one (ODQ, guanylyl cyclase inhibitor) 1.0 mg/kg b.w. (group S, n = 3) or i.v. 7-nitroindazole (nNOS inhibitor) 9.0 mg/kg b.w. (group SN, n = 3) or i.v. L-N6-(1-iminoethyl) lysine hydrochloride (iNOS inhibitor) 5 mg/kg b.w. (group SNI, n = 3). (2) Ischemic rats (group I, n = 25) were treated with 1.5 mL vehicle (physiological saline solution), i.v. injected, and subjected to 30 min of BCCAO and 60 min of reperfusion. Three rats were treated with aCSF containing 250 mM DCFH-DA during BCCAO and three animals during reperfusion (RE). Moreover, nine animals were utilized for microvascular studies, five ones to evaluate the eNOS expression by Western blotting and five animals were used to determine neuronal damage by TTC staining. (3) The third group (subgroup Q1, n = 20; subgroup Q2, n = 2; and subgroup Q3, n = 25) was treated with i.v. quercetin 1.0, 2.5, and 5.0 mg/kg b.w., respectively. In three rats, belonging to each subgroup Q1, Q2, and Q3, during BCCAO and three rats during reperfusion were treated with aCSF containing 250 mM DCFH-DA. Nine animals belonging to each subgroup Q1, Q2, and Q3 were utilized for microvascular studies, while five animals belonging to each subgroup were used to evaluate the eNOS expression by Western blotting. In addition, five animals belonging to subgroup Q3 were used to determine neuronal damage by TTC staining. (4) The fourth group of animals (group LQ, n = 9) received i.v. L-NAME, 10 mg/kg b.w., prior to i.v. quercetin (5 mg/kg b.w.) before BCCAO and at the beginning of reperfusion. (5) The fifth group of rats (group NQ, n = 9) received i.v. 7-nitroindazole (9 mg/kg b.w.) prior to i.v. quercetin (5 mg/kg b.w.) before BCCAO and at the beginning of reperfusion. (6) The sixth groups of animals (group NIQ, n = 9) received i.v. L-N6-(1-iminoethyl) lysine chloride (5 mg/kg b.w.) prior to i.v. quercetin (5 mg/kg b.w.) before BCCAO and at the beginning of reperfusion. (7) The seventh group of rats (OQ group, n = 9) was treated with i.v. injected 1H-(1, 2, 4) oxadiazolo (4, 3-alpha) quinoxalin-1-one (ODQ; 1 mg/kg b.w.) and i.v. quercetin (5 mg/kg b.w.) before BCCAO and at the beginning of reperfusion. (8) Finally, the last group of rats received L-NAME (10 mg/kg b.w.; group L, n = 9), i.v. injected 10 min before BCCAO and at the beginning of reperfusion (Tables 1 and 2).
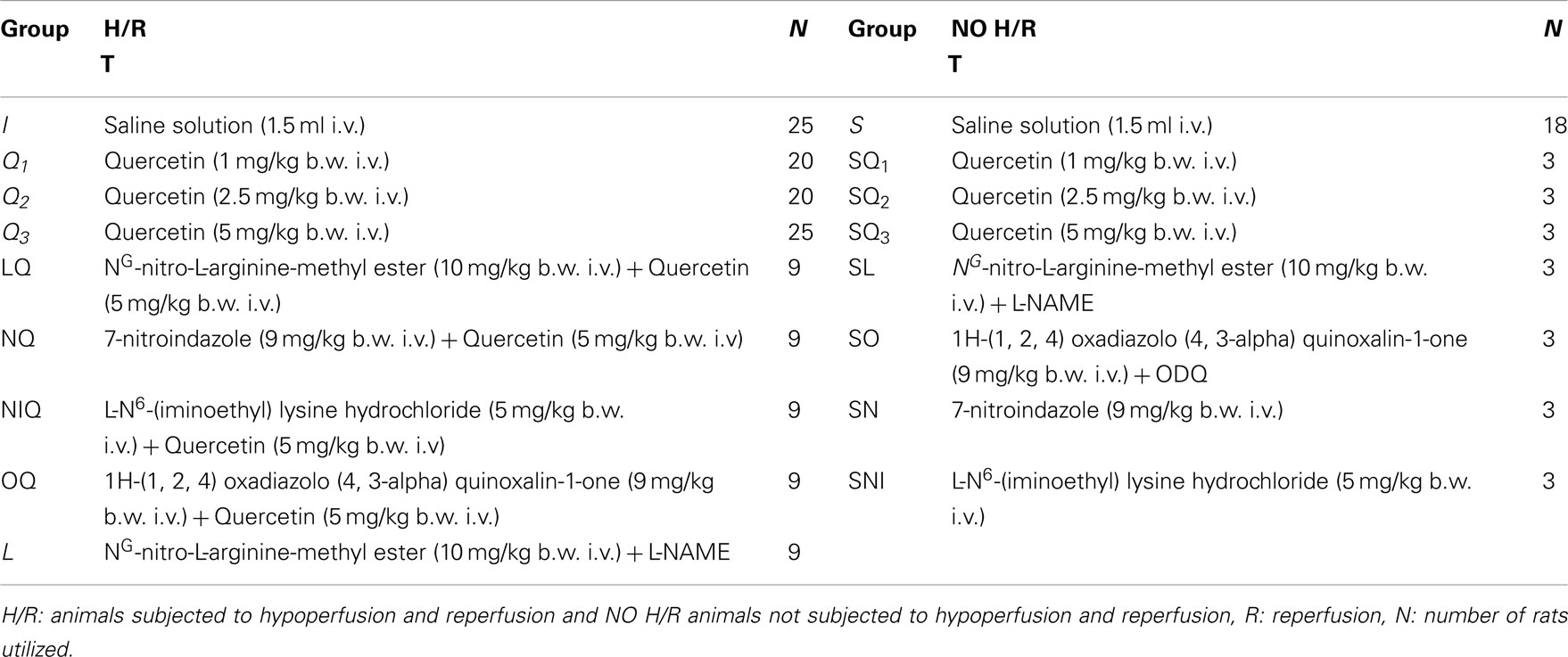
Table 1. Drug treatment (T), doses and route of administration in the different experimental groups.
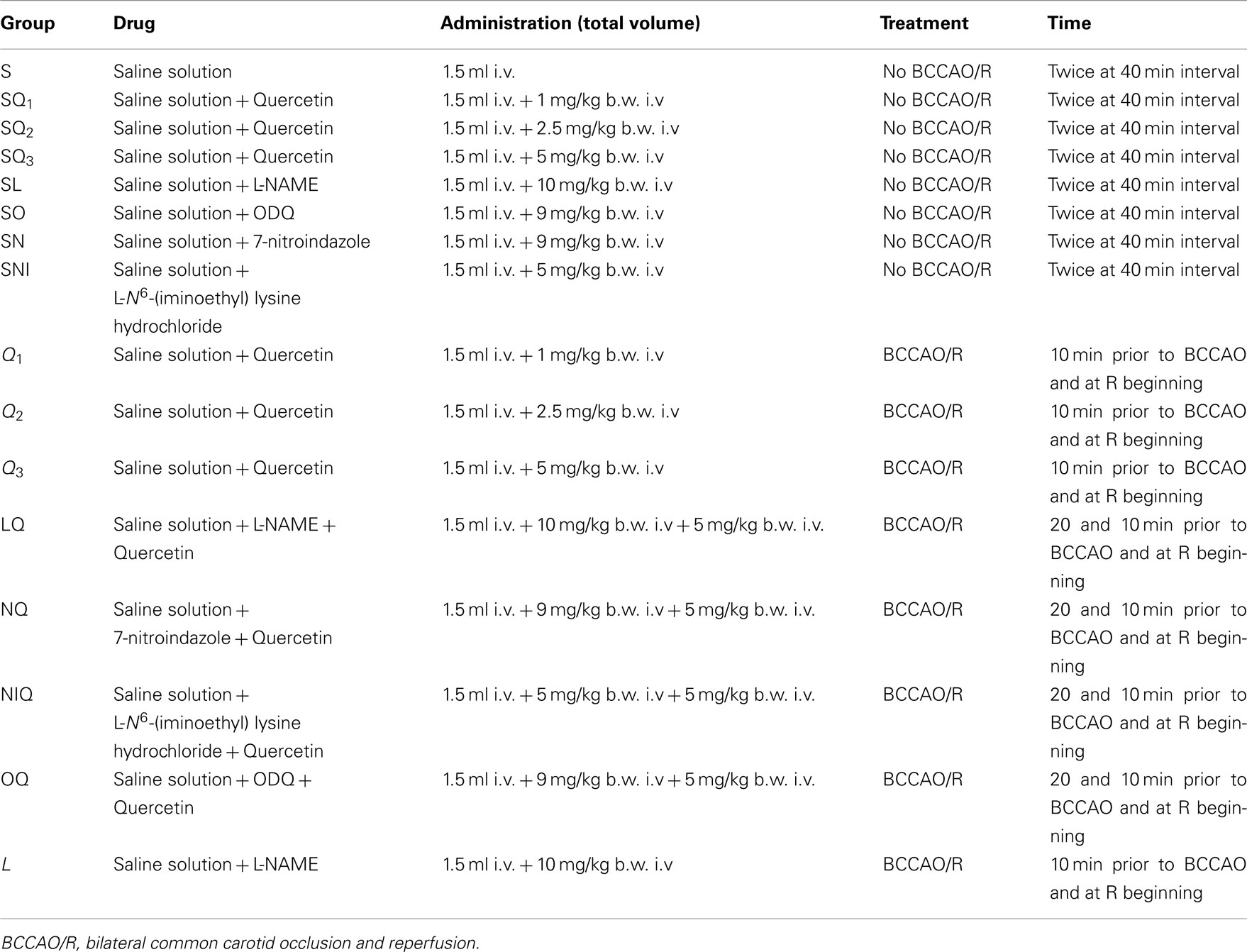
Table 2. Drugs, doses and route of administration, type of treatment and time of administration in the different experimental groups.
Administration of Drugs
Quercetin solutions were obtained dissolving 1.0, 2.5, and 5.0 mg/kg b.w. in 0.5 mL of saline solution and i.v. infused (3 min) to the rats 10 min before BCCAO and at the beginning of reperfusion (Shutenko et al., 1999).
L-NAME (10 mg/kg b.w.) (3), 7-nitroindazole (9 mg/kg b.w.; Bush and Pollak, 2000), L-N6-(1-iminoethyl) lysine chloride (5 mg/kg b.w.; Sakamoto et al., 2005) were dissolved in 0.5 ml of saline solution, respectively; ODQ (1.0 mg/kg b.w.; Zingarelli et al., 1999) was suspended in ethanol and saline solution (1.2 mg/mL). Each substance was i.v. administered prior to quercetin (5.0 mg/kg b.w.) according to the protocol time schedule reported in Figure 1.
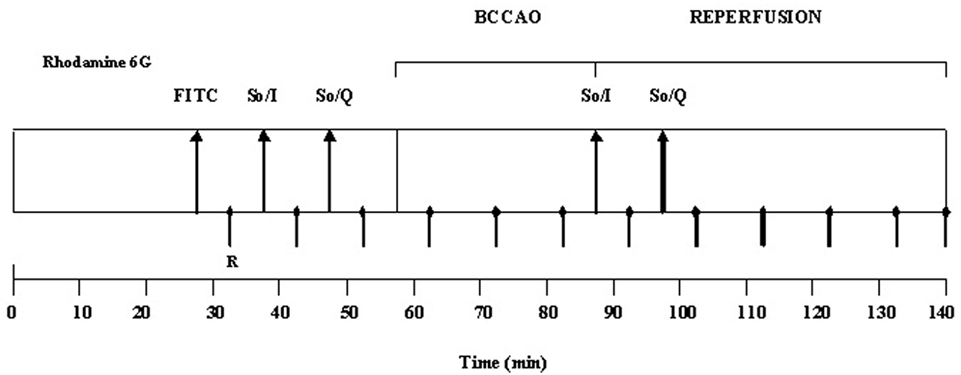
Figure 1. Experimental design of drug administration and measurement times. R: recording points. FITC: fluorescent-dextran (70 kDa) and rhodamine 6G. So/I: solvent, 0.9% NaCl, and/or inhibitor administration (L-NAME, ODQ). So/Q: solvent, 0.9% NaCl, and/or quercetin administration. BCCAO: bilateral common carotid artery occlusion. Rhodamine 6G was injected after FITC-dextran as a bolus and then infused during BCCAO and reperfusion.
2′-7′-dichlorofluorescein-diacetate (DCFH-DA) was mixed with aCSF to obtain a concentration of 250 mM (Watanabe, 1998). This solution was superfused over the pial surface for 15 min during ischemia or reperfusion. The drugs were purchased by Sigma Chemical, St. Louis, MO, USA.
In pilot experiments quercetin was tested in different concentrations; we chose the highest dosage, 5 mg/kg b.w., according to our preliminary results and to previous data (Shutenko et al., 1999). In preliminary experiments i.v. infusion of L-NAME at the dosage of 10 mg/kg b.w., chosen for the present study, abolished vasodilation due to topical application of acetylcholine, 100 μM (n = 10), while the diameter increase was by 42.3 ± 3.2% of baseline in sham-operated animals treated with acetylcholine, n = 10 (Moreau et al., 1995).
In pilot experiments nNOS and iNOS inhibitors were tested in different concentrations; we chose the dose of 9 and 5 mg/kg for 7-nitroindazole and L-N6-(1-iminoethyl) lysine hydrochloride, respectively, according to previous data (Zingarelli et al., 1999; Sakamoto et al., 2005).
Animal Preparation
Anesthesia was induced with α-chloralose (50 mg/kg b.w., i.p.) plus urethane (600 mg/kg b.w., i.p.) and maintained with urethane alone (100 mg/kg b.w., i.v. every hour). Rats were tracheotomized, paralyzed with tubocurarine chloride (1 mg/kg·h, i.v.) and mechanically ventilated with room air and supplemental oxygen. The right and left common carotid arteries were isolated for successive clamping. A catheter was placed in the left femoral artery for arterial blood pressure recording and blood gases sampling. Another catheter was placed in the right femoral vein for injection of the fluorescent tracers [fluorescein isothiocyanate bound to dextran, molecular weight 70 kDa (FD 70), 50 mg/100 g b.w., i.v. as 5% wt/vol solution in 3 min administered just once at the beginning of experiment after 30 min of the preparation stabilization; rhodamine 6G, 1 mg/100 g b.w. in 0.3 mL, as a bolus with supplemental injection throughout BCCAO and reperfusion (final volume 0.3 ml·100 g−1·h−1) to label leukocytes for adhesion evaluation] and of drugs. Blood gas measurements were carried out on arterial blood samples withdrawn from arterial catheter at 30 min time period intervals (ABL5; Radiometer, Copenhagen, Denmark). Throughout all experiments, mean arterial blood pressure (MABP), heart rate, respiratory CO2, and blood gases values were recorded and maintained stable within physiological ranges. Rectal temperature was monitored and preserved at 37.0 ± 0.5°C with a heating stereotaxic frame where the rats were secured.
To observe the pial microcirculation, a closed cranial window (4 mm × 5 mm) was implanted above the left frontoparietal cortex (posterior 1.5 mm to bregma; lateral, 3 mm to the midline; Ngai et al., 1988). To prevent overheating of the cerebral cortex during drilling, cold saline solution was suffused on the skull. The dura mater was gently removed and a 150-μm-thick quartz microscope coverglass was sealed to the bone with dental cement. The window inflow and outflow were assured by two needles secured in the dental cement of the window so that the brain parenchyma was continuously superfused with aCSF (Morii et al., 1986; Hudetz et al., 1995). The rate of superfusion was 0.5 mL/min controlled by a peristaltic pump. During superfusion the intracranial pressure (ICP) was maintained at 5 ± 1 mmHg and measured by a Pressure Transducer connected to a computer. The composition of the aCSF was: 119.0 mM NaCl, 2.5 mM KCl, 1.3 mM MgSO4·7H2O, 1.0 mM NaH2PO4, 26.2 mM NaHCO3, 2.5 mM CaCl2, and 11.0 mM glucose (equilibrated with 10.0% O2, 6.0% CO2, and 84.0% N2; pH 7.38 ± 0.02). The temperature was maintained at 37.0 ± 0.5°C.
The reduction of cerebral blood flow was produced by placement of two atraumatic microvascular clips for 30 min on common carotid arteries, previously isolated. After removing the clamp, the pial microcirculation was observed for 60 min (reperfusion period).
All experiments conform to the Guide for the Care and Use of Laboratory Animals published by the US National Institutes of Health (NIH Publication No. 85-23, revised 1996) and to institutional rules for the care and handling of experimental animals. The protocol was approved by the “Federico II” University of Naples Ethical Committee.
Intravital Microscopy and Microvascular Parameter Evaluation
Observations of pial vessels were conducted by a fluorescence microscope (Leitz Orthoplan) fitted with long-distance objectives [2.5×, numerical aperture (NA) 0.08; 10×, NA 0.20; 20×, NA 0.25; 32×, NA 0.40], a 10× eyepiece and a filter block (Ploemopak, Leitz). Epiillumination was provided by a 100 W mercury lamp using the appropriate filters for FITC, for rhodamine 6G and a heat filter (Leitz KG1). The pial microcirculation was televised with a DAGE MTI 300RC low-light level digital camera and recorded by a computer based frame grabber (Pinnacle DC 10 plus, Avid Technology, MA, USA).
Microvascular measurements were made off-line using a computer-assisted imaging software system (MIP Image, CNR, Institute of Clinical Physiology, Pisa, Italy). The visualization of pial microcirculation was carried out according to the protocol time schedule reported in Figure 1. Briefly, recording of microvascular images was performed for 1 min every 5 min during substance administration, before BCCAO and at the beginning of reperfusion. Afterward, recording was carried out every 10 min during BCCAO and the remaining reperfusion. The baseline conditions were represented by microvascular values detected within 2 min of FITC administration.
However, pial arteriole responses to the highest dose of quercetin and to the different substances were homogeneous during BCCAO and reperfusion. Therefore, we chose to present data recorded under the baseline conditions, at the end of BCCAO and at the end of reperfusion (RE).
Under baseline conditions, the arteriolar network was mapped by stop-frame images and pial arterioles were classified according to a centripetal ordering scheme (Strahler method, modified according to diameter), as previously described (Lapi et al., 2008b). In each animal one order 4 arteriole, two order 3, and two order 2 arterioles were studied during each experiment.
We tried to investigate the responses of each arteriolar order to the experimental conditions. However, because the responses were homogeneously distributed in the different arteriolar orders, we chose to present only the data regarding order 2 vessels, the most responsive arterioles. When there were differentiated arteriolar responses, we pointed out these differences for each experimental group. To clarify the time-dependent changes of arteriolar diameter we chose to present the different patterns of order 2 arteriole diameter responses only in Figure 2.
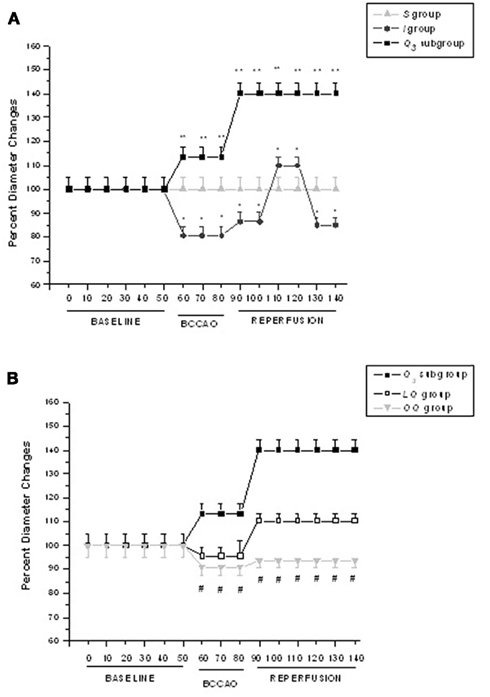
Figure 2. Time course plots of diameter changes in the experimental groups. (A) Diameter changes of order 2 arterioles, expressed as percent of baseline, under baseline conditions, during BCCAO and reperfusion in S = sham-operated group, I = ischemic group, and in Q3 = quercetin (5.0 mg/kg b.w.) subgroup; (B) Diameter changes of order 2 arterioles, expressed as percent of baseline, under baseline conditions, during BCCAO and reperfusion in Q3 = quercetin (5.0 mg/kg b.w.) subgroup, LQ = L-NAME + quercetin (5.0 mg/kg b.w.) group, OQ = 1H-(1, 2, 4) oxadiazolo (4, 3-alpha) quinoxalin-1-one (ODQ) + quercetin (5.0 mg/kg b.w.) group.°p < 0.01 vs. baseline; *p < 0.01 vs. I group; #p < 0.01 vs. Q3 subgroup.
Arteriolar diameters were measured with a computer-assisted method (MIP Image program, frame by frame). The results of diameter measurements were in accord with those obtained by shearing method (±0.5 μm). To avoid bias due to single operator measurements, two independent “blinded” operators measured the vessel diameters. Their measurements overlapped in all cases.
The increase in permeability was calculated and reported as normalized gray levels (NGL): NGL = (I–Ir)/Ir, where Ir is the average baseline gray level at the end of vessel filling with fluorescence (average of five windows located outside the blood vessels with the same windows being used throughout the experimental procedure), and I is the same parameter at the end of BCCAO or RE. Gray levels ranging from 0 to 255 were determined by the MIP Image program in five regions of interest (ROI) measuring 50 μm × 50 μm (10× objective). The same location of ROI during recordings along the microvascular networks was provided by a computer-assisted device for XY movement of the microscope table.
Adherent leukocytes (i.e., cells on vessel walls that did not move over a 30-s observation period) were quantified in terms of number/100 μm of venular length (v.l.)/30 s using higher magnification (20× and 32×, microscope objectives). In each experimental group 45 venules were studied.
Perfused capillary length (PCL) was measured by MIP image in an area of 150 μm × 150 μm. In this system the length of perfused capillaries is easily established by the automated process because it is outlined by dextran (Colantuoni et al., 2005).
Mean arterial blood pressure (Viggo-Spectramed P10E2 transducer – Oxnard, CA, USA – connected to a catheter in the femoral artery) and heart rate were monitored with a Gould Windograf recorder (model 13-6615-10S, Gould, OH, USA). Data were recorded and stored in a computer. Blood gas measurements were carried out on arterial blood samples withdrawn from arterial catheter at 30 min time period intervals (ABL5; Radiometer, Copenhagen, Denmark). The hematocrit was measured under baseline conditions, at the end of BCCAO and at RE.
Furthermore, to determine the degree of perfusion decrease during BCCAO, microvascular blood flow by laser Doppler perfusion monitoring (LDPM) was measured on the skull of all animals by a Perimed PF5001 flowmeter, using a probe (407; Perimed, Sweden) attached to the bone. The sampling rate was 32 Hz and blood flow was expressed as perfusion units (PU).
Finally, single pial venule blood flow, Q, was calculated according to the following equation: , where α was a constant, related to the vessel diameter, VCL was the red blood cell centerline velocity and A was the cross-sectional area. However, we chose to present only LDPM and venular blood flow data of ischemic rats (I group) and quercetin-treated animals (Q3 subgroup) to simplify data presentation.
Oxygen-Reactive Species Quantification
Artificial cerebrospinal fluid containing 250 mM DCFH-DA at 37.0 ± 0.5°C was superfused over the pial surface. The lipophilic DCFH-DA is a stable non-fluorescent probe with a high cellular permeability. DCFH-DA reacts with intracellular radicals to be converted to its fluorescent product (DCF). The remaining extracellular DCFH-DA was washed out with aCSF. The intensity of DCF fluorescence is proportional to the intracellular ROS level.
The fluorescence intensity was determined by the use of an appropriate filter (522 nm) and the evaluation of NGL, with the baseline represented by pial surface just superfused by DCFH-DA (Watanabe, 1998).
Western Blot Analysis
Protein concentrations were determined by the Bio-Rad protein assay (Bio-Rad). To detect the proteins of interest, specific antibodies: anti-eNOS (mouse monoclonal antibody, 1:200, Santa Cruz Biotechnology, Santa Cruz, CA, USA), anti-phosphorylated eNOS [Rabbit (polyclonal) Anti-eNOS (pS116) phosphospecific antibody unconjugated, Invitrogen] and anti-β-actin (mouse monoclonal, 1:1000, Sigma) were used. Immunoreaction was revealed using anti-mouse IgG conjugated to peroxidase, 1:2000 (GE Healthcare) by the ECL reagent (GE Healthcare). The optical density of the bands was determined by Chemi Doc Imaging System (Bio-Rad) and normalized to the optical density of β-actin. eNOS and phosphorylated eNOS were evaluated to define concentrations of the expressed and active proteins, respectively.
2, 3, 5-Triphenyltetrazolium Chloride (TTC) Staining
Rats were sacrificed after 30 min BCCAO and 60 min reperfusion. Tissue damage was evaluated by 2,3,5-Triphenyltetrazolium Chloride (TTC) staining. The brains were cut into 1 mm coronal slices with a vibratome (Campden Instrument, 752 M). Sections were incubated in 2% TTC for 20 min at 37°C and in 10% formalin overnight. The necrotic area site and extent in each section were evaluated by image analysis software (Image-Pro Plus; Bederson et al., 1986).
Statistical Analysis
All reported values are means ± SD. Data were tested for normal distribution with the Kolmogorov–Smirnov test. Parametric (Student’s t tests, ANOVA, and Bonferroni post hoc test) or non-parametric tests (Wilcoxon, Mann–Whitney, and Kruskal–Wallis tests) were used; non-parametric tests were applied to compare diameter and length data among experimental groups. Due to the small sample size of DCFH-DA treated rats we used non-parametric tests to compare the results obtained in these animals. The statistical analysis was carried out by SPSS 14.0 statistical package. Statistical significance was set at p < 0.05.
Results
Under baseline conditions pial arterioles were classified in five orders according to diameter, length, and branching. Capillaries, assigned order 0, originated from the smallest arterioles (order 1, average diameter: 16.4 ± 2.1 μm and average length: 155 ± 70 μm; n = 160). The largest pial arterioles, order 5, showed diameter of 62.8 ± 4.8 μm and length of 1114 ± 350 μm (n = 96). The values of diameters and lengths were significantly different among the different arteriolar orders (p < 0.01; Lapi et al., 2008b).
Sham-Operated Animals
Sham-operated animals did not show changes in arteriolar diameter nor increase in leakage nor adhesion of leukocytes nor decrease in capillary perfusion during the same period of observation as in the other experimental groups.
The rats treated with DCFH-DA superfusion (n = 3) showed no significant increase in DCF fluorescence intensity at the end of observations (0.03 ± 0.01 NGL), indicating no ROS formation.
The sham-operated animals treated with quercetin (at the doses of 1.0 or 2.5 or 5.0 mg/kg b.w.) showed a dose-dependent dilatation of all arterioles (order 2 arterioles: by 12 ± 3% of baseline, at the highest dosage, p < 0.01 vs. baseline), but no significant changes in the other parameters when compared with baseline (Table 3). L-NAME, ODQ, 7-nitroindazole, and L-N6-(1-iminoethyl) lysine chloride did not significantly affect diameter of vessels nor the other parameters.
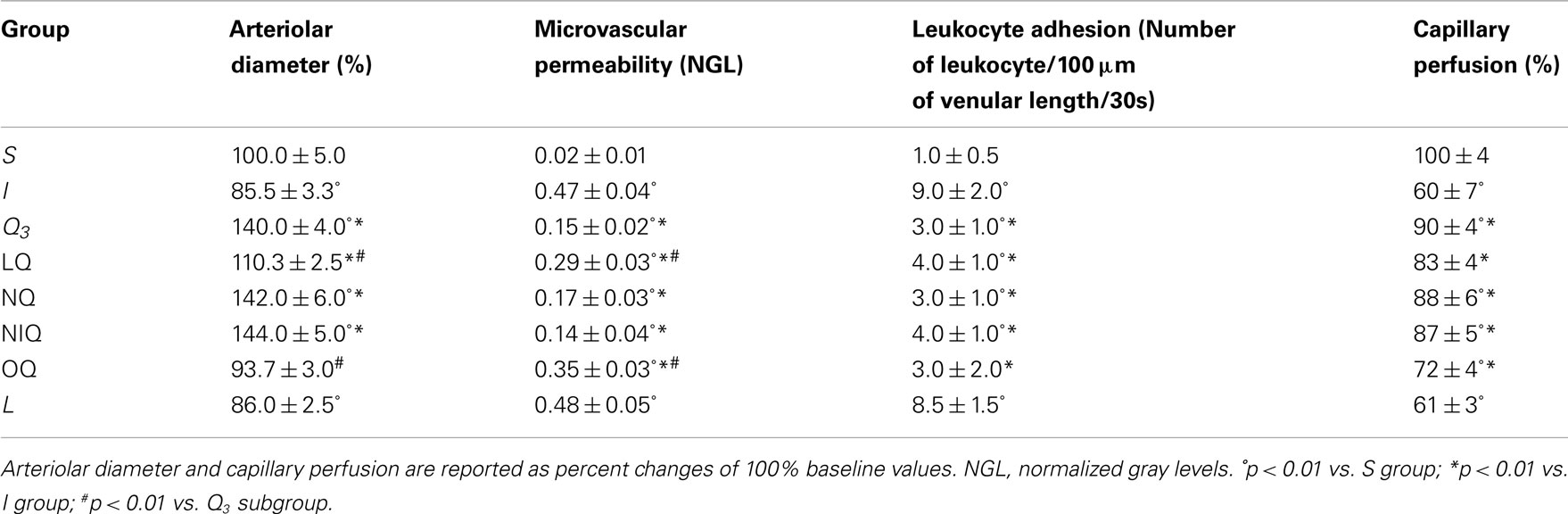
Table 3. Variations of the main parameters in sham-operated (S) group, ischemic (I) group, and quercetin (Q3) subgroup (5 mg/Kg b.w.), NG-nitro-L-arginine-methyl ester plus quercetin (LQ) group, 7-nitroindazole plus quercetin (NQ) group, L-N6-(l-iminoethyl) lysine hydrochloride plus quercetinA (NIQ), and 1H-(1, 2, 4) oxadiazolo (4, 3-alpha) quinoxalin-1-one plus quercetin (OQ) group at the end of reperfusion.
BCCAO-Reperfusion
At the end of BCCAO ischemic animals (group I) showed a decrease in diameter of all arteriolar orders; in order 2 vessels the reduction was by 19.0 ± 3.2% of baseline (Figure 2A), while MABP did not significantly change compared with baseline (Table 4). Venular permeability significantly increased compared with baseline (p < 0.01); no changes in venular diameter were detected, while most capillaries were not perfused.
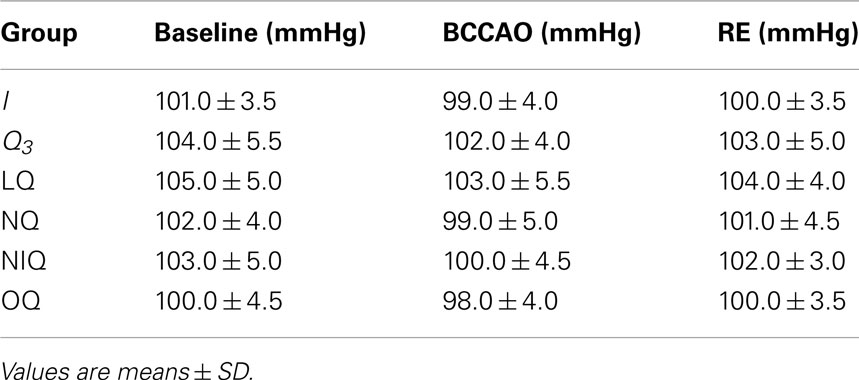
Table 4. Mean arterial blood pressure (MABP) under baseline conditions, at the end of bilateral common carotid artery occlusion (BCCAO) and at the end of reperfusion (RE) in sham-operated (S) group, ischemic (I) group, and quercetin (Q3) subgroup (5 mg/kg b.w.), NG-nitro-L-arginine-methyl ester plus quercetin (LQ) group, 7-nitroindazole plus quercetin (NQ) group, L-N6-(l-iminoethyl) lysine hydrochloride plus quercetina (NIQ), and 1H-(1, 2, 4) oxadiazolo (4, 3-alpha) quinoxalin-1-one plus quercetin (OQ) group.
At reperfusion, all arteriolar orders presented a triphasic response, because at first order 2 arterioles constricted by 13.5 ± 3.2% of baseline; then dilated by 10.0 ± 2.2% of baseline; at RE they constricted again by 14.5 ± 3.3% of baseline (Figure 2A). MABP did not change at all measurement points (Table 4). Venular diameter did not vary; venular permeability significantly increased (Figures 3A,B; p < 0.01 compared with baseline) and leukocyte adhesion was marked (9.0 ± 2.0/100 μm v.l./30 s; p < 0.01 vs. baseline) in venules (diameter 20–50 μm, n = 45), while capillary perfusion length (PCL) decreased (p < 0.01 vs. baseline; Table 3).
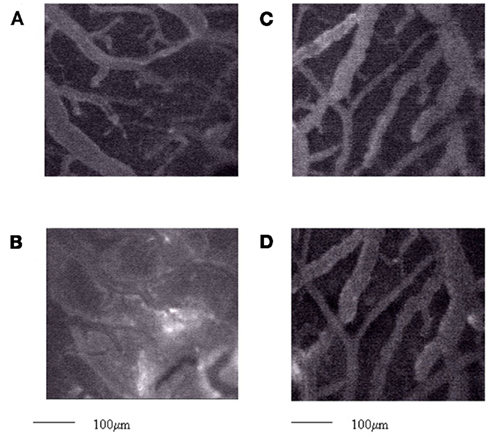
Figure 3. Computer-assisted image of a pial microvascular network under baseline conditions (A) and at the end of reperfusion (B) in an ischemic rat. The increase in permeability is outlined by the marked change in the color of interstitium (from black to white). Computer-assisted image of a pial microvascular network under baseline conditions (C) and at the end of reperfusion (D) in a quercetin-treated rat (5.0 mg/kg b.w.), where there was no leakage of fluorescent-dextran. Scale bar = 100 μm.
At the end of BCCAO, microvascular blood flow recorded by LDPM on the contralateral parietal skull was reduced by 60.5 ± 4.5% of baseline (p < 0.01 vs. baseline, 36.2 ± 3.5 PU). In single pial venules (SPV, diameter 30–40 μm) the blood flow was reduced by 63.5 ± 6% of baseline (p < 0.01 vs. baseline: 247 ± 10 nL/s). At the beginning of reperfusion there was an increase in blood flow (reactive hyperemia), but within 6 ± 2 min the microvascular blood flow, recorded by LDPM, decreased by 35.0 ± 3.5% of baseline up to the end of observations. At RE, SPV blood flow was reduced by 30 ± 7% of baseline (p < 0.01 vs. baseline).
The rats treated with DCFH-DA superfusion (n = 3) showed an increased DCF fluorescence intensity at the end of BCCAO (0.20 ± 0.03 NGL, p < 0.01 vs. S group), indicating an increase in ROS formation. The fluorescence was higher at RE in the remaining animals (n = 3; 0.32 ± 0.04 NGL, p < 0.01 vs. S group) showing a further increase in ROS production.
Quercetin
Quercetin, administered at the doses of 1.0 or 2.5 or 5.0 mg/kg b.w. (subgroup Q1, Q2, and Q3, respectively), caused dose-dependent effects. At the highest dose, there was an increase in all arteriolar diameters at the end of BCCAO: by 13.4 ± 3.4% of baseline in order 2 (p < 0.01 vs. I group; Figure 2A). No significant changes in MABP were detected (Table 4). Leakage was significantly reduced compared with ischemic rats (p < 0.01).
At RE all pial arterioles dilated: by 40 ± 4% of baseline in order 2 (p < 0.01 vs. I group; Figure 2A). MABP did not significantly change at this time of observation (Table 4). Leakage of FD70 was prevented as well as leukocyte adhesion (Figures 3C,D; p < 0.01 vs. I group), while capillary perfusion was protected (p < 0.01 vs. I group; Table 3).
Quercetin highest dosage increased microvascular blood flow by 8 ± 2% of baseline (p < 0.01 vs. baseline) as detected by LDPM on the contralateral parietal skull prior to BCCAO. At the end of BCCAO, LDPM measurements indicated that quercetin prevented the marked reduction in microvascular blood flow as observed in ischemic rats. The decrease was by 25 ± 5% of baseline (p < 0.01 vs. baseline and I group). Similar results were found for SPV blood flow, reduced by 33.5 ± 5.0% of baseline: 235 ± 12 nl/s, p < 0.01 vs. baseline and I group, at the end of BCCAO.
At the beginning of reperfusion there was steep increase in blood flow (reactive hyperemia); at the end of reperfusion blood flow increased by 39 ± 4% of baseline (p < 0.01 vs. baseline and I group). At RE, SPV blood flow was significantly increased by 50 ± 6% of baseline (p < 0.01 vs. baseline); the difference was statistically significant when compared with ischemic rat response.
In the animals treated with DCFH-DA, the effects of quercetin were dose-dependent. At the highest dosage, quercetin decreased ROS formation at the end of BCCAO and at RE: NGL, indeed, were 0.08 ± 0.03 (p < 0.01 vs. I group, n = 3) and 0.16 ± 0.03 (p < 0.01 vs. I group, n = 3), respectively.
NO Synthase Inhibition Plus Quercetin
At the end of BCCAO, L-NAME prior to quercetin (group LQ) caused reduction in diameter of all arteriolar orders: by 4.5 ± 1.0% of baseline in order 2 (p < 0.05 vs. I and p < 0.01 vs. Q3 groups; Figure 2B), while MABP did not significantly change (Table 4). Leakage was reduced compared with ischemic animals (p < 0.01 vs. I group).
At RE, all arterioles dilated: by 10.3 ± 2.5% of baseline in order 2 (p < 0.01 vs. I group; Figure 2B). MABP did not significantly vary (Table 4). The protective effect of quercetin on leakage was attenuated (p < 0.01, vs. I and Q3 groups). The adherent leukocytes were reduced and PCL decreased (p < 0.01 vs. I group; Table 3). L-NAME administered 10 min before quercetin, prior to BCCAO, did not significantly affect arteriolar diameter and microvascular permeability.
Neuronal NO Synthase Inhibition Plus Quercetin
The inhibition of nNOS by 7-nitroindazole prior to quercetin (group NQ) did not affect quercetin-induced responses (Table 3).
Inducible NO Synthase Inhibition Plus Quercetin
The selective inhibition of iNOS by L-N6-(1-iminoethyl) lysine hydrochloride prior to quercetin (group NIQ) did not influence quercetin-induced effects (Table 3).
Guanylyl Cyclase Inhibition Plus Quercetin
At the end of BCCAO, ODQ prior to quercetin (group OQ) induced a decrease in diameter of all arterioles: by 9.0 ± 3.2% of baseline in order 2 (p < 0.01 vs. Q3 subgroup; Figure 2B), but MABP did not significantly change (Table 4). Leakage increased as in ischemic animals (p = NS vs. I group).
At RE all arterioles constricted: by 6.3 ± 3.0% of baseline in order 2 (p < 0.01 vs. Q3 subgroup; Figure 2B), without significant changes in MABP (Table 4). Leakage was slightly attenuated (p < 0.01 vs. I and Q3 groups), while the adherent leukocytes were reduced in number (p < 0.01 vs. I group) and capillary perfusion was higher than in ischemic animals (p < 0.01 vs. I and Q3 groups; Table 3). ODQ administered 10 min before quercetin prior to BCCAO did not significantly affect arteriolar diameter and microvascular permeability.
L-NAME prior to BCCAO and Reperfusion
The inhibition of NOS by L-NAME (L group) did not significantly influence the microvascular changes observed in rats submitted to BCCAO and reperfusion (Table 3).
Finally, physiological parameters, such as hematocrit, MABP, heart rate, pH, PCO2, and PO2 did not change in the different experimental groups up to RE.
eNOS Expression
Western blot analysis showed that at the end of reperfusion eNOS protein concentration significantly increased in animals treated with quercetin compared with I and S group. The eNOS protein concentrations was the higher in hippocampus and cortex, while the lower was detected in striatum of all animals. Both total and phosphorylated eNOS proteins increased to the same extent. In S group the striatum eNOS protein concentration was the highest compared with the other groups (Figure 4).
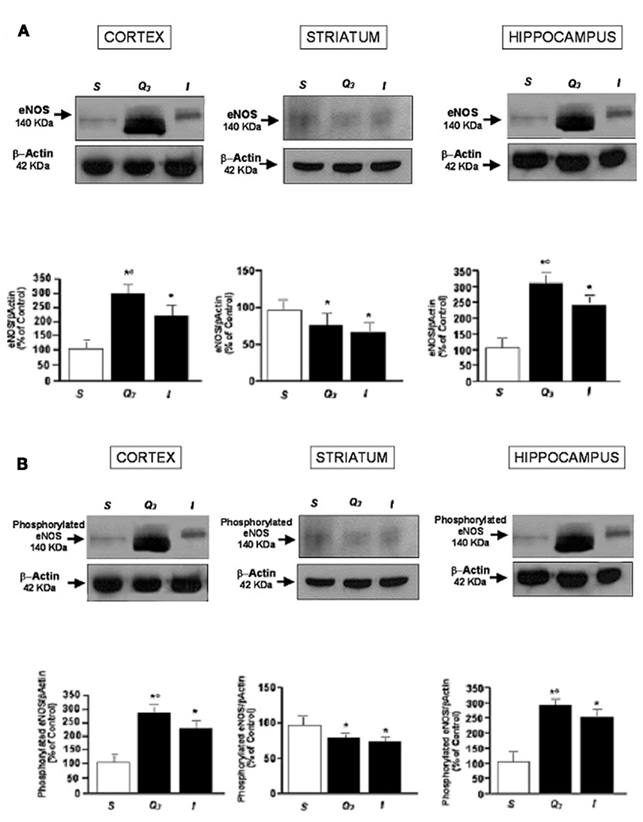
Figure 4. Western blotting of eNOS expression (A) and phosphorylated eNOS expression (B) in three cerebral zones (cortex, striatum, hippocampus) at the end of reperfusion in sham- operated group, in Q3, quercetin-treated (5.0 mg/kg b.w.) subgroup and in I group, and the corresponding densitometric values (mean ± SD). *p < 0.01 vs. S group,°p < 0.01 vs. I group.
Tissue Damage Evaluation
The neuroprotective effects of quercetin administration were assessed by evaluating damaged area after 30 min BCCAO and 60 min reperfusion. Ischemic rats showed a clear lesion, index of striatum neuronal loss in both hemispheres, while cortex did not show any significant macroscopic damage. Highest dose quercetin significantly reduced neuronal injury compared with that observed in ischemic animals (Figure 5).
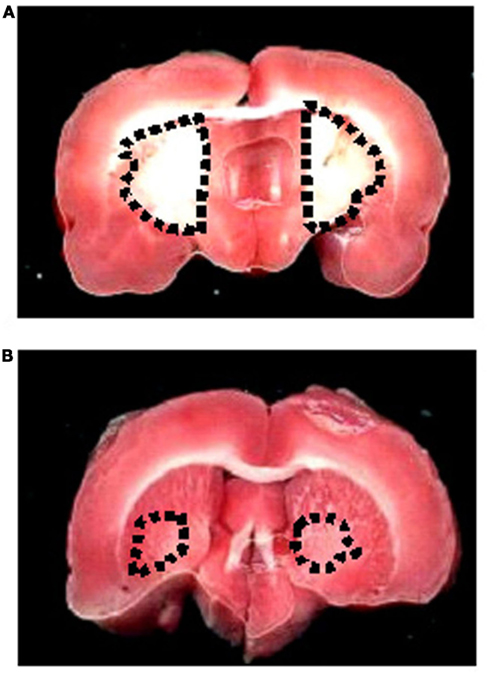
Figure 5. TTC staining of coronal brain slice from a rat submitted to BCCAO and reperfusion. The lesion in the striatum is outlined by the dashed black line (A). TTC staining of coronal brain slice from a rat treated with highest dose quercetin (5.0 mg/kg b.w.; subgroup Q3) and submitted to BCCAO and reperfusion, where there was reduced injury (B).
Discussion
The data reported in the present study indicate that the bilateral occlusion of common carotid artery induced marked modifications of pial microvasculature affecting the microenvironment vital for the central nervous system functions.
In ischemic rats pial arteriolar diameter decreased during hypoperfusion while during reperfusion the arterioles showed an interesting triphasic response. It is reasonable to suggest that the changes in vascular tone were due to smooth muscle cell activity, contracting at the beginning of reperfusion in response to the increase in blood pressure. Then, they relaxed for several minutes and constricted again at the end of reperfusion. These responses might be related to myogenic mechanisms; however, in additional experiments we observed myosin light chain expression did not increase, while was enhanced myosin light chain phosphorylation (data not reported). LDPM recordings, indeed, indicated an increase in microvascular blood flow at the beginning of reperfusion, followed by a decrease after few minutes. Therefore, reperfusion caused reactive hyperemia, as observed in different experimental models, and consequent changes in vessel diameter (Hori and Kitakade, 1991; Tsapenko et al., 2001). However, there were no significant variations in the MABP recorded through the femoral artery.
Microvascular permeability was pronounced indicating a severe impairment of the blood–brain barrier; the venular vessels showed many adherent leukocytes and almost half of the capillaries were not perfused. In our preparation we found an increase in leukocyte adhesion as observed primarily in the model of transient middle cerebral artery occlusion in animals implanted with a closed cranial window (Del Zoppo, 1994). Discrepancies in leukocyte sticking, reported in different experimental conditions, could be related to the different time period of ischemia, as discussed by Abels et al. (2000). Our data indicate that leukocyte adhesion may be facilitated by at least 30 min of hypoperfusion.
Bilateral common carotid artery occlusion model was introduced by Eklöf and Siesjö (1972, 1973) to investigate cerebral circulation damage and neuronal function impairment. Iwasaki et al. (1989) demonstrated that 30 min of BCCAO are sufficient to cause neuronal injury in the hippocampus and cerebral cortex.
Quercetin was dose-dependently able to ameliorate pial microvascular alterations caused by BCCAO and reperfusion. During hypoperfusion the pial arterioles showed a slight increase in diameter, but a significant dilation at the end of reperfusion; microvascular permeability was prevented as well as adhesion of leukocytes to venular walls. Most capillaries were perfused when compared with ischemic animals. These findings corresponded to LDPM measurements and single venule blood flow estimation: under baseline conditions, quercetin was able to increase blood flow; at the end of BCCAO microvascular blood flow was significantly higher than in ischemic animals; finally, at the end of reperfusion the increase in perfusion was highly significant. These hemodynamic changes were accompanied by the increase in eNOS expression, both total and phosphorylated eNOS proteins, in hippocampus, and cerebral cortex induced by quercetin, as detected by Western blotting. Finally, we observed highest dose quercetin reduced striatum neuronal damage induced by BCCAO and reperfusion, as detected by TTC staining.
The arteriolar dilation triggered by quercetin, higher at the end of reperfusion than in sham-operated animals, may be strengthened by reactive hyperemia after transient ischemia, as observed in experimental models or in human forearm after high pressure cuff inflation. In this case, it is possible to observe a peak increase in blood flow after brachial artery occlusion for few minutes. The higher reactivity caused by quercetin may be due to NO release by arteriolar walls, facilitated in this response by changes in vascular hemodynamics during recovery of reperfusion after brain hypoperfusion. These effects might have been produced by shear stress and consequent NO release, because of larger NOS expression, quickly triggered within 30 min of hypoperfusion and 60 min of reperfusion.
Quercetin, a key representative flavonoid molecule of the polyphenol compounds present in fruits and vegetables, has been widely studied, showing beneficial and deleterious effects on different cell types (Dajas et al., 2003). This flavonoid presents dose-related biphasic effects, because low concentrations of quercetin are able to activate some intracellular signaling pathways, inhibited at high doses (Chen and Pace-Asciak, 1996). In particular, protein kinase C and tyrosine kinase signaling pathways have been reported to be modulated by quercetin in different experimental models (Picq et al., 1989; Cogolludo et al., 2007; Negash et al., 2007; Chiwororo and Ojewole, 2010). However, after so many studies quercetin’s mechanism of action remains unclear.
Previous study has demonstrated that during ischemia–reperfusion injury in rabbit hind limb (2.5 h ischemia, 2 h reperfusion), quercetin protects skeletal muscle by scavenging superoxide, and enhancing cytoprotective nitric oxide concentration. Moreover, Shutenko et al. (1999) confirmed that the intravenous administration of quercetin in rats subjected to ischemia and reperfusion increased the NO levels in the cerebral cortex. On the other hand, inhibition of eNOS by L-NAME did not influence dilation induced by quercetin in isolated rat thoracic aorta.
To clarify the intracellular pathways involved in the quercetin-induced vasodilation, we tested, as first approach, the effects of L-NAME, eNOS inhibitor, prior to quercetin. We observed that the inhibition of eNOS caused a significant attenuation of arterial dilation induced by quercetin, with arterioles dilating by 10.3 ± 2.5% of baseline. The quercetin’s protective effect on venular permeability was reduced while leukocyte adhesion decreased. Consequently, quercetin effects appear to be complex, likely related to more than one intracellular signaling pathway. L-NAME dosage we used did not cause a significant increase in arterial blood pressure in our experiments as previously detected (Moreau et al., 1995).
The guanylyl cyclase inhibition by ODQ before quercetin, administered prior to BCCAO and at reperfusion beginning, caused moderate constriction of all pial arteriolar orders, indicating that the main effect of quercetin on arteriolar diameter was mediated by NO release. The consequent activation of guanylyl cyclase triggered the vessel smooth muscle cell relaxation. Moreover, ODQ caused increase in permeability of blood–brain barrier and decrease in capillary perfusion. However, ODQ did not affect leukocyte adhesion, prevented to the same extent as by quercetin alone. Interestingly nNOS and iNOS inhibition did not affect protection exerted by quercetin.
It is important to note NO exerts several functions activating or inhibiting different intracellular pathways. NO derived from endothelial cells causes vasodilation, decrease in vascular resistance and blood pressure, inhibition of platelet aggregation and adhesion, inhibition of leukocyte adhesion and migration. These NO functions are mediated by cyclic GMP synthesized through soluble guanylyl cyclase, a heme-containing enzyme, which is directly activated by NO (Toda et al., 2009).
Previous experiments carried out by in vitro methods indicated the scavenger activity of quercetin (Lopez et al., 2004; Moreira et al., 2004; Cho et al., 2006). Recently, Ahmad et al. (2011) showed quercetin administration in rats submitted to transient middle cerebral artery occlusion upregulates the antioxidant status and lowers thiobarbituric acid reactive substance (TBARS) levels, reducing the infarct size and neurological deficits. We tried to assess the in vivo effects of quercetin on ROS formation utilizing the fluorescent DCF, able to reveal ROS generation in tissues. Our data show that quercetin was dose-dependently able to decrease fluorescent spots located outside the vessels; the decrease was significant when compared with DCF fluorescence observed in ischemic rats. Therefore, quercetin may significantly reduce ROS formation through a direct scavenger activity. The present paper confirm that 30 min of BCCAO and 60 min of reperfusion cause ROS formation, able to cause lipoperoxidation, as reported by Yampallewar et al. (2004). However, the small sample size of our study requires further experiments to increase the number of observations.
All these data indicate that NO release triggered by quercetin may play a key role for dilation of arterioles and preservation of blood–brain barrier, facilitating capillary perfusion, while leukocyte adhesion appears to be mainly related to ROS formation. The increase in ROS may facilitate adhesion of leukocytes to venular walls, contributing to blood–brain barrier disruption and increase in tissue damage due to increase in perivascular edema.
Therefore, our results are in agreement with previous findings (Shutenko et al., 1999; Ahmad et al., 2011) supporting quercetin-induced protective effects by NO release modulation and scavenger activity. However, it is worth noting for the first time in the present study quercetin was shown to prevent leukocyte adhesion and capillary perfusion decrease induced by hypoperfusion and reperfusion in pial networks.
In conclusion, in the present experimental preparation quercetin was able to prevent permeability increase and leukocyte sticking. These effects accompanied an increase in arteriolar diameter and protection of capillary perfusion. Our data indicate that quercetin’s effect may be observed in the in vivo rat model of brain hypoperfusion and reperfusion. These quercetin’s properties appear to be related mostly to an increase in eNOS expression, NO release, and activity. The resulting arteriolar dilation can contribute to the increase in arteriolar shear stress, known to enhance further release of NO with the decrease in platelet and leukocyte adhesion and increase in capillary viability. Finally, quercetin scavenger activity, which reduces formation of ROS known to be deleterious to vessel walls and to blood–brain barrier, contributes to the overall protection exerted by quercetin.
Conflict of Interest Statement
The authors declare that the research was conducted in the absence of any commercial or financial relationships that could be construed as a potential conflict of interest.
References
Abels, C., Röhrich, F., Corvin, S., Meyermann, R., Baethmann, A., and Schürer, L. (2000). Leukocyte-endothelium-interaction in pial vessels following global, cerebral ischaemia. Acta Neurochir. 142, 333–339.
Ahmad, A., Khan, M. M., Hoda, M. N., Raza, S. S., Khan, M. B., Javed, H., Ishrat, T., Ashafaq, M., Ahmad, M. E., Safhi, M. M., and Islam, F. (2011). Quercetin protects against oxidative stress associated damages in a rat model of transient focal cerebral ischemia. Neurochem. Res. 36, 1360–1371.
Ajay, M., Gilani, A. H., and Mustafa, M. R. (2003). Effects of flavonoids on vascular smooth muscle of the isolated rat thoracic aorta. Life Sci. 74, 603–612.
Bederson, J. B., Pitts, L. H., Germano, S. M., Nishimura, M. C., Davis, R. L., and Bartkowski, H. M. (1986). Evaluation of 2,3,5-triphenyltetrazolium chloride as a stain for detection and quantification of experimental cerebral infarction in rats. Stroke 17, 1304–1308.
Bush, M. A., and Pollak, G. M. (2000). Phamacokinetics and protein binding of the selective neuronal nitric oxide synthase inhibitor 7-nitroindazole. Biopharm. Drug Dispos. 21, 221–228.
Chen, C. K., and Pace-Asciak, C. R. (1996). Vasorelaxing activity of resveratrol and quercetin in isolated rat aorta. Gen. Pharmacol. 27, 363–366.
Chiwororo, W. D. H., and Ojewole, J. A. O. (2010). Dual effects of quercetin on rat isolated portal vein smooth muscle contractility. Cardiovasc. J. Afr. 21, 132–136.
Cho, J. Y., Kim, I. S., Jang, Y. H., Kim, A. R., and Lee, S. R. (2006). Protective effect of quercetin, a natural flavonoid against neuronal damage after transient global cerebral ischemia. Neurosci. Lett. 404, 330–335.
Cogolludo, A., Frazziano, G., Briones, A. M., Cobeno, L., Moreno, L., Lodi, F., Salaices, M., Tamargo, J., and Perez-Vizcaino, F. (2007). The dietary flavonoid quercetin activates BKCa currents in coronary arteries via production H2O2. Role in vasodilation. Cardiovasc. Res. 73, 424–431.
Colantuoni, A., Lapi, D., Paterni, M., and Marchiafava, P. L. (2005). Protective effects of insulin during ischemia-reperfusion injury in hamster cheek pouch microcirculation. J. Vasc. Res. 42, 55–66.
Dajas, F., Rivera-Megret, F., Blasina, F., Arredondo, F., Abin-Carriquiry, J. A., Costa, G., Echeverry, C., Lafon, L., Heizen, H., Ferreira, M., and Morquio, A. (2003). Neuroprotection by flavonoids. Braz. J. Med. Biol. Res. 36, 1613–1620.
Del Zoppo, G. J. (1994). Microvascular changes during cerebral ischemia and reperfusion. Cerebovasc. Brain Metab. Rev. 6, 47–96.
Dugan, L., and Kim-Han, J. S. (2006). Hypoxic-ischemic brain injury and oxidative stress. Trans. Am. Soc. Neurochem. 32, 559–569.
Eklöf, B., and Siesjö, B. K. (1972). The effects of bilateral carotid artery ligation upon the blood flow and the energy state of the rat. Acta Physiol. Scand. 86, 1755–1765.
Eklöf, B., and Siesjö, B. K. (1973). Cerebral blood flow in ischemia caused by carotid artery ligation in the rat. Acta Physiol. Scand. 87, 69–77.
Hori, M., and Kitakade, M. (1991). Adenosine, the heart, and coronary circulation. Hypertension 18, 565–574.
Hudetz, A. G., Feher, G., Weigle, C. G. M., Knese, D. E., and Kampine, J. P. (1995). Video microscopy of cerebrocortical capillary flow: response to hypotension and intracranial hypertension. Am. J. Physiol. 268, H2202–H2210.
Ikeda, K., Negishi, H., and Yamori, Y. (2003). Antioxidant nutrients and hypoxia/ischemia brain injury in rodents. Toxicology 189, 55–61.
Iwasaki, Y., Ito, S., Suzuki, M., Nagahori, T., Yamamoto, T., and Konno, H. (1989). Forebrain ischemia induced by temporary bilateral common carotid occlusion in normotensive rats. J. Neurol. Sci. 90, 155–165.
Kumura, E., Yoshimine, T., Iwatsuki, K. I., Yamanaka, K., Tanaka, S., Hayakawa, T., Shiga, T., and Kosaka, H. (1996). Generation of nitric oxide and superoxide during reperfusion after focal cerebral ischemia in rats. Am. J. Physiol. 270, C748–C752.
Lapi, D., Marchiafava, P. L., and Colantuoni, A. (2008a). Pial microvascular responses to transient bilateral common carotid artery occlusion: effects of hypertonic glycerol. J. Vasc. Res. 45, 89–102.
Lapi, D., Marchiafava, P. L., and Colantuoni, A. (2008b). Geometric Characteristics of arterial network of rat pial microcirculation. J. Vasc. Res. 45, 69–77.
Lopez, G., Moreno, L., and Cogolludo, A. (2004). Nitric oxide (NO) scavenging and NO protecting effects of quercetin and their biological significance in vascular smooth muscle. Mol. Pharmacol. 65, 851–859.
Moreau, P., Takasa, H., Küng, C. F., van Rooijen, M. M., Schaffner, T., and Lüscher, T. F. (1995). Structure and function of the rat basilar artery during chronic nitric oxide synthase inhibition. Stroke 26, 1922–1928.
Moreira, A. J., Fraga, C., Alonso, M., Collado, P. S., Zetller, C., Marroni, C., Marroni, N., and Gonzàlez-Gallego, J. (2004). Quercetin prevents oxidative stress and NF-kappaB activation in gastric mucosa of portal hypertensive rats. Biochem. Pharmacol. 68, 1939–1946.
Morii, S., Ngai, A. C., and Winn, R. (1986). Reactivity of rat pial arterioles and venules to adenosine and carbon dioxine: with detailed description of the closed cranial window technique in rats. J. Cereb. Blood Flow Metab. 6, 34–41.
Nakashima, M., Niwa, M., Iwai, T., and Uematsu, T. (1999). Involvement of free radicals in cerebral vascular reperfusion injury evaluated in a transient focal cerebral ischemia model of rat. Free Radic. Biol. Med. 26, 722–729.
Negash, S., Gao, Y., Zhou, W., Liu, J., Chinta, S., and Raj, J. U. (2007). Regulation of cGMP-dependent protein kinase-mediated vasodilation by hypoxia-induced reactive species in ovine fetal pulmonary veins. Am. J. Physiol. Lung Cell Mol. Physiol. 293, L1012–L1020.
Ngai, A. C., Ko, K. R., Morii, S., and Winn, H. R. (1988). Effect of sciatic nerve stimulation on pial arterioles in rats. Am. J. Physiol. 254, H133–H139.
Picq, M., Dubois, M., Munari-Silem, Y., Prigent, A. F., and Pacheco, H. (1989). Flavonoid modulation of protein kinase C activation. Life Sci. 44, 1563–1571.
Sakamoto, K., Yonoki, Y., Kubota, Y., Kuwagata, M., Saito, M., Nakahara, T., and Ishii, K. (2005). Inducible nitric oxide synthase inhibitors abolished histological protection by late ischemic preconditioning in rat retina. Exp. Eye Res. 82, 512–518.
Shutenko, Z., Henry, Y., Pinard, E., Seylaz, J., Potier, P., Berthet, F., Girard, P., and Sercombe, R. (1999). Influence of the antioxidant quercetin in vivo on the level of nitric oxide determined by electron paramagnetic resonance in rat brain during global ischemia and reperfusion. Biochem. Pharmacol. 57, 199–208.
Toda, N., Ayajiki, K., and Okamura, T. (2009). Cerebral blood flow regulation by nitric oxide: recent advances. Pharmacol. Rev. 61, 62–97.
Tsapenko, M. V., Weber, M., Moser, A., Keupp, M., Timmermann, W., Debus, S., Thiede, A., and Henrich, H. A. (2001). Restoration of blood circulation in reperfusion in ischemic tissues. Klin. Khir. 1, 45–47.
Watanabe, S. (1998). In vivo fluorometric measurement of cerebral oxidative stress using 2’-7’-dichlorofluorescein (DCF). Keio J. Med. 47, 92–98.
Xu, H. L., Feinstein, D. L., Santizo, R. A., Koenig, H. M., and Pelligrino, D. A. (2002). Agonist-specific differences in mechanisms mediating eNOS-dependent pial arteriolar dilation in rats. Am. J. Physiol. Heart Circ. Physiol. 282, H237–H243.
Yampallewar, S. U., Hota, D., Rai, S., Kumar, M., and Acharya, S. B. (2004). Nimodipine attenuates biochemical, behavioural and histopatological alterations induced by acute transient and long term bilateral common carotid occlusion in rats. Pharmacol. Res. 49, 143–150.
Keywords: bilateral common carotid artery occlusion, reperfusion, pial microcirculation, quercetin, endothelial nitric oxide, vasodilation
Citation: Lapi D, Vagnani S, Pignataro G, Esposito E, Paterni M and Colantuoni A (2012) Protective effects of quercetin on rat pial microvascular changes during transient bilateral common carotid artery occlusion and reperfusion. Front. Physio. 3:32. doi: 10.3389/fphys.2012.00032
Received: 07 September 2011; Accepted: 06 February 2012;
Published online: 01 March 2012.
Edited by:
Steffen-Sebastian Bolz, University of Toronto, CanadaReviewed by:
Lina Badimon, Cardiovascular Research Center, CSIC-ICCC, SpainSanjukta Chakraborty, Texas A&M Health Science Center, USA
Copyright: © 2012 Lapi, Vagnani, Pignataro, Esposito, Paterni and Colantuoni. This is an open-access article distributed under the terms of the Creative Commons Attribution Non Commercial License, which permits non-commercial use, distribution, and reproduction in other forums, provided the original authors and source are credited.
*Correspondence: Dominga Lapi and Antonio Colantuoni, Department of Neuroscience, “Federico II” University Medical School, Via S. Pansini, 5, 80121 Naples, Italy. e-mail: d.lapi@dfb.unipi.it