- 1 Department of Conservation Ecology and Entomology, Stellenbosch University, Stellenbosch, South Africa
- 2 Department of Bioscience, Aarhus University, Silkeborg, Denmark
Controlled atmosphere treatments using carbon dioxide, oxygen, and/or nitrogen, together with controlled temperature and humidity, form an important method for post-harvest sterilization against insect-infested fruit. However, in insects, the cross tolerance and biochemical interactions between the various stresses of modified gas conditions and low temperature may either elicit or block standard stress responses which can potentiate (or limit) lethal low temperature exposure. Thus, the success of such treatments is sometimes erratic and does not always result in the desired pest mortality. This review focuses on the biochemical modes of action whereby controlled atmospheres affect insects low temperature tolerance, making them more (or occasionally, less) susceptible to cold sterilization. Insights into the integrated biochemical modes of action may be used together with the pests’ low temperature tolerance physiology to determine which treatments may be of value in post-harvest sterilization.
Introduction
Many treatment regimes rely on one of several stresses [e.g., temperature/controlled atmospheres (CAs)] to reduce survival of insects for pest management or post-harvest disinfestation. However, current research is increasingly exploring the use of multiple, combined, or sequential stresses to act synergistically and enhance insect mortality rates, while reducing commodity damage. The phasing out of numerous chemicals previously used to sterilize fruit and other commodities has prompted renewed interest in CA treatments [reviewed by Fields and White (2002)]. Controlled atmosphere treatments usually involve augmenting temperature sterilization with either high CO2 (18–90 kPa) or low O2 (0–11.5 kPa; Hallman and Denlinger, 1998). These gas conditions are frequently used along with controlled humidity, and can be combined to give a high CO2 and low O2 environment. There has also been research into using ozone for pest control in stored produce (Tiwari et al., 2010). Cross tolerance of insects to different environmental stressors can disrupt post-harvest control measures in unexpected ways and the success of CA treatments is species-dependent, erratic and does not always result in additional pest mortality (e.g., Navarro, 1978; see Mitcham et al., 2001; Pryke and Pringle, 2008).
In general, CAs at high temperatures reduce the exposure time of fruit thereby reducing fruit damage. However, some commodities are particularly intolerant to warm temperatures (e.g., grapes and cut flowers). At low temperatures, CAs can improve pest mortality during low temperature sterilization, and sometimes shorten the treatment duration too. Whilst the effects of high temperature treatments together with CAs are relatively well documented for several insect pests (e.g., Neven and Rehfield-Ray, 2006), CA treatments coupled with low temperature exposures are not well understood. Treatments with minimal deleterious effects on fresh commodities are challenging to develop (but see Table 1 for a list of published potentially viable low temperature CA protocols). The inconsistent results may indicate that the pre-treatments either elicit or block standard stress responses which potentiate (or limit) lethal low temperature exposure. In addition, insects may also be exposed to conditions that enhance thermal tolerance during the harvesting process prior to sterilization or during the actual CA process which could ultimately affect treatment efficacy.
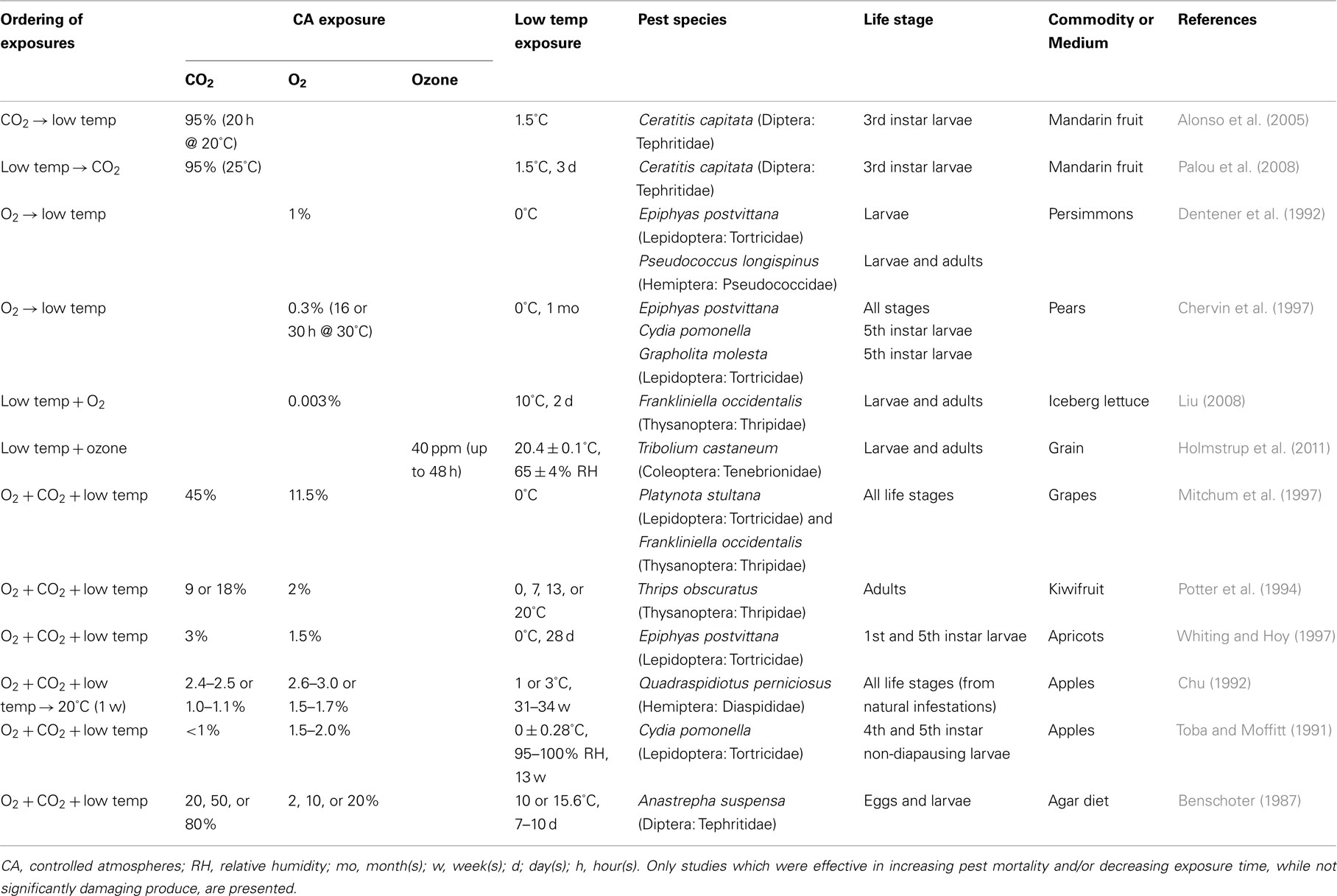
Table 1. Examples of insect pest post-harvest sterilization methods using low temperatures augmented with controlled atmospheres.
This mini-review focuses on the biochemical mechanisms whereby CAs affect insect low temperature tolerance, potentially making them more susceptible (or tolerant) to low temperature sterilization protocols. While previous reviews have highlighted the need for better understanding of the mechanisms (Mitcham et al., 2006; Phillips and Throne, 2010), none have considered in detail the biochemical interactions between the various stresses of modified gas conditions and low temperature (though see Storey and Storey, 2004). Here we provide an overview of the mechanisms involved in low temperature tolerance and CA responses, both from insect physiology and pest control case studies. The aims of this mini-review are therefore (i) to briefly highlight the biochemical links between the seemingly unrelated stressors of gas and temperature, (ii) identify potential commonalities between stress pathways in order to better understand the mechanisms of low temperature mortality and CA treatments, and (iii) identify future directions for insect physiology research which might be of benefit to applied pest management.
Molecular Mechanisms of Low Temperature Tolerance
Insect tolerance to low temperature treatments for post-harvest sterilization depends on basal low temperature tolerance, the ability to withstand or repair the stress associated with long-term low temperature exposure, or the ability to rapidly develop biochemical protection (for introduction to this literature, see recent reviews in, e.g., Clark and Worland, 2008; Doucet et al., 2009; Lee, 2010). This ability to rapidly adapt to the external stressor is a form of phenotypic plasticity, in particular, if it occurs within the organism’s life-time. Both the immediate environment as well as the species’ evolutionary history will determine the extent to which an individuals’ response is plastic (e.g., Chown and Terblanche, 2007; Nyamukondiwa et al., 2011; Overgaard et al., 2011).
In most cases, commodities cannot withstand temperatures below freezing and are held at, or just above, 0°C for the duration of the treatment. While a large proportion of insects will enter chill coma at these temperatures, and some species or life stages could die (e.g., Drosophila melanogaster survives about 8 h at 0°C), those with mechanisms of enhanced low temperature tolerance are able to survive more severe exposures. However, such generalizations are complicated by a range of factors. Firstly, it is important to remember that most of these mechanisms are closely linked to one another, as well as to external factors, e.g., diet (see e.g., Shreve et al., 2007). Thus, in order for biochemical mechanisms to buffer the effects of low temperature exposure in an insect, the basic components enabling these processes need to be present, together with active metabolism. These known mechanisms of low temperature tolerance are understood in some detail and rely on interactions between genes, proteins, cryoprotectants, and the regulation of biological membranes to preserve cell structure and function (see Figure 1 and Table 2 for summary and synthesis).
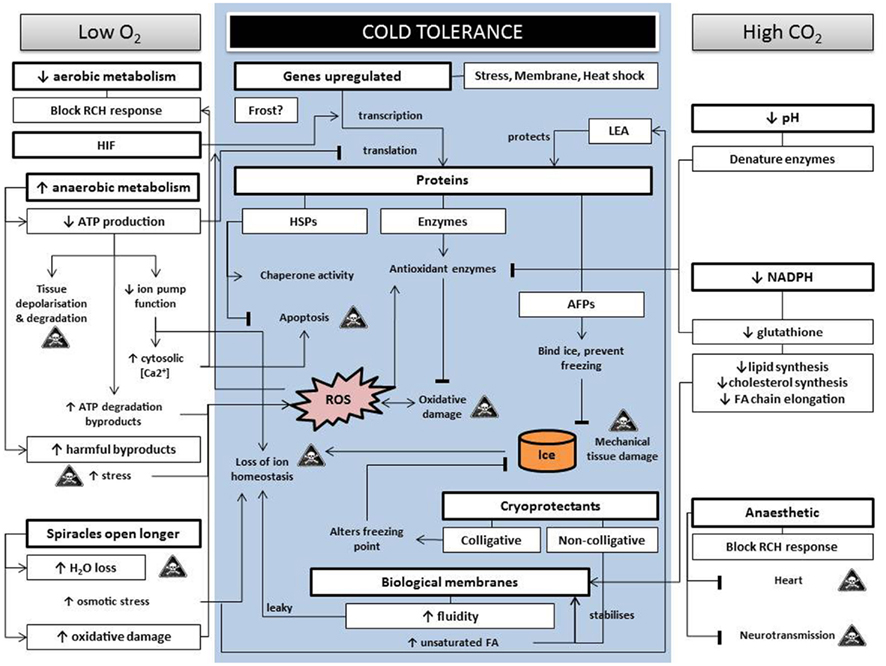
Figure 1. A schematic representation of the cross tolerance between controlled atmospheres (low oxygen and high carbon dioxide) with low temperature stress. The central shaded block represents the mechanisms for survival of low temperatures. Arrows indicate preceding steps in reactions, while solid lines indicate inhibition. The “skull and crossbones” icon represents processes that are likely to lead to mortality. RCH, rapid cold hardening; ATP, adenosine triphosphate; HIF, hypoxia inducible factor; LEA, late embryogenesis abundant proteins; HSPs, heat shock proteins; AFPs, anti-freeze proteins; ROS, reactive oxygen species; FA, fatty acids; NADPH, reduced form of nicotinamide adenine dinucleotide phosphate.
Ultimately, the gene and biochemical responses described (Table 2) are all dependent on the availability of energy provided by metabolism. The “membrane pacemaker” theory of metabolism provides a link between cellular metabolism and lipid membrane bilayers. It suggests that the balance between monounsaturated and long-chain polyunsaturated acyl chains within the bilayer plays a role in establishing the metabolic rate (reviewed in Hulbert, 2003). Aging membranes will be subjected to a change in acyl composition which may in turn influence a species’ lifespan. Interestingly, the carbon units in polyunsaturated acyl chains are very susceptible to oxidative damage, whilst saturated and monounsaturated acyl chains do not possess these carbon atoms (Hulbert, 2003). Molecular changes also occur in the acyl chains’ packing which decreases membrane permeability (Tomczak et al., 2002). Additionally, changes in cholesterol content and temperature can result in changes in membrane fluidity by altering the phase of the lipid bilayer (M’Baye et al., 2008).
Thus, an insect’s low temperature tolerance depends on interactions between the different molecular levels to ensure function and survival. The cellular mechanisms work together to limit oxidative damage and maintain the integrity of membranes and essential proteins during the low temperature exposure, as well as to buffer the potential heat-stress of returning to warmer temperatures thereafter. A breakdown in just one of these systems is potentially sufficient to stress and overwhelm the entire low temperature tolerance response, perhaps directly or indirectly resulting in insect mortality. In addition, selected tissues may be particularly susceptible to low temperature stress, with knock-on effects for the whole organism if the low temperature-sensitive tissue becomes irreparably damaged (e.g., MacMillan and Sinclair, 2011).
Augmentation with Manipulated Atmospheres
Pörtner (2001) proposed a theory for thermal tolerance of animals that centered round O2 limitation as the major factor. The theory of O2 and capacity limitation of thermal tolerance (OCLT) proposes that once aerobic capacity has been exhausted at temperatures close to critical limits, anaerobic mitochondrial metabolism begins. Although this theory was proposed for marine animals, it is plausible that O2 limitation may induce a similar sequence of mechanisms responsible for insect low temperature tolerance. To date, little evidence supporting the OCLT has been found in insects (e.g., Klok et al., 2004; Stevens et al., 2010; but see Verberk and Bilton, 2011). Regardless, OCLT serves as an important theory which requires further testing since it has direct applicability to understanding the mechanisms of thermal death (Stevens et al., 2010; Verberk and Bilton, 2011).
In the next section, we briefly consider the cellular responses induced by low O2 in insects, followed by responses to high CO2 and ozone.
Oxygen
Responses to low O2 can be broadly classified into two classes: the regulating class and the conforming class (Hochachka, 1991; Makarieva et al., 2006). Organisms in the regulating class maintain the energy required for normal processing during the exposure by increasing glycolytic flux and using large amounts of substrates, while those in the conforming class decrease energy use, respiration rate, and substrate usage without engaging in glycolytic pathways. Organisms in the conforming class will usually survive long-term exposures to low O2 better than those in the regulating class. Damage repair processes are ongoing in the regulating class and only occur upon return to normoxia in the conforming class; repair mechanisms are nevertheless essential for survival of hypoxia.
Insects exposed to hypoxia (or anoxia) will decrease aerobic metabolism, which has been shown to block rapid cold hardening (RCH) in the housefly, Musca domestica (Coulson and Bale, 1991). Similarly, Nilson et al (2006) showed that anoxia blocked RCH in D. melanogaster after 1 h of exposure, but had no effect at shorter times. RCH is a form of phenotypic plasticity whereby a non-lethal low temperature “shock” protects against subsequent, previously lethal, low temperature exposure (Lee et al., 1987; Lee and Denlinger, 2010). In contrast, Yocum and Delinger (1994) found that RCH is not induced under anoxic conditions in the flesh fly (Sarcophaga crassipalpis) indicating that these processes required aerobic conditions to occur. It is therefore clear that gas conditions may interact with low temperature stress responses in at least some species.
The decrease in aerobic metabolism caused by decreasing temperature can be accompanied by a switch to anaerobic metabolism, which can be detrimental if experienced for long periods (Figure 1). Increased anaerobic metabolism decreases the amount of adenosine triphosphate (ATP) production which, in turn, can depolarize and degrade tissues, increase ATP degradation and reduce the functioning of ion pumps (e.g., Chiappini et al., 2009). Byproducts of ATP degradation can react with O2 to form reactive oxygen species (ROS) that cause oxidative damage (Weyel and Wegener, 1996), while a malfunctioning of ion pumps will increase cytosolic calcium concentrations which, if left unbuffered, can trigger apoptosis and block RCH (reviewed by Orrenius et al., 2003; Teets et al., 2008). However, during hypoxia, coprophilous beetles are able to extract sufficient O2 from air that contained a very low concentration of O2 (1%) without switching to anaerobic metabolism, which suggests that not all insects are affected in the same way (Holter and Spangenberg, 1997). In addition, during hypoxia insects may keep their spiracles open for longer periods in order to meet their O2 demands (Hetz and Bradley, 2005). Opening of the spiracles can lead to an increased water loss rate, which is believed to be how low O2 concentrations can augment a low temperature sterilization regime (Navarro, 1978). Based on studies of insect gas exchange, keeping spiracles open for long periods may result in oxidative damage (Hetz and Bradley, 2005) – possibly through the formation of ROS acting as a signaling mechanism (Boardman et al., 2011). In addition, hypoxia also stimulates hypoxia inducible factor (HIF), a transcription factor stabilized by the presence of ROS, which is necessary for the upregulation of hypoxia-responsive genes (Huang et al., 1996).
Carbon Dioxide
High CO2 concentrations may decrease pH which can be detrimental to membranes and cellular function. A decrease in pH will also denature enzymes, including antioxidant enzymes needed for low temperature tolerance, especially if there are no additional heat shock proteins (HSPs) to act as chaperones. In addition, high CO2 causes a decrease in NADPH (reduced form of nicotinamide adenine dinucleotide phosphate) enzyme and a subsequent decrease in glutathione production (Friedlander, 1983). NADPH and the antioxidant glutathione are involved in protecting against the toxicity of ROS, while NADPH also contributes to lipid synthesis, cholesterol synthesis, and fatty acid chain elongation.
Secondly, high concentrations of CO2 are commonly used as an anesthetic for insect handling. Identical to low O2, CO2 anesthesia blocked RCH in D. melanogaster after 1 h of exposure, but had no effect at shorter times (Nilson et al., 2006). Badre et al. (2005) investigated the mechanism underlying this response and found that in D. melanogaster larvae, with intact spiracles, high CO2 caused their hearts to stop, and blocked synaptic transmission at the neuromuscular junction by decreasing the number of glutamate receptors. Further investigations showed that these effects were not due to hypoxia, low pH, or action of the central nervous system.
Ozone
Recently, there has been increased research into the use of ozone, a strong oxidizing agent, for pest control in stored products (e.g., Tiwari et al., 2010; Holmstrup et al., 2011). The mechanisms of action of ozone are attributed to an increase in ROS, together with direct deleterious reactions with proteins, DNA, and polyunsaturated fatty acids (Hermes-Lima, 2004).
Implications of Interactions between Temperature and Atmosphere
Figure 1 illustrates some of the potential biochemical mechanisms of cross tolerance of CAs with low temperature tolerance, and shows how the altered gas conditions can potentially interfere with typical low temperature tolerance mechanisms. Simply put, low O2 decreases aerobic metabolism which, apart from directly blocking RCH responses, decreases transcription and ion homeostasis, and increases oxidative damage. Furthermore, water loss rate is increased through the opening of the spiracles, perhaps leading to increased osmotic and ionic stress, and downstream oxidative damage. High CO2 directly affects the heart and nervous system (anesthetic effects), and decreases pH and NADPH which affect the antioxidant response and membrane functioning. Any one (or combination) of these factors may be the key reason as to why survival may be reduced under combination CA low temperature treatments. Yet some observations suggest that survival may improve under these combination treatments. Possible reasons for the improved survival may be cross tolerance between temperature and gases (where the mechanisms of either low temperature or gas tolerance are sufficiently protective against both stresses to ensure survival) or natural tolerance to one or more of the potential stressors.
At high temperatures, heat hardening can also benefit insects by increasing survival at previously lethal high temperatures, as well as upregulating general stress responses. While high temperature responses fall largely outside the scope of this review, the physiological and biochemical changes that insects undergo to survive high temperature shock may provide insights into how they survive low temperature shock. Moreover, cross tolerance to temperature stress has been demonstrated in a variety of species (e.g., Burton et al., 1988; Chen et al., 1991; Sinclair and Chown, 2003). The heat shock transcription factor that regulates HSPs induces stress-associated genes, including some HSPs, after long-term low temperature acclimation, but typically these do not play a role in resistance to low temperature shock, RCH, or high temperature knock-down in D. melanogaster (Nielsen et al., 2005). Therefore, any stress of heat exposure prior to CA low temperature sterilization may inadvertently improve survival of the insect pest through cross tolerance of low and high temperatures, as well as low temperatures and desiccation stress.
Innate Tolerance to Extreme Atmospheres
Controlled atmospheres can also occur naturally in an insects’ immediate environment. For example, insects living at high altitudes will experience hypoxic conditions (Frazier et al., 2006), while those that burrow underground or live in dung pats may experience both hypoxic and hypercapnic (high CO2) conditions (Holter and Spangenberg, 1997). Thus, some pest species may be pre-adapted for survival to CAs, and it may be argued that an understanding of basic ecology and biology may be important for selecting effective treatments and understanding why certain treatments fail to increase mortality. In addition, the adaptations present in these organisms may highlight the mechanisms that are needed to ensure survival under these conditions and, in turn, reveal insights into the cellular or whole organism physiological systems responsible for mortality under altered environmental conditions.
Some insects are naturally more tolerant of changes in gas concentrations during specific life stages. For example, embryos of D. melanogaster are able to arrest the cell cycle at the metaphase or S-phase in response to hypoxia, and will resume normal cell cycling within 20 min of reoxygenation (Douglas et al., 2001). In addition to the cell cycle arrest, D. melanogaster are able to detect the O2 changes through the accumulation of nitric oxide in sensor cells in order to change their behavior, and alter tracheal development, possibly with the aid of HIF (Wingrove and O’Farrell, 1999). Similarly, pupae of Tribolium confusum are able to survive 48 h of anoxia, but die if the anoxia continues beyond this point, possibly due to depletion in carbohydrate stores by anaerobic glycolysis (Kennington and Cannell, 1967).
Tolerance to gases is aided by the insects’ ability to withstand reoxygenation. After anoxia, return to normoxia has been shown to cause damage in both D. melanogaster (Lighton and Schilman, 2007) and other vertebrate species (Hermes-Lima and Zenteno-Savín, 2002). One possible cause of increased hypoxia/anoxia tolerance may be the ability to return to efficient energy metabolism, without generating excess heat, upon reoxygenation (Wegener and Moratzky, 1995). During anoxia, ATP is degraded and the resulting byproducts can react with O2 to become ROS (Figure 1). The ability of insects to recover from anoxia may be further attributed to their ability to limit the degradation of adenine nucleotides during anoxia, and re-synthesize ATP from these nucleotides during recovery (Weyel and Wegener, 1996).
Conclusion and Future Directions
Reactions to similar treatments vary widely between insect species, hence treatment regimes should be investigated in a species-specific manner, based on the known physiology and biochemical responses of each species (Navarro, 1978). Species relatedness may aid generalizing thermal tolerance and its plasticity but even then should be undertaken with caution (e.g., Nyamukondiwa et al., 2011). Established thermal responses or lack thereof should be used as background information to determine which treatments may be worth testing to determine their suitability for post-harvest sterilization or control of grain pests. Furthermore, as emphasized in this mini-review, there is a large degree of overlap between the mechanisms of gas and temperature stress responses. Tolerance to these stressors depends largely on limiting oxidative damage and maintaining the integrity of biological membranes and essential proteins. The success of CA augmentation of low temperature treatments may be attributed to their ability to interfere with basal low temperature tolerance mechanisms, causing sufficient cellular and/or tissue damage to result in insect mortality, or perhaps influencing damage and repair mechanisms during recovery periods from CAs. Future work aimed at expanding the potentiating and blocking types of action in these stress pathways, and the interactions among them, will likely enhance both applied pest management, as well as understanding of whole organism physiology to extreme conditions.
Conflict of Interest Statement
The authors declare that the research was conducted in the absence of any commercial or financial relationships that could be construed as a potential conflict of interest.
Acknowledgments
The authors wish to thank Citrus Research International, Fruitgro Science, NRF-THRIP and Sub-committee B (Stellenbosch University) for financial support and the two referees for constructive comments on an early version of the manuscript. This work was partly supported by the National Research Foundation IFR 2011032600009 to John S. Terblanche. Any opinion, findings, and conclusions or recommendations expressed in this material are those of the authors and therefore the NRF do not accept liability thereto.
References
Alonso, M., del Rio, M. A., and Jacas, J. A. (2005). Carbon dioxide diminishes cold tolerance of third instar larvae of Ceratitis capitata Wiedemann (Diptera: Tephritidae) in “Fortune” mandarins: implications for citrus quarantine treatments. Postharvest Biol. Technol. 36, 103–111.
Badre, N. H., Martin, M. E., and Cooper, R. L. (2005). The physiological and behavioral effects of carbon dioxide on Drosophila melanogaster larvae. Comp. Biochem. Physiol. A Mol. Integr. Physiol. 140, 363–376.
Barnes, P. T., Holland, B., and Courreges, V. (1989). Genotype-by-environment and epistatic interactions in Drosophila melanogaster: the effects of Gpdh allozymes, genetic background and rearing temperature on larval developmental time and viability. Genetics 122, 859–868.
Beere, H. M., Wolf, B. B., Cain, K., Mosser, D. D., Mahboubi, A., Kuwana, T., Tailor, P., Morimoto, R. I., Cohen, G. M., and Green, D. R. (2000). Heat-shock protein 70 inhibits apoptosis by preventing recruitment of procaspase-9 to the Apaf-1 apoptosome. Nat. Cell Biol. 2, 469–475.
Benschoter, C. A. (1987). Effects of modified atmosphere and refrigeration temperatures on survival of eggs and larvae of the Caribbean fruit fly (Diptera: Tephritidae) in laboratory diet. J. Econ. Entomol. 80, 1223–1225.
Boardman, L., Terblanche, J. S., Hetz, S. K., Marais, E., and Chown, S. L. (2011). Reactive oxygen species and discontinuous gas exchange in insects. Proc. Biol. Sci. doi: 10.1098/rspb.2011.1243
Burton, V., Mitchell, H. K., Young, P., and Petersen, N. S. (1988). Heat shock protection against cold stress of Drosophila melanogaster. Mol. Cell. Biol. 8, 3550–3552.
Chen, C. P., Lee, R. E., and Denlinger, D. L. (1991). Cold shock and heat-shock – a comparison of the protection generated by brief pretreatment at less severe temperatures. Physiol. Entomol. 16, 19–26.
Chervin, C., Kulkarni, S., Kreidl, S., Birrell, F., and Glenn, D. (1997). A high temperature/low oxygen pulse improves cold storage disinfestations. Postharvest Biol. Technol. 10, 239–245.
Chiappini, E., Molinari, P., and Cravedi, P. (2009). Mortality of Tribolium confusum J. du Val (Coleoptera: Tenebrionidae) in controlled atmospheres at different oxygen percentages. J. Stored Prod. Res. 45, 10–13.
Chown, S. L., and Terblanche, J. S. (2007). Physiological diversity in insects: ecological and evolutionary contexts. Adv. In Insect Phys. 33, 50–152.
Chu, C. L. (1992). Postharvest control of San Jose scale on apples by controlled atmosphere storage. Postharvest Biol. Technol. 1, 361–369.
Clark, M. S., and Worland, M. R. (2008). How insects survive the cold: molecular mechanisms-a review. J. Comp. Physiol. B Biochem. Syst. Environ. Physiol. 178, 917–933.
Colinet, H., Fai Lee, S., and Hoffmann, A. (2010a). Functional characterization of the Frost gene in Drosophila melanogaster: importance for recovery from chill coma. PLoS ONE 5, e10925. doi: 10.1371/journal.pone.0010925
Colinet, H., Fai Lee, S., and Hoffmann, A. (2010b). Temporal expression of heat shock genes during cold stress and recovery from chill coma in adult Drosophila melanogaster. FEBS J. 277, 174–185.
Coulson, S. J., and Bale, J. S. (1991). Anoxia induces rapid cold hardening in the housefly Musca domestica (Diptera, Muscidae). J. Insect Physiol. 37, 497–501.
Dentener, P. R., Peetz, S. M., and Birtles, D. B. (1992). Modified atmospheres for the postharvest disinfestation of New Zealand persimmons. N. Z. J. Crop Hortic. Sci. 20, 203–208.
Doucet, D., Walker, V. K., and Qin, W. (2009). The bugs that came in from the cold: molecular adaptations to low temperatures in insects. Cell. Mol. Life Sci. 66, 1404–1418.
Douglas, R. M., Xu, T., and Haddad, G. G. (2001). Cell cycle progression and cell division are sensitive to hypoxia in Drosophila melanogaster embryos. Am. J. Physiol. Regul. Integr. Comp. Physiol. 280, R1555–R1563.
Feder, M. E., and Hofmann, G. E. (1999). Heat-shock proteins, molecular chaperones, and the stress response: evolutionary and ecological physiology. Annu. Rev. Physiol. 61, 243–282.
Fields, P. G., and White, N. D. G. (2002). Alternatives to methyl bromide treatments for stored-product and quarantine insects. Annu. Rev. Entomol. 47, 331–359.
Frazier, M. R., Dillon, M. E., and Crane, S. (2006). Altitudinal clines in insect body size: physiological interpretations of mixed patterns. Integr. Comp. Biol. 46, E193.
Friedlander, A. (1983). “Biochemical reflection on a non-chemical control method: the effect of controlled atmosphere on the biochemical processes in stored product insects,” in Proceedings, 3rd International Working Conference on Stored Product Entomology (Manhattan: Kansas State University), 471–486.
Greenberg, A. J., Moran, J. R., Fang, S., and Wu, C. -I. (2006). Adaptive loss of an old duplicated gene during incipient speciation. Mol. Biol. Evol. 23, 401–410.
Hallman, G. J., and Denlinger, D. L. (eds). (1998). Temperature Sensitivity in Insects and Application in Integrated Pest Management. Boulder, CO: Westview Press.
Hand, S. C., Menze, M. A., Toner, M., Boswell, L., and Moore, D. (2011). LEA proteins during water stress: not just for plants anymore. Annu. Rev. Physiol. 73, 115–134.
Hermes-Lima, M. (2004). “Oxygen in biology and biochemistry: role of free radicals,” in Functional Metabolism: Regulation and Adaptation, ed. K. B. Storey (Hoboken: John Wiley and Sons, Inc.), 319–368.
Hermes-Lima, M., Storey, J. M., and Storey, K. B. (2001). “Antioxidant defenses and animal adaptation to oxygen availability during environmental stress,” in Cell and Molecular Response to Stress Protein Adaptations and Signal Transduction, ed. K. B. Storey (Amsterdam: Elsevier), 263–287.
Hermes-Lima, M., and Zenteno-Savín, T. (2002). Animal responses to drastic changes in oxygen availability and physiological oxidative stress. Comp. Biochem. Physiol. C Comp. Pharmacol. Toxicol. 133, 537–556.
Hetz, S. K., and Bradley, T. J. (2005). Insects breathe discontinuously to avoid oxygen toxicity. Nature 433, 516–519.
Hochachka, P. W. (1991). “Metabolic strategies of defense against hypoxia in animals,” in Plant Life Under Oxygen Deprivation, eds M. B. Jackson, D. D. Davies, and H. Lambers (Hague: SPB Academic Publishing), 121–128.
Holmstrup, M., Sørensen, J. G., Heckmann, L.-H., Slotsbo, S., Hansen, P., and Hansen, L. S. (2011). Effects of ozone on gene expression and lipid peroxidation in adults and larvae of the red flour beetle (Tribolium castaneum). J. Stored Prod. Res. 47, 378–384.
Holter, P., and Spangenberg, A. (1997). Oxygen uptake in coprophilous beetles (Aphodius, Geotrupes, Sphaeridium) at low oxygen and high carbon dioxide concentrations. Physiol. Entomol. 22, 339–343.
Huang, L. E., Arany, Z., Livingston, D. M., and Bunn, H. F. (1996). Activation of hypoxia inducible transcription factor depends primarily on redox-sensitive stabilization of 580 its α subunit. J. Biol. Chem. 271, 32253–32259.
Jäättelä, M., Wissing, D., Kokholm, K., Kallunki, T., and Egeblad, M. (1998). Hsp70 exerts its anti-apoptotic function downstream of caspase-3-like proteases. EMBO J. 17, 6124–6134.
Joanisse, D. R., and Storey, K. B. (1996). Oxidative stress and antioxidants in overwintering larvae of cold-hardy goldenrod gall insects. J. Exp. Biol. 199, 1483–1491.
Kennington, G. S., and Cannell, S. (1967). Biochemical correlates of respiratory and developmental changes in anoxic Tribolium confusum pupae. Physiol. Zool. 40, 403–408.
Klok, C. J., Sinclair, B. J., and Chown, S. L. (2004). Upper thermal tolerance and oxygen limitation in terrestrial arthropods. J. Exp. Biol. 207, 2361–2370.
Koštál, V., Zahradnikova, H., Simek, P., and Zeleny, J. (2007). Multiple component system of sugars and polyols in the overwintering spruce bark beetle, Ips typographus. J. Insect Physiol. 53, 580–586.
Lee, R. E. (2010). “A primer on insect cold-tolerance,” in Low temperature Biology of Insects, eds D. L. Denlinger, and R. E. Lee (Cambridge: Cambridge University Press), 3–34.
Lee, R. E., Chen, C. P., and Denlinger, D. L. (1987). A rapid cold-hardening process in insects. Science 238, 1415–1417.
Lee, R. E., Damodaran, K., Yi, S. X., and Lorigan, G. A. (2006). Rapid cold-hardening increases membrane fluidity and cold tolerance of insect cells. Cryobiology 52, 459–463.
Lee, R. E., and Denlinger, D. L. (2010). “Rapid cold-hardening: ecological significance and underpinning mechanisms,” in Low temperature Biology of Insects, eds D. L. Denlinger, and R. E. Lee (Cambridge: Cambridge University Press), 35–57.
Leemans, R., Egger, B., Loop, T., Kammermeier, L., He, H., Hartmann, B., Certa, U., Hirth, F., and Reichert, H. (2000). Quantitive transcript imaging in normal and heat-shocked Drosophila embryos by using high-density oligonucleotide arrays. Proc. Natl. Acad. Sci. U.S.A. 97, 12138–12143.
Lighton, J. R. B., and Schilman, P. E. (2007). Oxygen reperfusion damage in an insect. PLoS ONE 12, e1267. doi: 10.1371/journal.pone.0001267
Liu, Y. B. (2008). Ultralow oxygen treatment for postharvest control of western flower thrips, Frankliniella occidentalis (Thysanoptera: Thripidae), on iceberg lettuce I. Effects of temperature, time, and oxygen level on insect mortality and lettuce quality. Postharvest Biol. Technol. 49, 129–134.
MacMillan, H., and Sinclair, B. J. (2011). The role of the gut in insect chilling-injury: cold-induced disruption of osmoregulation in the fall field cricket, Gryllus pennsylvanicus. J. Exp. Biol. 214, 726–734.
Makarieva, A. M., Gorshkov, V. G., Li, B.-L., and Chown, S. L. (2006). Size- and temperature-independence of minimum life-supporting metabolic rates. Funct. Ecol. 20, 83–96.
M’Baye, G., Mely, Y., Duportail, G., and Klymchenko, A. S. (2008). Liquid ordered and gel phases of lipid bilayers: fluorescent probes reveal close fluidity but different hydration. Biophys. J. 95, 1217–1225.
Mitcham, E., Martin, T., and Zhou, S. (2006). The mode of action of insecticidal controlled atmospheres. Bull. Entomol. Res. 96, 213–222.
Mitcham, E. J., Zhou, S., and Kader, A. A. (2001). Potential of CA for Postharvest Insect Control in Fresh Horticultural Perishables: An Update of Summary Tables Compiled by Ke and Kader 1992. Davis, CA: Department of Pomology, University of California.
Mitchum, E. J., Zhou, S., and Bikoba, V. (1997). Controlled atmospheres for quarantine control of three pests of table grapes. J. Econ. Entomol. 90, 1360–1370.
Morgan, T. J., and Mackay, T. F. C. (2006). Quantitative trait loci for thermotolerance phenotypes in Drosophila melanogaster. Heredity 96, 232–242.
Murray, P., Hayward, S. A. L., Govan, G. G., Gracey, A. Y., and Cossins, A. R. (2007). An explicit test of the phospholipid saturation hypothesis of acquired cold tolerance in Caenorhabditis elegans. Proc. Natl. Acad. Sci. U.S.A. 104, 5489–5494.
Navarro, S. (1978). The effects of low oxygen tensions on three stored-product insect pests. Phytoparasitica 6, 51–58.
Neven, L. G., and Rehfield-Ray, L. (2006). Confirmation and efficacy tests against codling moth and oriental fruit moth in apples using combined heat and controlled atmosphere treatments. J. Econ. Entomol. 99, 1620–1627.
Nielsen, M. M., Overgaard, J., Sorensen, J. G., Holmstrup, M., Justesen, J., and Loeschcke, V. (2005). Role of HSF activation for resistance to heat, cold and high-temperature knock-down. J. Insect Physiol. 51, 1320–1329.
Nilson, T. L., Sinclair, B. J., and Roberts, S. P. (2006). The effects of carbon dioxide anesthesia and anoxia on rapid cold-hardening and chill coma recovery in Drosophila melanogaster. J. Insect Physiol. 52, 1027–1033.
Nyamukondiwa, C., Terblanche, J. S., Marshall, K. E., and Sinclair, B. J. (2011). Basal cold but not heat tolerance constrains plasticity among Drosophila species (Diptera: Drosophilidae). J. Evol. Biol. 24, 1927–1938.
Orrenius, S., Zhivotovsky, B., and Nicotera, P. (2003). Regulation of cell death: the calcium-apoptosis link. Nat. Rev. Mol. Cell Biol. 4, 552–565.
Overgaard, J., Kristensen, T. N., Mitchell, K. A., and Hoffmann, A. A. (2011). Thermal tolerance in widespread and tropical Drosophila species: does phenotypic plasticity increase with latitude? Am. Nat. 178(Suppl. 1), S80–S96.
Overgaard, J., Malmendal, A., Sørensen, J. G., Bundy, J. G., Loeschcke, V., Nielsen, N. C., and Holmstrup, M. (2007). Metabolomic profiling of rapid cold hardening and cold shock in Drosophila melanogaster. J. Insect Physiol. 53, 1218–1232.
Overgaard, J., Sørensen, J. G., Petersen, S. O., Loeschcke, V., and Holmstrup, M. (2005). Changes in membrane lipid composition following rapid cold hardening in Drosophila melanogaster. J. Insect Physiol. 51, 1173–1182.
Palou, L., Jacas, J. A., Marcilla, A., Alonso, M., and del Rio, M. A. (2008). Physico-chemical and sensory quality of “Clemenules” mandarins and survival of the Mediterranean fruit fly as affected by complementary cold and carbon dioxide. Postharvest Biol. Technol. 48, 443–450.
Phillips, T. W., and Throne, J. E. (2010). Biorational approaches to managing stored-product insects. Annu. Rev. Entomol. 55, 375–397.
Pörtner, H. O. (2001). Climate change and temperature-dependent biogeography: oxygen limitation of thermal tolerance in animals. Naturwissenschaften 88, 137–146.
Potter, M. A., Carpenter, A., Stocker, A., and Wright, S. (1994). Controlled atmospheres for postharvest disinfestation of Thrips obscuratus (Thysanoptera: Thripidae). J. Econ. Entomol. 87, 1251–1255.
Pryke, J. S., and Pringle, K. L. (2008). Postharvest disinfestations treatments for deciduous and citrus fruits of the Western Cape, South Africa: a database analysis. S. Afr. J. Sci. 104, 85–89.
Qin, W., Neal, S. J., Robertson, R. M., Westwood, J. T., and Walker, V. K. (2005). Cold hardening and transcriptional change in Drosophila melanogaster. Insect Mol. Biol. 14, 607–613.
Shreve, S. M., Yi, S. X., and Lee, R. E. (2007). Increased dietary cholesterol enhances cold tolerance in Drosophila melanogaster. Cryo Letters 28, 33–37.
Sinclair, B. J., and Chown, S. L. (2003). Rapid responses to high temperature and desiccation but not to low temperature in the freeze tolerant sub-Antarctic caterpillar Pringleophaga marioni (Lepidoptera, Tineidae). J. Insect Physiol. 49, 45–52.
Sinclair, B. J., Gibbs, A. G., and Roberts, S. P. (2007). Gene transcription during exposure to, and recovery from, cold and desiccation stress in Drosophila melanogaster. Insect Mol. Biol. 16, 435–443.
Sørensen, J. G. (2010). Application of heat shock protein expression for detecting natural adaptation and exposure to stress in natural populations. Curr. Zool. 56, 703–713.
Sørensen, J. G., Kristensen, T. N., and Loeschcke, V. (2003). The evolutionary and ecological role of heat shock proteins. Ecol. Lett. 6, 1025–1037.
Stevens, M. M., Jackson, S., Bester, S. A., Terblanche, J. S., and Chown, S. L. (2010). Oxygen limitation and thermal tolerance in two terrestrial arthropod species. J. Exp. Biol. 213, 2209–2218.
Storey, K. B., and Storey, J. M. (2004). “Cold hardiness and freeze tolerance,” in Functional Metabolism: Regulation and Adaptation, ed. K. B. Storey (New Jersey: Wiley-Liss, Inc.), 473–503.
Teets, N. M., Elnitsky, M. A., Benoit, J. B., Lopez-Martinez, G., Denlinger, D. L., and Lee, R. E. (2008). Rapid cold-hardening in larvae of the Antarctic midge Belgica antarctica: cellular cold-sensing and a role for calcium. Am. J. Physiol. Regul. Integr. Comp. Physiol. 294, R1938–R1946.
Tiwari, B. K., Brennan, C. S., Curran, T., Gallagher, E., Cullen, P. J., and Donnell, C. P. (2010). Application of ozone in grain processing. J. Cereal Sci. 51, 248e255.
Toba, H. H., and Moffitt, H. R. (1991). Controlled atmosphere cold storage as a quarantine treatment for nondiapausing codling moth (Lepidoptera: Tortricidae) larvae in apples. J. Econ. Entomol. 84, 1316–1319.
Tomczak, M. M., Hincha, D. K., Estrada, S. D., Wolkers, W. F., Crowe, L. M., Feeney, R. E., Tablin, F., and Crowe, J. H. (2002). A mechanism for stabilization of membranes at low temperatures by an antifreeze protein. Biophys. J. 82, 874–881.
Tunnacliffe, A., and Wise, M. J. (2007). The continuing conundrum of the LEA proteins. Natuurwissenschaften 94, 791–812.
Verberk, W. C. E. P., and Bilton, D. T. (2011). Can oxygen set thermal limits in an insect and drive gigantism? PLoS One 6, e22610. doi: 10.1371/journal.pone.0022610
Wegener, G., and Moratzky, T. (1995). Hypoxia and anoxia in insects: microcalorimetric studies on two species (Locusta migratoria and Manduca sexta) showing different degrees of anoxia tolerance. Thermochim. Acta 251, 209–218.
Weyel, W., and Wegener, G. (1996). Adenine nucleotide metabolism during anoxia and postanoxic recovery in insects. Experientia 52, 474–480.
Whiting, D. C., and Hoy, L. E. (1997). “Mortality response of light brown apple moth to a controlled atmosphere cold storage treatment for apricots,” in Proceedings of 50th New Zealand Plant Protection Conference, Lincoln, 431–435.
Wingrove, J. A., and O’Farrell, P. H. (1999). Nitric oxide contributes to behavioural, cellular, and developmental responses to low oxygen in Drosophila. Cell 98, 105–114.
Keywords: thermal biology, stored product, pest management, biological control
Citation: Boardman L, Sørensen JG, Johnson SA and Terblanche JS (2011) Interactions between controlled atmospheres and low temperature tolerance: a review of biochemical mechanisms. Front. Physio. 2:92. doi: 10.3389/fphys.2011.00092
Received: 09 September 2011; Paper pending published: 03 October 2011;
Accepted: 15 November 2011; Published online: 02 December 2011.
Edited by:
Sylvia Anton, Institut National de la Recherche Agronomique, FranceReviewed by:
Nigel Andrew, University of New England, AustraliaKlaus Fischer, Greifswald University, Germany
Copyright: © 2011 Boardman, Sørensen, Johnson and Terblanche. This is an open-access article subject to a non-exclusive license between the authors and Frontiers Media SA, which permits use, distribution and reproduction in other forums, provided the original authors and source are credited and other Frontiers conditions are complied with.
*Correspondence: John S. Terblanche, Department of Conservation Ecology and Entomology, Stellenbosch University, Private Bag X1, Matieland 7602, South Africa. e-mail:anN0QHN1bi5hYy56YQ==