- 1College of Electronic and Information Engineering, Jinling Institute of Technology, Nanjing, China
- 2Collaborative Innovation Center of Advanced Microstructures, National Laboratory of Solid-State Microstructures, Nanjing University, Nanjing, China
In our previous work, we deeply researched the absolute photoluminescence (PL) quantum yields of luminescent modulating a-SiNxOy films with various N/Si atom ratios under different measurement temperatures. In this work, we further systematically studied the temperature dependent kinetic processes of radiative and non-radiative recombinations in a-SiNxOy systems in the visible light range. First, we investigated the structure of a-SiNxOy films and obtained the concentrations of both trivalent Si and N-Si-O defects related dangling bonds through XPS, FTIR and EPR measurements. Then we further tested the transient fluorescence attenuation of a-SiNxOy films detected at different emission wavelengths. We found that the PL lifetimes of a-SiNxOy films vary with the change of N-Si-O defect state concentrations, which is different from the typical PL decay characteristics of band tail related a-SiNx films previously reported. By combining the resulting PL IQE values with the ns-PL lifetimes, we further intensively redetermined the radiative and non-radiative recombination lifetimes of a-SiNxOy systems. The related radiative recombination rates were obtained (kr∼108 s−1), which can be compared to the results in the direct band gap.
1 Introduction
To realize Si-based monolithic photoelectric integration, the most critical task of various components in the related integration manufacturing processes is to realize Si-based light sources with highly efficient luminescence. However, due to the indirect band gap of Si, the related luminescent efficiencies are very low. Therefore, based on improving the absolute photoluminescence quantum yields (PL AQYs) and PL internal quantum efficiencies (PL IQE), the study of the PL properties and the related dynamics processes in Si-based high-efficient luminescent materials has become one of the research hotspots for more than 2 decades [1, 2].
Most of the previous researches on the luminescence mechanisms and the related PL decay processes has focused on Si nanostructured materials, such as porous silicon (PS Si) [3, 4], colloidally passivated Si quantum dots [5–9], and nc-Si embedded Si-based films [10–15]. Only a few reports were talked about Si-based compounds, such as amorphous silicon carbide (a-SiCx) [16, 17], amorphous silicon nitride (a-SiNx) [18, 19], and amorphous silicon nitride oxide (a-SiOxNy) [20–25]. And they found that the PL lifetimes of a-SiNx and a-SiOxNy films are generally in the nanosecond range [15–18, 20]. Yang’s group studied the luminescence characteristics of a-SiNx films with tail states under different components and found that PL lifetime is related to luminescence peak position (EPL) [18]. Kato et al. studied in detail that the PL dynamics of a-SiNx and a-SiOxNy with tailed luminescence and found that the fluorescence decay time scale is in the range of 10−8 to 10−4 s. The EPL of transient fluorescence spectra changes over time in the nanosecond (∼100 ns) to microsecond time scales [20].
In the previous work, we deeply researched the absolute PL quantum yield of light-emitting modulating a-SiOxNy films in the visible range under different test temperatures, and briefly analyzed their luminescence origins [23]. In this paper, we combined temperature dependent PL (TD PL) and time-resolved PL (TR PL) spectroscopy to further systematically study the kinetic processes of radiation recombination and non-radiative recombination processes of a-SiNxOy films with various N/Si atom ratios under different test temperatures. First, we investigated the structure of the a-SiNxOy films through XPS and FTIR measurements, and obtained the concentrations of both silicon and N-Si-O defects related dangling bonds by EPR measurements. Then, we studied the transient fluorescence properties at different detection wavelengths, and found that the luminescence lifetimes of samples changed with the variation tendency of the concentrations of N-Si-O defect states, which was different from the typical band tail luminescence kinetic characteristics reported in the past. Furthermore, we tested the PL lifetimes of a-SiOxNy films at different temperatures, and analyzed the related radiative and non-radiative recombination processes. Based on the measured PL lifetimes of the a-SiOxNy films at different temperature range, combined with the PL IQE values obtained earlier, we calculated the radiative and non-radiative recombination lifetimes of a-SiOxNy films at different temperatures. At last, we deeply analyzed the change law between the radiative recombination processes of a-SiOxNy films and the concentration of N-Si-O defect states. We found that the radiation recombination rates of the films are almost unchanged over the whole temperature range. The obtained radiative recombination rates kr∼108 s−1 can be compared with the results in the direct band gap (such as CdSe nanocrystals).
2 Materials and methods
2.1 Material fabrication
The a-SiNxOy films (∼500 nm) were deposited on polished Si substrates in a plasma-enhanced chemical vapor deposition (PECVD, OXFORD Plasmalab 80PLus, Oxford, UK) system with a silane, ammonia, and nitrogen gas mixture The gas flow ratios R (R = NH3/SiH4) and the related fabrication parameters were well controlled through the whole fabrication processes, both of which were described in our previous work last year in detail [23]. After fabrication, the samples were subsequently oxidized in situ, and then post-treated by combining thermal annealing with pulsed laser annealing.
2.2 Characterization of A-SiNxOy thin films
The structure of the a-SiNxOy films was investigated through XPS and FTIR measurements. To intensively investigate the atom scale structure defects of a-SiNxOy thin films, we measured the EPR spectra under different R conditions at room temperature with a Bruker EMXplus in the X-band (microwave frequency f∼9.85 GHz, microwave power 20 mW). The temperature dependent time resolved PL (TD-TRPL) properties of a-SiNxOy films with different R were measured with a Fluorolo-3 system (Jobin Yvon) and a HP4284 LCR meter in a computer-controlled Delta 9,023 oven, using a FLS980 (Edinburgh Instrument) equipped with an EPL375 pulse diode laser (pulse width ∼53 ps, repetition rate = 20 MHz, λexc = 375 nm, pumping fluence WPF = 5 mJ/cm2) as light sources. A time-correlated single photo counting (TCSPC) system (time resolution ∼100 ps) were used to record the characteristics of TD-TRPL decay properties. Here we consider that the initial decay of the PL intensity coincides with the excitation signal which is origin from the instrumental response (the instrumental response is about 100 ps). Thus, to deduct the impact of the initial excitation signal, we carefully deconvolved the measured ns-PL decay curves and modified the
2.3 Time-correlated single photo counting (TCSPC) measurement methods
The principle of TCSPC is shown in Figure 1, where a sample is first excited with a narrow laser pulse, and the sample emits fluorescent photons after stimulus. It is assumed that the excitation laser pulse is weak enough so that the sample produces only a single fluorescent photon after each pulse. The time it takes for the first fluorescent photon emitted by the sample to reach the optical receiver (which can also be seen as the time t when a single photon appears) is then measured. This time is proportionally converted into a corresponding voltage pulse by TAC, which is then fed into a multichannel analyzer via A/D conversion. Multiple counts are then performed, and in the multi-channel analyzer, these output pulses are sequentially fed into each channel for cumulative storage. Since the probability of a photon being detected in a certain time interval is proportional to the intensity of the fluorescence emission, repeated measurements can obtain a P(t) histogram of the probability distribution of fluorescent photons that is essentially the same as the original waveform. The histogram measured in this case is equivalent to the I(t) curve of the decay of fluorescence intensity over time after excitation has stopped. This is as if a beam of light (many photons) passing through a small hole creates the same diffraction pattern as a single photon passing through a small hole over a long period of time.
2.4 The PL lifetimes fitting methods of a-SiNxOy films
In general, the PL of solid films is a non-equilibrium radiation process, and the luminescence can continue for a period (>10−11 s) after the excitation stops. When the excitation stops, the PL intensity I(t) decays exponentially over time. The time required when I(t) drops to 1/e of the maximum intensity at excitation is called PL lifetimes (τ) of the excited state, which indicates the average time that a particle exists in the excited state.
The stretched exponentials are used widely and accepted as good markers of light recombination in disordered systems [16, 18, 20]. For comparison, we firstly checked the recombination processes in the controlled a-SiNx films by using stretched exponential model and obtained nice fitting results. However, in the fitting processes of a-SiNxOy systems, we found that if we use stretched exponential model, the error is large and the fitting results are unstable. For band tail related a-SiNx films, the excited carriers relax to the deeper tail states then through the thermalization and radiative recombination to give luminescence. With the increasing excitation photon energy (Eexc), the excited carriers occupied the higher states in the band tail [18]. In our a-SiNxOy systems, we have confirmed that the light emission is mainly originated from the N-Si-O-related defect centers in our previous work [21, 23]. The defect luminescence model has been confirmed by the Stokes Shift between Urbach edge (EU Edge) and PL energy (EPL)
3 Results and discussion
3.1 Chemical composition and bonding configurations
The presence of Si, N, and O is measured from the binding energies of the Si 2p, N 1s, and O 1 s peaks in the XPS spectrum [23]. After the top layer (∼60 nm) was removed by Ar ion beam, the O concentration changed slightly, and the average oxygen content in a-SiNxOy films for different R was about 3.5%. As the sputtering time increases, the O concentration tends to stabilize, which is direct evidence of the incorporation of O into a-SiNx. In order to further verify the N-Si-O bonding configuration, we measured the Fourier infrared absorption spectra (FTIR) of a-SiNxOy films and the corresponding a-SiNx films.
As shown in Figure 2, the vibration peaks of the Si-Si disordered structure (460 cm−1), the Si-N stretching mode (840 cm−1), the N-H rocking mode (1,170 cm−1), the Si–H stretching mode (2,150 cm−1), and the N–H stretching mode (3,350 cm−1) are clearly visible in both of a-SiNxOy films and the controlled a-SiNx films. However, the Si-O stretch mode (1,070 cm−1) was not seen in all FTIR spectra, suggesting that the oxygen atoms were incorporated only as trace impurities. It is worth noting that, from the vibration peaks of the Si-N stretching mode, we found that the Si-N stretching peaks of a-SiNxOy films are slightly shifted to the direction of higher wavenumbers than those of a-SiNx, and there is already an obvious shoulder peak (such as R = 1). This shoulder is clearly derived from the N-Si-O bonding configuration formed in a-SiNx by the incorporation of O.
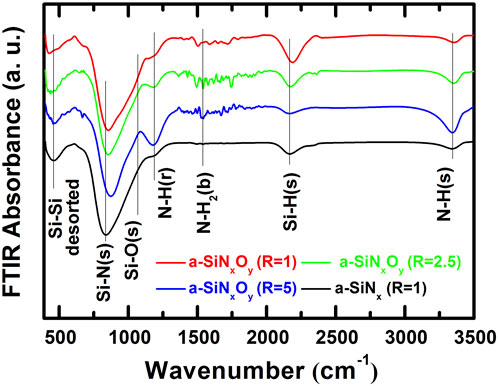
Figure 2. FTIR spectra of a-SiNxOy films with different N/Si ratios and the controlled a-SiNx films.
3.2 N-Si-O related Nx dangling bond defect states
Figure 3 shows the measurements of the EPR curves of a-SiNxOy films with different R. As can be seen from Figure 3, there is a strong resonance absorption peak near the x-axis with magnetic induction intensity of 3,515 G, indicating the presence of unpaired dangling bonds in a-SiNxOy films. Since the structure of a-SiNxOy films is regarded as an intermediate state with both a-SiNx and a-SiOx, it has been found that the suspensory bond defect states in the bandgap of a-SiNx and a-SiOx films may coexist in a-SiNxOy films. We calculated the value range of the zero-crossing g-factor under different R of a-SiNxOy films is 2.0025–2.0040, and the peak line width is 8.5–12.0 Gauss.
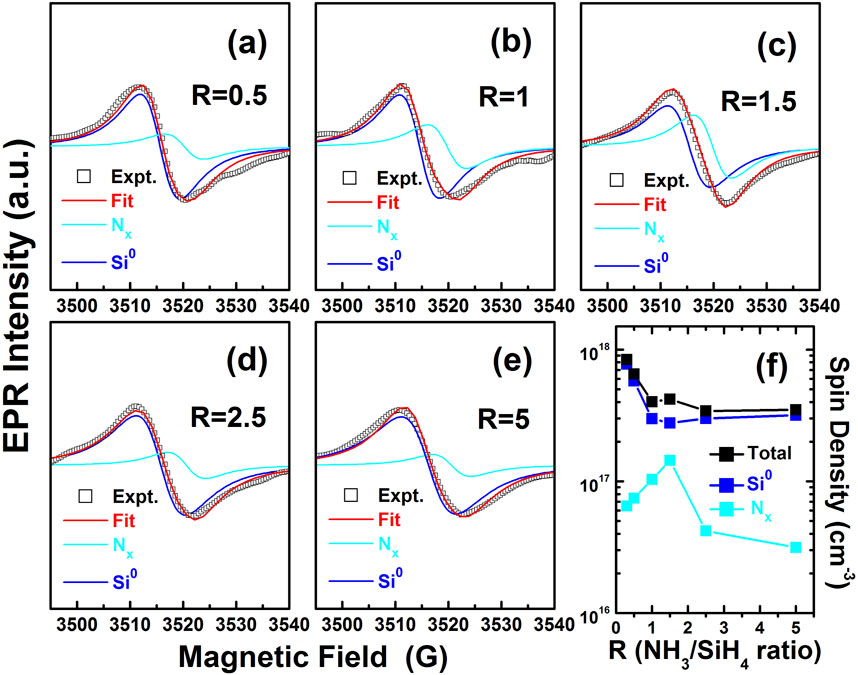
Figure 3. The measured EPR and the related deconvolved signals of the a-SiNxOy films with (A) R = 0.5; (B) R = 1; (C) R = 1.5; (D) R = 2.5; (E) R = 5. (F) The spin densities of total defects, Si DBs, and Nx defects vs R.
According to previous analysis [21], we selected the trivalent Si DBs and the N-Si-O configurations related Nx defect states, and performed Lorentz function fitting on the experimental EPR curves. The fitting results show that the trivalent Si DBs and Nx defect states coexist in the band gap of the a-SiNxOy film, and the relative dangling bond concentrations of the two defect centers are also obtained by fitting. Combined with the PL spectra of a-SiNxOy films of different R [23], we found that the PL integrated intensities (IPL) of a-SiNxOy films were proportional to the concentrations of N-Si-O related Nx dangling bond defect states. At the same time, we also found that the relative concentration of Si DBs in a-SiNxOy films was much higher than that in the Nx defect states. However, due to the low PL efficiency of Si DBs defects in a-SiNxOy thin films [20], they can even be regarded as non-radiative recombination centers.
3.3 Fluorescence properties of a-SiNxOy films
We measured the PL spectra of a-SiNxOy films with different R ratios at 8 K under He-Cd continuous laser excitation at a wavelength of 325 nm. As shown in Figure 4, by adjusting the flow ratio R, we achieved a fluorescence emission of a-SiNxOy films with modulated luminescence wavelengths (λPL) in the visible range. It is worth noting that as R increases from 0.3 to 5, the PL peak blue shifts from 590 nm to 425 nm as R gradually increases; the corresponding PL integrated intensity (IPL) first increases and reaches saturation (R = 1.5), and then gradually decreases.
3.4 TR PL decay properties of a-SiNxOy thin films
To further investigate the relationship between the PL properties of a-SiNxOy films and the defect states of N-Si-O-related Nx dangling bonds, we systematically measured the ns-transient fluorescence PL characteristics of a-SiNxOy films (R = 1) at different detection wavelengths. As a comparison reference, we also measured the related properties of a-SiNx films with the same flow ratio.
Figures 5A, B show the ns-TRPL decay spectra and the relative fitting decay curves for a-SiNx and a-SiNxOy thin films at R = 1, respectively. According to the formula
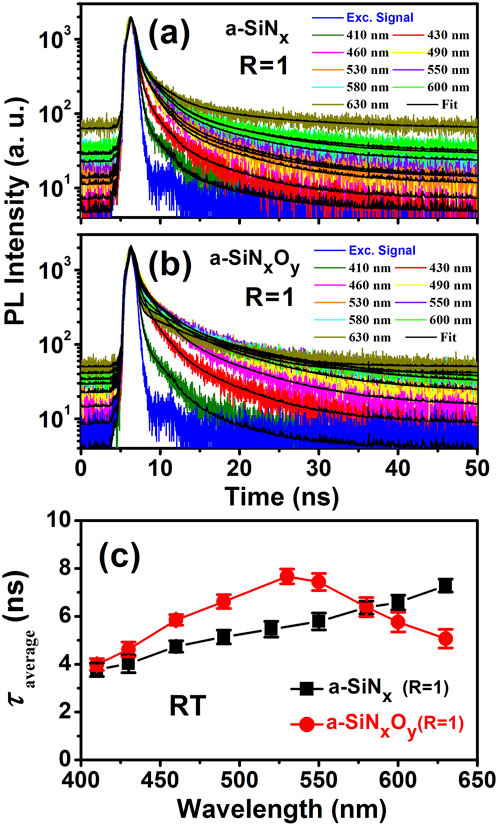
Figure 5. The excitation signal (blue line), the measured ns-TR PL decay spectra and the relative fitted decay curves (black line) of both (A) a-SiNx films (R = 1) and (B) the controlled a-SiNxOy films detected at different emission wavelengths
From Figure 5C, we can see that for a-SiNx films, the PL lifetimes of the samples continue to increase as the detection wavelength increases. This is a typical radiative recombination dynamics process of the band tail carrier transition, which is consistent with the previously reported results [18]. When the excitation photon energy (
However, for a-SiNxOy films, with the increasing detection wavelength, the fluorescence lifetime of the sample first increases to saturation, and then gradually decreases, and its change trend is obviously different from that of a-SiNx films, but consistent with the change trend of defect density distribution, and the corresponding fluorescence lifetime value is proportional to the concentration of Nx defect states. Therefore, we believe that the Nx luminescence defect states related to the N-Si-O configuration dominate the radiation recombination process of a-SiNxOy films. The ns-TRPL properties of the a-SiNxOy films mentioned above verify that our high-efficiency light emission originates from the N-Si-O-related defect luminescence centers of the ns-order radiative recombination processes.
3.5 Temperature dependent ns-PL lifetimes of a-SiNxOy films
Next, to gain a deeper understanding of the radiative and non-radiative recombination mechanisms of high-efficient light emission from N-Si-O-related defect luminescence centers, we studied the PL kinetics of a-SiNxOy films at different test temperatures. Figure 6 shows the PL decay curve for the ns time range of a-SiNxOy thin films (R = 1) at a measured temperature range of 8 K–300 K. As shown in Figure 6A, the PL decay curve profiles remain unchanged at the test temperature range below 100 K, indicating that radiative recombination dominates the entire recombination process. When the test temperature is increased from 120 K to 300 K, it can be seen from the PL decay curve that the PL lifetimes of a-SiNxOy films become shorter, and the non-radiative recombination effects become more and more obvious, as shown in Figure 6B.
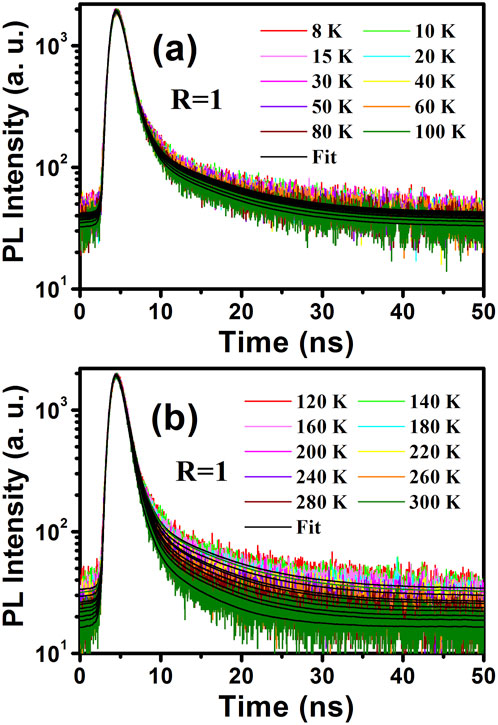
Figure 6. The measured ns-TRPL decay spectra and the relative fitted decay curves (black line) for a-SiNxOy samples with R = 1 under measurement temperature range of (A) from 8 K to 100 K, (B) from 120 K to 300 K, respectively.
At the same time, from the PL decay curve of a-SiNxOy films, we can further see two different ns fluorescence radiation recombination processes, which we call the fast ns-PL lifetimes and the slow ns-PL lifetimes, respectively. The PL decay curves of a-SiNxOy films were well fitted with a double exponential function, and the fitting results for all PL lifetimes are shown in Figure 7. The obtained ns-PL lifetimes τaverage of the samples were reduced from 11.9 ns (less than 100 K) to 7.8 ns As shown in Figure 7, τaverage tends to be stable (about 11.9 ns) and does not change with temperature in the low temperature range (less than 100 K), indicating that the PL lifetimes are mainly due to the radiation recombination processes of the carriers. However, the non-radiative recombination increases with increasing temperature, resulting in a continuous decrease in
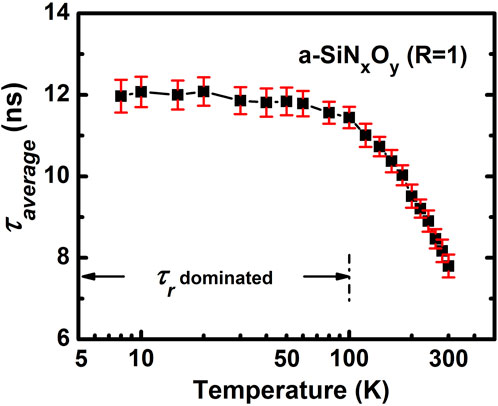
Figure 7. The obtained ns-PL lifetimes
3.6 Radiative and non-radiative recombination lifetimes of a-SiNxOy thin films
As Section 3.5 mentioned above, we use the ns-PL lifetimes
Here
Based on our previous work [23], we obtained the PL IQE values from TD PL spectra and the directly measured PL QYs. Thus, by combining the resulting PL IQE values with the ns-PL lifetimes, we can further determine the radiative recombination lifetimes and non-radiative recombination lifetimes of the sample according to Equation 2. Figure 8 shows the radiative recombination lifetimes and non-radiative recombination lifetimes of a-SiNxOy films with different R at different detection wavelengths. The detailed of the calculated
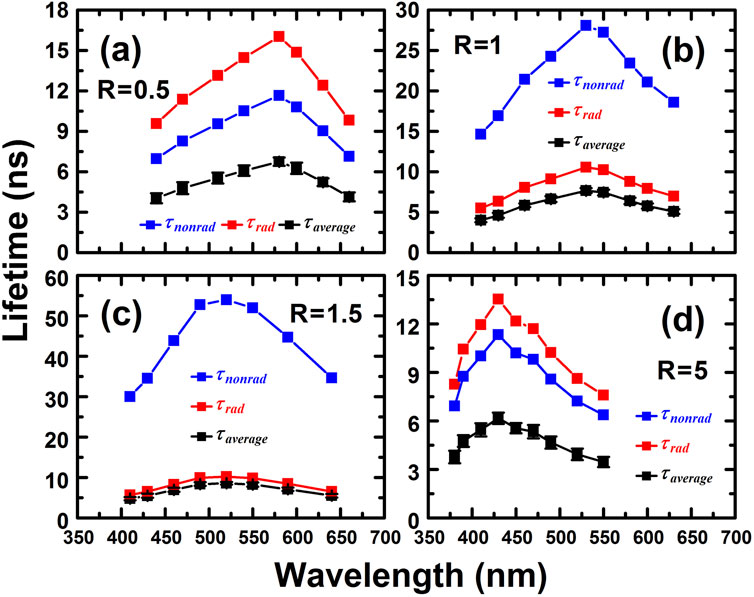
Figure 8. The ns-PL lifetimes
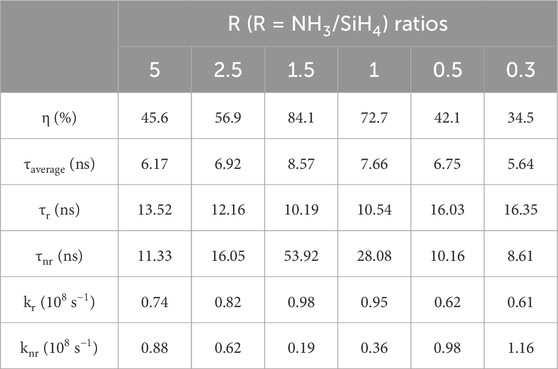
Table 1. Summary of the calculated
Since the fluorescence lifetimes at low temperature are hardly affected by non-radiative recombination, we also can use that as the radiative recombination lifetimes (
4 Conclusion
In summary, we systematically studied the temperature dependent kinetic processes of radiative and non-radiative recombination in a-SiNxOy systems. We found that the PL lifetimes of a-SiNxOy films vary with the change of N-Si-O defect states concentrations, which is different from the typical luminescent kinetic characteristics of band tail related a-SiNx films. By combining PL IQE values with the ns-PL lifetimes, the radiative and non-radiative recombination lifetimes of our a-SiNxOy systems ranged from low temperatures (∼8 K) to room temperature can be determined. The radiative recombination rates were also obtained (kr∼108 s−1), which can be compared to the results in the direct band gap.
Data availability statement
The original contributions presented in the study are included in the article/supplementary material, further inquiries can be directed to the corresponding author.
Author contributions
PZ: Writing–original draft. XL: Methodology, Writing–review and editing. LZ: Methodology, Writing–review and editing. DW: Methodology, Writing–review and editing. KW: Writing–review and editing, Software. SW: Writing–review and editing.
Funding
The author(s) declare that financial support was received for the research, authorship, and/or publication of this article. This research was funded by Jiangsu Province Industry-University-Research Cooperation Project of China (No.BY20230136), and the Open Project Foundation of the National Laboratory of Solid-State Microstructures (No. M37080).
Conflict of interest
The authors declare that the research was conducted in the absence of any commercial or financial relationships that could be construed as a potential conflict of interest.
Publisher’s note
All claims expressed in this article are solely those of the authors and do not necessarily represent those of their affiliated organizations, or those of the publisher, the editors and the reviewers. Any product that may be evaluated in this article, or claim that may be made by its manufacturer, is not guaranteed or endorsed by the publisher.
References
1. Pavesi L, Negro LD, Mazzoleni C, Franzo G, Priolo F. Optical gain in silicon nanocrystals. Nature (2000) 408(23):440–4. doi:10.1038/35044012
2. Fadaly EM, Dijkstra A, Suckert JR, Ziss DM, Tilburg AJ, Mao CY, et al. Direct-bandgap emission from hexagonal Ge and SiGe alloys. Nature (2020) 580:205–9. doi:10.1038/s41586-020-2150-y
3. Joo J, Defforge T, Loni A, Kim D, Li ZY, Sailor MJ, et al. Enhanced quantum yield of photoluminescent porous silicon prepared by supercritical drying. Appl Phys Lett (2022) 108:153111. doi:10.1063/1.4947084
4. Basak FK, Kayahan E. White, blue and cyan luminescence from thermally oxidized porous silicon coated by green synthesized carbon nanostructures. Opt Mater (2022) 124:111990. doi:10.1016/j.optmat.2022.111990
5. Reyes GB, Dasog M, Na MX, Titova LV, Veinot JG, Hegmann FA. Charge transfer state emission dynamics in blue-emitting functionalized silicon nanocrystals. Phys Chem Chem Phys (2015) 17:30125–33. doi:10.1039/c5cp04819b
6. Dam B, Osorio CI, Hink MA, Muller R, Koenderink AF, Dohnalova K. High internal emission efficiency of silicon nanoparticles emitting in the visible range. ACS Photon (2018) 5:2129–36. doi:10.1021/acsphotonics.7b01624
7. Fujii M, Minami A, Sugimoto H. Precise size separation of water soluble red to near infrared luminescent silicon quantum dots by gel electrophoresis. Nanoscale (2020) 12:9266–71. doi:10.1039/d0nr02764b
8. Romero JJ, Arciprete ML, Rodriguez HB, Gonik E, Cacciari D, Moore AL, et al. Incorporation of N and O into the shell of silicon nanoparticles offers tunable photoluminescence for imaging uses. ACS Appl Nano Mater (2022) 5:8105–19. doi:10.1021/acsanm.2c01241
9. Yamada H, Watanabe J, Nemoto K, Sun HT, Shirahata N. Postproduction approach to enhance the external quantum efficiency for red light-emitting diodes based on silicon nanocrystals. Nanomaterials (2022) 12:4314. doi:10.3390/nano12234314
10. Negro LD, Yi JH, Michel J, Kimerling LC, Chang TF, Sukhovatkin V, et al. Light emission efficiency and dynamics in silicon-rich silicon nitride films. Appl Phys Lett (2006) 88:233109. doi:10.1063/1.2208378
11. Lin KH, Liou SC, Chen WL, Wu CL, Lin GR, Chang YM. Tunable and stable UV-NIR photoluminescence from annealed SiOx with Si nanoparticles. Opt Express (2013) 21(20):23416–24. doi:10.1364/oe.21.023416
12. Valenta J, Greben M, Gutsch S, Hiller D, Zacharias M. Photoluminescence performance limits of Si nanocrystals in silicon oxynitride matrices. J Appl Phys (2017) 122:144303. doi:10.1063/1.4999023
13. Marquez BP, Flores KE, Garcia SA, Gonzalez ZM, Moreno M, Torres A, et al. Broad and nearly white photoluminescence induced by the nitrogen incorporation in Si/SiOxNy multilayers. J Lumin (2021) 239:118397. doi:10.1016/j.jlumin.2021.118397
14. Meng L, Li S, Chen H, Lei M, Yu G, Wen P, et al. In-situ fabricated amorphous silicon quantum dots embedded in silicon nitride matrix: photoluminescence control and electroluminescence device fabrication. J Lumin (2023) 261:119913. doi:10.1016/j.jlumin.2023.119913
15. Luo Y, Yang X, Yue L, Ren DS, Chen JR. Effect of phosphorus doping on the luminescence intensity of Si-NC in SiO/Si multilayers. Opt Express (2023) 31(15):24566–72. doi:10.1364/oe.494438
16. Tabassum N, Nikas V, Ford B, Huang M, Kaloyeros AE, Gallis S. Time-resolved analysis of the white photoluminescence from chemically synthesized SiCxOy thin films and nanowires. Appl Phys Lett (2016) 109:043104. doi:10.1063/1.4959834
17. Coyopol A, Agustin MA, Salgado GG, Ramirez RL, Trujillo RR, Vivanco MR, et al. Effect of carbon concentration on optical and structural properties in the transition from silicon rich oxide to SiCxOy films formation. J Lumin (2022) 246:118851. doi:10.1016/j.jlumin.2022.118851
18. Wang M, Xie M, Ferraioli L, Yuan Z, Li DS, Yang DR, et al. Light emission properties and mechanism of low temperature prepared amorphous SiNx films. I. Room-temperature band tail states photoluminescence. J Appl Phys (2008) 104:083504. doi:10.1063/1.2996292
19. Amosov AV, Kulchin YN, Dvurechenskii AV, Dzyuba VP. Photoluminescence and excitation energy transfer in non-stoichiometric silicon nitride. J Lumin (2022) 243:118615. doi:10.1016/j.jlumin.2021.118615
20. Kato H, Kashio N, Ohki Y, Seol KS, Noma T. Band-tail photoluminescence in hydrogenated amorphous silicon oxynitride and silicon nitride films. J Appl Phys (2003) 93:239–44. doi:10.1063/1.1529292
21. Chen KJ, Lin ZW, Zhang PZ, Huang R, Dong HP, Huang XF. Luminescence mechanism in amorphous silicon oxynitride films: band tail model or N-Si-O bond defects model. Front Phys (2019) 7:144. doi:10.3389/fphy.2019.00144
22. Zhang PZ, Wang SK, Chen KJ, Wu XL. Investigation on the luminescent stability in amorphous silicon oxynitride systems. Eur Phys J Appl Phys (2020) 89:10304. doi:10.1051/epjap/2020190258
23. Zhang PZ, Zhang L, Lyu F, Wang DB, Zhang L, Wu KP, et al. Luminescent amorphous silicon oxynitride systems: high quantum efficiencies in the visible range. Nanomaterials (2023) 13:1269. doi:10.3390/nano13071269
24. Xu LB, Piao H, Liu Z, Cui C, Yang DR. Sensitized electroluminescence from erbium doped silicon rich oxynitride light emitting devices. J Lumin (2021) 235:118009. doi:10.1016/j.jlumin.2021.118009
25. Topka KC, Diallo B, Puyo M, Papavasileiou P, Lebesgue C, Genevois C, et al. Critical level of nitrogen incorporation in silicon oxynitride films: transition of structure and properties, toward enhanced anticorrosion performance. ACS Appl Electron Mater (2022) 4:1741–55. doi:10.1021/acsaelm.2c00018
Keywords: a-SiNxOy, PL lifetimes, defect states, radiative recombination rates, non-radiative recombination lifetimes
Citation: Zhang P, Liu X, Zhang L, Wang D, Wu K and Wang S (2024) Temperature dependent radiative and non-radiative recombination lifetimes of luminescent amorphous silicon oxynitride systems. Front. Phys. 12:1503269. doi: 10.3389/fphy.2024.1503269
Received: 28 September 2024; Accepted: 23 October 2024;
Published: 31 October 2024.
Edited by:
Yaping Dan, Shanghai Jiao Tong University, ChinaReviewed by:
Massimo Cazzanelli, University of Trento, ItalyJiajing He, Chinese Academy of Sciences (CAS), China
Copyright © 2024 Zhang, Liu, Zhang, Wang, Wu and Wang. This is an open-access article distributed under the terms of the Creative Commons Attribution License (CC BY). The use, distribution or reproduction in other forums is permitted, provided the original author(s) and the copyright owner(s) are credited and that the original publication in this journal is cited, in accordance with accepted academic practice. No use, distribution or reproduction is permitted which does not comply with these terms.
*Correspondence: Pengzhan Zhang, cHp6aGFuZ0BqaXQuZWR1LmNu