- Laboratorio Subterráneo de Canfranc, Huesca, Spain
Deep underground laboratory infrastructures have extensively been used for exploring rare events, such as proton decay, dark matter searches or neutrino interactions, taking advantage of their large muon flux reduction. However, only very few investigations have evaluated the effects of low background radiation environments on living organisms. With this purpose, the Canfranc Underground Laboratory (LSC) launched the Biology Platform in 2021, which provides lab space for approved biology experiments. Two identical laboratories have been built (underground and on surface) to replicate biology experiments under the same conditions, with the main difference being the cosmic radiation background. The access protocol to use the LSC facilities includes two open calls per year and assigned time windows for executing the experimental program, which led to the first eight approved and already running experiments. We describe the scientific program of the Canfranc Biology Platform, which explores extremophiles, viral infection, immune system, multicellularity, development or aging in cosmic silence, and the first experimental results. The Platform also allows to observe the response of life to microgravity in absence of radiation, a key condition to explore life in space.
1 Introduction
Living organisms have evolved under constant exposure to natural environmental radiation, which has been demonstrated to play an important role in the development of life and contributes to many biological defense mechanisms [1]. In fact, the dose to which humans are exposed has been evaluated by international bodies. In particular, the UNSCEAR (United Nations Scientific Committee on the Effects of Atomic Radiation) in its 2008 report [2] describes that most of the radiation we receive comes from natural sources, such as cosmic or external terrestrial radiation. Surprisingly, the man-made sources present a very low contribution to the total ionizing radiation. In order to assess the risks of ionizing radiation exposure the Linear No-Threshold (LNT) model has been widely used, based on the extrapolation of high-dose and low-dose exposure on the effects of living organisms suggesting that even low doses of radiation can be harmful [3]. However, a limited understanding of the biological effects induced by ionizing radiation at low radiation background continues to be a challenge to predict the human health risk associated with low radiation exposure.
In this sense, several biological investigations have been carried out under low background radiation conditions taking advantage of the existence of deep underground laboratories (DULs). These DULs, traditionally used for astroparticle or nuclear physics purposes, have unique location features, shielding from cosmic radiation and reduction of natural radioactivity, which make them the ideal scenario for performing these radiobiological experiments [4, 5]. In fact in recent years, research carried out in these DULs has expanded into new fields, such as biology or radiobiology experiments, demonstrating negative effects induced on the development of the tested organisms in response to the reduction of ionization radiation [6, 7].
Regarding biological evidence, some experiments focused on evaluating the influence of cosmic radiation reduction, were performed at underground facilities of the Gran Sasso National Laboratory (LNGS, Italy) [8]. The first experiment called Pulex, demonstrated, employing yeast as a model organism, that this low background environment affects the defense mechanisms against radiomimetic compounds [9]. More recently, other complex organisms were investigated. Drosophila melanogaster was selected as a model for the evaluation of the effect of low doses of radiation. In this research, Morciano et al showed that the reduced radiation influences Drosophila development and the viability of flies [10]. Following these progresses carried out at LNGS, other underground laboratories have begun to develop their own biological research programs. Experiments at the Modane Underground Laboratory (LSM, France) have investigated the effect of different radiation conditions on Escherichia coli [11]. Several biological assays have been carried out at LBRE Laboratory hosted by the WIPP (United States) since 2011 [12, 13]. The REPAIR project started at SNOLAB (Canada) through using a combination of cell culture and whole organisms models [14]. With the aim of testing new prediction models that are direct competitors of the LNT model, the Surface Astrobiology Laboratory located in Boulby (United Kingdom) has performed bacterial growth assays as part of SELLR project [15]. As a result of all these relevant developments in the biology field, some specific workshops were organized by LSC and LNGS in 2015 and 2019 respectively. In these conferences, called DULIA-Bio, the most relevant progress of the different biology experiments performed at DULs facilities were exposed. The latest DULs to introduce its Biology platform was the LSC, whose construction and development started in 2021, both on surface and in the underground facility. The Bio-platform allows international research groups to submit experimental proposals and perform their research activities in the LSC underground facilities. So far, eight research proposals have been approved to study the influence of low-dose radiation exposure on different model organisms.
The Biology platform available at Canfranc is presented herein, offering an overview of the Canfranc facilities for the study of the influence of low radiation environment on living organisms, as well as the current experimental program.
2 Muons in biology
The LSC is located on the Spanish side of the Pyrenees, at a maximum depth of 800 m, under the Tobazo mountain. It is situated between the international Somport road tunnel (which connects Spain and France) and the historical train tunnel. The rock overburden is equivalent to approximately 2400 m water equivalent (mwe), which reduces the cosmic muon flux underground to 5 10−7 cm−2 s−1 [16]. The total area of the underground laboratory is about 1600 m2, divided in various experimental rooms, with clean rooms, mechanical workshop and the biolab. Several services are available, such us radiopurity service, with high purity germanium detectors and a high sensitivity elemental mass spectrometer, copper electroforming service, the radon abatement system (RAS) [17] or liquid nitrogen production, among others.
Muons are the dominant energetic charged particles at sea level. Muon flux is about one muon per square centimeter per minute at surface with mean energy of 4 GeV. Its flux and energy is affected by solar activity, the geomagnetic field and the amount of matter traveled to the observer. Two facts are important to qualitatively understand the influence of muons in life. Muon energy spectrum above GeV rapidly decreases with energy, with a power law with index close to three, and muon energy losses are dominated by ionization below 500 GeV [18]. Therefore, muons dominantly ionize the medium with a mean energy deposited of 2 MeV per column density units in g/cm2 [19]. Another ionization contribution is due to natural radioactivity, through the decay of the radioactive isotopes of Uranium, Thorium and Potassium and their radioactive products, occurring since the origin of life at the Earth’s surface. In summary, life on the Earth surface has been exposed and adapted to these sources of ionizing radiation. The mainstream question posed to the experiments discussed here is on the biological implications of the drastic reduction of the environmental ionization, i.e., exploring life in cosmic silence.
3 Biology platform at LSC
The LSC Biology Platform was created with the aim of hosting biology experiments in a low background radiation environment. This platform offers the opportunity to develop scientific studies in a multidisciplinary environment, where researchers working with very different microorganisms can share their results and ideas, promoting the development of knowledge and expanding the field of study.
The laboratory is prepared to host experiments with a variety of biological models. Underground and surface facilities are available to replicate experiments, the same model and brand was selected for the equipment and incubators used, to reduce variables that could influence the experiments. The main goal of the experimental proposals is to be able to compare above ground experiments, which are considered as reference radiation, and underground experiments where the muon flux is reduced. In addition, shielding for other types of radiation and a radon-free air system can be included in the experimental setup to study how the reduction of a particular radiation affects metabolism.
3.1 Biology laboratory facilities
At present, both laboratories have been equipped with microbiological and cell biology instruments. There are Class II biological safety cabinets, bacteriological incubators and CO2 incubators, autoclave, orbital incubators, spectrophotometers and a water purification equipment. In addition, the underground laboratory has a −80°C ultra-freezer, a bench-top flow cytometer, a stereomicroscope, and a clinostat, which allows experiments to be carried out in microgravity conditions. On surface, an inverted fluorescence microscope, which contains the necessary filters and objectives typically used for biological samples, is also available under request. In Figure 1, two pictures of the external and underground biology laboratories are shown, where some of the available instruments can be seen.
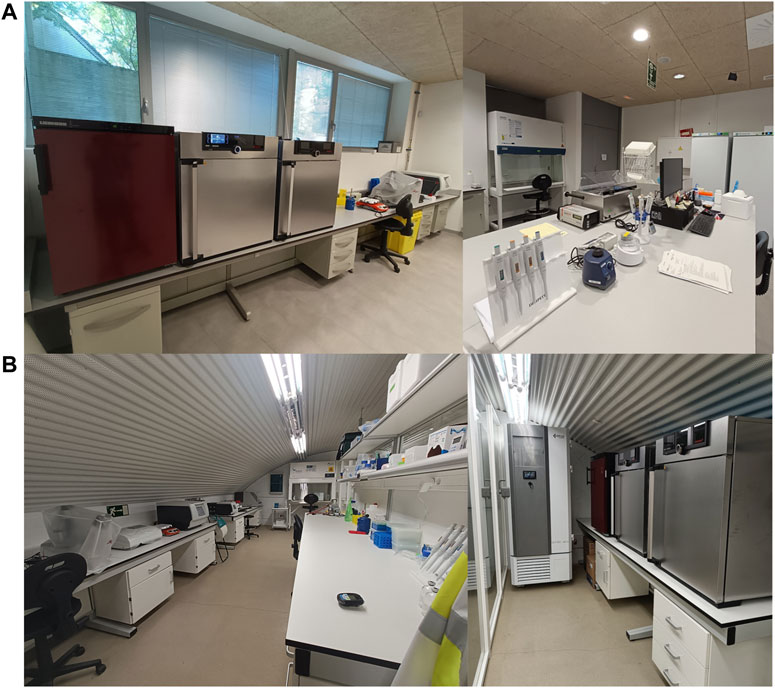
Figure 1. (A) Photograph of the above ground biology laboratory containing some of the equipment described. (B) Underground Biology Laboratory, as a replica of the external laboratory, except for a flow cytometer instrument, which is only located in this location.
In the underground facilities, the LSC can provide researchers its extensive experience in the design of physics experiments. In this vein, similar to rare event research, radiobiology experiments could require different types of shielding, such as lead castles, copper and polyethylene shields, in order to block gammas and neutrons A dedicated control of the radioactive background (background model) is a standard practice in low background physics experiments, but not yet common in biology experiments. This is one of the well-established coordinated efforts with other underground biology laboratories carried out during DULIA meetings, hosted by LSC (https://indico.cern.ch/event/436589) in 2015 and LNGS (https://agenda.infn.it/event/19116/) in 2019, to reach solid conclusions. A compilation of low background facilities, in comparison with underground labs worldwide, has been recently shown [4, 20].
Concerning this radioactive background characterization, continuous monitoring of ambient radiation is carried out at the LSC since 2011. Firstly, the incident radiation is monitored using thermoluminescent dosimeters (TLD), model TLD UD-802A supplied by Panasonic. These dosimeters consist of two different crystals (Li2B4O7 and CaSO4), placed in four independent areas, which are sensitive to different sources of radiation. These TLD are placed in 14 different locations of LSC and are replaced every month. After their exposure, the dose rate is measured employing a UD-716 instrument from Panasonic. The average results obtained for the TLD during 2022 show dose rates of 0.71 ± 0.03 and 1.36 ± 0.03 mSv/year, for Underground and above ground biological areas, respectively (2022 annual LSC data, not published).
Another relevant radiological parameter that must be taken into account is Radon environmental concentration, which is the main source of natural radiation [2]. Radon varies throughout the year and is continuously monitored with calibrated instruments located at six different sites in the LSC facilities. Alphaguard P30 portable radon detectors from Genitron Instruments are used for its determination. The current values obtained for underground facilities (60–100 Bq/m3) are shown to be one-third of the surface levels (200–280 Bq/m3). To improve these values, a Radon Abatement System was put into operation in the underground lab [17] and good ventilation in the surface laboratory is being implemented to match the lower levels of radon in the underground laboratory Background radiation of experiments hosted underground is reduced by eliminating the radon present in air next to the detector or, in this case, the biological samples. The radon abatement system is capable of providing levels of 1 mBq/m3, which are much lower than the Rn levels measured in the above ground laboratory. Taking advantage of this system, the radon-free dry air produced underground can in future be used directly in the microorganism incubators, making sure humidity is correctly added to the air, to explore life in radon-less air conditions.
3.2 Biology platform operation
Research groups or collaborations must submit a written proposal for an experimental activity through an Expression of Interest (EOI) to use the LSC Biology Platform services. This proposal is subject to evaluation by the LSC Scientific Committee, whose members are scientists of international repute. Their role is to give advice on the experimental proposals as well as to monitor the progress of the experiments that have already been approved. The LSC Scientific Committee meets twice a year, so any proposal for evaluation at the following committee meeting must be submitted to the LSC by the deadline specified in the call for proposals.
The EOI must contain the scientific, economic and operational elements necessary for the laboratory to initiate the evaluation process with the assistance of the Scientific Committee. These elements include the title of the project, the spokesperson and structure of the collaboration, the scientific proposal with objectives and work plan or the requirements it implies for the LSC. For more information on this section, please consult the official website of the laboratory (https://lsc-canfranc.es/en/rules-for-bio-proposals/).
3.3 Experiments currently hosted
Several national research groups have expressed interest in hosting their experiments in the underground facilities since the LSC decided to launch the Biology Platform. Between 2021 and 2023, 8 proposals from different research groups of Spanish universities have been approved and have become experiments. All of them have extensive experience of working under reference radiation conditions with the microorganisms selected for the experiments that are being carried out at Canfranc. In underground conditions, they are going to study such important parameters in biology as growth and viability, mutation rate, aging or fitness to the environment. According to the microorganisms studied, the experiments’ proposals have been classified in four groups: bacteria, yeast, cell culture and nematodes. A more detailed explanation of each of the proposals and their status, following this ranking, is given below.
3.3.1 Bacteria
Among all the biological characteristics that can be studied, the growth rate and viability of microorganisms are two of the most remarkable characteristics that can be measured most easily. Focusing on these parameters, the first of the proposals hosted by the LSC, which has been taking data since the platform’s inception in 2021 can be named as bacteria in D2O water. Before bacteria began to be studied at the LSC facilities, the bacteria communities present in D2O were analysed using 16S sequencing showing a low diversity of bacterial populations, dominated by yet uncultivated species of the Nitrosomodaceae family and microorganisms such as Curvibacter sp (non-photosynthetic). This experiment aims to evaluate the effect of reduced cosmic radiation on the viability of bacterial communities recovered from high purity D2O in the absence of externally added nutrients. With this purpose and taking advantage of the underground facilities, this research activity is analyzing bacterial growth and simultaneously determining the elemental content variations in the growth media by ICP-MS on samples taken from the D2O water every 2 months.
Another parameter that can be explored under low radiation conditions is the mutation rate. Selecting this parameter as a target, one of the approved experiments propose to revisit the classical experiment of Luria and Delbrück fluctuation test [21] to evaluate the possible role of cosmic radiation in the mutation rate of bacteria. The directed vs. random character of mutations will be also evaluated. The main hypothesis of this project is that under similar conditions, changes in the rate of point mutation should be observed depending on the radiation level. To perform this research, E. coli was selected as model organisms.
On the other hand, the effects of radiation on DNA damage, already explained in previous sections, can also be analyzed. Some microorganisms have shown to be very versatile by naturally surviving extreme conditions. These extremophiles organisms present natural mechanisms to resist DNA damage such as ionizing and non-ionizing radiation, extreme temperatures or low pressures. Recently, Deinococcus radiodurans, have been studied showing resistance to several extreme environments compatible with outer space [22]. In this sense, other representative microorganisms have been investigated such as Ramazzottius varieornatus or E. coli [23, 24]. At the same time, research developed in underground laboratories has shown that the absence of radiation may result in inefficient DNA repair mechanisms, therefore having a negative impact on cellular growth and survival. For all these reasons, one of the experimental proposals intends to prove if there is a minimum of radiation under which cellular growth can be deemed optimal, while above and below this level, cellular survival decreases. To do that, bacteria cultures will be exposed to increasingly higher levels of radiation analyzing the bacteria growth and response. In particular, the Dsup protein involved in efficient DNA repair mechanisms [24] will be characterized, in the first step of this project, on protecting E. coli from high levels of radiation and, in the following steps, its beneficial effects on mammalian cell cultures will be tested.
3.3.2 Yeast
Another interesting parameter that is under investigation is human aging. Yeast, and more specifically Saccharomyces cerevisiae, is one of the most important model organisms for the study of molecular mechanisms related to human aging and disease. It has played a central role in the discovery of important conserved longevity factors and pathways [25, 26]. The two paradigms to study aging in S. cerevisiae are the chronological and the replicative life span (CLS and RLS, respectively). Following this research, one of the approved experiments currently working at LSC, is focused on the determination of CLS, i.e., the measurement of the mean and maximum survival time of non-dividing yeast populations, aiming to gain insight into the biological impact of low levels of background radiation on the chronological aging of yeast. To achieve this goal, the results of CLS in reference radiation will be compared to those obtained under low radiation conditions. Previous investigations of yeast metabolism performed at Grand Sasso Underground facilities, showed that these decreased radiation levels impair the biological defense of S. cerevisiae against radiomimetic chemical agents [6]. However, no results on yeast lifespan under these conditions have been reported. Therefore, the main objective of the project is to understand the biological impact of low background radiation levels on yeast chronological aging and mutation rate. The experimental measurement of mutation rate in S. cerevisiae is based on the study of certain genes such as CAN1, because of the selectable phenotypes that are produced when the function of this gene is lost due to mutations. In particular, CAN1 is the transporter for the amino acid arginine, which can also be used by its toxic analogue L-canavanine. The presence of L-canavanine in the growth media is used to select yeast mutants. In this way, only mutants that have lost CAN1 functionality are able to grow on plates containing canavanine, as it cannot be introduced into the cells [27].
Finally, the latest yeast-based research accepted to date is focused on the evaluation of the influence of low background radiation on the alteration in subcellular enzymatic reactions, cell mitochondrial function, direct (DNA-repair mechanisms) and indirect (protective mechanisms by antioxidant activity) DNA damage. Most of the experiments carried out in low radiation environments show that, once the cultures have been conditioned, their ability to repair DNA after exposure to DNA-damaging agents (chemical substances, irradiation) is reduced [9, 28, 29]. Focus on those results, this research proposal seeks to determine whether this low radiation conditioned organisms to DNA damage is direct, due to the impairments of DNA-repair mechanisms, or indirect, due to the reduced efficiency of Reactive Oxygen Species (ROS)-scavenging species. The effect of low radiation on enzymatic chemical reactions involved in cellular protection against oxidative damage will be study through the employment of well-established biochemical assays focusing on glutathione peroxidase (GPx) and the determination of mitochondrial functions tests in S. cerevisiae.
3.3.3 Cell cultures
As explained for yeast-based experiments, human aging can also be investigated taking cell cultures as model organisms. Aging is a major risk factor for a wide range of human pathologies. There are two long-argued causes for human aging, namely, epigenetic aging and senescence. Cellular senescence is physiologically beneficial in several contexts. It was originally observed in normal human diploid fibroblasts, which were no longer able to divide after a finite number of cell divisions in culture. Subsequent research has shown that cellular senescence is a state of stable cell cycle arrest that occurs in response to irreparable damage and can be considered a hallmark of aging [30].
Epigenetic aging, which can be measured with so-called “epigenetic clocks,” is due to deterministic processes embedded in the mammalian genome. Stochastic (random) damage, which may be due to wear and tear and/or malfunctioning of stress response mechanisms, leads to cellular senescence. Epigenetic clocks are a maintenance system that appears to be conserved in all model organisms, including humans [31]. Thus, although the relationship between cellular senescence and aging is undisputed, epigenetic aging appears to act independently of the common stressors that induce senescence. Making use of the absence of background radiation from the LSC, the last experiment, which is currently taking data at Canfranc, aims to study the primordial nature of the epigenetic clocks of aging and senescence by measuring their functioning and timing capability in a low background radiation environment. The main objective is to examine the longitudinal aging trajectories of aging clocks across the replicative lifespan to senescence of primary human cells from healthy and premature aging syndromes in cosmic silence.
Apart from aging determination purposes, the reduced radiation observed in underground laboratories has been also correlated to alterations on the growing kinetics in several organisms including bacteria [32], unicellular eukaryotes [9], mammalian cell lines [33] or small multicellular organisms [34, 35]. The difference in growth phenotypes observed in unicellular versus multicellular organisms suggests a distinct response to this particular low background environment that may be influenced by the cellular organization state. Taking this into account, a research group proposed an experiment based on the study of the contribution of cellular organization in the response to low background radiation, by investigating this response in the context of models of unicellular relatives of animals which form multicellular structures during clonal stages in their life cycle (Sphaeroforma arctica) or by aggregation in response to environmental stimuli (Capsaspora owczarzaki) [36, 37]. These selected organisms have been well characterized in its laboratory, offering the opportunity to evaluate the changes that have occurred in cell division and grow of these unicellular organisms, their transition through stages of multicellular formations, and the associated genomic and/or transcriptomic while they are cultured in underground conditions.
3.3.4 Nematodes
To conclude, several studies demonstrated that environment is a critical factor in the virus-host interactions which modifies the outcome of the infection and its severity. Abiotic stresses cause changes in host susceptibility to infection and reduce innate and adaptive immune responses, modifying the infection evolution and the virulence of pathogens. Among these abiotic factors microgravity has been shown to be one that affects immunity [38, 39] and gene regulation [40].
Astronauts can experience various forms of stress during space missions, caused by environmental factors such as radiation, pressure and microgravity. These factors can significantly affect the human physiological state and cause a decline in their immune system [41], but the underlying causes are still not well understood. In this context, DULs become the perfect scenery to study the effects of microgravity as an isolated factor, independently of radiation effects.
For that purpose, Caenorhabditis elegans (C. elegans) and its natural pathogen, Orsay nodavirus have been selected to study the progression of viral infections in those conditions. Experiments assessing the fertility and viability of eggs are being carried out under conditions of infection, absence of muons and microgravity.
4 Preliminary results
The evidence obtained so far in underground radiobiology experiments, oxidative stress and impact on DNA reparation mechanisms, confirm that DULs offer an opportunity to advance in these scientific quests in a multidisciplinary environment, helped by the existing infrastructures for low background experiments exploring neutrino properties and searching for dark matter. Four experiments have already taken data in the underground and on surface labs: 1) Microorganisms observed in heavy water show different count rates when compared to replicas on the surface. Metagenomic content is under analysis [42] 2) Development of C. elegans and response to infection has been explored. The first experiments show differences in the development underground, with observed significant variations in the total number of eggs per generation and in the fraction of viable ones. Infection progress also shows significant differences. Very interestingly, cosmic silence stress is very important when compared to microgravity influence [43]. 3) The experiments studying the primordial nature of the epigenetic clocks of aging and senescence have completed the first phase of data taking. Data are currently under methylation analysis and epigenetic clocks characterization [44]. 4) The de Luria-Delbruck experiment completed underground shows different statistics than the one performed on the surface. Analysis of the results and more statistics are needed to reach firm conclusions [45].
5 Future and perspectives
DULs have been the ideal location for astroparticle physics experiments that require a well-characterized low background environment for many years, but their use for hosting biological experiments is a relatively new field of study that is gaining momentum. With regard to this new research field in underground laboratories, mechanisms underlying the effects observed in living organisms at low levels of radiation are not yet well understood, as the different experiments conducted to date have used different species and different conditions. In order to unify methodologies and results among the different, already established, biology programs, future experiments carried out in underground laboratories should involve the selection of a common model organism evaluated in the different bio-platforms. Further investigations testing different radiation environments over a long period of time should be performed. These steps will allow a deep knowledge of the selected organisms by comparing the results between different DULs, whose radiation doses and environment are different and the evolution of the microorganisms with time.
In addition to the undoubted advances in understanding the role of radiation in life, exploring life in underground labs has further implications on future experiments and applications. In the exploration of life in space (or in other planets), the impact of microgravity (or reduced gravity) on biological processes has been widely explored in experiments on surface and in space missions. The muon flux reduction and, in general, the lack of cosmic and natural radiation in underground laboratory conditions is able to isolate the influence of microgravity on life, from other important influences like cosmic protons and heavier nuclei [46, 47]. Therefore, installations in underground laboratories could serve to simulate specific conditions in space valid to perform biology experiments in long space trips or life on other planets.
Author contributions
RH-A: Conceptualization, Investigation, Writing–original draft, Writing–review and editing. LC-B: Conceptualization, Investigation, Supervision, Writing–original draft, Writing–review and editing. CP-G: Conceptualization, Supervision, Writing–original draft, Writing–review and editing.
Funding
The author(s) declare that financial support was received for the research, authorship, and/or publication of this article. Funding for this work was provided by Spanish Ministry of Science and Technology.
Conflict of interest
The authors declare that the research was conducted in the absence of any commercial or financial relationships that could be construed as a potential conflict of interest.
Publisher’s note
All claims expressed in this article are solely those of the authors and do not necessarily represent those of their affiliated organizations, or those of the publisher, the editors and the reviewers. Any product that may be evaluated in this article, or claim that may be made by its manufacturer, is not guaranteed or endorsed by the publisher.
References
1. Chew MT, Bradley DA, Jones B, Nisbet A, Hill M. Review of the effect of reduced levels of background radiation on living organisms. Radiat Phys Chem (2022) 200:110273–5. doi:10.1016/j.radphyschem.2022.110273
2. United Nations. Scientific committee on the effects of atomic radiation. Sources and effects of ionizing radiation: united Nations scientific committee on the effects of atomic radiation: UNSCEAR 2008 report to the general assembly, with scientific annexes. New York, New York, United States: United Nations (2010).
3. Tubiana M, Feinendegen LE, Yang C, Kaminski JM. The linear no-threshold relationship is inconsistent with radiation biologic and experimental data. Radiology (2009) 251:13–22. doi:10.1148/radiol.2511080671
4. Ianni A. Science in underground laboratories and DULIA-bio. Front Phys (2021) 9:7–10. doi:10.3389/fphy.2021.612417
5. Smith GB, Tabocchini MA, Garay CP. The biogeochemistry, biophysics, radiobiology, and technical challenges of deep subsurface. Front Phys (2021). doi:10.3389/978-2-88966-892-2
6. Liu J, Ma T, Liu Y, Zou J, Gao M, Zhang R, et al. History, advancements, and perspective of biological research in deep-underground laboratories: a brief review. Environ Int (2018) 120:207–14. doi:10.1016/j.envint.2018.07.031
7. Lampe N, Breton V, Sarramia D, Sime-Ngando T, Biron DG. Understanding low radiation background biology through controlled evolution experiments. Evol Appl (2017) 10:658–66. doi:10.1111/eva.12491
8. Esposito G, Anello P, Ampollini M, Bortolin E, Angelis CD, Imperio GD, et al. Underground radiobiology: a perspective at gran Sasso national laboratory. Front Public Heal (2020) 8:611146–7. doi:10.3389/fpubh.2020.611146
9. Satta L, Augusti-Tocco G, Ceccarelli R, Esposito A, Fiore M, Paggi P, et al. Low environmental radiation background impairs biological defence of the yeast Saccharomyces cerevisiae to chemical radiomimetic agents. Mutat Res Lett (1995) 347:129–33. doi:10.1016/0165-7992(95)00031-3
10. Morciano P, Iorio R, Iovino D, Cipressa F, Esposito G, Porrazzo A, et al. Effects of reduced natural background radiation on Drosophila melanogaster growth and development as revealed by the FLYINGLOW program. J Cel Physiol (2018) 233:23–9. doi:10.1002/jcp.25889
11. Lampe N, Biron DG, Brown JMC, Breton V, Marin P, Maigne L, et al. Simulating the impact of the natural radiation background on bacterial systems: implications for very low radiation biological experiments. PLoS One (2016) 11:e0166364–19. doi:10.1371/journal.pone.0166364
Smith GB, Grof Y, Navarrette A, Guilmette RA. Exploring biological effects of low level radiation from the other side of background. Health Phys (2011) 100:263–5. doi:10.1097/hp.0b013e318208cd44
13. Zarubin MP, Kuldoshina OA, Kravchenko EV. Biological effects of low background radiation: prospects for future research in the low-background laboratory DULB-4900 of baksan neutrino observatory INR RAS. Phys Part Nucl (2021) 52:19–30. doi:10.1134/S1063779621010056
14. Thome C, Tharmalingam S, Pirkkanen J, Zarnke A, Laframboise T, Boreham DR. The REPAIR project: examining the biological impacts of sub-background radiation exposure within SNOLAB, a deep underground laboratory. Radiat Res (2017) 188:470–4. doi:10.1667/RR14654.1
15. Wadsworth J, Cockell CS, Murphy ASJ, Nilima A, Paling S, Meehan E, et al. There’s plenty of room at the bottom: low radiation as a biological extreme. Front Astron Sp Sci (2020) 7:1–14. doi:10.3389/fspas.2020.00050
16. Trzaska WH, Slupecki M, Bandac I, Bayo A, Bettini A, Bezrukov L, et al. Cosmic-ray muon flux at Canfranc underground laboratory. Eur Phys J C (2019) 79:721–6. doi:10.1140/epjc/s10052-019-7239-9
17. Pérez-Pérez J, Amare JC, Bandac IC, Bayo A, Borjabad-Sanchez S, Calvo-Mozota JM, et al. Radon Mitigation Applications at the Laboratorio Subterráneo de Canfranc (LSC). Universe (2022) 8:112. doi:10.3390/universe8020112
18. Group. WRL particle D. Review of particle physics. Prog Theor Exp Phys (2022) 15:1–878. doi:10.1093/ptep/ptac097
19. Groom DE, Mokhov NV, Striganov SI. Muon stopping power and range tables 10 MeV-100 TeV. Data Nucl Data Tables (2001) 78:183–356. doi:10.1006/adnd.2001.0861
20. Kamaha A, Mount B, Schnee R. Supporting capabilities for underground facilities. AIP Conf Proc (2023) 2908. doi:10.1063/5.0161437
21. Luria SE, Delbrück M. Mutations of bacteria from virus sensitivity to virus resistance. Genetics (1943) 28:491–511. doi:10.1093/genetics/28.6.491
22. Kawaguchi Y, Shibuya M, Kinoshita I, Yatabe J, Narumi I, Shibata H, et al. DNA damage and survival time course of deinococcal cell pellets during 3 Years of exposure to outer space. Front Microbiol (2020) 11:2050. doi:10.3389/fmicb.2020.02050
23. Jönsson KI, Rabbow E, Schill RO, Harms-Ringdahl M, Rettberg P. Tardigrades survive exposure to space in low Earth orbit. Curr Biol (2008) 18:729–31. doi:10.1016/j.cub.2008.06.048
24. Kirke J, Jin XL, Zhang XH. Expression of a tardigrade Dsup gene enhances genome protection in plants. Mol Biotechnol (2020) 62:563–71. doi:10.1007/s12033-020-00273-9
25. Longo VD, Shadel GS, Kaeberlein M, Kennedy B. Replicative and chronological aging in saccharomyces cerevisiae. Cell Metab (2012) 16:18–31. doi:10.1016/j.cmet.2012.06.002
26. Jung PP, Zhang Z, Paczia N, Jaeger C, Ignac T, May P, et al. Natural variation of chronological aging in the Saccharomyces cerevisiae species reveals diet-dependent mechanisms of life span control. Aging Mech Dis (2018) 4:3–11. doi:10.1038/s41514-018-0022-6
27. Whelan WL, Gocke E, Manney TR. The CAN1 locus of Saccharomyces Cerevisiae: fine-structure analysis and forward mutation rates. Genetics (1979) 91:35–51. doi:10.1093/genetics/91.1.35
28. Satta L, Antonelli F, Belli M, Sapora O, Simone G, Sorrentino E, et al. Influence of a low background radiation environment on biochemical and biological responses in V79 cells. Radiat Environ Biophys (2002) 41:217–24. doi:10.1007/s00411-002-0159-2
29. Carbone MC, Pinto M, Antonelli F, Amicarelli F, Balata M, Belli M, et al. The Cosmic Silence experiment: on the putative adaptive role of environmental ionizing radiation. Radiat Environ Biophys (2009) 48:189–96. doi:10.1007/s00411-008-0208-6
30. Song S, Lam EWF, Tchkonia T, Kirkland JL, Sun Y. Senescent cells: emerging targets for human aging and age-related diseases. Trends Biochem Sci (2020) 45:578–92. doi:10.1016/J.TIBS.2020.03.008
31. Lowe D, Horvath S, Raj K. Epigenetic clock analyses of cellular senescence and ageing. Oncotarget (2016) 7. doi:10.18632/oncotarget.7383
32. Castillo H, Li X, Schilkey F, Smith GB. Transcriptome analysis reveals a stress response of Shewanella oneidensis deprived of background levels of ionizing radiation. PLoS One (2018) 13:e0196472–22. doi:10.1371/journal.pone.0196472
33. Liu J, Ma T, Gao M, Liu Y, Liu J, Wang S, et al. Proteomic characterization of proliferation inhibition of well-differentiated laryngeal squamous cell carcinoma cells under below-background radiation in a deep underground environment. Front Public Heal (2020) 8:584964. doi:10.3389/fpubh.2020.584964
34. Van Voorhies WA, Castillo HA, Thawng CN, Smith GB. The phenotypic and transcriptomic response of the Caenorhabditis elegans nematode to background and below-background radiation levels. Front Public Heal (2020) 8:581796–12. doi:10.3389/fpubh.2020.581796
35. Pirkkanen J, Zarnke AM, Laframboise T, Lees SJ, Tai TC, Boreham DR, et al. A research environment 2 km deep-underground impacts embryonic development in lake whitefish (Coregonus clupeaformis). Front Earth Sci (2020) 8:1–9. doi:10.3389/feart.2020.00327
36. Sebé-Pedrós A, Irimia M, del Campo J, Parra-Acero H, Russ C, Nusbaum C, et al. Regulated aggregative multicellularity in a close unicellular relative of metazoa. Elife (2013) 2013:e01287–20. doi:10.7554/eLife.01287
37. Dudin O, Ondracka A, Grau-Bové X, Haraldsen AAB, Toyoda A, Suga H, et al. A unicellular relative of animals generates a layer of polarized cells by actomyosin-dependent cellularization. Elife (2019) 8:e49801–26. doi:10.7554/eLife.49801
38. Zhu L, Nie L, Xie S, Li M, Zhu C, Qiu X, et al. Attenuation of antiviral immune response caused by perturbation of TRIM25-mediated RIG-I activation under simulated microgravity. Cell Rep (2021) 34:108600. doi:10.1016/j.celrep.2020.108600
39. Gilbert R, Torres M, Clemens R, Hateley S, Hosamani R, Wade W, et al. Spaceflight and simulated microgravity conditions increase virulence of Serratia marcescens in the Drosophila melanogaster infection model. npj Microgravity (2020) 6:4–9. doi:10.1038/s41526-019-0091-2
40. Corydon TJ, Schulz H, Richter P, Strauch SM, Böhmer M, Ricciardi DA, et al. Current knowledge about the impact of microgravity on gene regulation. Cells (2023) 12:1043. doi:10.3390/cells12071043
41. Garrett-Bakelman FE, Darshi M, Green SJ, Gur RC, Lin L, Macias BR, et al. The NASA twins study: a multidimensional analysis of a year-long human spaceflight. Science (2019) 80(364):eaau8650–52. doi:10.1126/science.aau8650
46. Durante M, Cucinotta FA. Physical basis of radiation protection in space travel. Rev Mod Phys (2011) 83:1245–81. doi:10.1103/RevModPhys.83.1245
Keywords: underground laboratories, underground biology, radiobiology, LSC, low radiation environment
Citation: Hernández-Antolín R, Cid-Barrio L and Peña-Garay C (2024) Canfranc biology platform: exploring life in cosmic silence. Front. Phys. 12:1397799. doi: 10.3389/fphy.2024.1397799
Received: 08 March 2024; Accepted: 04 April 2024;
Published: 24 April 2024.
Edited by:
Frank Franz Deppisch, University College London, United KingdomReviewed by:
Maria Antonella Tabocchini, National Institute of Health (ISS), ItalyCopyright © 2024 Hernández-Antolín, Cid-Barrio and Peña-Garay. This is an open-access article distributed under the terms of the Creative Commons Attribution License (CC BY). The use, distribution or reproduction in other forums is permitted, provided the original author(s) and the copyright owner(s) are credited and that the original publication in this journal is cited, in accordance with accepted academic practice. No use, distribution or reproduction is permitted which does not comply with these terms.
*Correspondence: Laura Cid-Barrio, lcid@lsc-canfranc.es