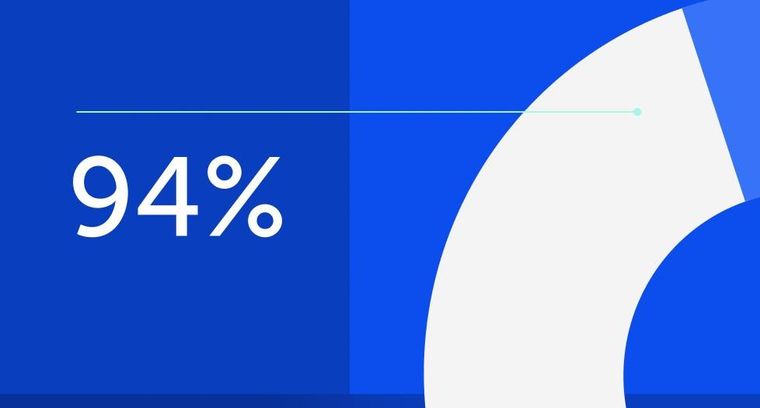
94% of researchers rate our articles as excellent or good
Learn more about the work of our research integrity team to safeguard the quality of each article we publish.
Find out more
REVIEW article
Front. Phys., 06 May 2024
Sec. Biophysics
Volume 12 - 2024 | https://doi.org/10.3389/fphy.2024.1393340
Hydrophobins are small amphiphilic extracellular proteins produced by filamentous fungi; they are surface-active proteins, and their functions are mainly related to their ability to self-assemble into amphipathic monolayers at hydrophobic–hydrophilic interfaces. Depending on their hydropathy patterns and purification requirements, they are classified into class I and class II; both present eight conserved cysteines throughout their sequence, forming four disulfide bridges, which generate four loops that give stability to the protein in its monomeric and folded forms. Class I hydrophobin loops are more extended than class II hydrophobin loops, resulting in differences in assembly on divergent surfaces, additionally accompanied by conformational changes in the protein structure. In the monomer hydrophobin glycosylated form, hydrophobins are rich in β-sheet structure, while being assembled at the water–air interface increases the content of the β-sheet in their structure and is at the interface with water, and a hydrophobic solid such as Teflon also induces the formation of an α-helix structure. The monolayers generated by class I are stable structures called fibrils or rodlets, and class II only produces aggregates. Class I presents a glycosylated chain in its sequence; this causes the formation of the α-helix structure, promoting ordered assemblies, which entails their stability and high insolubility. Fibrils could be dissociated with trifluoroacetic acid and formic acid, which unfolds the protein, while 60% ethanol and 2% sodium dodecyl sulfate solutions dissociate class II aggregates.
Microorganisms are often covered by a surface layer of proteins that shield microbes from external aggression or aid-to-aid microbial dispersion. In bacteria, this protein layer is called the S layer, and in fungi, the rodlet layer. The proteins present in fungal biology are called hydrophobins, and their multiple functions are related to their high surfactant activity, which results from self-assembly at hydrophilic–hydrophobic interfaces to form an amphipathic monolayer [1]. Hydrophobins are surface-active proteins whose activity is similar to that of traditional biosurfactants, which have some lipid in their structure. However, hydrophobin surface activity depends not on a lipid molecule but on the sequence of amino acids that forms it [2]. This activity allows hydrophobins to break up the water layer through self-assembly into hydrophobic–hydrophilic surfaces, which favors decreased surface tension during hyphal growth [1, 3].
These small proteins fulfill various functions in the growth and development of fungi, and they can be secreted out in the surroundings or retained in the fungal structures, such as fruiting bodies or mycelia. The biological functions are diverse but always seem to relate to interactions with interfaces or surfaces in some manner [2, 4]. Here, the pivotal role of the mechanical properties should be addressed in keeping the architecture of the fungal membrane under certain environmental conditions, which is crucial for designing efficient strategies against pathogen contamination [5, 6].
The hydrophobin SC3 is a class I hydrophobin obtained from Schizophyllum commune that forms monolayers called fibrils or rodlets; these structures are similar to those formed by amyloid proteins. It has been reported that the 3D structure of amyloids formed by the HET-s protein of the filamentous fungus Podospora anserina is composed of a β-solenoid (4 parallel β-chains) comprising a hydrophobic core, hydrogen bonds, and 2 helical windings, which are connected by a segment of 15 amino acid residues. Many proteins have been reported to aggregate into amyloids or similar states when the delicate balance between folding and aggregation is disturbed by heat denaturation and the involvement of side-chain interactions [7].
It has been reported that the glycosylation of the hydrophobins promotes the formation of the α-helix structure, which seems typical in class I hydrophobins and is induced during assembly on a hydrophobic solid. For this reason, it is related to the high insolubility of the assembly and the adhesion of the fungus to hydrophobic substrates, such as the insect cuticle, during pathogenesis [2]. For hydrophobin SC3, the monomers diffuse to the hydrophilic–hydrophobic interface, where they initially reach the α-helix structure. While at this interface, the α-helix structure changes to the β-sheet state; at the water–solid interface, hydrophobins remain at the α-helix structure, and the conformational change occurs only at 100 °C in the presence of 2% SDS (Figure 1) [2, 8].
Hydrophobins are proteins positioned as amyloid structure study models (diseases related to the presence of protein aggregates, such as Alzheimer’s disease, Parkinson’s disease, cystic fibrosis, type II diabetes, and Huntington’s disease). They were discovered in 1966 by [9], but it was not until 1980 that they began to be considered an object of research. With hydrophobins in mind as a study model, gathering as much information as possible about them is essential. This review comprehensively discusses the recent information about hydrophobins and their current applications.
Hydrophobins are a family of more than 1,000 proteins [10]. The surface activity of hydrophobins is a product of their amino acid sequences, which also provide information on their physicochemical properties [4]. These proteins are shorter than 160 amino acid residues, and their molecular mass is between 5 and 20 kDa [2, 11]. Hydrophobins are isolated from ascomycetes and basidiomycetes. The Protein Data Bank (PDB) presents information for seven hydrophobins (https://www.rcsb.org/), and the InterPro database (https://www.ebi.ac.uk/interpro/) presents information for 428 hydrophobins from ascomycetes (Trichoderma spp., Penicillium spp., Aspergillus spp., Neurospora spp., and Fusarium spp.) and 1,250 from basidiomycetes (Schizophyllum commune, Agaricus bisporus, Pleurotus ostreatus, and Dictyonema glabratum) [3]. Hydrophobins are classified into two classes, I and II, depending on the patterns, hydropathy, and purification requirements. However, all hydrophobins share eight cysteines that form disulfide bridges, which are found in the same position as in the primary structure (Figure 2). These disulfide bridges increase the rigidity and proteolytic resistance of the proteins, which support their secondary structure [2, 12]. In addition to being secreted in the monomer form with an α-helix topology [12], they self-assemble into amphipathic monolayers (β-barrel structure) when in contact with a hydrophobic–hydrophilic interface. Conversely, they all have a short signal sequence peptide that dictates the same protein secretion type. In addition, it is worth mentioning that the sequences of class I and II hydrophobins have a 34% similarity due to notable differences that lead to their division into two categories [13].
Figure 2. Cysteine positions in the primary structure of class I and II hydrophobins (X represents the amino acid numbers between each cysteine within the structure).
Hydrophobins of this class have between 85 and 95 amino acid residues. The length of its Cys3–Cys4 loops varies between 4 and 44 residues, while its Cys4–Cys5 loops vary between 8 and 23 residues (Figure 2) [14]. The monolayers generated by this class are robust and resistant to enzymatic treatments, detergents such as SDS (2%), and high temperatures (100°C). However, they are dissociable with strong acid treatments (formic acid and trifluoroacetic acid).
An example of this class is hydrophobin SC3, from the basidiomycete S. commune, which reduces the surface tension of water from 72 to 24 mJ/m2 at 25°C [2]. These proteins are the most studied within the hydrophobin family since they were among the first to be discovered and described. SC3 has 112 amino acids and weighs 13,431 Da (UniProt ID: P16933 SC3_SCHCO). In early experiments carried out by [15], it was discovered that the four disulfide bridges present in all hydrophobins, regardless of their classification, are essential for protein stability in their monomeric form since they prevent premature self-assembly (that is, without being in contact with the interface). In addition to these disulfide bridges, SC3 contains between 17 and 22 mannose residues linked to threonine residues in the N-terminal part of the protein, that is, exposed, which gives its characteristic properties to the hydrophilic side of the monolayer. To produce amphipathic monolayers, SC3 undergoes conformational changes within an oligomerization process, requiring a critical concentration of 50 μg/mL [2].
The first conformational change involves going from the monomeric configuration of the protein (the one that maintains a high solubility in aqueous media and is secreted by the cell) to the α-helical structure. This change occurs when the monomers are in contact with the hydrophilic–hydrophobic interface; such contact causes the hydrophobin SC3 to proceed into an intermediate stage, where the content of the α-helix structure increases, allowing hydrophobins to form amyloid fibers, that is, an intermediate between the monomeric and the β-folded conformation [13]. Therefore, it is considered that the change from the monomer state to a helical intermediate is responsible for initiating polymerization. Similarly, the second conformational change consists of the lateral association of β-sheet species to generate rods or fibers of 10 nm in diameter (each composed of 2–3 protofilaments of 2.5-nm wide) and monolayers on a surface or substrate [16]. In addition, Scholtmeijer et al. reported that specific components of the cell wall, such as schizophyllan and β-1-3 glucan, accelerate the formation of β-sheets. The latter was established after observing an increase (80%) in thioflavin T (ThT) fluorescence in 16 h when schizophyllan was added to the solution; in its absence, only a 25% increase in fluorescence was observed. However, the exact mechanism by which they promote agglutination is barely known [17].
EAS is another example of a class I hydrophobin secreted from Neurospora crassa. Its function is to facilitate the formation and dispersal of spores and the formation of impermeable amphipathic monolayers on them. This monolayer is made up of rods associated laterally, which optimizes the dispersion of spores in air and water, thus favoring the survival of N. crassa. It has been established that its Cys7–Cys8 loop spans from residues 19 to 45, constituting the largest loop, giving this region a crucial role in the protein aggregation propensity [18].
Monolayers generated by class I hydrophobins could be detected with fluorescence assays using ThT and staining with Congo red (CR) dye. Implementing these techniques aims, through agitation, to create hydrophobic–hydrophilic interfaces that promote intermolecular association (polymerization) and the consequent formation of amyloid fibers. The amphipathic nuclear structure (present in all these proteins despite the considerable sequence variation in the family) determines the unique properties of hydrophobins. Regardless of the differences between classes I and II, they have no distinction regarding their life cycle [18].
Hydrophobins of this class have approximately 70 residues. The length of its Cys3–Cys4 and Cys7–Cys8 loops is preserved [18]. Class II hydrophobins are abundantly secreted into the growth medium and the cell surface. Monolayers generated are not amyloid in nature as they dissociate into 70% ethanol, detergents such as SDS (2%), and high temperatures (100°C). Furthermore, they are not resistant to acid or enzymatic treatments [2]. An example of this class is the hydrophobin cerato-ulmin (CU) obtained from Ophiostoma ulmi, isolated and partially characterized approximately 25 years ago. CU self-assembles in stirred aqueous solutions subjected to vacuum or aeration, and in large quantities, it forms fibrils that increase the turbidity of the solution and membranes on the surface of an aqueous solution [19].
Class I and II hydrophobins differ in their arrangement of amino acids, that is, while the loops formed by disulfide bridges vary in the number and type of amino acids among class I hydrophobins, the loops of class II hydrophobins are more conserved [2] (Figure 3).
Another significant difference between both classes is that in class I, the charged residues are delimited to a section within the three-dimensional conformation of the proteins, that is, the charge of the protein is localized. In contrast, the charged residues in class II hydrophobins are better distributed throughout the three-dimensional structure (Figure 4). This difference is responsible for the distinction between a hydrophobic and hydrophilic side in class I proteins and the absence of this distinction in class II hydrophobins. However, in the latter, hydrophobic regions could also be exposed to the phase gas from a hydrophobic–hydrophilic interface to form the characteristic monolayers of these proteins. This quality explains the ability of hydrophobins to form amphipathic monolayers in both classes [18].
Figure 4. Electrostatic surface of hydrophobins. (A) Class I hydrophobin from S. commune. PDB ID: 7S7S. (B) Class II hydrophobin from Trichoderma reesei. PDB ID:2B97. Structure representations were prepared using ChimeraX 1.7.1.
Hydrophobins play a vital role in the growth and development of most filamentous fungi. The formation of aerial hyphae is a process in which the secretion of surfactant molecules reduces surface tension, affecting the cohesive force between water molecules on the surface of aqueous environments (Figures 5A, B) [20, 21]. These surfactant molecules are hydrophobins, which are excreted to form a layer or rodlet around the hyphae, conferring hydrophobicity to the surface of the fungus. Self-assembly of hydrophobins on the cell wall surface gives an amphipathic trait to aerial hyphae (Figure 5C), fruiting bodies, the lining of air cavities in fruiting bodies (Figure 5D), and spores. The preceding results in the attachment of hyphae to hydrophobic surfaces. Class I hydrophobins assemble into a stable, amyloid-type fibril that can only be dissociated using trifluoroacetic and formic acid. In contrast, class II hydrophobins do not assemble into amyloid-type fibrils and can dissociate in 70% ethanol, 2% SDS, or by applying pressure [21, 22].
Figure 5. Representation of the biological role of hydrophobins during the growth and development of filamentous fungi. (A) Secretion of hydrophobin monomers, (B) formation of the monolayer at the interface and germination of a new hypha, (C) hydrophobin secreted by emerging aerial hyphae cannot diffuse, and (D) formation of a new monolayer on the surface of the exited hypha.
The first step in forming fungal aerial structures is the escape of individual hyphae from the moist substrate into air. Hyphae secrete hydrophobins, which remain free in the aqueous medium and, therefore, cannot diffuse, remaining at the cell wall–air interface. This process induces the formation of a film of these amphipathic proteins, conferring hydrophobicity to the fungal hyphae. On one hand, the hydrophilic part faces the hydrophilic cell wall, while the hydrophobic part is exposed [20, 22].
The hydrophobic part of hydrophobins has a characteristic rodlet pattern, which is observed in layers on the surfaces of fungal aerial structures. As Wosten suggests, these layers could result from assembling class I hydrophobins [22].
The dikaryotic phase involves coating air channels within the fruiting bodies. This maintains the hydrophobic nature of air channels and avoids waterlogging, thus allowing the aeration of multicellular structures. The last phenomenon was observed in the hydrophobins SC4 and ABH1 produced by S. commune and A. bisporus, respectively [23].
Hydrophobins in an aqueous solution can self-assemble, at the interfaces, into an amphipathic film, reversing the wettability of surfaces and, thus, changing a hydrophilic surface to a hydrophobic surface and vice versa. A hydrophilic surface has a contact angle of less than 90°, while a hydrophobic surface shows a contact angle between 90° and 120° (Figure 6) [2]. Hydrophobin surface activity has been measured by the contact angle of water droplets on different surfaces, such as Teflon, glass, mica, and polyethylene [24].
Figure 6. Schematization of the contact angles between water droplets and surfaces with different hydrophobicity degrees.
Hydrophobins facilitate adhesion to the insect cuticle for invasion. The infection begins with the attachment of the spore to the insect cuticle, and then, enzymes, proteins, and other factors are expressed to facilitate the penetration of the cuticle [25, 26]. It has been reported that hydrophobins from Aspergillus fumigatus contribute to the immune evasion of conidia by hiding host Dectin-1- and Dectin-2-dependent immune recognition of fungal spores [27].
Many conidiospores are characterized by having a rodlet surface made up of class I hydrophobins, while class II hydrophobins are present in yeast-type cells. In addition to aiding the dispersion of contagious propagules by wind, these hydrophobin layers also serve as a scaffold for the attachment to the host surface. Once the infectious propagule attaches, it can colonize the host, which may be mediated by forming an infection structure called the appressorium [22, 25].
In the food industry, foams constitute many products manufactured and sold today. These foams range from the foam of a beer to the porous structure of bread. Hydrophobins are ideal as a case study for foams and emulsions over other small proteins used to stabilize emulsions and foams due to their high surfactant nature and self-assembling properties [28]. These proteins present significant structural variability, generating even more tremendous potential as new surfactant molecules. Hydrophobins bind together, producing an elastic membrane at the interface that produces a barrier against the escape of air particles. These small proteins reduce the surface tension and cover the surface quickly, allowing smaller air cells to be created, thus producing a buffer against coalescence [29].
Amyloid proteins are biomolecules that result from unconventional folding and are characterized by their ability to self-assemble through hydrogen bonds into fibrous-type quaternary structures. These aberrant proteins are involved in diseases known as amyloidosis, such as Alzheimer’s disease, Parkinson’s disease, cystic fibrosis, type II diabetes, and Huntington’s disease, which have no cure [30, 31]. Functional amyloids are considered double-edged swords since, due to this, they have gained the interest of many scientists to try to understand the conditions and the mechanism that allow the transformation of normal proteins into toxic species capable of causing diseases so severe that they can lead to death. To make this possible, scientific research is dedicated to proposing and consolidating biological models that can adequately represent diseases, making it viable to extrapolate behavior from the model to human physiology. Those structures require amyloid conformation to carry out their biological function (which does not derive from unconventional protein folding). There are multiple examples of functional amyloids in nature, ranging from yeasts, bacteria of various genera (both pathogenic and non-pathogenic), protozoans, plants, and even mammals [14].
The self-assembly of insoluble proteins as aggregates or deposits can be toxic and harmful to any organism. However, hydrophobins represent the duality of amyloids since their study allows the generation of knowledge about organisms that use non-traditional proteins to carry out their biological functions. In contrast, in other situations, these proteins imply incurable diseases [30].
Staphylococcus epidermidis is a microorganism that prevails in the normal human epidermal microbiota. It is not a pathogen but has attracted attention due to its ability to colonize medical devices through biofilms, thus compromising the health of patients with weakened immune systems. Furthermore, it has been suggested that these biofilms could allow antibiotic resistance by S. epidermidis. The protein Sbp (small basic protein) functions as a scaffold for biofilms and the cell adhesion required for their assembly; its amyloid nature allows it to form repeated structures along a surface (biotic or abiotic) [32].
BslA is a hydrophobin responsible for the specialized coating of biofilms generated by Bacillus subtilis. The main characteristic of this coating is its elasticity, which allows the viability and resistance of the biofilm. The structure of BslA presents a highly hydrophobic region responsible for the waterproofing of the biofilm; it is considered the most representative quality of this protein [33]. It should be noted that this waterproof function of the coating is selective, giving the possibility of diffusion of nutrients essential for the survival of this organism. In addition to the above property, this amphipathic monolayer presents two conformational states. One is compressed, where curvatures are perceived on the surface, and the other is in equilibrium, where the leveling of the proteins at the same height is observed. The presence of the equilibrium implicitly suggests the existence of a mixture of different structures [34].
Amphipathic hydrophobins can be used for many purposes, such as increasing biocompatibility in medical implants, immobilizing enzymes, and improving the solubility of some water-insoluble drugs [35].
Hydrophobin applications are extensive and novel. Recently, their study has focused on their physical properties for biotechnological applications, ranging from hydrophobic or hydrophilic surface modifications to facilitate the attachment of enzymes or other proteins to their use in biosensors to attach enzymes to electrodes. On the other hand, it has been observed that hydrophobins can also stabilize emulsions and could, therefore, act as surfactants and emulsifiers in processed foods and cleaning agents [36].
Hydrophobins offer a simple and efficient alternative to optimize the solubility and stability of medications in suspension. Solubility and stability are factors directly related to the availability of the drug, which can be modified in terms of the concentration of the active molecule or the release time (when this is a treatment that requires the prolonged release of a drug). In addition to the above application, hydrophobins in the pharmaceutical industry protect the drug during processing, formulation, and storage, maintaining its properties under optimal conditions to provide a more effective treatment [37]. This application is useful when it comes to drugs administered orally due to the process that takes place before the drug reaches its active site [38].
Class II hydrophobins from Trichoderma reesei (HFBI and HFBII) were used to interact strongly with nonionic surfactants, thus optimizing the purification of recombinant proteins in biphasic aqueous systems [20]. [39] tested this application for the first time, obtaining a yield of 90% and a 6-fold increase in purification in the concentration of the purified protein. Another example within this category is the ability of hydrophobins to facilitate the overexpression of proteins from plant extracts. In this case, hydrophobins fuse with a marker to form protein bodies within the host cell, protecting external proteins from degradation due to host defense action, thus reducing necrosis in the leaves [40].
[41] proposed a methodology for coating biomedical silicone implants with titanium dioxide biofilms. This compound, with electrical, optical, and chemical properties, helps develop microelectronic devices, photonic materials, catalysts, and bioremediation and medical treatments among others. Biofilms were generated from a modified class I hydrophobin (derived from the DewA protein of the organism Aspergillus nidulans). The implant coating was uniform, resistant, and elastic, characteristics necessary for biocompatibility.
In another study, [42] modified the same hydrophobin mentioned above, inserting the RGD sequence, or the globular domain of laminin, to functionalize the surfaces of orthopedic titanium implants. This functionalization optimized the adhesion of human cells and impaired the adhesion of pathogens such as Staphylococcus aureus, thus minimizing the risk of bacterial infection.
Functionalization of plastic surfaces began with class I hydrophobins SC3 and SC4 (both in their native and recombinant forms) on the Teflon surface [2]. The interest in functionalizing these materials (plastics) appeared due to the hypothesis of biocompatibility that would allow cell adhesion and tissue regeneration. Subsequently, it was extended to antimicrobial functionalization with two types of hydrophobins (Vmh2 and Pac3), originating from the organism Acremonium sclerotigenum, where the reduction of biofilms formed by different strains of S. epidermidis on polystyrene surfaces was observed [43].
Regarding biosensors on plastic surfaces, the EAS (for “easily wettable”) hydrophobin from the organism N. crassa was used for the α-factor detection and quantification, a peptide hormone from yeast. The methodology consists of functionalizing a polystyrene surface on which an inverted enzyme-linked immunosorbent assay (ELISA) is performed [44].
Another application is pesticide detection using the hydrophobin Vmh2 from Pleurotus ostreatus. In this technique, Vmh2 is fused with the enzyme glutathione S-transferase to quantify pesticides such as molinate and captan, which inhibits its enzymatic activity. It is worth mentioning that this test is also carried out on a functionalized polystyrene surface [45].
The modification of plastic materials using hydrophobins has increased in recent years. Different plastics have been reported using class I hydrophobins from native and recombinant sources. Such is the case with Teflon, polystyrene, polycaprolactone, and plastic biliary stents, which have applications after being modified in the medical area, biosensors, and enzyme immobilization, respectively [46].
The 2D materials have a large surface area about their volume and offer the possibility of a wide variety of uses through their functionalization. These 2D materials have allowed the synthesis of high-quality, stable liquid dispersions, which consist of a few layers, maintaining an ideal thickness, for applications related to photoluminescence [47]. Carbon nanotubes (CNTs) are allotropes of carbon (such as diamond, graphite, and fullerenes); their structure is described as a sheet of graphene rolled to form a tube. This winding can be single or multiple, resulting in a single-walled tube or one with multiple walls. The biggest problem with a CNT is that its low solubility in water and toxicity limit its use in some biomedical issues [48]. Due to this, hydrophobins have been widely used to minimize these drawbacks and provide these CNTs with a plethora of applications, including biological, pharmaceutical, chemical, and industrial applications [49].
CNTs, 2D materials, and other materials could offer applications related to enzyme immobilization, optimization of reaction kinetics, and biocatalytic efficiency in industrial processes. For example, the recombinant hydrophobin HFB (HYDPt-1) from the organism Pisolithus tinctorius was used to immobilize small molecules of an electroactive nature on three different substrates [50]. The immobilization of molecules with hydrophobins allows the optimization of immunoassays since materials coated by these proteins are used as platforms in antibody capture systems. One of the materials that can be covered with hydrophobins, such as Vmh2, is glass. The functionalization of this surface allowed the creation of a platform for immobilizing nanomaterials, such as quantum dots and graphene oxides [51].
Due to the biodegradable and non-toxic properties of hydrophobins, they have been proposed as an ecological alternative in many commercial sectors, the textile industry being one of the most striking. Among these innovations is the modification of fabrics, making them more hydrophobic, functionalization with antimicrobial agents (such as zinc and silver oxide nanocomposites), and even ignition resistance. The advantage of using hydrophobins in the textile sector is that the coating of the fabrics does not change their feel, comfort, or weight, thus making them ideal green alternatives. Among the properties that hydrophobins can attribute to textiles when functionalized, the following stand out.
The mechanism by which the functionalization of cotton with hydrophobins retards fire consists of the dehydration of the glycosylated units that are part of the cotton fabric, which, in turn, results in them not detaching or undergoing depolymerization. By avoiding this, the glycosylated units cannot fuel a flame and prevent the material from catching fire, becoming a carbon structure that does not spread fire [52].
Zinc and silver oxide nanocomposites have antimicrobial effects, but the main problem lies in adhering them to fabrics such as cotton and polyester. At this point, hydrophobins prove useful since their ability to form resistant monolayers on surfaces is advantageous in distributing the nanoparticles over the entire fabric evenly without affecting the garment comfort. Hydrophobins in fabrics have been reported to inhibit the growth of microorganisms and protect the material against odors, stains, and degradation specific to said growth [53].
In the medical area, hydrophobins can be used to promote cell adhesion in the presence of biomaterials by being used as an antifouling agent. Likewise, it has been shown that coatings with hydrophobins can improve the growth and morphology of cells such as fibroblasts on surfaces such as Teflon [54]. In personal care and pharmaceuticals, hydrophobins also play an important role as stabilizers in creams, ointments, and formulations in general [54]. Various authors have studied hydrophobins in different applications, as shown in Table 1.
The binding of various molecules could be manipulated by changing the surface hydrophobicity of hydrophobins, which could be used in antifouling applications. Generally, antifouling increases the hydrophobicity of surfaces to repel dirt and prevent the growth of organisms, which represents a significant problem for the shipping industry [54]. For this reason, hydrophobin coatings could offer a solution and even replace existing applications while protecting the environment.
Hydrophobins are involved in producing immune reactions and allergies. In this sense, it has been reported that the conidia of fungus genera Cladosporium, Penicillium, Alternaria, and Aspergillus contain antigens and allergens. Aimanianda et al. (2009) found that the rodlet layer produced by the hydrophobin from the pathogen A. fumigatus was immunologically inert. It did not induce dendritic cell or alveolar macrophage maturation and activation and failed to activate helper T-cell immune responses in vivo [61].
In fungi and bacteria, hydrophobins are proteins with unique characteristics, such as amphipathicity, enabling them to self-assemble at hydrophobic–hydrophilic interfaces. Due to their non-toxic nature, these proteins are suitable for medical and pharmaceutical applications. The classification (class I and II) of these proteins also makes them ideal for use as biosurfactants, surface modifiers (textile industry), and protein purification agents. When hydrophobins self-assemble on hydrophobic and hydrophilic surfaces and interfaces, they develop surface activity; this property favors the formation of monolayers that promote the adhesion of antimicrobial elements, decrease the surface tension at the liquid–air interface, enable the fungal hyphae to interact at another interface, and facilitates protein overexpression and foam and emulsion stabilization. Given the importance of the surface phenomena of hydrophobins, their use is feasible in multiple applications. In recent years, research has allowed us to understand the self-assembly process of hydrophobins; however, it is necessary to continue studying the relationship between the structure and functions of these proteins to know how to work with them and, thus, to contribute scientific knowledge in the area.
JR-O: conceptualization, data curation, formal analysis, funding acquisition, investigation, methodology, software, supervision, validation, visualization, writing–original draft, and writing–review and editing. MQ-Q: data curation, formal analysis, investigation, methodology, software, writing–original draft, and writing–review and editing. AE-V: conceptualization, data curation, formal analysis, investigation, methodology, and writing–original draft. AB: conceptualization, data curation, formal analysis, investigation, methodology, writing–original draft, and writing–review and editing. HN: conceptualization, data curation, formal analysis, funding acquisition, investigation, methodology, project administration, resources, software, supervision, validation, visualization, writing–original draft, and writing–review and editing.
The author(s) declare that no financial support was received for the research, authorship, and/or publication of this article.
The authors acknowledge Consejo Nacional de Humanidades, Ciencias y Tecnologías (CONAHCYT) for funding and granting the scholarship (JR-O).
The authors declare that the research was conducted in the absence of any commercial or financial relationships that could be construed as a potential conflict of interest.
All claims expressed in this article are solely those of the authors and do not necessarily represent those of their affiliated organizations, or those of the publisher, the editors, and the reviewers. Any product that may be evaluated in this article, or claim that may be made by its manufacturer, is not guaranteed or endorsed by the publisher.
1. Bayry J, Aimanianda V, Guijarro JI, Sunde M, Latge JP. Hydrophobins--Unique fungal proteins. Plos Pathog (2012) 8(5):e1002700. Epub 20120531. doi:10.1371/journal.ppat.1002700
2. Wosten HA, de Vocht ML. Hydrophobins, the fungal coat unravelled. Biochim Biophys Acta (2000) 1469(2):79–86. doi:10.1016/s0304-4157(00)00002-2
3. Kulkarni S, Nene S, Joshi K. Production of hydrophobins from fungi. Process Biochem (2017) 61:1–11. doi:10.1016/j.procbio.2017.06.012
4. Linder MB. Hydrophobins: proteins that self assemble at interfaces. Curr Opin Colloid (2009) 14(5):356–63. doi:10.1016/j.cocis.2009.04.001
5. Magazzu A, Marcuello C. Investigation of soft matter nanomechanics by atomic force microscopy and optical tweezers: a comprehensive review. Nanomaterials (Basel) (2023) 13(6):963. Epub 20230307. doi:10.3390/nano13060963
6. Winandy L, Schlebusch O, Fischer R. Fungal hydrophobins render stones impermeable for water but keep them permeable for vapor. Sci Rep (2019) 9(1):6264. Epub 20190418. doi:10.1038/s41598-019-42705-w
7. Riek R. The three-dimensional structures of amyloids. Cold Spring Harb Perspect Biol (2017) 9(2):a023572. Epub 20170201. doi:10.1101/cshperspect.a023572
8. Scholtmeijer K, Wessels JG, Wosten HA. Fungal hydrophobins in medical and technical applications. Appl Microbiol Biotechnol (2001) 56(1-2):1–8. doi:10.1007/s002530100632
9. Hess WM, Sassen MM, Remsen CC. Surface structures of frozen-etched Penicillium conidiospores. Naturwissenschaften (1966) 53(24):708. doi:10.1007/BF00602737
10. Ball SR, Kwan AH, Sunde M. Hydrophobin rodlets on the fungal cell wall. Curr Top Microbiol Immunol (2020) 425:29–51. doi:10.1007/82_2019_186
11. Pennacchio A, Cicatiello P, Notomista E, Giardina P, Piscitelli A. New clues into the self-assembly of Vmh2, a basidiomycota class I hydrophobin. Biol Chem (2018) 399(8):895–901. doi:10.1515/hsz-2018-0124
12. Macindoe I, Kwan AH, Ren Q, Morris VK, Yang W, Mackay JP, et al. Self-assembly of functional, amphipathic amyloid monolayers by the fungal hydrophobin eas. Proc Natl Acad Sci U S A (2012) 109(14):E804–11. Epub 20120123. doi:10.1073/pnas.1114052109
13. Butko P, Buford JP, Goodwin JS, Stroud PA, McCormick CL, Cannon GC. Spectroscopic evidence for amyloid-like interfacial self-assembly of hydrophobin Sc3. Biochem Biophys Res Commun (2001) 280(1):212–5. doi:10.1006/bbrc.2000.4098
14. Pham CL, Kwan AH, Sunde M. Functional amyloid: widespread in nature, diverse in purpose. Essays Biochem (2014) 56:207–19. doi:10.1042/bse0560207
15. de Vocht ML, Reviakine I, Wosten HA, Brisson A, Wessels JG, Robillard GT. Structural and functional role of the disulfide bridges in the hydrophobin Sc3. J Biol Chem (2000) 275(37):28428–32. doi:10.1074/jbc.M000691200
16. Wang X, Shi F, Wosten HA, Hektor H, Poolman B, Robillard GT. The Sc3 hydrophobin self-assembles into a membrane with distinct mass transfer properties. Biophys J (2005) 88(5):3434–43. Epub 20050304. doi:10.1529/biophysj.104.057794
17. Scholtmeijer K, de Vocht ML, Rink R, Robillard GT, Wosten HA. Assembly of the fungal Sc3 hydrophobin into functional amyloid fibrils depends on its concentration and is promoted by cell wall polysaccharides. J Biol Chem (2009) 284(39):26309–14. Epub 20090804. doi:10.1074/jbc.M109.005553
18. Kwan AH, Macindoe I, Vukasin PV, Morris VK, Kass I, Gupte R, et al. The cys3-cys4 loop of the hydrophobin eas is not required for rodlet formation and surface activity. J Mol Biol (2008) 382(3):708–20. Epub 20080722. doi:10.1016/j.jmb.2008.07.034
19. Temple B, Horgen PA. Biological roles for cerato-ulmin, a hydrophobin secreted by the elm pathogens, ophiostoma ulmi and O. Novo-ulmi. Mycologia (2000) 92(1):1–9. doi:10.1080/00275514.2000.12061123
20. Ren Q, Kwan AH, Sunde M. Two forms and two faces, multiple states and multiple uses: properties and applications of the self-assembling fungal hydrophobins. Biopolymers (2013) 100(6):601–12. doi:10.1002/bip.22259
21. Wosten HA, Scholtmeijer K. Applications of hydrophobins: current state and perspectives. Appl Microbiol Biotechnol (2015) 99(4):1587–97. Epub 20150108. doi:10.1007/s00253-014-6319-x
22. Wosten HA. Hydrophobins: multipurpose proteins. Annu Rev Microbiol (2001) 55:625–46. doi:10.1146/annurev.micro.55.1.625
23. Whiteford JR, Spanu PD. Hydrophobins and the interactions between fungi and plants. Mol Plant Pathol (2002) 3(5):391–400. doi:10.1046/j.1364-3703.2002.00129.x
24. Lumsdon SO, Green J, Stieglitz B. Adsorption of hydrophobin proteins at hydrophobic and hydrophilic interfaces. Colloids Surf B Biointerfaces (2005) 44(4):172–8. doi:10.1016/j.colsurfb.2005.06.012
25. Wessels J, De Vries O, Asgeirsdottir SA, Schuren F. Hydrophobin genes involved in formation of aerial hyphae and fruit bodies in Schizophyllum. Plant Cell (1991) 3(8):793–9. doi:10.1105/tpc.3.8.793
26. Zhang S, Xia YX, Kim B, Keyhani NO. Two hydrophobins are involved in fungal spore coat rodlet layer assembly and each play distinct roles in surface interactions, development and pathogenesis in the entomopathogenic fungus, Beauveria bassiana. Mol Microbiol (2011) 80(3):811–26. Epub 20110314. doi:10.1111/j.1365-2958.2011.07613.x
27. Blango MG, Kniemeyer O, Brakhage AA. Conidial surface proteins at the interface of fungal infections. Plos Pathog (2019) 15(9):e1007939. Epub 20190912. doi:10.1371/journal.ppat.1007939
28. Green AJ, Littlejohn KA, Hooley P, Cox PW. Formation and stability of food foams and aerated emulsions: hydrophobins as novel functional ingredients. Curr Opin Colloid (2013) 18(4):292–301. doi:10.1016/j.cocis.2013.04.008
29. Shokribousjein Z, Deckers SM, Gebruers K, Lorgouilloux Y, Baggerman G, Verachtert H, et al. Hydrophobins, beer foaming and gushing. Cerevisia (2011) 35(4):85–101. doi:10.1016/j.cervis.2010.12.001
30. Ghiso J, Frangione B. Amyloidosis and Alzheimer's disease. Adv Drug Deliv Rev (2002) 54(12):1539–51. doi:10.1016/s0169-409x(02)00149-7
31. Iadanza MG, Jackson MP, Hewitt EW, Ranson NA, Radford SE. A new era for understanding amyloid structures and disease. Nat Rev Mol Cel Biol (2018) 19(12):755–73. doi:10.1038/s41580-018-0060-8
32. Wang Y, Jiang J, Gao Y, Sun Y, Dai J, Wu Y, et al. Staphylococcus epidermidis small basic protein (Sbp) forms amyloid fibrils, consistent with its function as a scaffolding protein in biofilms. J Biol Chem (2018) 293(37):14296–311. Epub 20180726. doi:10.1074/jbc.RA118.002448
33. Hobley L, Ostrowski A, Rao FV, Bromley KM, Porter M, Prescott AR, et al. Bsla is a self-assembling bacterial hydrophobin that coats the Bacillus subtilis biofilm. Proc Natl Acad Sci U S A (2013) 110(33):13600–5. Epub 20130731. doi:10.1073/pnas.1306390110
34. Kobayashi K, Iwano M. Bsla (yuab) forms a hydrophobic layer on the surface of Bacillus subtilis biofilms. Mol Microbiol (2012) 85(1):51–66. Epub 20120528. doi:10.1111/j.1365-2958.2012.08094.x
35. Zampieri F, Wosten HAB, Scholtmeijer K. Creating surface properties using a palette of hydrophobins. Materials (Basel) (2010) 3(9):4607–25. Epub 20100906. doi:10.3390/ma3094607
36. Sunde M, Kwan AH, Templeton MD, Beever RE, Mackay JP. Structural Analysis of hydrophobins. Micron (2008) 39(7):773–84. Epub 20070810. doi:10.1016/j.micron.2007.08.003
37. Heinonen H, Laaksonen P, Linder MB, Hentze H-P. Engineered hydrophobin for biomimetic mineralization of functional calcium carbonate microparticles. J Biomater Nanobiotechnology (2014) 05(No.01):1–7. doi:10.4236/jbnb.2014.51001
38. Valo HK, Laaksonen PH, Peltonen LJ, Linder MB, Hirvonen JT, Laaksonen TJ. Multifunctional hydrophobin: toward functional coatings for drug nanoparticles. ACS Nano (2010) 4(3):1750–8. doi:10.1021/nn9017558
39. Collen A, Persson J, Linder M, Nakari-Setala T, Penttila M, Tjerneld F, et al. A novel two-step extraction method with detergent/polymer systems for primary recovery of the fusion protein endoglucanase I-hydrophobin I. Biochim Biophys Acta (2002) 1569(1-3):139–50. doi:10.1016/s0304-4165(01)00244-6
40. Joensuu JJ, Conley AJ, Lienemann M, Brandle JE, Linder MB, Menassa R. Hydrophobin fusions for high-level transient protein expression and purification in Nicotiana benthamiana. Plant Physiol (2010) 152(2):622–33. Epub 20091211. doi:10.1104/pp.109.149021
41. Santhiya D, Burghard Z, Greiner C, Jeurgens LP, Subkowski T, Bill J. Bioinspired deposition of Tio2 thin films induced by hydrophobins. Langmuir (2010) 26(9):6494–502. doi:10.1021/la9039557
42. Boeuf S, Throm T, Gutt B, Strunk T, Hoffmann M, Seebach E, et al. Engineering hydrophobin dewa to generate surfaces that enhance adhesion of human but not bacterial cells. Acta Biomater (2012) 8(3):1037–47. Epub 20111202. doi:10.1016/j.actbio.2011.11.022
43. Cicatiello P, Dardano P, Pirozzi M, Gravagnuolo AM, De Stefano L, Giardina P. Self-assembly of two hydrophobins from marine fungi affected by interaction with surfaces. Biotechnol Bioeng (2017) 114(10):2173–86. Epub 20170605. doi:10.1002/bit.26344
44. Hennig S, Rodel G, Ostermann K. Hydrophobin-based surface engineering for sensitive and robust quantification of yeast pheromones. Sensors (Basel) (2016) 16(5):602. Epub 20160427. doi:10.3390/s16050602
45. Piscitelli A, Pennacchio A, Longobardi S, Velotta R, Giardina P. Vmh2 hydrophobin as a tool for the development of "Self-Immobilizing" enzymes for biosensing. Biotechnol Bioeng (2017) 114(1):46–52. Epub 20160726. doi:10.1002/bit.26049
46. Piscitelli A, Cicatiello P, Gravagnuolo AM, Sorrentino I, Pezzella C, Giardina P. Applications of functional amyloids from fungi: surface modification by class I hydrophobins. Biomolecules (2017) 7(3):45. Epub 20170626. doi:10.3390/biom7030045
47. Kaur J, Gravagnuolo AM, Maddalena P, Altucci C, Giardina P, Gesuele F. Green synthesis of luminescent and defect-free bio-nanosheets of MoS2: interfacing two-dimensional crystals with hydrophobins. Rsc Adv (2017) 7(36):22400–8. doi:10.1039/c7ra01680h
48. De Volder MF, Tawfick SH, Baughman RH, Hart AJ. Carbon nanotubes: present and future commercial applications. Science (2013) 339(6119):535–9. doi:10.1126/science.1222453
49. Wang ZF, Wang YY, Huang YJ, Li S, Feng SR, Xu HJ, et al. Characterization and application of hydrophobin-dispersed multi-walled carbon nanotubes. Carbon (2010) 48(10):2890–8. doi:10.1016/j.carbon.2010.04.022
50. Bilewicz R, Witomski J, Van der Heyden A, Tagu D, Palin B, Rogalska E. Modification of electrodes with self-assembled hydrophobin layers. J Phys Chem B (2001) 105(40):9772–7. doi:10.1021/jp0113782
51. Gravagnuolo AM, Morales-Narváez E, Matos CRS, Longobardi S, Giardina P, Merkoçi A. On-the-Spot immobilization of quantum dots, graphene oxide, and proteins via hydrophobins. Adv Funct Mater (2015) 25(38):6084–92. doi:10.1002/adfm.201502837
52. Alongi J, Carletto RA, Bosco F, Carosio F, Di Blasio A, Cuttica F, et al. Caseins and hydrophobins as novel green flame retardants for cotton fabrics. Polym Degrad Stabil (2014) 99:111–7. doi:10.1016/j.polymdegradstab.2013.11.016
53. Dumitrescu I, Iordache OG, Mocioiu AM, Nicula G. Antimicrobial functionalization of textile materials with hydrophobins and Ag/zno composite nanopowders. Ind Textila (2013) 64(6):303–12.
54. Hektor HJ, Scholtmeijer K. Hydrophobins: proteins with potential. Curr Opin Biotechnol (2005) 16(4):434–9. doi:10.1016/j.copbio.2005.05.004
55. Haas JAM, Post E, Meter-Arkema A, Rink R, Robillard GT, Wang X, et al. Use of hydrophobins in formulation of water insoluble drugs for oral administration. Colloids Surf B Biointerfaces (2010) 75(2):526–31. Epub 20090926. doi:10.1016/j.colsurfb.2009.09.030
56. Misra R, Li J, Cannon GC, Morgan SE. Nanoscale reduction in surface friction of polymer surfaces modified with Sc3 hydrophobin from Schizophyllum commune. Biomacromolecules (2006) 7(5):1463–70. doi:10.1021/bm050983y
57. Palomo JM, Penas MM, Fernandez-Lorente G, Mateo C, Pisabarro AG, Fernandez-Lafuente R, et al. Solid-phase handling of hydrophobins: immobilized hydrophobins as a new tool to study lipases. Biomacromolecules (2003) 4(2):204–10. doi:10.1021/bm020071l
58. Basheva ES, Kralchevsky PA, Christov NC, Danov KD, Stoyanov SD, Blijdenstein TB, et al. Unique properties of bubbles and foam films stabilized by hfbii hydrophobin. Langmuir (2011) 27(6):2382–92. Epub 20110214. doi:10.1021/la104726w
59. Linder MB, Qiao M, Laumen F, Selber K, Hyytia T, Nakari-Setala T, et al. Efficient purification of recombinant proteins using hydrophobins as tags in surfactant-based two-phase systems. Biochemistry (2004) 43(37):11873–82. doi:10.1021/bi0488202
60. Sarparanta MP, Bimbo LM, Makila EM, Salonen JJ, Laaksonen PH, Helariutta AM, et al. The mucoadhesive and gastroretentive properties of hydrophobin-coated porous silicon nanoparticle oral drug delivery systems. Biomaterials (2012) 33(11):3353–62. Epub 20120129. doi:10.1016/j.biomaterials.2012.01.029
Keywords: hydrophobins, fungi, interfacial activity, multitask proteins, applications
Citation: Rojas-Osnaya J, Quintana-Quirino M, Espinosa-Valencia A, Bravo AL and Nájera H (2024) Hydrophobins: multitask proteins. Front. Phys. 12:1393340. doi: 10.3389/fphy.2024.1393340
Received: 28 February 2024; Accepted: 12 April 2024;
Published: 06 May 2024.
Edited by:
José G. Sampedro, Autonomous University of San Luis Potosí, MexicoReviewed by:
Jesus Perez-Gil, Complutense University of Madrid, SpainCopyright © 2024 Rojas-Osnaya, Quintana-Quirino, Espinosa-Valencia, Bravo and Nájera. This is an open-access article distributed under the terms of the Creative Commons Attribution License (CC BY). The use, distribution or reproduction in other forums is permitted, provided the original author(s) and the copyright owner(s) are credited and that the original publication in this journal is cited, in accordance with accepted academic practice. No use, distribution or reproduction is permitted which does not comply with these terms.
*Correspondence: Hugo Nájera, aG5hamVyYUBjdWEudWFtLm14
Disclaimer: All claims expressed in this article are solely those of the authors and do not necessarily represent those of their affiliated organizations, or those of the publisher, the editors and the reviewers. Any product that may be evaluated in this article or claim that may be made by its manufacturer is not guaranteed or endorsed by the publisher.
Research integrity at Frontiers
Learn more about the work of our research integrity team to safeguard the quality of each article we publish.