- 1Gran Sasso Science Institute, L’Aquila, Italy
- 2INFN Laboratori Nazionali del Gran Sasso, Assergi (AQ), Italy
- 3Savannah River National Laboratory, Jackson, SC, United States
- 4INFN Cagliari, Cagliari, Italy
- 5Department of Physics, Carleton University, Ottawa, ON, Canada
- 6INFN Napoli, Napoli, Italy
- 7Centro de Astropartículas y Física de Altas Energías (CAPA), Universidad de Zaragoza, Zaragoza, Spain
- 8Centro de Investigaciones Energeticas, Medioambiéntales y Tecnologicas (CIEMAT), Madrid, Spain
- 9APC, Université de Paris Cité, CNRS, Astroparticule et Cosmologie, Paris, France
- 10Physics Department, Università degli Studi di Cagliari, Cagliari, Italy
- 11Physics Department, Princeton University, Princeton, NJ, United States
- 12Institute for Particle Physics, ETH Zürich, Zürich, Switzerland
- 13Department of Industrial Engineering, Information Engineering, and Economics, Università degli Studi dell’Aquila, L’Aquila, Italy
- 14Department of Physics, University of Houston, Houston, TX, United States
- 15Chemical, Materials, and Industrial Production Engineering Department, Università degli Studi “Federico II” di Napoli, Napoli, Italy
- 16Department of Electrical and Electronic Engineering, Università degli Studi di Cagliari, Cagliari, Italy
The existence of dark matter in the universe is inferred from abundant astrophysical and cosmological observations. The Global Argon Dark Matter Collaboration (GADMC) aims to perform the searches for dark matter in the form of weakly interacting massive particles (WIMPs), whose collisions with argon nuclei would produce nuclear recoils with tens of keV energy. Argon has been considered an excellent medium for the direct detection of WIMPs as argon-based scintillation detectors can make use of pulse shape discrimination (PSD) to separate WIMP-induced nuclear recoil signals from electron recoil backgrounds with extremely high efficiency. However, argon-based direct dark matter searches must confront the presence of intrinsic 39Ar as the predominant source of electron recoil backgrounds (it is a beta-emitter with an endpoint energy of 565 keV and half-life of 269 years). Even with PSD, the 39Ar activity in atmospheric argon (AAr), mainly produced and maintained by cosmic ray-induced nuclear reactions, limits the ultimate size of argon-based detectors and restricts their ability to probe very-low-energy events. The discovery of argon from deep underground wells with significantly less 39Ar than that in AAr was an important step in the development of direct dark matter detection experiments using argon as the active target. Thanks to pioneering research and successful R&D, in 2012, the first 160 kg batch of underground argon (UAr) was extracted from a CO2 well in Cortez, Colorado. The DarkSide-50 experiment at the Gran Sasso National Laboratory (LNGS) in Italy, the first liquid argon detector ever operated with a UAr target, demonstrated a ∼ 1,400 suppression of the 39Ar activity with respect to the atmospheric argon. An even larger suppression is expected for 42Ar (another intrinsic beta-emitter with the 42K daughter isotope, also a beta-emitter) as its production is expected mainly in the upper atmosphere. Following the results of DarkSide-50, the GADMC initiated the UAr project for extraction from underground and cryogenic purification of 100 t of argon to be used as a target in the next-generation experiment DarkSide-20k. This paper contains a description of the Urania Plant in Cortez, Colorado, where UAr is extracted; the Aria Plant in Sardinia, Italy, an industrial-scale plant comprising a 350-m state-of-the-art cryogenic isotopic distillation column, designed for further purification of the extracted argon and further reduction of the isotopic abundance of 39Ar; and DArT, a facility for UAr radiopurity qualification at the Canfranc Underground Laboratory (LSC), Spain. Moreover, the high radiopurity of UAr leads to other possible applications, for instance, for those neutrinoless double-beta decay experiments using argon as shielding material or, more generally, for all those activities on argon-based detectors in high-energy physics or nuclear physics, which will be briefly discussed.
1 Introduction
Liquefied noble gases have been employed in rare event searches since more than 3 decades, due to their good scintillation and ionization yields, chemical stability, and good intrinsic radiopurity. Argon offers excellent particle identification capability thanks to pulse shape discrimination (PSD), which makes it an ideal candidate for searching for rare nuclear recoil processes, such as dark matter orcoherent neutrino–nucleus interactions [1].
Several experiments are currently underway [2,3] or will employ a liquid argon target in the future [4–6]. The relative abundance of argon in the Earth atmosphere (0.94%) makes the extraction of this gas from air convenient. Although argon derived from the atmosphere is predominated by 40Ar, it contains the cosmogenically produced long-lived radioactive isotopes 39Ar and 42Ar. These radioactive contaminants are of little concern for searches at the GeV scale but may represent a limiting background for experiments aiming at discovering low-energy processes such as those expected from the weakly interacting massive particle (WIMP)–nuclei interaction. These experiments are typically operated in the dual-phase configuration, in which the liquid bulk is immersed in a uniform electric drift field (200 ÷ 500 V/cm) to extract ionization electrons from the interaction sites. These electrons are collected in the gaseous phase at the anode and provide, in combination with prompt scintillation, 3D vertex reconstruction and extremely low thresholds [7,8]. The reconstruction of one full event becomes possible at the cost of long acquisition time, corresponding to the maximum drift time along the drift direction (the drift velocity is
Argon extracted from the atmosphere contains approximately 1 part in 1015 of 39Ar, giving a specific activity of approximately 1 Bq/kg [10,11], whereas the β-decay of 42Ar (as its daughter 42K in secular equilibrium) gives approximately four orders of magnitude less specific activity than 39Ar [12]. Both the direct background and pileup from 39Ar decays set limits on the sensitivity and maximum practical size of liquid argon dark matter searches in the case of single-phase experiments because of the background rate and also in the case of dual-phase experiments because of pileup. A source of argon with a reduced 39Ar content is necessary to allow sensitive argon-based dark matter searches at the ton scale and beyond. Moreover 42Ar and, in particular, its progeny 42K is a dominant background in neutrinoless double-beta decay experiments using argon as shielding, such as GERDA [13] and Large Enriched Germanium Experiment for Neutrinoless Double-Beta Decay (LEGEND) [14].
Because of the long half-life of 39Ar (half-life 269 ± 9 years, [2]), established methods to reduce the amount of 39Ar in atmospheric argon (AAr) are centrifugation and differential thermal diffusion together with isotope separation, some rather costly and time-consuming processes. Alternatively, considering that much of the 40Ar in the atmosphere is produced by electron capture decays of long-lived 40K within the Earth and much of the 40Ar remains underground, it is expected that argon from underground sources would have less 39Ar radioactivity since cosmic rays, producing 39Ar in the upper atmosphere principally via the 40Ar(n,2n)39Ar reaction, are effectively attenuated by rocks. Nevertheless, α-decays in the decay chains of long-lived natural uranium and thorium can lead to in situ production of 39Ar, meaning that not all underground argon samples from different underground gas wells show reduced 39Ar-specific activity compared to AAr [15]. As the concentration of uranium and thorium in the mantle is typically of the order of a thousand times lower than that in the crust, it was also suggested that the concentration of 39Ar in argon gas from the Earth’s mantle should have to be lower [16]. That possibility pushed the DarkSide collaboration to perform pioneering research and R&D, leading to the discovery of less 39Ar radioactivity argon in the National Helium Reserve in Amarillo, Texas, in 2007; in that case, the 39Ar concentration was measured with a factor of at least 20 below that of AAr [17]. Afterward, argon with low 39Ar radioactivity was found in gas samples from the Reliant Dry Ice Plant in Bueyeros, New Mexico, and from a CO2 well in Cortez, southwestern Colorado (CO), for which preliminary measurements studies showed a promising upper limit of 39Ar concentration of less than 0.65% of the 39Ar concentration in AAr [18]. This was an important step in the development of the next-generation direct dark matter detection experiments using underground argon (UAr) as the active target.
The first use of low-radioactivity argon in a dark matter search is pioneered by the DarkSide-50 experiment; after an initial use of AAr as target material, the DarkSide-50 detector was filled with UAr from the Cortez CO2 gas field source in April 2015. DarkSide-50 ultimately showed that the 39Ar activity in the Cortez UAr is reduced by a factor of (1.4 ± 0.2) × 103 relative to AAr; that is, an 39Ar activity of 0.73 mBq/kg was measured [19]. Figure 1 shows the comparison of the DarkSide-50 data collected with AAr and UAr. The energy spectra extend to above 2.6 MeV. Below approximately 570 keV, the AAr spectrum is dominated by 39Ar, clearly suppressed in the UAr dataset. A spectral analysis using Monte Carlo-generated spectra to describe the background components was performed, in order to measure the 39Ar activity. This analysis revealed the presence of 85Kr in the UAr spectrum, which was unexpected; this was interpreted as the result of a possible air leak during that UAr batch extraction, leading to the possibility to obtain even higher depletion factors in next UAr batches.
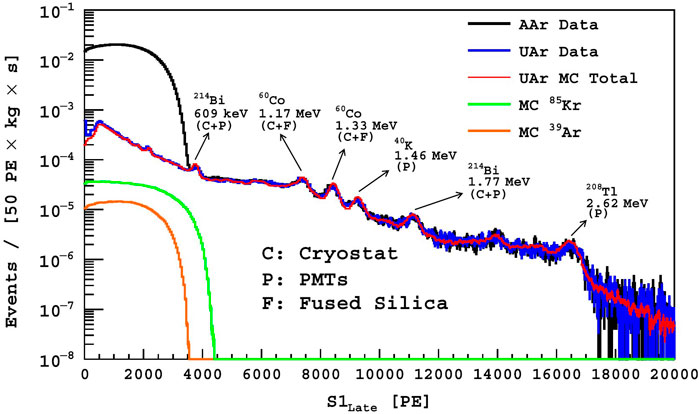
Figure 1. Figure obtained from [19]: spectra for the UAr (blue) and AAr (black) targets, normalized to exposure. Also shown are the Monte Carlo (MC) fit to the UAr data (red) and individual components of 85Kr (green) and 39Ar (orange) extracted from the fit. The presence of 85Kr on the UAr spectrum was unexpected.
It is worth reporting that due to the fact that the dominant production channel of 42Ar is ultimately through interactions of alpha particles on 40Ar via 40Ar(α,2p)42Ar with an energy threshold of approximately 14 MeV (not available from radioisotopic decays rather in the upper atmosphere where primary cosmic rays are “harder”), it is expected that the underground production of 42Ar is strongly suppressed [20,21].
The Global Argon Dark Matter Collaboration (GADMC) aims at performing the most sensitive WIMP dark matter search of the next decade with the DarkSide-20k experiment, using the low-radioactivity UAr as the target from the Cortez CO2 gas field [4]. A 5-ton active (20-ton fiducial) UAr mass will be hosted in a double-phase time projection chamber (TPC) of dimension 3.5 m wide and 3.5 m high. The TPC will be installed in a steel vessel, in turn operated in a DUNE-like cryostat, currently under construction in Hall C at Laboratori Nazionali del Gran Sasso (LNGS). The UAr filling the vessel, outside the TPC, will be instrumented and used as active veto to suppress the radiogenic neutron background. A total amount of approximately 100 t of UAr will be required in order to operate the full system.
The supply chain begins with the Urania Plant in CO, which can produce UAr at a purity of 99.99% from a CO2 stream sourced from a deep well that reaches the Earth’s mantle at a rate of approximately 330 kg/day. After this initial purification stage, the argon will be transported to Carbosulcis S.L.R. in Sardinia, Italy, where the Aria Plant, based on a 350-m cryogenic distillation column, will further suppress impurities. After processing UAr in the Aria Plant, it will be transported from Aria to LNGS, Abruzzo, Italy. A fraction of the production will be shipped to the Canfranc Underground Laboratory (LSC), Spain, where it will be qualified at the DArT through the ArDM experiment [22].
The remainder of the article is organized as follows: Sections 2, 3, and 4 describe the aforementioned steps. Since the importance of this supply chain and associated techniques extends well beyond DarkSide-20k, Section 5 addresses some possible additional applications.
2 The Urania Plant
The Urania Plant is the gas processing plant built by the Italian company Polaris S.r.l. under the supervision of the GADMC to extract 120 tons of UAr for the DarkSide-20k experiment. It will be installed in Cortez, CO, in a new facility operated by the GADMC and currently under construction. The Urania facility will be located in proximity to a private commercial plant for the extraction of CO2 from underground. Urania will process the CO2-rich stream extracted from deep underground to separate UAr, which is present at a concentration of 400 ppm ca. In addition to CO2 and Ar, the plant input also contains small amounts of nitrogen and methane. The UAr separation process is based on cryogenic distillation and adsorption, as schematized in the block flow diagram in Figure 2. For more details, please refer to [4].
A first group of distillation columns operating at high pressure removes bulk CO2, and full removal of CO2 is achieved in the pressure swing adsorption (PSA) unit operating in loop with one of the distillation columns upstream. The stream leaving the PSA unit is essentially made of nitrogen, methane, and UAr and enters a second group of distillation columns operating at lower pressure, at much lower temperature and located inside a coldbox. The second group of distillation columns separates nitrogen, methane, and UAr and delivers liquid UAr at a nominal rate of 330 kg/day and with a purity better than 99.99% based on the simulation done using Aspen HYSYS® software. With the exception of UAr, all other components are merged and refed to the main plant in full.
The installation of the Urania Plant should begin in mid-2024 in order to enter full operation in 2025. The extraction of UAr needed for DarkSide-20k should be completed by mid-2026. To account for processing losses during the UAr purification phase in Aria, during the filling of the transportation vessels and during shipping, Urania target production quantity for the DarkSide-20k experiment is 120 t.
3 The Aria Plant
The crude UAr from the Urania Plant is shipped to Sardinia, Italy, where it is purified through cryogenic distillation in the so-called Aria Plant. Aria is an industrial-scale plant comprising a 350-m-high cryogenic distillation column, the highest distillation column in the world, and is currently being installed in an underground vertical mine shaft, excavated in 1940, with dimensions 5 m diameter and 350 m deep, at Carbosulcis S.p.A., Nuraxi-Figus (SU), Italy (Figure 3).
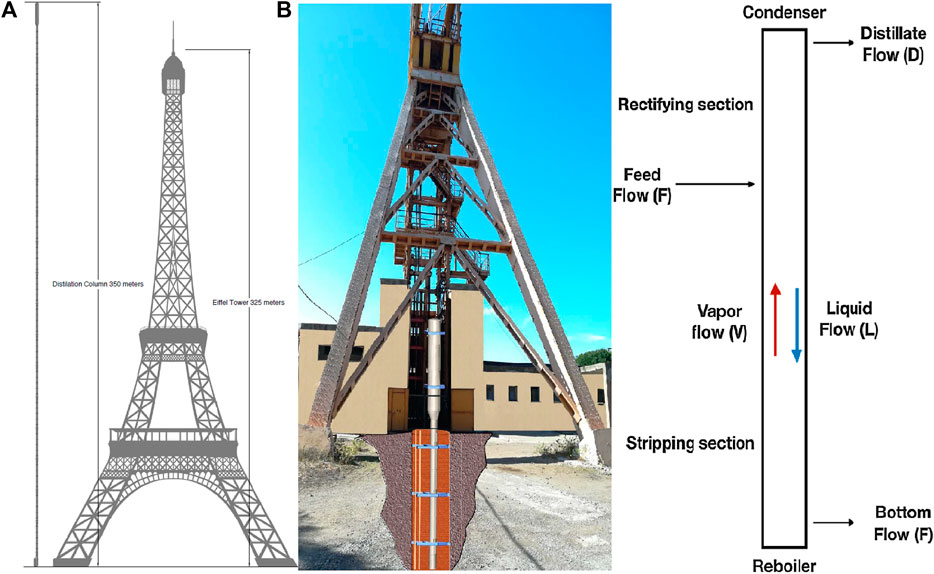
Figure 3. (A) Drawing to scale with the Aria 350-m-high column and the Eiffel Tower. (B) Picture showing some parts (drawn to scale) of the above-ground vertical mine shaft with the higher part of the column emerging from the mine shaft (from http://www.carbosulcis.eu/). (C) Basic operation principles of a continuous distillation column (from obtained [23]).
Aria was designed to be capable of argon isotopic separation. Cryogenic isotopic distillation with rectifying columns is a well-established technique. However, this is the first time that such a plant is being proposed and constructed for argon isotopic distillation.
Isotopic separation by cryogenic distillation exploits the relative volatility of different isotopes, which for ideal mixtures is given by the ratio of the isotope’s vapor pressures at a given temperature. Although the relative volatility of isotopes is close to unity, it allows for the separation of isotopes by continuous distillation using a large number of distillation stages, where the liquid and vapor phases undergo a countercurrent exchange at thermodynamic equilibrium. Aria has been designed to further reduce the concentration of 39Ar by cryogenic distillation in UAr by a factor of 10 per pass and at a rate of approximately 7 kg/day. More information on this, together with a full description of the Aria Plant and the column structure and expected performances, has been provided in [23].
Although the Aria Plant is still in the installation phase, a smaller version of the Aria column, 28 m high, using only the reboiler, the condenser, and one central module, together with all the auxiliary equipment of the full column, installed in a tall surface building has been tested over the last 3 years providing positive results. The first important achievement of this project was an isotopic nitrogen distillation run of the prototype plant [23]. Subsequent to this first achievement, an isotopic separation of argon with the same prototype of the cryogenic distillation plant was achieved. In this case, the column demonstrated the ability to isotopically separate the two stable isotopes, 36Ar and 38Ar, other than 40Ar in AAr, which in AAr, have the non-negligible isotopic abundance of 0.334% and 0.063%, respectively [24], and was considered a milestone for the UAr project. The successful outcome of those runs paved the way to the continuation of the project and the construction of the full plant.
For the DarkSide-20k experiment, the Aria Plant will not be used to reduce 39Ar but rather to chemically purify crude UAr from Urania (with purity already better than 99.99%) to produce detector-grade UAr, i.e., to further reduce nitrogen concentration to 1 ppm, given that from simulations, we can assume that all other impurities are removed by Urania. For this chemical purification, Aria will produce approximately 1,000 kg/day of purified UAr.
Beyond argon isotopic enrichment, the Aria Plant has also commercial applications in the production of isotopes for nuclear energy and medicine.
4 The DArT in the ArDM experiment
The goal of the DArT in the ArDM experiment at LSC is to measure the 39Ar content in batches of UAr delivered by Urania and Aria [22]. DArT in ArDM will be sensitive to very high depletion factors of 39Ar, which is of the order of 1,000, reaching 10% statistical uncertainty in 1 week of data-taking. It will use a single-phase detector, with a total capacity of approximately 1 L (the DArT chamber) filled with samples of UAr. The DArT chamber is a hollow cylinder made of acrylic (PMMA), and it is hosted inside a radiopure copper vessel. The internal surfaces are coated with tetraphenyl butadiene (TPB) wavelength-shifter and the outer surfaces with an enhancer specular reflector. On the top and bottom caps, two
Assuming the 39Ar activity in the UAr as measured by DarkSide-50 (0.73 mBq/kg), the expected number of signal events is approximately 600 per week. Minimizing radiogenic backgrounds is therefore critical for identifying 39Ar signals. In DArT, the internal background generated by the detector’s components is effectively reduced through the measurement of radioactive contamination within the materials and the identification of the radiopure samples used to construct the detector’s components. To mitigate the background produced by gamma rays originating from the surrounding rock, the DArT vessel will be installed inside the active volume of ArDM shielding [25]. A detailed background model of the experiment based on Monte Carlo simulations reveals that the combination of the ArDM passive shielding (50 cm of HDPE + 6 cm of lead shield) and active veto (40 cm of AAr instrumented with 13 × 8″ photomultipliers) is sufficient to achieve an overall background of a few hundreds of untagged events per week in the DArT region of interest. Figures 4A,B show a rendering of the DArT in the ArDM experiment and a picture of the DArT chamber.
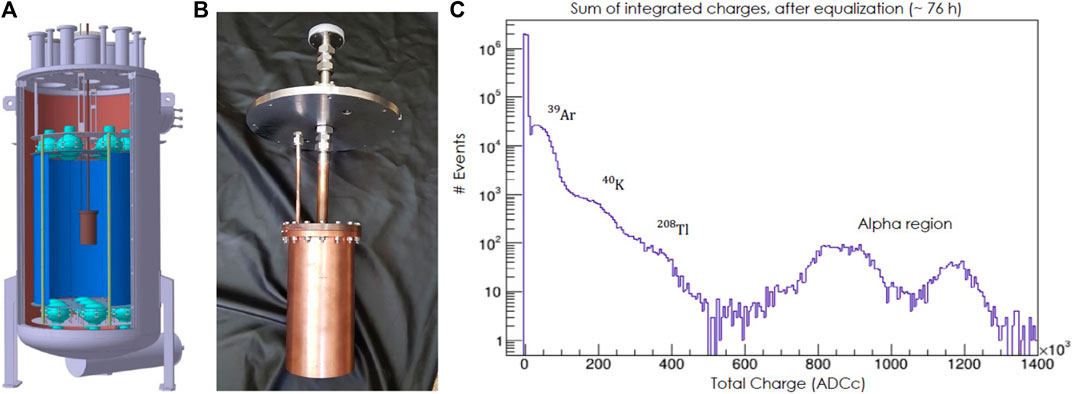
Figure 4. (A) Rendering of the DArT in the ArDM experiment. (B) Picture of the DArT chamber before its installation inside the ArDM detector. (C) Energy spectrum of data collected during a test run underground at LSC.
In order to study the detector’s performance and gain a preliminary understanding of the internal background, the DArT detector has been operating underground in a test setup for several months, concurrently with the refurbishment of the ArDM setup. The operating conditions in this temporary setup were optimized for cooling and maintaining DArT at 85 K, achieved using liquid nitrogen (LN2). Hardware and software parameters for the data acquisition system were optimized; noise was minimized through the use of filters, external insulation on cables and connections, common grounding, and setting trigger threshold conditions for robust continuous operation. The setup was installed within a purpose-built lead castle that was flushed with Rn-free air. A preliminary plot of the data analysis taken underground in the test setup is reported in Figure 4B. It shows the alpha peaks arising from the 222Rn contamination in LAr (eventually identifiable by PSD), along with two bumps consistent with the gamma emissions, resulting from 40K and 208Tl contaminations present in the detector materials. The low-energy spectrum aligns with the 39Ar beta-spectrum. A run with UAr from the DarkSide-50 experiment aimed to evaluate 39Ar activity and is expected to begin in 2024 to validate the designed setup.
5 Beyond the WIMP search
In addition to WIMP dark matter detection, leading experimental searches across particle physics will benefit from the availability of low-radioactivity UAr.
The 39Ar decays represent the dominant intrinsic background source in argon-based experiments searching for coherent elastic neutrino–nucleus scattering. A
The LEGEND-1000 experiment will search for 0νββ-decay of 76Ge in cryogenic Ge detector strings distributed among four 250-kg modules. The modules are immersed in liquid argon serving simultaneously as a radiation shield, coolant, and scintillation detector. Beta decays from 42K (beta-emitter daughter of 42Ar) are a potential intrinsic background in the LEGEND-1000 LAr shield, as reported using the GERDA experiment [13]. The 42Ar depletion of UAr makes it an ideal candidate to suppress this class of backgrounds in the argon that surrounds the germanium crystals, with a consequent improvement in the experiment sensitivity.
A reduction of 42K and 39Ar in DUNE-like detectors would also enable the measurement of low-energy neutrinos [6]. 42K and 39Ar are currently one of the largest expected backgrounds at low energy, impacting the energy resolution for ≲ 10 MeV events and representing a challenge from the trigger strategy and event classification. Although the procurement of multi-kiloton target of UAr is currently an open challenge, its availability would enlarge the physics reach of a DUNE-like detector to improve, among the others, the sensitivity to supernova bursts.
Furthermore, a dedicated ton-scale detector optimized for the collection of ∼ keV ionization signals from low-mass dark matter candidates could benefit from lower-energy thresholds [26].
The potential needs for UAr, motivated by disparate scientific goals as those described above, span from tens of kilograms (i.e., for the COHERENT experiments) and tens of tons (i.e., for LEGEND-1000) to tens of kilotons (i.e., for a DUNE-like modules), across several orders of magnitude in the reduction of long-lived argon radioisotope concentration.
6 Conclusion
The ability to extract argon from deep underground was demonstrated for the first time using the DarkSide-50 experiment, which measured an 39Ar depletion factor of (1.4 ± 0.2) × 103 compared to the argon found in the atmosphere. The discovery of UAr with reduced 39Ar content represents a major breakthrough for the argon-based technology and broadens the physics reach of argon detectors in high energy and nuclear physics.
The GADMC aims at performing the most sensitive search for WIMP dark matter in the next decade using the DarkSide-20k detector at LNGS. One of the requirements to meet this ambitious goal is the extraction of 120 t of low-radioactivity argon from deep underground. The supply chain for extraction, purification, and quality check of the target for DarkSide-20k includes the Urania (Cortez, CO), Aria (Sardinia, Italy), and DArT in ArDM (LSC, Spain) facilities, currently being constructed or commissioned.
The scaling up of these facilities is under consideration to meet the increased demand for low-radioactivity argon beyond the DarkSide-20k WIMP search and toward Argo, the next-generation UAr multi-hundred-ton dark matter detector, aiming to reach sensitivity beyond the neutrino floor with a 3,000 t-yr exposure run [27].
Data availability statement
The original contributions presented in the study are included in the article/Supplementary Material; further inquiries can be directed to the corresponding author.
Author contributions
PA: writing–original draft. HB: writing–original draft, writing–review and editing. WB: writing–review and editing. MB: writing–review and editing. NC: writing–review and editing. MC: writing–original draft. SC: writing–review and editing. VC: writing–review and editing. DM: writing–review and editing. DF: writing–review and editing. FG: writing–original draft, writing–review and editing. DG: writing–review and editing. CG: writing–review and editing. PG: writing–review and editing. AG: writing–review and editing. TH: writing–review and editing. SH: writing–review and editing. RL: writing–review and editing. LL: writing–review and editing. MM: writing–review and editing. VP: writing–review and editing. MR: writing–original draft, writing–review and editing. ALR: writing–review and editing. LR: writing–review and editing. ARu: writing–review and editing. RSa: writing–review and editing. MS: writing–review and editing. RSt: writing–review and editing. AS: writing–review and editing. SS: writing–review and editing.
Funding
The author(s) declare that financial support was received for the research, authorship, and/or publication of this article. This research is part supported by the Spanish Ministry of Science and Innovation (MICINN) through the grant PID2022-138357NB-C22.
Conflict of interest
The authors declare that the research was conducted in the absence of any commercial or financial relationships that could be construed as a potential conflict of interest.
Publisher’s note
All claims expressed in this article are solely those of the authors and do not necessarily represent those of their affiliated organizations, or those of the publisher, the editors, and the reviewers. Any product that may be evaluated in this article, or claim that may be made by its manufacturer, is not guaranteed or endorsed by the publisher.
References
1. Adhikari P, Ajaj R, Araujo GR, Batygov M, Beltran B, Bina CE, et al. The liquid-argon scintillation pulseshape in deap-3600. The Eur Phys J C (2020) 80:303. doi:10.1140/epjc/s10052-020-7789-x
2. Adhikari P, Ajaj R, Alpízar-Venegas M, Amaudruz P-A, Anstey J, Araujo GR, et al. Precision measurement of the specific activity of 39Ar in atmospheric argon with the deap-3600 detector. Eur Phys J C (2023) 83:642. doi:10.1140/epjc/s10052-023-11678-6
3. Abratenko P, Andrade Aldana D, Anthony J, Arellano L, Asaadi J, Ashkenazi A, et al. First constraints on light sterile neutrino oscillations from combined appearance and disappearance searches with the microboone detector. Phys Rev Lett (2023) 130:011801. doi:10.1103/PhysRevLett.130.011801
4. Aalseth CE, Acerbi F, Agnes P, Albuquerque IFM, Alexander T, Alici A, et al. Darkside-20k: a 20 tonne two-phase lar tpc for direct dark matter detection at lngs. The Eur Phys J Plus (2018) 133:131. doi:10.1140/epjp/i2018-11973-4
5. Akimov D, Albert JB, An P, Awe C, Barbeau PS, Becker B, et al. First measurement of coherent elastic neutrino-nucleus scattering on argon. Phys Rev Lett (2021) 126:012002. doi:10.1103/PhysRevLett.126.012002
6. Abi B, Acciarri R, Acero M, Adamov G, Adams D, Adinolfi M, et al. Volume i. introduction to dune. J Instrumentation (2020) 15:T08008. doi:10.1088/1748-0221/15/08/T08008
7. Agnes P, Albuquerque IFM, Alexander T, Alton AK, Araujo GR, Asner DM, et al. Low-mass dark matter search with the darkside-50 experiment. Phys Rev Lett (2018) 121:081307. doi:10.1103/PhysRevLett.121.081307
8. Agnes P, Albuquerque IFM, Alexander T, Alton AK, Ave M, Back HO, et al. Search for low mass dark matter in darkside-50: the bayesian network approach. Eur Phys J C (2023) 83:322. doi:10.1140/epjc/s10052-023-11410-4
9. Agnes P, Albuquerque IFM, Alexander T, Alton AK, Araujo GR, Ave M, et al. Darkside-50 532-day dark matter search with low-radioactivity argon. Phys Rev D (2018) 98:102006. doi:10.1103/PhysRevD.98.102006
10. Loosli H. A dating method with39ar. Earth Planet Sci Lett (1983) 63:51–62. doi:10.1016/0012-821X(83)90021-3
11. Benetti P, Calaprice F, Calligarich E, Cambiaghi M, Carbonara F, Cavanna F, et al. Measurement of the specific activity of 39ar in natural argon. Nucl Instr Methods Phys Res Section A: Acc Spectrometers, Detectors Associated Equipment (2007) 574:83–8. doi:10.1016/j.nima.2007.01.106
12. Ajaj R, Araujo GR, Batygov M, Beltran B, Bina CE, Boulay MG, et al. Electromagnetic backgrounds and potassium-42 activity in the deap-3600 dark matter detector. Phys Rev D (2019) 100:072009. doi:10.1103/PhysRevD.100.072009
13. Lubashevskiy A, Agostini M, Budjáš D, Gangapshev A, Gusev K, Heisel M, et al. Mitigation of 42Ar/42K background for the gerda phase ii experiment. Eur Phys J C (2018) 78:15. doi:10.1140/epjc/s10052-017-5499-9
14. Abgrall N, Abt I, Agostini M, Alexander A, Andreoiu C, Araujo GR, et al. LEGEND-1000 preconceptual design report. arXiv (2021). e-prints, arXiv:2107.11462. doi:10.48550/arXiv.2107.11462
15. Loosli H, Lehmann B, Balderer W. Argon-39, argon-37 and krypton-85 isotopes in stripa groundwaters. Geochimica et Cosmochimica Acta (1989) 53:1825–9. doi:10.1016/0016-7037(89)90303-7
16. Šrámek O, Stevens L, McDonough WF, Mukhopadhyay S, Peterson R. Subterranean production of neutrons, 39ar and 21ne: rates and uncertainties. Geochimica et Cosmochimica Acta (2017) 196:370–87. doi:10.1016/j.gca.2016.09.040
17. Galbiati C, Acosta-Kane D, Acciarri R, Amaize O, Antonello M, Baibussinov B, et al. Discovery of underground argon with a low level of radioactive39Ar and possible applications to WIMP dark matter detectors. J Phys Conf Ser (2008) 120:042015. doi:10.1088/1742-6596/120/4/042015
18. Back HO, Calaprice F, Condon C, de Haas E, Ford R, Galbiati C, et al. First large scale production of low radioactivity argon from underground sources (2012).
19. Agnes P, Agostino L, Albuquerque IFM, Alexander T, Alton AK, Arisaka K, et al. Results from the first use of low radioactivity argon in a dark matter search. Phys Rev D (2016) 93:081101. doi:10.1103/PhysRevD.93.081101
20. Zhang C, Mei D-M. Evaluation of cosmogenic production of 39Ar and 42Ar for rare-event physics using underground argon. Astroparticle Phys (2022) 142:102733. doi:10.1016/j.astropartphys.2022.102733
21. Poudel SS, Loer B, Saldanha R, Hackett BR, Back HO. Subsurface cosmogenic and radiogenic production of 42Ar (2023). doi:10.48550/arXiv.2309.16169
22. Aalseth C, Abdelhakim S, Acerbi F, Agnes P, Ajaj R, Albuquerque I, et al. Design and construction of a new detector to measure ultra-low radioactive-isotope contamination of argon. J Instrumentation (2020) 15:P02024. doi:10.1088/1748-0221/15/02/P02024
23. Agnes P, Albergo S, Albuquerque IFM, Alexander T, Alici A, Alton AK, et al. Separating 39Ar 40Ar by cryogenic distillation with aria for dark-matter searches. Eur Phys J C (2021) 81:359. doi:10.1140/epjc/s10052-021-09121-9
24. Agnes P, Ahmad I, Albergo S, Albuquerque IFM, Alexander T, et al. Measurement of isotopic separation of argon with the prototype of the cryogenic distillation plant aria for dark matter searches. Eur Phys J C (2023) 83:453. doi:10.1140/epjc/s10052-023-11430-0
25. Calvo J, Cantini C, Crivelli P, Daniel M, Luise SD, Gendotti A, et al. Commissioning of the ardm experiment at the canfranc underground laboratory: first steps towards a tonne-scale liquid argon time projection chamber for dark matter searches. J Cosmology Astroparticle Phys (2017) 2017:003. doi:10.1088/1475-7516/2017/03/003
26. Agnes P, Ahmad I, Albergo S, Albuquerque IFM, Alexander T, Alton AK, et al. Sensitivity projections for a dual-phase argon tpc optimized for light dark matter searches through the ionization channel. Phys Rev D (2023) 107:112006. doi:10.1103/PhysRevD.107.112006
Keywords: underground argon, 39Ar, 42Ar, dark matter instrumentation, 0νββ-decay instrumentation, low-radioactivity technique, low-background counting
Citation: Agnes P, Back HO, Bonivento W, Boulay MG, Canci N, Caravati M, Cebrian S, Cocco V, Diaz Mairena D, Franco D, Gabriele F, Gahan D, Galbiati C, Garcia Abia P, Gendotti A, Hessel T, Horikawa S, Lopez Manzano R, Luzzi L, Martinez M, Pesudo V, Razeti M, Renshaw AL, Romero L, Rubbia A, Santorelli R, Simeone M, Stefanizzi R, Steri A and Sulis S (2024) The underground argon project: procurement and purification of argon for dark matter searches and beyond. Front. Phys. 12:1387069. doi: 10.3389/fphy.2024.1387069
Received: 16 February 2024; Accepted: 09 April 2024;
Published: 05 December 2024.
Edited by:
Jeter Hall, SNOLAB, CanadaReviewed by:
Olga Gileva, Institute for Basic Science (IBS), Republic of KoreaMichel Sorel, Spanish National Research Council (CSIC), Spain
Copyright © 2024 Agnes, Back, Bonivento, Boulay, Canci, Caravati, Cebrian, Cocco, Diaz Mairena, Franco, Gabriele, Gahan, Galbiati, Garcia Abia, Gendotti, Hessel, Horikawa, Lopez Manzano, Luzzi, Martinez, Pesudo, Razeti, Renshaw, Romero, Rubbia, Santorelli, Simeone, Stefanizzi, Steri and Sulis. This is an open-access article distributed under the terms of the Creative Commons Attribution License (CC BY). The use, distribution or reproduction in other forums is permitted, provided the original author(s) and the copyright owner(s) are credited and that the original publication in this journal is cited, in accordance with accepted academic practice. No use, distribution or reproduction is permitted which does not comply with these terms.
*Correspondence: M. Caravati, bWF1cm8uY2FyYXZhdGlAZ3NzaS5pdA==