- 1Center for Advanced Laser Technology, Hebei University of Technology, Tianjin, China
- 2Hebei Key Laboratory of Advanced Laser Technology and Equipment, Tianjin, China
- 3Hebei Thahoo Photoelectric Technology Co. Ltd., Shijiazhuang, China
- 4Department of Vehicle and Electrical Engineering, Shijiazhuang Branch, Army Engineering University of PLA, Shijiazhuang, China
Lasers operating around 2-µm have been extensively researched for various applications, such as LiDAR, remote sensing, optical communication, and medicine. One of the approaches to generate 2-µm emission is to employ an optical parametric oscillator pumped by 1-µm laser; however, improving the 2-µm laser conversion efficiency of optical parametric oscillators is still a challenge. In this study, we exploit the high nonlinear coefficient of the KTP crystal at the 2-µm band to realize a high-efficiency optical parametric oscillator. The OPO is driven by a laser diode (LD) side-pumped, electro-optic Q-modulated Nd:YAG laser. The cavity was designed and optimized based on mode matching to increase the peak power density of the pump laser. Different powers were obtained for different pump laser focusing conditions, and the maximum power was obtained when the KTP crystal was placed in front of the pump laser focus. The output characteristics of the KTP at different phase-matching angles were also investigated. An output power of 2.44 W and an output pulse width of 9.04 ns were obtained at 2154.4 nm with an optical-to-optical conversion efficiency of 23.39% and a slope efficiency of 36.09%.
1 Introduction
The 2-μm laser owing to its wavelength in the high absorption band of water and atmospheric transmission window, finds various applications in material processing [1, 2], medical procedures [3, 4], and LiDAR [5–7]. It can also be used as the primary pump source for generating 3–5 μm laser optical parametric oscillators (OPOs) [8]. The main types of lasers generating 2-μm output include Ho3+ and Tm3+ doped solid-state lasers [9], fiber lasers, OPOs, Raman lasers, and waveguide lasers. The solid-state lasers produce 2-µm emission using 1.9-µm fiber laser or diode laser pump source, which is expensive. For fiber lasers, it is difficult to achieve high-energy operations. In contrast, OPOs pumped by well-developed 1-µm lasers have high conversion efficiency and wide tunable range in short pulses [10]. However, improving the 2-µm laser conversion efficiency of OPO is still a challenge.
In recent years, scholars have conducted several studies on OPO to generate 2-μm laser to improve conversion efficiency [11–17]. In 2013, Haiyong Zhu et al. achieved a 2-μm laser output of 1.01 W with an optical-to-optical conversion efficiency of 10.3% using a single KTA crystal [18]. Mei et al. investigated PPLN crystals with large nonlinear coefficients and achieved an optical-to-optical conversion efficiency of 18.5%, with a maximum power of 3.41 W [19]. In 2020, Da et al. investigated internal cavity OPOs to increase the peak power density in the cavity. The power of 4.7 W with a pulse duration of 8.55 ns and a conversion efficiency of 11.6% was achieved [20]. In 2021, Zhao et al. studied the pumping of a novel Nd: MgO: PPLN to achieve 2.1-μm output. A 1.21 W of 2.1-µm with a pulse width of 7.8 ns and a conversion efficiency of 15.1% was achieved [21]. Whether it is selecting a nonlinear crystal with superior performance or changing the structure of the OPO, the conversion efficiency of 2.1-µm OPOs needs further improvement.
In this study, the KTP crystal was placed in front of the focal point of the pump laser so that the pump laser reflected from the OPO output mirror could be focused on the KTP crystal, which further increased the conversion efficiency. Finally, a 2.44 W laser was obtained with a conversion efficiency of 36.09%.
2 Experimental setup and measurements
In this study, a pulse-pumped high-efficiency OPO based on nonlinear optics is presented. As shown in Figure 1, the front part is the pump source of the OPO and the rear part is the OPO. In the experiment, The pump source for the OPO was a Nd: YAG solid-state laser. The Nd: YAG solid-state laser was pumped by an integrated Laser Diode (LD, Oriental OLM 22406), which had an output wavelength of 808 nm at 25°C. A simple linear cavity comprising of two flat mirrors was used in the Nd: YAG system. One has high reflectivity at a wavelength of 1,064 nm (R > 99.5% @1064 nm), and the other is the output coupler (OC), which has partial transmission at 1,064 nm (T = 80% @1064 nm). The 0.5 at% doped Nd: YAG crystal has dimensions of 3 × 3 × 60 mm3. Both end faces of the Nd: YAG are antireflective to the laser beam. The BBO crystal was 3 × 3 × 25 mm3 in size and coated with a 1064 high-transmittance coating at both cross-sections of the crystals, which was used as an electro-optic Q-switch.

FIGURE 1. Experimental setup for 2 μm generation pumped by a Nd: YAG laser. The Nd: YAG laser was designed for the pumping of KTP crystal. OC: output coupler.
As shown in Figure 1, the optical isolator was located in front of the OPO cavity to prevent the 1,064 nm return light from damaging the device. To make the nonlinear conversion in the OPO cavity more efficient, a half-wave plate was placed behind the isolator to change the polarization direction of the 1,064 nm light. A focusing lens was placed behind the half-wave plate. The OPO is a simple linear cavity consisting of two cavity mirrors and a KTP crystal. In the configuration, the one cavity mirror has high transmission at the pump laser wavelength and high reflectivity at oscillating light (T > 95% @1064 nm; R > 99.5% @2128 nm), the other is the OC, with high reflectivity at the fundamental laser wavelength and partial transmission at oscillating light (R > 99.5% @1064 nm; T = 25% ± 2% @2128 nm). The size of the KTP crystal is 5 × 5 × 15 mm3 and is cut along the x-axis (θ = 51.5°,ϕ = 0°). The films with high-transmittance at 1,064 nm and 2,128 nm wavelengths were coated on the KTP crystals. The crystal was wrapped in an indium foil and clamped to a copper heat sink. To increase the nonlinear conversion efficiency, the OPO cavity was placed in front of the fundamental laser focus, the fundamental laser beam reflected from the OC of the OPO was focused on the KTP crystal.
The energies of 1-μm and 2-μm lasers were measured by an energy meter (Vega Pyroelectric PE50DIF-ER (s/n. 917,609), Ophir Optronics, Israel), and the pulse widths were measured using a photodetector (LSIPD-UL0.3, Light Sensing).
3 Experimental results and discussion
3.1 The Nd: YAG laser system results
As shown in Figure 2A, when the output energy was 12.4 mJ with a LD current of 85 A, the pulse width was 9.4 ns. The laser spot shape, pulse width, and bandwidth for the LD current of 82 A are shown in Figure 2B. In addition, the 1,064 nm laser exhibited a high beam quality of 1.32, close to the fundamental transverse mode, and can be used to pump OPO with higher conversion efficiencies.
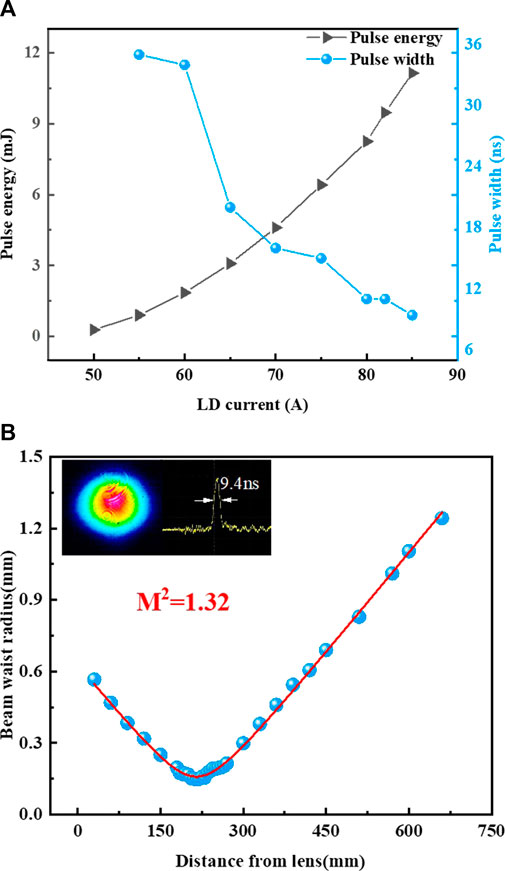
FIGURE 2. (A) The output energy and pulse width of the Nd: YAG laser. (B) The output spot shape, pulse width, and M2 for the LD current of 85 (A)
3.2 The OPO system results
We alter the beam waist radius of the pump light by incorporating convex lenses of varying focal lengths in front of the OPO cavity. The powers at different beam radius of the pump laser are shown in Figure 3. The maximum power was 2.44 W, with an optical-to-optical conversion efficiency of 23.39% and a slope efficiency of 36.09%. As shown in Figure 3A, we first focused the pump laser at the center of the KTP and tested the 2-μm power at different waist radius, with a higher conversion efficiency at a beam radius of 0.47 mm, but reached the damage threshold of the cavity mirror coating rapidly. The conversion efficiency is significantly lower at a waist radius of 0.54 mm. To maintain high conversion efficiency and avoid damage to the coating of the cavity mirror, we placed the OPO in front of the focal point of the pump laser. As shown in Figure 3B, the waist radius of the pump laser was 0.40 mm, and the power was measured by placing the OPO at different beam radii of the pump. At low pumping power, the conversion efficiency at a beam radius of 0.47 mm is higher than that at a beam radius of 0.54 mm, whereas at high pumping power, the conversion efficiency at latter is higher. The reason for this phenomenon may be that the coupling efficiency of the pump laser and oscillating light was higher when the beam radius was larger.
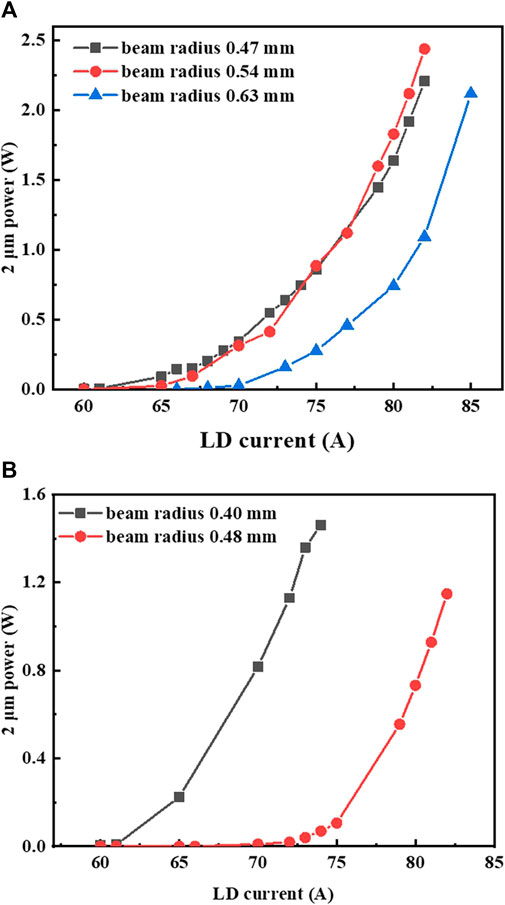
FIGURE 3. 2-µm power at different beam radius of pump: (A) pump laser is focused on the center of the KTP and (B) pump laser is not focused on the center of the KTP.
The temporal behavior and spectral characteristics of the output were also investigated. Different wavelengths of the output laser were obtained by adjusting the angle of the KTP crystal. When the KTP crystal was adjusted to degenerate, the maximum output power was 1.5 W, pulse width was 8.3 ns, the output center wavelength was 2127.4 nm, and the spectral width was 2.7 nm, as shown in Figures 4A, B. Adjusting the angle of the KTP crystal to an output wavelength of 2,154 nm gives a maximum output power of 2.44 W, pulse width of 9 ns and spectral width of 2.6 nm, as shown in Figures 5A, B. We observed a wider spectral width and lower power at the degeneracy, which may have been due to a larger phase mismatch. The higher the output energy, the longer the pulse width of the output. This is most likely because smaller losses allow the light to oscillate for a longer period. Another experimental phenomenon is involved in this argument.
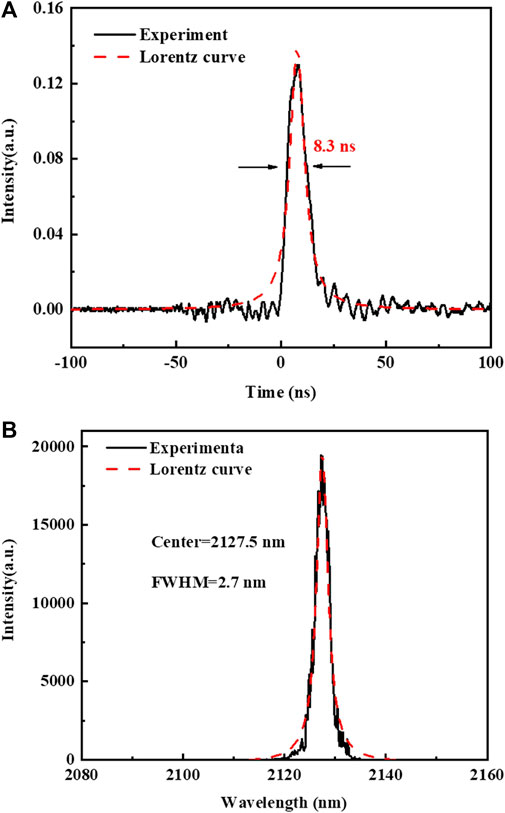
FIGURE 4. Obtained characteristic curves of a 2-μm laser in the time and frequency domains: (A) pulse width and (B) linewidth at 2127.4 nm.
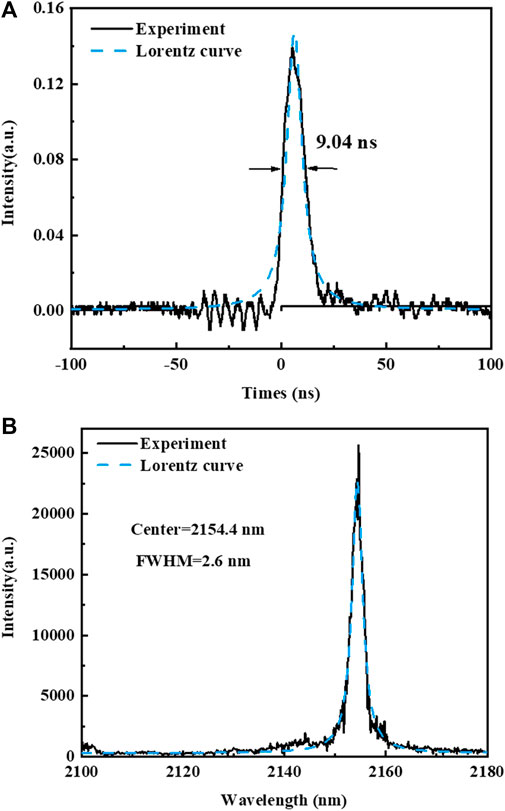
FIGURE 5. Obtained characteristic curves of a 2-μm laser in the time and frequency domains: (A) pulse width and (B) linewidth at 2154.4 nm.
We investigated the relationship between the pump and output pulse widths at different KTP phase-matching angles. As shown in Figure 6, as the pump pulse width decreases, the pulse of the output light increases at the output wavelength of 2127.4 nm. This is because when the pump energy is small, the nonlinear conversion efficiency is low; a longer time is required to generate oscillating light, which shortens the pulse time of the oscillating light. However, as the pump energy increased, the output pulse width stabilized at approximately 9 ns. The pump pulse width is 11 ns, which means that owing to the very small loss, the oscillation time of the oscillating light in the cavity is greatly improved, and the output pulse width is close to the pump pulse width.
4 Conclusion
In conclusion, we developed an efficient and high-power OPO for 2-μm wavelength generation. By placing the OPO cavity in the Rayleigh region in front of the focal point of the pump laser, a maximum slope efficiency of 36.09%, and a conversion efficiency of 23.39% were obtained. The relationship between the pulse width and the pump power at different output wavelengths was investigated, with an output power of 1.5 W and a minimum output pulse width of 8.3 ns at an output wavelength of 2,127 nm. An output power of 2.44 W and an output pulse width of 9.04 ns at an output wavelength of 2,154 nm were realized: the higher the OPO intracavity gain, the closer the output pulse width was to the pump pulse width. The developed OPO system can be used in LiDAR, medicine, and other fields.
Data availability statement
The original contributions presented in the study are included in the article, further inquiries can be directed to the corresponding author.
Author contributions
S-YC: Conceptualization, Formal Analysis, Investigation, Methodology, Project administration, Validation, Writing–original draft. Y-FY: Conceptualization, Data curation, Formal Analysis, Investigation, Methodology, Project administration, Writing–original draft. Y-FL: Funding acquisition, Methodology, Writing–review and editing, Formal Analysis, Investigation, Resources. GW: Formal Analysis, Funding acquisition, Resources, Writing–review and editing. YY: Funding acquisition, Methodology, Resources, Writing–review and editing. H-YD: Funding acquisition, Resources, Writing–review and editing. F-LC: Writing–review and editing. H-WY: Investigation, Methodology, Writing–original draft. C-YZ: Investigation, Methodology, Writing–original draft, Writing–review and editing. Y-LW: Funding acquisition, Resources, Writing–review and editing. Z-WL: Funding acquisition, Resources, Writing–review and editing.
Funding
The author(s) declare that financial support was received for the research, authorship, and/or publication of this article. This work was supported by the Key Laboratory Fund Project (Grant No. 2021JCJQLB055004), 173 Project Technical Fund (JSLY-16-B16006), the Central Government Guides Local Funds for Scientific and Technological Development (236Z1813G), National Natural Science Foundation of China (Grant No. 62004059), Natural Science Foundation of Hebei Province (Grant Nos F2021202047 and F2021202002), Funding Projects for the Introduction of Overseas Staff of Hebei Province (Grant No. C20210334), and the Natural Science Research Foundation of Hebei University of Technology (JBKYXX2203).
Acknowledgments
The authors thank Dr. Zhen-Guo Zhang, Hebei University of Technology, for his valuable support.
Conflict of interest
Authors H-YD were employed by Hebei Thahoo Photoelectric Technology Co. Ltd.
The remaining authors declare that the research was conducted in the absence of any commercial or financial relationships that could be construed as a potential conflict of interest.
Publisher’s note
All claims expressed in this article are solely those of the authors and do not necessarily represent those of their affiliated organizations, or those of the publisher, the editors and the reviewers. Any product that may be evaluated in this article, or claim that may be made by its manufacturer, is not guaranteed or endorsed by the publisher.
References
1. Fuhrberg P, Ahrens A, Schkutow A, Frick T. 2.0 μm Laser Transmission Welding: welding of transparent and opaque polymers with single-mode Tm-doped fiber lasers. PhotonicsViews (2020) 17(2):64–8. doi:10.1002/phvs.202000013
2. Scholle K, Schaefer M, Lamrini S, Fuhrberg P, Wysmolek M, Steinke M. All-fiber linearly polarized high power 2-µm single mode Tm-fiber laser for plastic processing and Ho-laser pumping applications. In: AL Carter, and I Hartl, editors. Fiber lasers XV: Technology and systems. France: SPIE (2018). p. 23. doi:10.1117/12.2289957
3. White CJ, Ramee SR, Collins TJ, Mesa JE, Murgo JP. Holmium: YAG laser-assisted coronary angioplasty with multifiber delivery catheters. Cathet Cardiovasc Diagn (1993) 30(3):205–10. doi:10.1002/ccd.1810300305
4. Jou YC, Shen CH, Cheng MC, Lin CT, Chen PC. High-power holmium: yttrium-aluminum-garnet laser for percutaneous treatment of large renal stones. Urology (2007) 69(1):22–5. doi:10.1016/j.urology.2006.08.1114
5. Ebert V, Teichert H, Strauch P, Kolb T, Seifert H, Wolfrum J. Sensitive in situ detection of CO and O2 in a rotary kiln-based hazardous waste incinerator using 760 nm and new 2.3 μm diode lasers. Proc Combust Inst (2005) 30(1):1611–8. doi:10.1016/j.proci.2004.08.224
6. Chao X, Jeffries JB, Hanson RK. Absorption sensor for CO in combustion gases using 2.3 µm tunable diode lasers. Meas Sci Technol (2009) 20(11):115201. doi:10.1088/09570233/20/11/115201
7. Theisen D, Ott V, Bernd HW, Danicke V, Keller R, Brinkmann R. CW high-power IR laser at 2 μm for minimally invasive surgery. Washington, DC, USA: Optica Publishing Group (2003). p. 96. doi:10.1117/12.500624
8. Encai J, Mingming N, Qiang L. 13.5 mJ polarized 2.09 μm fiber-bulk holmium laser and its application to a mid-infrared ZnGeP2 optical parametric oscillator. Chin Opt Lett (2017) 15(9):091402. doi:10.3788/COL201715.091402
9. Yan BZ, Mu XK, An JS, Qi YY, Ding J, Bai ZX, et al. Advances in 2 μm single-longitudinal-mode all-solid-state pulsed lasers (cover paper invited). Infrared Laser Eng (2024) 53(2):20230730. doi:10.3788/IRLA20230730
10. Li PF, Zhang F, Li K, Cai C, Li Y, Zhang JC, et al. Research progress of high-frequency and high-energy solid state lasers at 1.6 µm (invited). Infrared Laser Eng (2023) 52(8):20230403. doi:10.3788/IRLA20230403
11. Cui Q, Shu X, Le X, Zhang X. 70-W average-power doubly resonant optical parametric oscillator at 2 μm with single KTP. Appl Phys B (2014) 117(2):639–43. doi:10.1007/s00340-014-5877-z
12. Arisholm G, Lippert E, Rustad G, Stenersen K. Efficient conversion from 1 to 2 µm by a KTP-based ring optical parametric oscillator. Opt Lett (2002) 27(15):1336–8. doi:10.1364/OL.27.001336
13. Geng YF, Tan XL, Li XJ, Yao JQ. Compact and tunable mid-infrared source based on a 2 μm dual-wavelength KTiOPO4 intracavity optical parametric oscillator. Chin Phys B (2010) 19(11):114209. doi:10.1088/1674-1056/19/11/114209
14. Mei J, Zhong K, Wang MR, Liu PX, Xu DG, Wang YY, et al. High-Repetition-Rate terahertz generation in QPM GaAs with a compact efficient 2-μm KTP OPO. IEEE Photon Technol Lett (2016) 28(14):1501–4. doi:10.1109/LPT.2016.2557583
15. Chen T, Jiang P, Yang D, Hu C, Wu B, Shen Y. High-power PPMgLN-based optical parametric oscillator pumped by a linearly polarized, semi-fiber-coupled acousto-optic Q-switched fiber master oscillator power amplifier. Appl Opt (2013) 52(25):6316. doi:10.1364/AO.52.006316
16. Kostyukova NY, Erushin EY, Boyko AA, Kolker DB. Radiation source based on an optical parametric oscillator with MgO: PPLN crystal and volume Bragg grating, tunable in ranges of 2050 – 2117 and 2140 – 2208 nm. Quan Electron (2022) 52(2):144–8. doi:10.1070/QEL17981
17. Wang X, Jia K, Chen M, Cheng S, Ni X, Guo J, et al. 2 μm optical frequency comb generation via optical parametric oscillation from a lithium niobate optical superlattice box resonator. Photon Res (2022) 10(2):509–15. doi:10.1364/PRJ.432076
18. Zhu H, Duan Y, Feng Z, Zhang G, Xu C, Zhang Y, et al. Generation of 2-μm light based on a noncritical phase matching OPO technique. IEEE Photon Technol Lett (2013) 25(7):690–3. doi:10.1109/LPT.2013.2247395
19. Mei J, Zhong K, Liu Y, Shi J, Qiao H, Xu D, et al. Compact, efficient and widely tunable 2- μm high-repetition-rate optical parametric oscillators. Opt Commun (2018) 426:119–25. doi:10.1016/j.optcom.2018.05.031
20. Li D, Yu Y, Li Y, Wang Y, Liu H, Jin G-Y. Narrow linewidth 2.1 μm optical parametric oscillator with intra-cavity configuration based on wavelength-locked 878.6 nm in-band pumping. Op Laser Technol (2020) 131:106412. doi:10.1016/j.optlastec.2020.106412
Keywords: 2 μm, KTP, optical parametric oscillator, side-pump, 1064 nm
Citation: Chen S-Y, Yang Y-F, Li Y-F, Wang G, Yu Y, Du H-Y, Cao F-L, Yin H-W, Zhu C-Y, Wang Y-L and Lu Z-W (2024) Efficient 10 ns-scale 2-μm optical parametric oscillator based on 1064 nm pump source. Front. Phys. 12:1383254. doi: 10.3389/fphy.2024.1383254
Received: 07 February 2024; Accepted: 15 February 2024;
Published: 01 March 2024.
Edited by:
Xiaoming Duan, Harbin Institute of Technology, ChinaCopyright © 2024 Chen, Yang, Li, Wang, Yu, Du, Cao, Yin, Zhu, Wang and Lu. This is an open-access article distributed under the terms of the Creative Commons Attribution License (CC BY). The use, distribution or reproduction in other forums is permitted, provided the original author(s) and the copyright owner(s) are credited and that the original publication in this journal is cited, in accordance with accepted academic practice. No use, distribution or reproduction is permitted which does not comply with these terms.
*Correspondence: Yun-Fei Li, eWZsaUBoZWJ1dC5lZHUuY24=
†These authors have contributed equally to this work and share first authorship