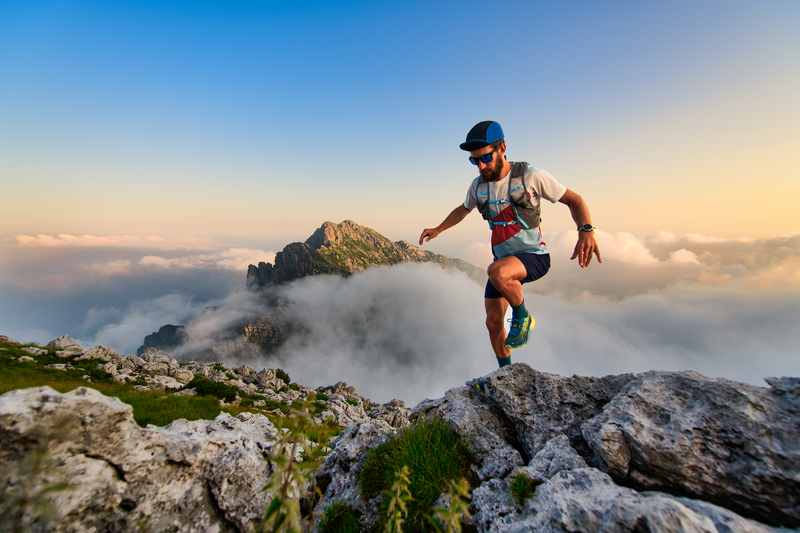
95% of researchers rate our articles as excellent or good
Learn more about the work of our research integrity team to safeguard the quality of each article we publish.
Find out more
MINI REVIEW article
Front. Phys. , 14 March 2024
Sec. Optics and Photonics
Volume 12 - 2024 | https://doi.org/10.3389/fphy.2024.1380905
This article is part of the Research Topic Advances in Infrared Lasers and Their Applications View all 9 articles
An external-cavity wavelength-swept laser, characterized by its exceptional temporal coherence and extensive tuning range, serves as a crucial light source for cutting-edge fields such as fiber sensing, lidar, and spectroscopy. The burgeoning growth of optical communication technology has escalated the demand for lasers with narrow linewidth and broad tuning range, thereby catalyzing the swift advancement of external-cavity wavelength-swept diode lasers and their diverse applications. This article comprehensively presents the configurations and operating principles of these lasers, and provides an in-depth review of their development status, specifically focusing on those with narrow linewidth and wide tuning range. The aim is to offer a valuable reference for researchers involved in the development and application of wavelength-swept lasers.
Narrow-linewidth lasers with extensive spectral tunable ranges are indispensable for a variety of applications, including quantum optics, molecular physics, gas detection, and space detection [1–4]. However, achieving a balance between narrow linewidth and tunable wavelength continues to pose a significant challenge in the field of laser technology. Currently, prevalent strategies for attaining laser output with these characteristics encompass the use of spectral selection devices (viz. gratings, prisms, etc.) and nonlinear optical frequency conversion (viz. Raman scattering, optical parametric oscillator/amplifier, etc.) [3–8]. Semiconductor-based wavelength-swept lasers, such as external-cavity tunable lasers (ECTLs), distributed Bragg reflector lasers (DBRs), and distributed feedback lasers (DFBs), are renowned for their highly controllable emission properties. These lasers have become indispensable in a multitude of applications, including distributed optical fiber sensing [9–11], atomic and molecular laser spectroscopy [12, 13], reconfigurable optical add/drop multiplexing systems [14], trace gas analysis [15], laser lidar [16], space strontium optical clocks [17, 18], and laser cooling [19]. The swift progression and application of these lasers have led to heightened requirements for linewidth and tunable range. Compared to semiconductor-based wavelength-swept lasers operating with internal-cavity feedback, ECTLs boast prestigious characteristics such as narrower linewidth, wider tuning range without mode-hopping, higher signal-to-source spontaneous emission ratio, and lower cost, making them one of the most versatile measuring tools. Consequently, research into narrow linewidth and wide tuning range ECTLs has emerged as a hot topic in recent years [20–22]. Over the past few decades, synchronous tuning with mode matching and mode selection has matured, leading to constant updates in the configurations of external-cavity wavelength-swept lasers [23, 24].
This article reviews typical external-cavity configurations of wavelength-swept lasers, summarizing the characteristics, operating theories, and development status of each ECTL. Finally, it provides a summary and an outlook for the future development of narrow linewidth and wide tuning range external-cavity wavelength-swept lasers.
In the realm of optical communication networks and other fields, there exist at least four distinct configurations of the wavelength-swept laser. One such configuration involves the use of a microring resonator (MRR) external-cavity, which can adjust its resonance wavelength through the thermo-optic effect (i.e., thermal control) or the carrier-dispersion effect (i.e., electrical control). Typically, an MRR-based external-cavity configuration comprises a semiconductor optical amplifier (SOA), a phase-adjustment region, and a double-microring optical waveguide structure with unequal radii, which can be based on silicon (Si), silicon oxynitride (SiON), or silicon nitride (Si3N4) [25–27]. Figure 1A highlights a schematic drawing of the MRR-based external-cavity configuration. The main mode-selection element in the external-cavity is the superimposed spectrum of two MRR spectra with different free spectral ranges (FSRs), which facilitates wavelength tuning and linewidth narrowing. The Vernier spectrum FSRVernier shaped by the double-microring resonators can be described as follows [28]:
where FSRm is FSR of the mth ring. When the double-microring optical waveguide undergoes synchronous tuning, the output spectra maintain alignment and shift collectively as described in Eq. 1, thereby achieving a continuous wavelength-tunable output.
FIGURE 1. (A) Schematic drawing of the MRR-based external-cavity configuration, (B) configuration of the filter-type external-cavity tunable laser, (C) the Littman-Metcalf cavity configuration, and (D) the Littrow cavity configuration.
The subsequent configuration involves filter-based tunable external-cavity semiconductor lasers that incorporate an optical filter component within the external lasing cavity. Typical filter components encompass the Fabry-Perot interferometer (FPI) [29], nematic liquid crystal [30], birefringence filter [31], acousto-optic tunable filter (AOTF) [32], interference filter (IF) [33], electro-optic tunable filter [34], among others. A general structure diagram is depicted in Figure 1B. The principle of outputting different lasing wavelengths is predicated on the shift in the position of the maximum transmission peak wavelength. This can be achieved by altering the external angle of incidence, the external medium refractive index, or the FSR of the periodic comb spectrum. Concurrently, through the precise mode selection of the chosen optical filter component and a flexible increase in effective cavity length, the spectral linewidth can be further narrowed.
As depicted in Figure 1C, another configuration is the Littman-Metcalf cavity. The primary components of the Littman-Metcalf cavity encompass the gain chip, the collimating lens, the diffraction grating, and the tuning mirror. The diffraction grating, which includes transmission [35], reflection [36], and blazed gratings [37, 38], functions as the principal mode selection element, facilitating wavelength tuning and spectral linewidth narrowing. Light emanating from the gain chip impinges on the diffraction grating, which spatially segregates the spectral components of the gain chip output. The mode-selective effect of the diffraction grating can be expressed by a function associated with its equivalent reflectance RG. The output of the Littman-Metcalf configuration is the coupled effect of the diffraction grating, external-cavity, and internal-cavity. Consequently, the output mode structure, EMS, can be described as follows [39]:
where TG, GIC, GEC, E0 denotes the equivalent transmittance of the diffraction grating, the gain of the internal-cavity, the gain of the external-cavity, the initial energy, respectively. By rotating the tuning mirror, the wavelength is tuned. The linewidth of the Littman-Metcalf cavity is given by the formula [40]:
where
The final configuration is the Littrow cavity shown in Figure 1D. In this geometry, the tuning mirror is removed compared to the Littman-Metcalf cavity. The lasing wavelength is tuned by rotating the diffraction grating and thereby changing the wavelength of the optical feedback. The output wavelength that satisfies the resonant condition is determined by Eqs 4, 5, respectively [41]:
where L denotes the effective cavity length, q denotes a positive integer,
where
Owing to the combination of the availability of complementary metal oxide semiconductor (CMOS) fabrication technology and high index contrast. Thus, the silicon photonics (SiPh) dual MRRs ECTLs with a wide tuning range and narrow linewidth have attracted increasing research attention. In 2009, the first ECTL fabricated with SiPh wire waveguides on a silicon-on-insulator (SOI) substrate was demonstrated by Chu et al. [25], achieving a maximum tuning span of 38 nm in the 1,530 nm–1,565 nm (namely, C-band) or 1,565 nm–1,610 nm (namely, L-band) and a side-mode suppression ratio (SMSR) more than 30 dB along the whole tuning range. Using a similar hybrid silicon platform, in 2013, Hulme et al. designed and fabricated dual intra-cavity ring resonators ECTL [43]. The laser has a thermal tuning range of more than 40 nm, the SMSR greater than 35 dB, and the minimum on-chip output power of 0.45 mW. Meanwhile, they measured the linewidth based on the delayed self-heterodyne method [44], and the linewidth value was calculated to be 338 kHz. In 2018, Guan et al. conducted a study on the III-V/silicon hybrid ECTL based on a SOI platform [26]. The corresponding experimental results showed that the ECDL can be tuned from 1,515 nm to 1,575 nm with a SMSR in excess of 46 dB, the linewidth was as narrow as 37 kHz, and the maximal relative intensity noise (RIN) was better than −135 dB/Hz. However, due to the large linear-propagation loss and large thermo-optic coefficient of the Si material, it is difficult to further improve linewidth characteristics. Compared with Si-based material, the Si3N4-based materials are advantageous in terms of low linear-propagation loss and small thermo-optic coefficient, which are conductive to narrowing the linewidth. Later that same year, Yi et al. reported a hybrid photonic integrated ECTL based on Si3N4 MRRs [27]. Experiments have proved that the fine-tuning of the lasing wavelength can be realized by changing the temperature of the phase section. In this study, the tuning range of the ECTL was approximately 50 nm, with a SMSR exceeding 50 dB, and the narrowest linewidth measured was 35 kHz. In 2021, a novel InP-Si3N4 dual laser module was demonstrated by Dass et al. [45], each of which was fabricated using hybrid coupling of an InP-based SOA and a low loss Si3N4 feedback circuit. This work presented an ECTL which can be tuned over 100 nm while maintaining the SMSR greater than 50 dB with a RIN of about −160 dB/Hz. In 2023, Chen et al. reported a hybrid integrated Si3N4 ECTL with full C-band wavelength tenability and narrow-linwidth output [46]. In this study, the InP gain chip was coupled with the Si3N4 dual micro-ring, then integrated with the AlGaInAs quantum well rib waveguide SOA through the dual-collimating lens coupling. A wavelength tuning range of 55 nm was obtained, the SMSR over 50 dB was measured, and the linewidth was narrower than 8 kHz.
Compared with the above mentioned ECTLs that use dual MRRs as mode-selection component, the filter-type-ECTL provides the advantage of higher flexibility in optical filters. In 1993, Choi et al. verified that piezoelectric ceramics (PZT) can fine-tune the effective length of the external-cavity to obtain the tuning of the lasing wavelength [47]. Similarly, the filter-type-ECTLs are tuned by changing the effective length of the external-cavity or rotating the filter. In 2011, Zhang et al. reported their work on ECTL, which utilized two etalons in the external-cavity to realize narrow-linewidth and wide tuning output [48]. A tuning range of about 40 nm with the linewidth less than 100 kHz was measured. In 2012, Thompson et al. carried out a study on wide-bandwidth filter-type-ECTL [49]. The linewidth of the ECTL was around 26 kHz, and the tuning range exceeded 14 nm. In 2017, Kasai et al. demonstrated a long external-cavity structure with a tunable optical filter [33]. The coarse and fine wavelength-tuning can be obtained by simultaneously changing the peak transmittance wavelength of the multi-layered dielectric interference filter and the length of the AR-coated SiO2 plate. The lasing wavelength was successfully tuned over 40 nm without mode hopping in the full C or L-bands, and the linewidth of less than 8 kHz and 7.7 kHz were measured, respectively. In 2020, Zhang et al. used a bandwidth of 0.48 nm IF with a peak transmittance of up to 96% to adjust the lasing wavelength [17]. It had a coarse wavelength tuning range over 40 GHz by current-controlled method, a fine tuning range over 3 GHz by PZT-controlled method, and the output linewidth was about 180 kHz. In the same year, Magdich et al. reported an ECTL with a linear external resonator and two AOTFs [32]. This study indicated that this optical scheme can significantly compress the output spectral linewidth in both sweep and stationary modes. A minimum linewidth of 0.022 nm can be achieved when the tuning rate of the AOTF reaches 104 nm/s, with a maximum tuning range of 64 nm.
The Littman-Metcalf-type ECTL provides the advantageous of a wider tuning range, and a narrower linewidth in the order of hundreds of kilohertz. Unfortunately, the introduction of additional optical components reduces the flexibility of the external-cavity structure. In 1978, Littman et al. designed the Littman external-cavity structure for the first time and used it in dye lasers [50]. After decades of development, this configuration has been widely used in ECTL, and has become a mainstream structure. In 2003, Jin et al. reported a narrow-band ECTL using a classical Littman-Metcalf structure [51]. The continuously tuning in wavelength region of 797.38 nm ∼ 807.26 nm was obtained, and the linewidth is smaller than 0.06 nm. In 2012, Zhang et al. presented a compact ECTL based on a simple single-axis-MEMS mirror to boost tuning speed and compress linewidth [52]. A wide tuning range about 40 nm with a narrow linewidth of less than 50 kHz was obtained, and its tuning speed can reach the order of kilohertz. In 2013, Wei et al. adopted a star-flexure hinge as the tuning mechanism to construct the ECDL [53]. Its external-cavity structure parameters were calculated and optimized according to Eq. 2, showing tuning range over 80 GHz without mode-hopping, and the linewidth of 200 kHz. In 2017, Jiménez et al. proposed a micro-packing ECTL which overcome the drawbacks of traditional ECTL [54]. The experimental results showed that the laser provided a below 100 kHz output linewidth, and the SMSR can reach 60 dB. However, the lasing wavelength can only be slightly changed by current-controlled, which limited the tuning range of GHz-level. In 2018, Shirazi et al. adopted a transmissive mode selection element to develop an ECTL [35]. The output laser beam derived from the transmitted light of the diffraction grating. A tuning range of 52 nm was realized. In 2021, the ECTL was modeled to study the longitudinal allowance error for mode-hopping free tuning by our group [55]. The influence of the distance from pivot point to motion axis of PZT motor and the diffracting point of the diffraction grating, the installation angle of the diffraction grating, and the grating groove density were analyzed, respectively. The results showed that mode-hopping can be effectively avoided with careful choosing the parameters above. Based on the above theoretical analysis results, in 2023, our group reported a narrow linewidth ECTL without mode-hopping and experimentally studied its tuning characteristics [38]. The ECDL achieved a continuous wavelength tuning range of 100 nm from 1,480 nm to 1,580 nm with no mode-hopping, with a SMSR of more than 65.54 dB, and with a linewidth of less than 98.27 kHz. Besides, mode compensation [56] and/or roof prisms [57] have been used to improve the performance of the ECTL.
Compared with the Littman-Metcalf-type ECTL, the difference is that the Littrow cavity selects the resonant frequency only once using the mode selection component. Therefore, the linewidth of this type is relatively wide, the output power is relatively high, and the ability to resist the influence of external noise is relatively strong. In 1969, Hard designed the first Littrow configuration for lasing frequency selection [58]. After nearly 55 years of development, impressive results have been achieved. In 2007, Guan et al. reported an ECTL based on Littrow configuration [59]. By changing the angle of the mode selection component, wavelength of the ECTL can be tuned from 775 nm to 785 nm with the output linewidth of less than 2.5 MHz. In 2016, A single-mode frequency-stabilized by frequency locking method ECTL was proposed by Bayrakli, which was locked to a FPI with a FSR of 1.5 GHz [60]. By rotating the diffraction grating, the ECTL can obtain a coarse wavelength-tuning of 135 nm, linewidth of less than 2.9 kHz. However, the main disadvantage of the Littrow-type ECTL is that the output beam steers as the mode selection component is rotated. In 2018, Guo et al. designed a new and enhanced configuration for mirror-grating Littrow-type ECTL, which had the characteristics of the large mode-hop-free tuning range and the direction of the output beam was basically unchanged [61]. The experiment showed that the continuous tuning range of the ECTL reached 4.34 nm operating at 805 nm, with a 0.033 mm lateral displacement. In 2019, Wang et al. constructed the improved ECTLs to investigate the relationship between the grating features and the SMSR, which outputted from the rear face of the commercial gain chip keeping the direction unchanged [62]. The ECTL can achieve a tunable range of 209.9 nm, SMSR of more than 65 dB, and output power of 48.9 mW. In 2021, Giraud et al. adopted the AR-coated interband cascade laser as the gain chip to develop the improved Littrow-type ECTL [63]. A continuous tuning range of 360 nm from 3,220 nm to 3,580 nm with a maximum output power of 13 mW was obtained at 293 K. In addition, in order to reduce the influence of the internal-cavity mode, some scholars began to use ridge waveguide gain chip instead of straight waveguide [64–66]. Meanwhile, by translating the collimating lens, the tuning of the Littrow-type ECTL can also be realized [67].
In conclusion, owing to their exceptional output characteristics, such as narrow linewidth and wide tuning range, ECTLs can cater to the needs of numerous applications. However, to adapt to diverse application scenarios, it is crucial to analyze and summarize the typical configurations of ECTLs, as shown in Table 1. Currently, the MRR-ECTL, with its balanced output characteristics and high integration, is witnessing rapid development and finds the most extensive application among ECTLs. The Littman-Metcalf-type ECTL is also frequently employed as a primary structural design due to its superior spectral resolution. Concurrently, it is essential to explore strategies to mitigate the challenges associated with coupling difficulty, alignment, and overall system size. A noteworthy point is the long-term stability, which will inevitably influence the development trajectory of ECTLs. Furthermore, the task of calibrating parameters for narrow linewidth wavelength tunable lasers presents a substantial challenge in the current progression of laser technology [68–71]. This has been one of the key catalysts in the swift advancement of laser linewidth, noise, and stability measurement technologies over recent decades.
LS: Writing–review and editing, Writing–original draft, Funding acquisition. JW: Writing–review and editing, Conceptualization. LH: Writing–review and editing, Supervision, Investigation, Funding acquisition. AZ: Writing–review and editing, Supervision, Investigation. ZZ: Writing–review and editing, Investigation. SQ: Writing–review and editing, Supervision, Investigation. YW: Writing–review and editing, Investigation. ZL: Writing–review and editing, Resources, Investigation, Funding acquisition. JJ: Writing–review and editing, Supervision, Investigation. SZ: Writing–review and editing, Supervision, JL: Writing–review and editing, Supervision, Funding acquisition. JH: Writing–review and editing, Investigation. HJ: Writing–review and editing, Supervision, Investigation.
The authors declare financial support was received for the research, authorship, and/or publication of this article. This research was funded by the National Key Research and Development Plan (2022YFF0705900 and 2022YFF0707102).
Authors LS, JW, LH, AZ, ZZ, SQ, YW, ZL, JJ, SZ, JL, JH, and HJ were employed by Ceyear Technologies Co., Ltd.
All claims expressed in this article are solely those of the authors and do not necessarily represent those of their affiliated organizations, or those of the publisher, the editors and the reviewers. Any product that may be evaluated in this article, or claim that may be made by its manufacturer, is not guaranteed or endorsed by the publisher.
1. Chen H, Babin F, Leblanc M, Schinn G. Widely tunable single-frequency erbium-doped fiber lasers. IEEE Photon Tech Lett (2003) 15(2):185–9. doi:10.1109/LPT.2002.806833
2. Bai Z, Zhao Z, Tian M, Jin D, Pang Y, Li S, et al. A comprehensive review on the development and applications of narrow-linewidth lasers. Microwave Opt Tech Lett (2022) 64(12):2244–55. doi:10.1002/mop.33046
3. Yang X, Bai Z, Chen D, Chen W, Feng Y, Mildren R. Widely-tunable single-frequency diamond Raman laser. Opt Express (2021) 29(18):29449–57. doi:10.1364/OE.435023
4. Chen H, Cui Y, Li X, Zhang B, Cai Y, Ding J, et al. High-power dual-wavelength intracavity diamond Raman laser. Funct Diamond (2023) 3(1):2282527. doi:10.1080/26941112.2023.2282527
5. Zheng H, Zhao C, Zhang F, Li P, Yan B, Wang Y, et al. Study on the longitudinal mode characteristic of idler wave in MgO:PPLN infrared optical parametric oscillator. Infrared Laser Eng (2023) 52(12):20230378. doi:10.3788/IRLA20230378
6. Lang X, Jia P, Chen Y, Qin L, Liang L, Chen C, et al. Advances in narrow linewidth diode lasers. Sci China Inf Sci (2019) 62:61401. doi:10.1007/s11432-019-9870-0
7. Corato-Zanarella M, Gil-Molina A, Ji X, Shin MC, Mohanty A, Lipson M. Widely tunable and narrow-linewidth chip-scale lasers from near-ultraviolet to near-infrared wavelengths. Nat Photon (2023) 17(2):157–64. doi:10.1038/s41566-022-01120-w
8. Wang R, Wang N, Teng H, Wei Z. High-power tunable narrow-linewidth Ti: sapphire laser at repetition rate of 1 kHz. Appl Opt (2012) 51(22):5527–30. doi:10.1364/AO.51.005527
9. Sheng L, Huang L, Yan J, Qiao S, Zhang A, Jin H, et al. Distributed multi-parameter sensing based on the Brillouin scattering effect in orbital angular momentum guiding fiber. Opt Continuum (2022) 1(1):133–42. doi:10.1364/OPTCON.446952
10. Xu P, Yu X, Chen Z, Sheng L, Liu J, Zhou S, et al. Distributed refractive index sensing based on bending-induced multimodal interference and Rayleigh backscattering spectrum. Opt Express (2021) 29(14):21530–8. doi:10.1364/OE.430637
11. Sheng L, Li L, Liu L, Hu L, Yuan M, Yan J. Study on the simultaneous distributed measurement of temperature and strain based on Brillouin scattering in dispersion-shifted fiber. OSA Continuum (2020) 3(8):2078–85. doi:10.1364/OSAC.398410
12. Robins N, Lance A, Close J, Sheng L, Liu J, Zhou S, et al. Piezo-locking a diode laser with saturated absorption spectroscopy. Appl Opt (2008) 47(28):5163–6. doi:10.1364/AO.47.005163
13. Lv Z, Liu Z, Chen H, Jin D, Hao X, Fan W, et al. Review of multi-wavelength laser technology based on crystalline Raman conversion (invited). Infrared Laser Eng (2023) 52(8):20230420. doi:10.3788/IRLA20230420
14. Ma Y, Yang Q, Tang Y, Chen S, Shieh W. 1-Tb/s single-channel coherent optical OFDM transmission over 600-km SSMF fiber with subwavelength bandwidth access. Opt Express (2009) 17(28):9421–7. doi:10.1364/OE.17.009421
15. Centeno R, Marchenko D, Mandon J, Cristescu S, Wulterkens G, Harren F. High power, widely tunable, mode-hop free, continuous wave external cavity quantum cascade laser for multi-species trace gas detection. Appl Phys Lett (2014) 105(26):261907. doi:10.1063/1.4905281
16. Shi G, Wang W, Zhang F. Precision improvement of frequency-modulated continuous-wave laser ranging system with two auxiliary interferometers. Opt Commun (2018) 411:152–7. doi:10.1016/j.optcom.2017.11.062
17. Zhang L, Liu T, Chen L, Xu G, Jiang C, Liu J, et al. Development of an interference filter-stabilized external-cavity diode laser for space applications. Photonics (2020) 7(12):7010012. doi:10.3390/photonics7010012
18. Schioppo M, Brown R, McGrew W, Hinkley N, Fasano R, Beloy K, et al. Ultrastable optical clock with two cold-atom ensembles. Nat Photon (2017) 11(48):48–52. doi:10.1038/NPHOTON.2016.231
19. Yang X, Yin Y, Li X, Sheng L, Liu J, Zhou S, et al. External cavity diode laser as a stable-frequency light source for application in laser cooling of molecules. Chin Opt Lett (2016) 14(7):071403. doi:10.3788/COL201614.071403
20. Gong H, Liu Z, Zhou Y, Zhang W. Extending the mode-hop-free tuning range of an external-cavity diode laser by synchronous tuning with mode matching. Appl Opt (2014) 53(33):7878–84. doi:10.1364/AO.53.007878
21. Gao Y, Lo J, Lee S, Patel R, Zhu L, Nee J, et al. High-power, narrow-linewidth, miniaturized silicon photonic tunable laser with accurate frequency control. J Lightwave Technol (2020) 38(2):265–71. doi:10.1109/JLT.2019.2940589
22. Zhu Y, Liu Z, Zhang X. Command-shaping based on impulse response function for dynamic-mode control of internal and external cavities in external-cavity diode laser. Rev Sci Instrum (2020) 91(2):023101. doi:10.1063/1.5117784
23. Zhang A, Qiao S, Sheng L, Huang L, Liu Z, Ju J, et al. Study on external cavity diode laser with a wide mode-hopping free tuning range. Front Phys (2022) 10:1093179. doi:10.3389/fphy.2022.1093179
24. Duca L, Perego E, Berto F, Sias C. Design of a Littrow-type diode laser with independent control of cavity length and grating rotation. Opt Lett (2021) 46(12):2840–3. doi:10.1364/OL.423813
25. Chu T, Fujioka N, Ishizaka M. Compact, lower-power-consumption wavelength tunable laser fabricated with silicon photonic-wire waveguide micro-ring resonators. Opt Express (2009) 17(16):14063–8. doi:10.1364/OE.17.014063
26. Guan H, Novack A, Galfsky T, Ma Y, Fathololoumi S, Horth A, et al. Widely-tunable, narrow-linewidth III-V/silicon hybrid external-cavity laser for coherent communication. Opt Express (2018) 26(7):7920–33. doi:10.1364/OE.26.007920
27. Lin Y, Browning C, Timens R, Geuzebroek D, Roeloffzen C, Hoekman M, et al. Characterization of hybrid InP-TriPleX photonic integrated tunable lasers based on silicon nitride (Si3N4/SiO2) microring resonators for optical coherent system. IEEE Photon J (2018) 10(3):1–8. doi:10.1109/JPHOT.2018.2842026
28. Tran M, Huang D, Guo J, Komljenovic T, Morton P, Bowers J. Ring-resonator based widely-tunable narrow-linewidth Si/InP integrated lasers. IEEE J Selected Top Quan Elect (2020) 26(2):1–14. doi:10.1109/JSTQE.2019.2935274
29. Zhu J, Qiao D, Jones A, Zhang B, Li K, Copner N. 1.7 THz tuning range pivot-point-independent mode-hop-free external cavity diode laser. Opt Express (2023) 31(3):3970–83. doi:10.1364/OE.478580
30. Mizutani K, Merlier J, Sudo S, Sato K, Kudo K. Liquid crystal mirror-based wavelength-tunable laser module with asynchronous mode cavity. IEEE Photon Technol Lett (2006) 18(12):1299–301. doi:10.1109/LPT.2006.876750
31. Alfieri C, Waldburger D, Nürnberg J, Golling M, Jaurigue L, Lüdge K, et al. Mode-locking instabilities for high-gain semiconductor disk lasers based on active submonolayer quantum dots. Phys Rev Appl (2018) 10(4):044015. doi:10.1103/PhysRevApplied.10.044015
32. Magdich L, Chamorovskiy A, Shidlovsky V, Shramenko M, Yakubovich S. Tunable semiconductor laser with two acousto-optic tunable filters in its external cavity. Quan Electron (2020) 50(2):136–40. doi:10.1070/QEL17180
33. Kasai K, Nakazawa M, Tomomatsu Y, Endo T. 1.5μm, mode-hop-free full C-band wavelength tunable laser diode with a linewidth of 8 kHz and a RIN of -130 dB/Hz and its extension to the L-band. Opt Express (2017) 25(18):22113–24. doi:10.1364/OE.25.022113
34. Ménager L, Cabaret L, Lorgeré I, Gouët L. Diode laser extended cavity for broad-range fast ramping. Opt Lett (2000) 25(17):1246–8. doi:10.1364/OL.25.001246
35. Shirazi M, Kim P, Jeon M, Kim C, Kim J. Free space broad-bandwidth tunable laser diode based on Littman configuration for 3D profile measurement. Opt Laser Tech (2018) 101:462–7. doi:10.1016/j.optlastec.2017.11.035
36. Podoskin A, Golovin V, Gavrina P, Veselov D, Zolotarev V, Shamakhov V, et al. Ultrabroad tuning range (100 nm) of external-cavity continuous-wave high-power semiconductor lasers based on a single InGaAs quantum well. Appl Opt (2019) 58(33):9089–93. doi:10.1364/AO.58.009089
37. Kapasi D, Eichholz J, McRae T, Ward R, Slagmolen B, Legge S, et al. Tunable narrow-linewidth laser at 2 μm wavelength for gravitational wave detector research. Opt Express (2020) 28(3):3280–8. doi:10.1364/OE.383685
38. Sheng L, Ge C, Cao Q, Huang L, Zhao Z, Li L, et al. Wide-range external-cavity tunable semiconductor laser with mode-hopping free. Infrared Laser Eng (2023) 52(8):20230374. doi:10.3788/IRLA20230374
39. Gong H, Liu Z, Zhou Y, Zhang W, Lv T. Mode-hopping suppression of external cavity diode laser by mode matching. Appl Opt (2014) 53(4):694–701. doi:10.1364/AO.53.000694
40. Zhou P, Wu Y, Zhang R. Effect of collimating lens misalignment on linewidth of Littman-Metcalf grating external cavity laser. Infrared Laser Eng (2022) 51(4):20210168. doi:10.3788/IRLA2010168
41. Labachelerie M, Sasada H, Passedat G. Mode-hop suppression of Littrow grating-tuned lasers: erratum. Appl Opt (1994) 33(18):3817–9. doi:10.1364/AO.33.003817
42. Long R, Wang H, Gong Q, Song Z, Feng S. Optical feedback of semiconductor external cavity laser. Commun Technol (2013) 46(6):142–4.
43. Hulme JC, Doylend JK, Bowers JE. Widely tunable Vernier ring laser on hybrid silicon. Opt Express (2013) 21(17):19718–22. doi:10.1364/OE.21.019718
44. Bai Z, Zhao Z, Qi Y, Ding J, Li S, Yan X, et al. Narrow-linewidth laser linewidth measurement technology. Front Phys (2021) 9:768165. doi:10.3389/fphy.2021.768165
45. Dass D, Costas M, Barry L, O’Duill S, Roeloffzen C, Geuzebroek D, et al. 28 GBd PAM-8 transmission over a 100 nm range using an InP-Si3N4 based integrated dual tunable laser module. Opt Express (2021) 29(11):16563–71. doi:10.1364/OE.421929
46. Chen C, Wei F, Han X, Su Q, Pi H, Xin G, et al. Hybrid integrated Si3N4 external cavity laser with high power and narrow linewidth. Opt Express (2023) 31(16):26078–91. doi:10.1364/OE.487850
47. Choi K, Menders J, Searcy P, Korevaar E. Optical feedback locking of a diode laser using a cesium Faraday filter. Opt Commun (1993) 96(4):240–4. doi:10.1016/0030-4018(93)90268-a
48. Zhang X, Wang N, Gao L, Feng M, Chen B, Tsang Y, et al. Narrow-linewidth external-cavity tunable lasers. Proc Int Conf Opt Commun Networks (2011) 1–2. doi:10.1049/cp.2011.1322
49. Thompson D, Scholten R. Narrow linewidth tunable external cavity diode laser using wide bandwidth filter. Rev Sci Instrum (2012) 83(2):023107. doi:10.1063/1.3687441
50. Littman M, Metcalf H. Spectrally narrow pulsed dye laser without beam expander. Appl Opt (1978) 17(14):2224–7. doi:10.1364/AO.17.002224
51. Jin J, Jiao Q, Li Y, Lv F, Zhang G. Study on external-cavity semiconductor laser. Chin Opt Lett (2003) 1(3):147–8.
52. Zhang D, Zhao J, Yang Q, Liu W, Fu Y, Li C, et al. Compact MEMS external cavity tunable laser with ultra-narrow linewidth for coherent detection. Opt Express (2012) 20(18):19670–82. doi:10.1364/oe.20.019670
53. Wei F, Chen DJ, Xin G, Sun Y, Fang Z, Cai H, et al. A compact and rugged tunable external cavity diode laser with Littman-Metcalf configuration. Chin J Lasers (2013) 40(11):1102012. doi:10.3788/CJL201340.1102012
54. Jiménez A, Milde T, Staacke N, Abmann C, Carpintero G, Sacher J. Narrow-line external cavity diode laser micro-packaging in the NIR and MIR spectral range. Appl Phys B (2017) 123:207. doi:10.1007/s00340-017-6777-9
55. Sheng L, Zhang A, Qiao S, Huang L, Zhang Z, Liu Z, et al. Study of longitudinal allowance error for mode-hopping free external cavity tunable semiconductor lasers (Invited). Electro-optic Tech Applicaiton (2021) 36(5):66–72.
56. Saliba S, Junker M, Turner L, Scholten R. Mode stability of external cavity diode lasers. Appl Opt (2009) 48(35):6692–700. doi:10.1364/AO.48.006692
57. Breguet J, Henein S, Kjelberg I, Gumy M, Glettig W, Lecomte S, et al. Tunable extended-cavity diode laser based on a novel flexure-mechanism. Int J Optomechatron (2013) 7:1–6. doi:10.1109/isot.2012.6403286
58. Hard T. Laser wavelength selector and output coupler. IEEE J Quan Electron (1969) 5(6):321. doi:10.1109/JQE.1969.1082003
59. Guan H, Huang G, Huang X, Guo B, Shu H, Gao K. Construction of the tunable diode laser based on Littrow configuration. Chin J Lasers (2007) 34(1):31–4. doi:10.3321/j.issn:0258-7025.2007.01.004
60. Bayrakli I. Actively frequency-stabilized external cavity diode laser with a linewidth of 2.9 kHz. Opt Quan Elect (2016) 48(1):29. doi:10.1007/s11082-015-0305-0
61. Guo H, Olamax G. Analysis of no mode-hop tuning of mirror-grating external-cavity diode laser. Opt Commun (2018) 421:90–3. doi:10.1016/j.optcom.2018.03.074
62. Wang Y, Wu H, Chen C, Zhou Y, Wang Y, Liang L, et al. An ultra-high-SMSR external-cavity diode laser with a wide tunable range around 1550 nm. Appl Sci (2019) 9:4390. doi:10.3390/app9204390
63. Giraud E, Demolon P, Gresch T, Urio L, Muller A, Maulini R. Room-temperature continuous-wave external cavity interband cascade laser tunable from 3.2 to 3.6 µm. Opt Express (2021) 29(23):38291–7. doi:10.1364/OE.440525
64. Ojanen S, Viheriälä J, Cherchi M, Zia N, Koivusalo E, Karioja P, et al. GaSb diode lasers tunable around 2.6 μm using silicon photonics resonators or external diffractive gratings. Appl Phys Lett (2020) 116(8):081105. doi:10.1063/1.5140062
65. Kruczek T, Fedorova K, Sokolovskii G, Teissier R, Baranov A, Rafailov E. InAs/AlSb widely tunable external cavity quantum cascade laser around 3.2 μm. Appl Phys Lett (2013) 102:011124. doi:10.1063/1.4774088
66. Liu Z, Jia T, Xu X, Chu C, Zhang Y, Zhang Z. Research progress of AlGaN-based UDV μLED (invited). Infrared Laser Eng (2023) 52(8):20230390. doi:10.3788/IRLA20230390
67. Okamura H. Shift lens external-cavity diode laser for broad wavelength tuning and switching. Opt Lett (2010) 35:1175–7. doi:10.1364/OL.35.001175
68. Huang S, Zhu T, Liu M, Huang W. Precise measurement of ultra-narrow laser linewidths using the strong coherent envelope. Sci Rep (2017) 7(1):41988. doi:10.1038/srep41988
69. Jin D, Bai Z, Zhao Z, Chen Y, Fan W, Wang Y, et al. Linewidth narrowing in free-space-running diamond Brillouin lasers. High Power Laser Sci Eng (2023) 11:1–16. doi:10.1017/hpl.2023.48
70. Zhao Z, Bai Z, Jin D, Qi Y, Ding J, Yan B, et al. Narrow laser-linewidth measurement using short delay self-heterodyne interferometry. Opt Express (2022) 30(17):30600–10. doi:10.1364/OE.455028
Keywords: narrow linewidth, wide tuning range, external-cavity, wavelength-swept laser, Littman-Metcalf, Littrow
Citation: Sheng L, Wang J, Huang L, Zhang A, Zhang Z, Qiao S, Wei Y, Liu Z, Ju J, Zhou S, Liu J, Han J and Jin H (2024) Advances in narrow linewidth and wide tuning range external-cavity wavelength-swept lasers. Front. Phys. 12:1380905. doi: 10.3389/fphy.2024.1380905
Received: 02 February 2024; Accepted: 29 February 2024;
Published: 14 March 2024.
Edited by:
Quan Sheng, Tianjin University, ChinaReviewed by:
Xiaobo Hu, Zhejiang Sci-Tech University, ChinaCopyright © 2024 Sheng, Wang, Huang, Zhang, Zhang, Qiao, Wei, Liu, Ju, Zhou, Liu, Han and Jin. This is an open-access article distributed under the terms of the Creative Commons Attribution License (CC BY). The use, distribution or reproduction in other forums is permitted, provided the original author(s) and the copyright owner(s) are credited and that the original publication in this journal is cited, in accordance with accepted academic practice. No use, distribution or reproduction is permitted which does not comply with these terms.
*Correspondence: Lin Huang, aHVhbmdsaW5AY2V5ZWFyLmNvbQ==; Zhiming Liu, bHptc2t5d2VpbGFpQDE2My5jb20=
Disclaimer: All claims expressed in this article are solely those of the authors and do not necessarily represent those of their affiliated organizations, or those of the publisher, the editors and the reviewers. Any product that may be evaluated in this article or claim that may be made by its manufacturer is not guaranteed or endorsed by the publisher.
Research integrity at Frontiers
Learn more about the work of our research integrity team to safeguard the quality of each article we publish.